- 1Bird Ecology Group, Faculty of Biology, Human and Animal Biology Department, Universidad de la Habana, Havana, Cuba
- 2Wildlife Ecology Laboratory, Faculty of Forestry and Nature Conservation, Universidad de Chile, Santiago, Chile
- 3Department of Biology, Biological and Geological Sciences Building, Western University, London, ON, Canada
- 4National Botanical Garden of Cuba, Universidad de la Habana, Havana, Cuba
- 5Epidemiology Group, National Center for Animal and Plant Health (CENSA), World Organisation for Animal Health (WOAH founded as OIE) Collaborating Center for the Reduction of the Risk of Disaster in Animal Health, San José de las Lajas, Cuba
- 6Cuban Sports Hunting Federation, Havana, Cuba
- 7Science and Technology Branch, Division of Environment and Climate Change, Government of Canada, Saskatoon, SK, Canada
In recent years, the complex evolution of the highly pathogenic avian influenza (HPAI) situation reflects a change in the eco-epidemiology of the causative agent which, among other demands, renews the need for better understanding of the connectivity between countries through the main virus reservoirs to improve prevention, early warning and mitigate the associated risks. Our objective was to determine migratory connectivity of Blue-winged Teal and evaluate the risk of AIV introduction to Cuba by this species. The stable hydrogen isotope (δ2H) value in flight feathers was analyzed. Individuals were sampled during the migratory season of 2021 (N=126) and winter residence of 2020 (N=152), in western and central Cuba, respectively. Based on banding records from 1955 to 2018, the transition probabilities from 3 breeding areas in North America to 9 wintering areas was estimated with a Burnham’s live-recapture dead-recovery modeling framework. A map of likely origin in North America of the individuals sampled for each season was generated, combining the isotopic information and transition probabilities. Evidence of an age-related and phenological pattern in migratory origins was identified. Individuals harvested in the migratory season in western Cuba were most likely from molt or natal areas in the prairies and forest regions from United States and southern Canada. Alternatively, individuals harvested in winter in the center of Cuba had the most likely origin in the U.S. prairie region. The spatial-temporal pattern of AIV prevalence in the estimated region was analyzed and suggestions are made to assist the Cuban system of active AIV surveillance of wild birds.
1 Introduction
The emergence of the H5N1 subtype of highly pathogenic avian influenza (HPAI) of Asian origin in 2003 marked a dramatic change in terms of disease occurrence and distribution. Since then, billions of domestic birds have died or been culled due to HPAI (WAHIS, 2024). Currently, the global recurrence, spread and significant increase HPAI outbreaks is affecting domestic and wild birds, and some terrestrial and aquatic mammals, reflecting a distinct change in the epidemiology and ecology of the virus and posing a threat to animal health, public health, food security and biodiversity (WOAH, 2024).
The dynamics and evolution of avian influenza virus (AIV) are significantly influenced by the ecology and biogeography of its primary hosts, which are primarily wild waterfowl (Olsen et al., 2006). This virus has the capacity to infect over 90 bird species across 13 orders (Breban et al., 2009), with the most representative hosts being from the orders Anseriformes and Charadriiformes. Species within these orders are regarded as reservoirs for the virus and thus, play a crucial role in AIV maintenance and dispersion (Kilpatrick et al., 2006; Newman et al., 2012). Long-distance migration is a central trait of these species, contributing significantly to the virus spread (van Toor et al., 2018). This underscores the importance of considering wild birds and ecological processes like migration in active surveillance strategies and AIV circulation studies.
Surveillance programs are crucial for the early detection of the virus and for identifying the most likely seasons and areas for outbreaks (Bevins et al., 2014). However, investigations and monitoring have varied in intensity regionally because conducting active virus surveys in wild birds is both costly and challenging, requiring a significant investment in effort and resources (Brown et al., 2018). Consequently, studies and active surveillance programs remain limited in regions like Latin America and the Caribbean (Olsen et al., 2006; Ferrer et al., 2014; Brown et al., 2018). However, risk-based surveillance can be helpful in directing resources and efforts to those locations or periods of the year where the risk is most likely to occur, leading to improvements in cost-effectiveness of surveillance (Cameron, 2012).
The description and analysis of migratory connectivity in host species can provide information on viral circulation. Establishing migratory connectivity requires identifying the geographical links between individuals and populations from one season of their life cycle to another, offering insights into the degree of mixing between breeding populations on wintering grounds or vice versa (Webster et al., 2002; Bauer et al., 2016; Hobson et al., 2019). This information allows for inferences to be made regarding the origin of infected individuals and the pathways of virus spread (Jourdain et al., 2007; Prosser et al., 2009; Gunnarsson et al., 2012; Newman et al., 2012; Bessell et al., 2016).
The Caribbean region, situated adjacent to North America, serves as a critical wintering area for numerous waterbird species that breed in North America (Aguilar et al., 2020). However, research on the connectivity of Neotropical migratory species in the Caribbean is limited, with a focus on a few passerines, seabirds, shorebirds, and a single waterfowl species (Rubenstein et al., 2002; Szymanski and Dubovsky, 2013; Cohen et al., 2014; Hobson et al., 2014; Ramey et al., 2016; Lyons et al., 2017; Reed et al., 2018). Given the potential for the introduction and spread of North American-origin viruses through bird migration in the Caribbean (Brown et al., 2018; Pupo-Antunez et al., 2018), there is a need for active virus surveillance programs, especially in key locations. Cuba serves as a crucial stopover site for birds en route to other Caribbean islands and South America (Aguilar et al., 2020), making it of regional significance
In addition to the passive surveillance of HPAI, Cuba has implemented an active surveillance system based on risk focused on poultry (Ferrer et al., 2014), which was later improved through geospatial multi-criteria analysis of the disease risk of occurrence (Ippoliti et al., 2019; Montano et al., 2020). For wild birds, locations and species in which avian influenza viruses are most likely to be found were deduced (Alfonso et al., in press). Results highlight certain waterfowl species, such as the Blue-winged Teal (Spatula discors, hereafter BWTE) and Lesser Scaup (Aythya affinis), as potential introducers of the virus. Specifically, BWTE stands out as the most abundant Anatidae species in Cuba (Aguilar et al., 2020).
BWTE is recognized as a significant contributor to the exchange of parasites and pathogens between North America and the Neotropics (Smith and Ramey, 2015; Ramey et al., 2016). This species plays a role in the spread of AIV in both North and South America (González-Reiche et al., 2012; Ramey et al., 2014; Humphreys et al., 2020), given its high prevalence and diversity of AIV subtypes (Olsen et al., 2006; Munster et al., 2007; Bevins et al., 2014; Papp et al., 2017). To the best of our knowledge, the migratory connectivity of wild birds has been utilized to a limited extent in combination with the analysis of the risk of pathogen incursion transmitted by them to new areas in the Neotropics, which could represent opportunities for managing this risk in the face of a lack of information.
The aim of this study was to assess the migratory connectivity between North America and Cuba of BWTE and its implications for the risk of AIV introduction to Cuba. Our work is expected to inform recommendations for further improvements of the cost-effective active surveillance system in Cuba.
2 Materials and methods
2.1 Feather sampling areas and preparation
Feather samples from BWTE were collected during the autumn migration and wintering seasons in Cuba. During the autumn migration of 2021, samples were collected on ten different dates, from September to November, in western Cuba. The sampling area was Bajos de Santa Ana, situated west of Havana on the north coast of Cuba (23°04’06’’ N, 82°31’33’’ W). This location is a known stopover site for waterfowl during migration and consists of a mangrove forest covering approximately 21.9 hectares. The site experiences significant human activity due to its proximity to the town of Santa Fe. Presently, because of habitat degradation and hunting pressure, it serves as a stopover site for only a brief period. These characteristics ensured that the samples were obtained from individuals exclusively engaged in migration.
During the winter season, feathers were collected from the Southern Sancti Spíritus Wetland, located in the central region of Cuba (21°38’09’’ N, 79°12’20’’ W), in December 2020. This area is recognized as an Important Bird Area. The majority of this wetland region is occupied by rice fields, covering approximately 27,000 hectares (Acosta et al., 2002). Separated from the sea by a coastal belt of wetlands containing mangroves and several lagoons (BirdLife International, 2020) to the west, it adjoins a group of lagoons and intertidal mudflats that are part of the Tunas de Zaza Fauna Refuge. The entire coastal area serves as a vital refuge for migratory birds, including those from the Charadriiformes and Anseriformes orders. It hosts large congregations, sometimes numbering up to 100,000 individuals of BWTE (BirdLife International, 2020).
All sampled individuals were from carcasses obtained from the hunting season in Cuba. The right wing of each individual was taken, and their sex (Female/Male) and age (After Hatch Year: AHY and Hatch Year: HY) were identified based on wing plumage characteristics following the methods described by Pyle (2008). The primary flight feathers were added to the feather collection at the Felipe Poey y Aloy Museum, University of Havana. For isotopic analysis, the most distal primary flight feather (P10) was selected.
2.2 Stable-hydrogen isotope analysis
Primary feathers (P10; n = 276) were analyzed for δ2H at the Laboratory for Stable-Isotope Science – Advance Facility for Avian Research (LSIS-AFAR, Western University, London, Ontario, Canada). Feathers were cleaned of surface oils using a 2:1 methanol:chloroform solvent (>12h) and allowed to air dry (24h). Feather vane was clipped from the distal end of the feather and a subsample (0.35 ± 0.02 mg) packed into silver cups and crushed. Samples were loaded, simultaneously with laboratory standards, in a Uni-Prep autosampler (Eurovector, Milan, Italy), heated to 60°C and flushed with dry helium and maintained under helium pressure (Wassenaar et al., 2015). Samples were combusted using flash pyrolysis (~1,350°C) on glassy carbon, separated via a Eurovector 3000 elemental analyzer interfaced with a Thermo Delta V Plus continuous-flow isotope-ratio mass spectrometer (CF-IRMS; Thermo Instruments, Bremen, Germany). Feather δ2H values were derived using the comparative equilibration method of Wassenaar and Hobson (2003). Two keratin standards (CBS, δ2H = −197‰; KHS, δ2H = −54.1‰) were included at the beginning (n = 3, per standard) and end (n = 2, per standard) of each run (n = 38 samples per tray). All values were corrected for linear instrumental drift. At LSIS-AFAR, across-run error has been estimated to be ±2‰. All values are reported in delta (δ) notation in per mil (‰) deviations from the Vienna Mean Ocean Water standard (VSMOW).
2.3 Seasons, date of migration, age and sex comparisons
The effect of season, date of migration, sex and age on feather δ2H was investigated. We compared isotopic compositions between seasons using a Mann-Whitney test due to the non-normal distribution of the data. The result of the tests was interpreted based on the degree of evidence provided by the p-values (Muff et al., 2022). Furthermore, confidence intervals were calculated using the Monte Carlo resampling method (10,000 Iterations). Non-overlapping confidence intervals were considered as additional evidence of differences among samples.
To evaluate the effect of migration date, age and sex we employed linear regression models and the Information Theoretic framework with the Akaike index corrected for small sample sizes (AICc) (Dochtermann and Jenkins, 2011). We analyzed seven models for migration and four models for the winter season. That set of models included different combinations of age, sex, and migration date effects, along with a null model, to identify the most parsimonious models (Table 1). To satisfy the assumption of data normality, we removed five extreme values from the dataset for the migration season. The ΔAICc value and the ranking relative to the null model served as evidence for the model(s) with the strongest support. In cases of model selection uncertainty, we used model averaged estimates, and we calculated 85% and 95% confidence intervals for parameter estimates. Predictive variables with 85 and 95% confidence intervals excluding zero, along with a relatively high AIC weight, were considered important (Arnold, 2010). In addition, we plotted the predicted values and 95% confidence intervals for variables in model set to visualize relationships. To assess the fit of models with stronger support, we examined residuals using graphical tools. All the analyses were conducted using the program R version 4.3.1 [R Core Team, 2023; R Project for Statistical Computing (RRID: SCR_001905)] and the Excel complement program Poptools V.3.2.3 [Hood, 2010; PopTools (RRID: SCR_022840)].
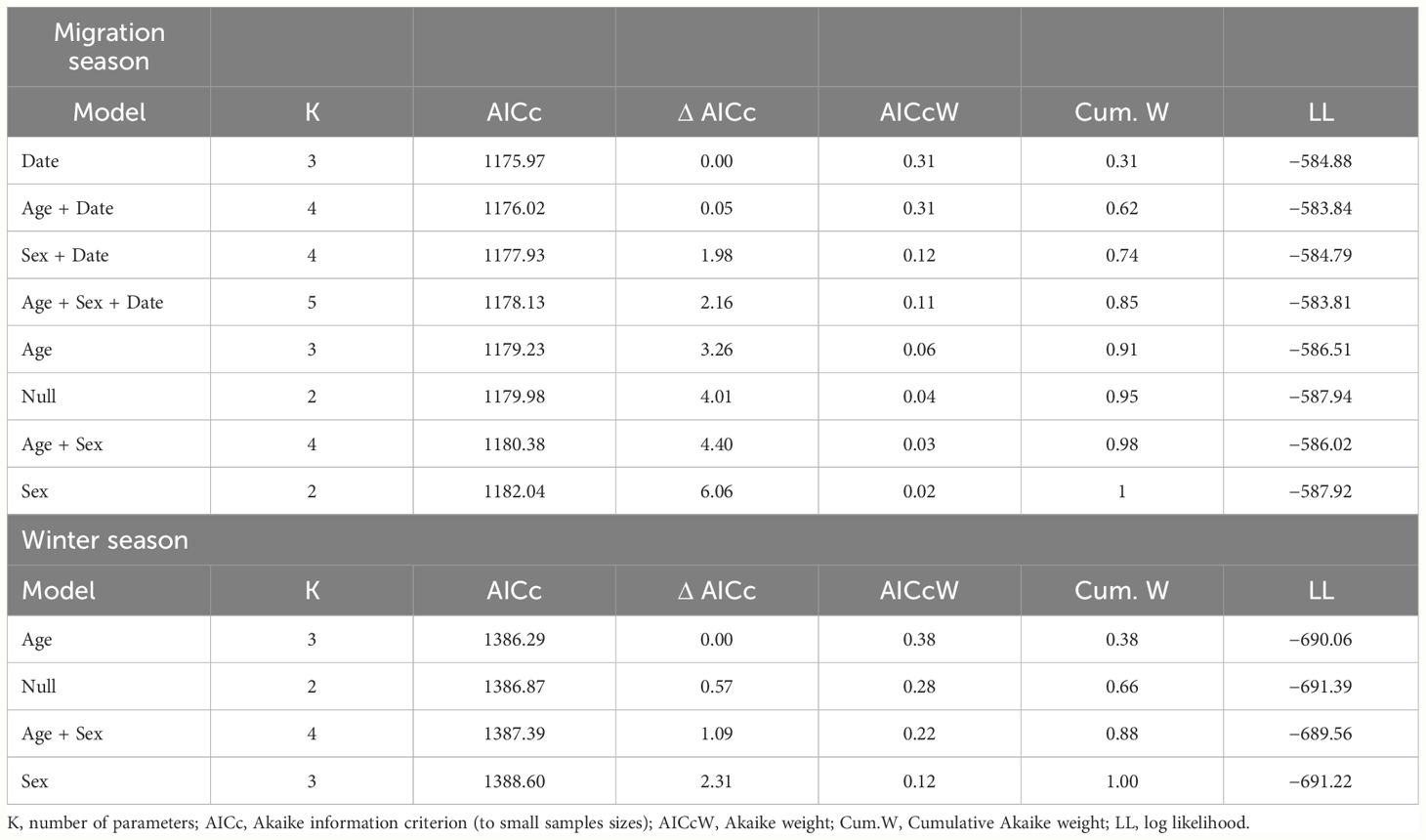
Table 1 Model selection results for models to estimate the influence of age, sex, and date of migration on stable hydrogen isotopes (δ2Hf) recorded in Blue-winged Teal wing feathers during fall migration season 2021 in the west of Cuba and winter season 2020–2021 in the center of Cuba.
2.4 Banding data analysis
The transition probabilities from each breeding area to the region of Cuba were estimated using banding recovery data. These estimates were subsequently employed as prior information in the origin assignment analysis (Hobson et al., 2009). Banding data were requested from the North American Bird Banding Laboratory (BBL) (www.pwrc.usgs.gov/bbl). This database, which is freely accessible, includes capture and recapture information for thousands of bird species from 1955 to the present (Cohen et al., 2014).
The transition probabilities were estimated based on Burnham’s live-recapture dead-recovery modeling framework (Cohen et al., 2014). This model utilizes encounter histories of birds that were captured, marked, released, and subsequently either recaptured, resighted, recovered, or never re-encountered. The model provides estimates for four demographic parameters: survival probability (S), live recapture probability (p), dead recovery probability (r), and transition probability between strata (Psi). The encounter histories were constructed assuming a life span of 12 years (Rohwer et al., 2020), for individuals banded during the reproductive season (May to September) and with records during the migratory and wintering season (September to April).
For BWTE, the estimation of transition probabilities initially included four breeding areas in North America: Western Prairies (Alberta, Saskatchewan and Montana), Eastern Prairies (Manitoba, North and South Dakota), Upper Midwest (Wisconsin, Minnesota, Iowa) and Eastern Region (Ontario, Quebec, Michigan, Ohio, Pennsylvania, Maryland, Delaware, New York, New Jersey, Connecticut, Massachusetts, Rhode Island, New Hampshire, Vermont, Maine). These breeding areas are a modification of the proposals of Szymanski and Dubovsky (2013), based on the availability of banding information and their respective contributions to different harvest areas.
The delineation of populations and their distributions is a fundamental step in assessing migratory connectivity, making it important to establish breeding populations based on ecological criteria (Cohen et al., 2017). Subsequently, validation of these delineated areas was conducted utilizing population characteristics. We estimated population trajectories using the bbsBayes package (Edwards and Smith, 2021), which allows users to access breeding bird survey (BBS) data and to model annual indices and trends using hierarchical Bayesian models. We used a general additive model with a random effect of year (chains = 3, burn-in = 20,000, iterations = 10,000, thinning = 10, samples = 3,000). We used a t-distribution to model the extra-Poisson error distribution, as suggested by Edwards and Smith (2021). We modelled annual indices (1970–2018) stratified by the four regions: Western and Eastern Prairies, Upper Midwest, and Eastern. Based on the results, it was decided to consolidate the original number of breeding regions from four to three, merging the Western and Eastern Prairie regions due to their similarity. Details of these analyses can be found in Supplementary Material 1.
The nine non-breeding regions corresponded to the flyways in which the recapture and recovery records were grouped in the original banding data (Canada, Pacific Migratory Flyways (MF), Central MF, Mississippi MF, Atlantic MF, and Central America) along with northern South America, the Caribbean, and Cuba (which was treated separately from the Caribbean). These nine regions satisfy the assumption that the recovery, resighting, and survival probabilities of banded individuals are uniform within the same area. This assumption is crucial for estimating transition probabilities (Cohen et al., 2014).
Reports of recapture and recovery often exhibit variations among non-breeding regions, with greater irregularities observed in the Neotropics. To reduce the error in estimating these parameters, covariates of effort for ‘r’ and ‘p’ were included in the model, as suggested by Cohen et al. (2014). Effort covariates for each region were calculated based on the number of recaptures and recoveries for all Neotropical migratory duck species in the BBL database relative to the area of available habitats. Available habitats were identified as wetlands and waterbodies, extracted from the Global Land Cover Map of 2015 (Kobayashi et al., 2017). Survival probability was assumed to be constant across all regions. For the analysis, we had data on 87,567 individuals banded in the breeding study areas, including 4,369 total records of recapture or recovery 897 of which were from Cuba. All analyses were conducted using the RMark package (Laake, 2013).
2.5 Estimating origin based on feather isotopic composition
A Bayesian framework was used to establish and map the area of origin of individuals sampled (Vander Zanden et al., 2014; Campbell et al., 2020). Stable-isotope values within waterfowl flight feathers are representative of the natal site for hatch-year birds and the molting site for adults, as they are grown during a synchronous post-breeding molt.
We created an isotopic distribution map (isoscape) for North America, representing the expected δ2H values for BWTE feathers (δ2Hf). To accomplish this, a mean growing-season precipitation (δ2Hp) isoscape (Bowen et al., 2005; Bowen, 2024) was calibrated using the equation based on mallard (Anas platyrhynchos) feathers (Equation 1) (van Dijk et al., 2014), as no calibration data of known-origin feathers were available for this species.
We estimated the likelihood that each cell (pixel) on the map represents a potential area of origin for each individual, using a normal probability density function. This function compares the individual feather δ2H value against the expected (mean) δ2Hf value at a given cell, while accounting for expected variation (i.e., calibration error and isoscape error). We used a residual standard deviation of 12.8‰ (Palumbo et al., 2019). The assignment of the area of origin was confined within the known breeding range (BirdLife International and Handbook of the Birds of the World, 2024) and the most relevant breeding area origin for Cuba based on the banding data analysis.
In order to encompass all possible origins of the sample and facilitate the interpretation of probable surface maps, a hierarchical clustering approach was applied to group individuals into similar geographic regions of origin for each season. Following Campbell et al. (2020), we generated a surface similarity matrix by conducting pairwise comparisons among the probability surfaces for all individuals using the Schoener’s D-metric. Subsequently, a hierarchical clustering analysis was performed on each similarity matrix using the average method. The approximately unbiased p-values (AU) of each cluster were computed via bootstrap resampling. To determine clusters, we cut the trees at a height of 0.5.
The estimated probabilities surfaces were normalized to sum to 1 and binarized by selecting cells with origin probability values exceeding 67%, corresponding to a 2:1 ratio (Van Wilgenburg and Hobson, 2011). Layers depicting the areas of probable origin for each individual were summed for each season and within each origin cluster for the season. This resulted in a representation of origin based on the number of individuals assigned to each pixel. All analyses were conducted using the isocat package version 0.2.6 (Campbell et al., 2020).
2.6 Dynamics of AIV prevalence
To comprehend the epidemiological implications of BWTE migratory connectivity in the context of Cuba, an analysis of the annual dynamics of Avian Influenza Virus (AIV) prevalence was conducted. This analysis encompassed both the molting and natal areas and the likely migratory flyways. To accomplish this, the predictive prevalence models by Kent et al. (2022) were used, which are freely accessible. Those authors provided a comprehensive spatiotemporal assessment of AIV prevalence in 30 duck species, utilizing data from two major surveillance databases in the continental United States: the U.S. Department of Agriculture (USDA) Animal and Plant Health Inspection Service (APHIS) National Wild Bird Avian Influenza Surveillance Program and the National Institutes of Health (NIH) National Institute of Allergy and Infectious Diseases (NIAID) Influenza Research Database.
For the analysis of the annual dynamics AIV prevalence, we worked with the average prevalence values for BWTE derived from the two aforementioned databases, covering all counties within each area of interest. With these data, a maximum and average weekly prevalence value was calculated for each area. These results are specific to the United States, as Kent et al. (2022) indicated that the data availability for Canada was insufficient to ensure robust predictions.
3 Results
The δ2Hf composition of 278 feather samples of BWTE was measured, with 126 samples collected from the western region of Cuba during the fall migration season of 2021 and 152 from the central region of Cuba during the winter season of 2020–2021 (Table 2). The samples were fairly evenly distributed across sex and age classes for both seasons. During the winter season, the highest δ2Hf values were detected (Mean: −111.9‰, CL: −115.5‰ to −108.3‰), with strong evidence of differences compared to the migratory season (Mean: −129.5‰, CL: −135.4‰ to −123.9‰) (Mann Whitney test, U=6,425, p<0.001). Conversely, during the migration season, all sex and age classes exhibited a greater variation in values. Subsequent analyses were conducted separately for each season.
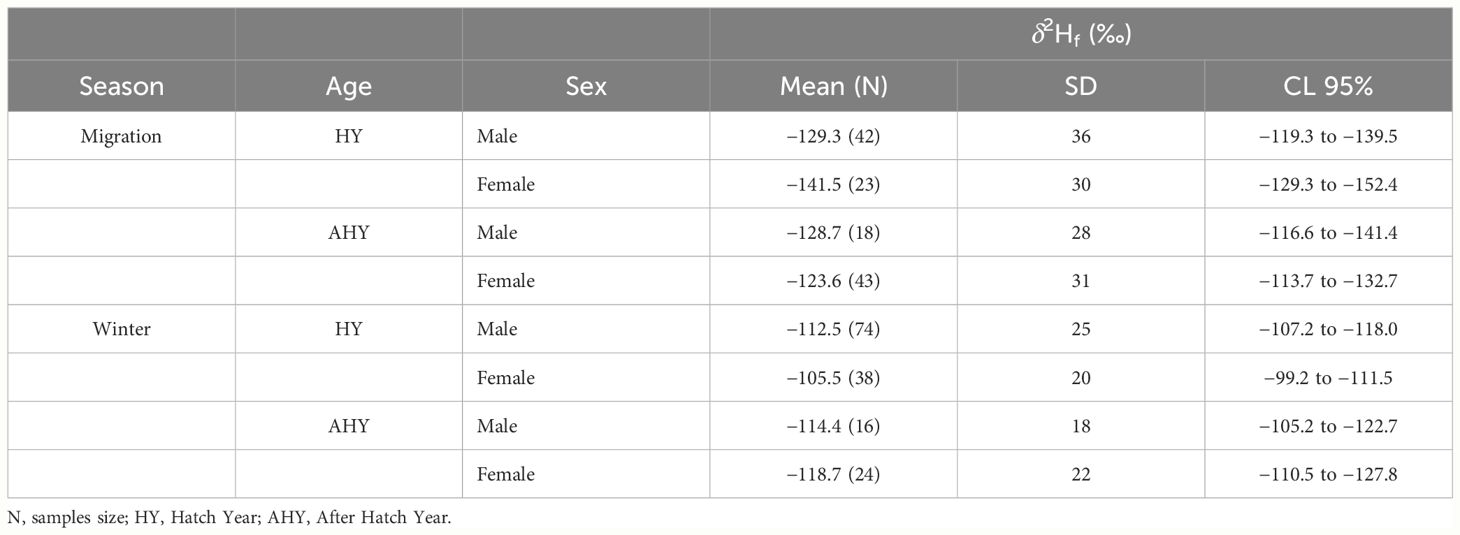
Table 2 Values of mean, standard deviation (SD) and confidence intervals (CL 95%) for Stable hydrogen isotopes (δ2Hf) for age and sex classes, recorded in Blue-winged Teal wing feathers during winter season 2020–2021 in the west of Cuba and fall migration season 2021 in the center of Cuba.
During assessment of the effect of migration date, sex and age, we observed model selection uncertainty. The first two models had an equal probability of being the most parsimonious (Table 1), and five models ranked higher than the null model. Consequently, we utilized model-averaged estimates, 85% confidence intervals, and cumulative Akaike weights to identify crucial variables. Based on the model-averaged estimates and their associated 85% confidence intervals, we found that the day of migration and age were significant factors in explaining δ2Hf during the Blue-winged Teal migratory season (Table 3). The likely origins based on δ2Hf, tended to be more northerly as migration days progressed (Figure 1A). Conversely, AHY individuals exhibited more southerly origins compared to HY individuals (Figure 1B). For the winter season, the null model was among the most supported models, with a ΔAICc of less than two units, which we interpreted as no evidence of age and sex effects on the isotopic composition (Table 1).
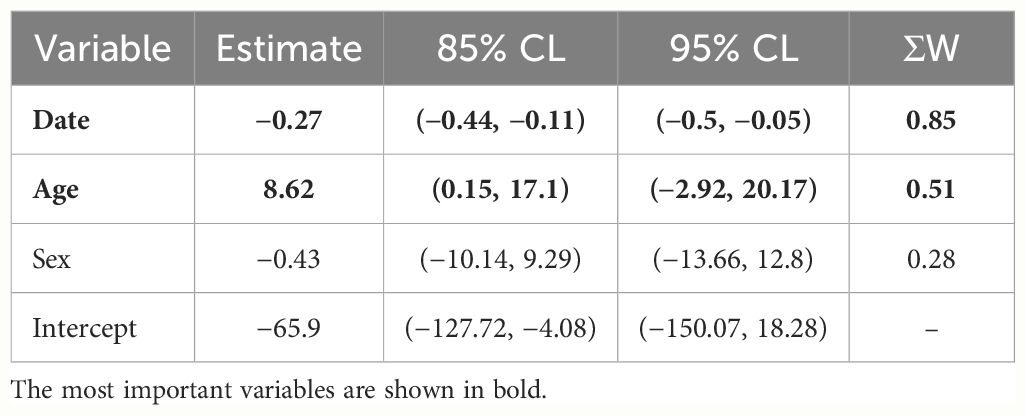
Table 3 Model-averaged parameter estimates, associated confidence intervals (85% CL and 95% CL) and cumulative Akaike weight (ΣW) for the variables used to model stable hydrogen isotopes (δ2Hf) recorded in Blue-winged Teal wing feathers during fall migration season 2021 in Cuba.
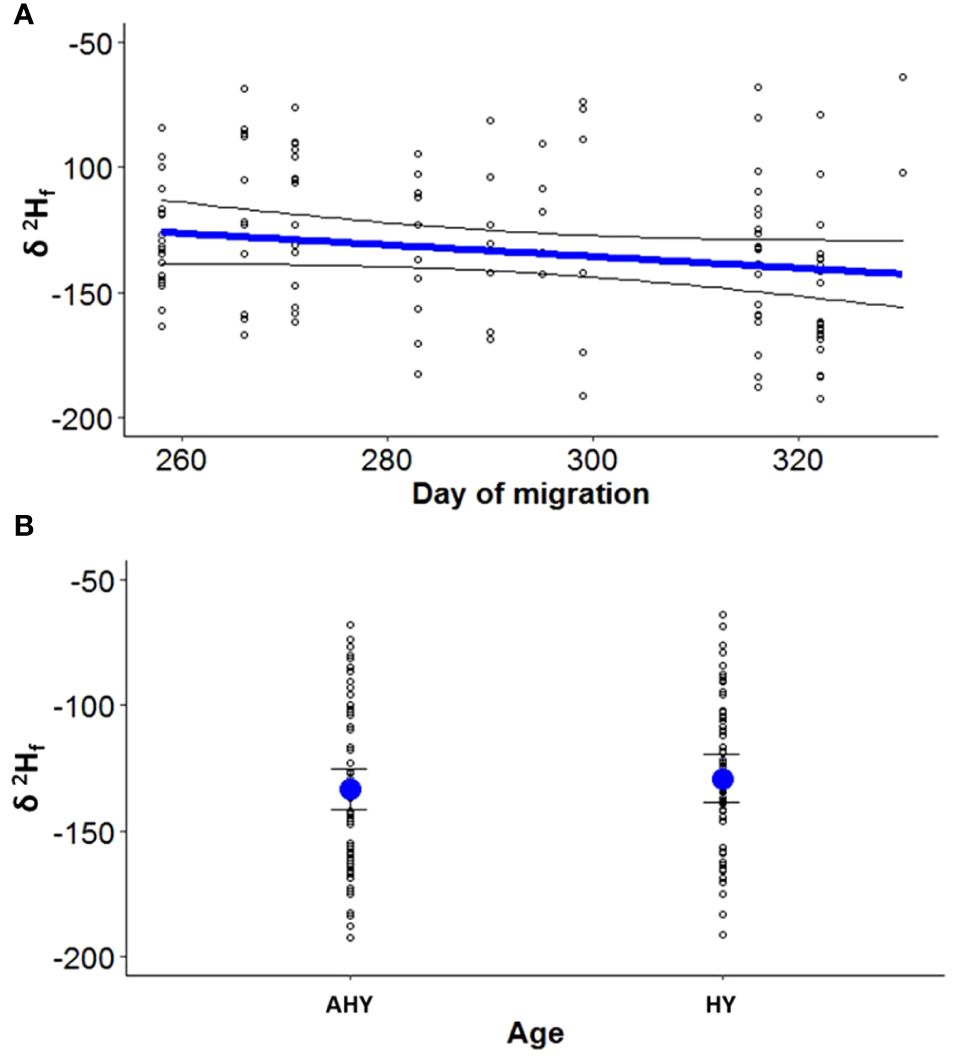
Figure 1 Feather stable hydrogen isotope values (δ2Hf in ‰ VSMOW) recorded in Blue-winged Teal wing feathers during fall migration season of 2021 in Cuba, as a function of day of migration (A) and age (B). Age, AHY, After Hatch Year; HY, Hatch Year. Shown are model-averaged estimates (solid blue dots and blue lines) and associated 95% confidence intervals (black lines), along with raw data (open black dots).
Based on the recapture and recovery history from banding data, the transition probability of BWTE to Cuba was found to be significantly different from zero only for the Prairie breeding area (Table 4), which had the lowest value compared to other non-breeding Neotropical areas. Regarding the Upper Midwest and Eastern breeding areas, the highest transition probabilities were observed for the Mississippi Flyway and Canada. For the Prairie region, there was a notable transition probability to the Central and Mississippi Flyways.
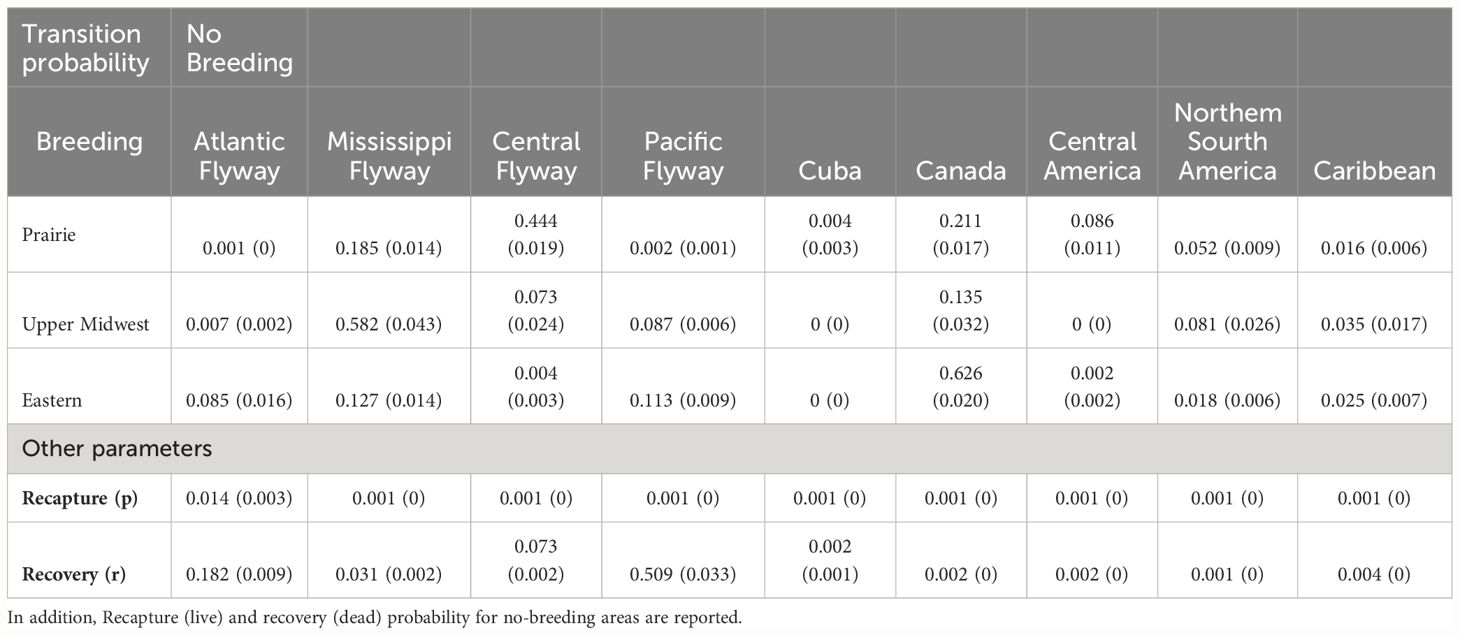
Table 4 Transition probability estimates (error standard) between breeding and no-breeding areas for Blue-winged Teal, based on Burnham’s live-recapture dead-recovery model.
The recapture probability (live) for non-breeding areas exhibited relatively low values, ranging from 0.0137 to 0.0005. Conversely, the recovery probability (dead) showed higher values for the Pacific and Atlantic flyways, followed by the Central and Mississippi flyways. Canada and the other non-breeding Neotropical areas presented lower values, between 0.0015 and 0.0035. The estimated survival probability (S) was 0.4962577 (SD=0.0015).
Based on the transition probability estimated for Cuba using banding data analysis, the areas of probable origin for BWTE, as derived from isotopic composition, were constrained to the west of −95° longitude. Individuals sampled during the migratory season in western Cuba exhibited the most likely molting or natal areas in the prairie and coniferous forest regions of the United States and the prairies of Canada (Figure 2A). Conversely, individuals sampled during the winter season in central Cuba had their most likely origins in prairie areas, primarily from the United States (Figure 2B). The hierarchical clustering analysis indicated the presence of four clusters for each season (Supplementary Material 2). However, the statistical support for these clusters was moderate for the migratory season (AU=75, 77) and poor for the winter season (AU=56, 56). The clusters of individuals from the migratory season confirm a high diversity of origins, with many individuals originating from the north and several having likely origins in the southern distribution.
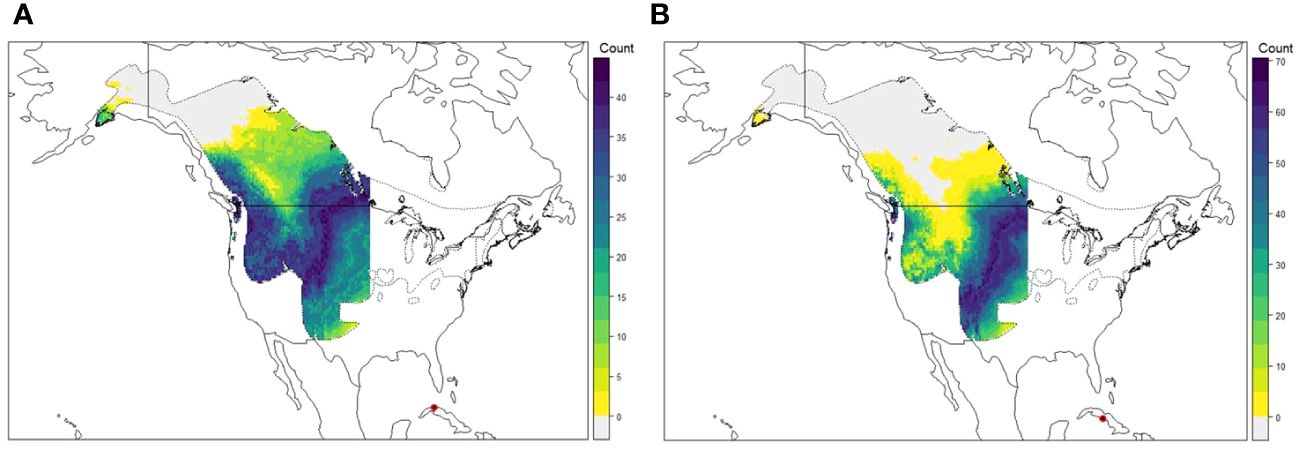
Figure 2 Depiction of probable origin of Blue-winged Teal harvested in (A) western Cuba during migration season 2021 and (B) central Cuba during winter season 2020–2021. Legend number corresponds to number of individuals assigned to each pixel based on a 2:1 odds ratio. The harvest locations are indicated by red dots. Polygon outlines the breeding range of Blue-winged Teal (BirdLife International and Handbook of the Birds of the World, 2024).
In the prairies of the United States, the spatiotemporal AIV prevalence pattern forecasted by Kent et al. (2022) for BWTE indicates an increase starting in August (week 32, mean positive proportion: 0.1; range: 0.05–0.16) and peaking in September (week 35, mean positive proportion: 0.18; range: 0.09–0.3). Elevated positive proportions were predicted throughout the autumn migration period, extending until December.
In the U.S. segment of the Mississippi Flyway, a gradual increase in the proportion of positives was observed in August (weeks 31 to 35, mean positive proportion: 0.02 to 0.09). This region maintained relatively elevated values until March, during the spring migration, and peaked in December (week 50, mean positive proportion: 0.1; range: 004–0.23). A similar trend is observed in the Central Flyway, albeit with even higher average positive proportions and a peak in September (weeks 31 to 35, mean positive proportion: 0.03 to 0.1). These elevated values persisted until approximately mid-March.
4 Discussion
In this study, we provided a comprehensive account of migratory connectivity for a crucial species involved in AIV circulation in the Neotropical region, BWTE, including the relatively understudied area of Cuba. To achieve this, we utilized two distinct sources of information that differ in temporal and spatial scales, yet complement each other: feather isotopic values and banding data. The use of these two data in combination has been limited in Neotropical regions, primarily due to biases associated with the capture and recovery probabilities of bands specific to these areas.
The utilization of information derived from banding records was made feasible by incorporating models that account for regional biases (Cohen et al., 2014). These banding data analyses were employed for the first time with regard to this species, enabling us to generate pertinent information covering its entire distribution range. Furthermore, we identified evidence of an age-related and phenological pattern in migration concerning the origins of these individuals.
In the Neotropical region, surveillance efforts remain scarce, especially when compared to the comprehensive spatial, temporal, and species-specific monitoring carried out in North America (Hill et al., 2012; Bevins et al., 2014; Papp et al., 2017). However, identifying priority areas for monitoring viral evolution or viral incursion can help capitalize resources for early warning. The delineation of potential molting or natal areas and migratory routes has provided a foundation for prioritizing areas and information of interest within North America. This information is crucial for developing monitoring strategies in Cuba, and other Neotropical areas.
4.1 Probable origin for Blue-winged Teal sampled in Cuba
The most probable molting or natal area of individuals sampled during migration in western Cuba was extensive and located further north than those sampled in the central region of Cuba during winter. The substantial isotopic variation observed in the migratory season samples, along with strong evidence of differences from the wintering season samples, suggests a diverse range of potential origins for BWTE individuals using Cuba as a wintering or stopover site en route to the rest of the Caribbean, Central, and South America. However, during the migratory season, the probable area of origin for the samples corresponds to wetlands in the forests and prairies of the central-western United States and southern Canada, whereas during the winter season, it is confined mainly to prairie regions within the United States.
Both identified areas align with the regions characterized by the highest density of breeding pairs for the species, spanning from northeastern Iowa and the Dakotas to southern Manitoba, Saskatchewan, and Alberta (Rohwer et al., 2020). In this core breeding area, BWTE was one of the most abundant duck species, while in the remaining parts of its distribution range, pairs are found at lower densities and in irregular patterns.
During migration, BWTE individuals typically align their route with the migratory flyway closest to their breeding area; although there is approximately a 4.8% probability that some will cross to another flyway (Sharp, 1972; Garvon et al., 2011). The migration to wintering areas spans a duration of 6–8 weeks (Szymanski and Dubovsky, 2013). According to the analysis of Szymanski and Dubovsky (2013), a significant portion of individuals use the Central and Mississippi flyways with a smaller contingent migrating via the Pacific and Atlantic flyways. More specifically, birds originating from core breeding areas tend to follow the course of the Mississippi River Valley, ultimately reaching Mexico, Central America, and South America through Texas and Louisiana (Bellrose, 1980; Garvon et al., 2016).
Our results, which draw on banding data analysis, validate the notion that individuals predominantly utilize the migratory flyway closest to their breeding grounds. Specifically, for the Prairie breeding population, the primary migratory routes align with the Central and Mississippi flyways. The relative stability that these connectivity patterns support inferences of increased risk of AIV incursion in certain areas of Cuba based on publicly available information on virus detection in wild birds in the regions of origin at least in Canada and the United States (CWHC, 2024; USDA, 2024).
According to Kent et al. (2022) the prairie region of the United States, which coincides with the core breeding area of BWTE, is one of the regions with the highest incidence of AIV primarily in the months of October to November and mainly associated with the H5 subtype. These findings were exclusively derived from USDA data. However, we observed that, for birds from the Prairies, the peak starts earlier (August with peak in September). While Kent et al. (2022) focused on the U.S. region, it is likely that a similar pattern occurs in the core breeding areas of southern Canada, given their geographic continuity and shared ecoregion. Indeed, Papp et al. (2017) reported that the likelihood of a duck testing positive for AIV reached a peak in mid-August in the Prairie Provinces of Canada. On the other hand, for the U.S. segment of the Central and Mississippi Flyway, the proportion of positive cases remains relatively high until March during the spring migration. However, the elevated values observed during the spring season are likely associated with the H7 subtype (Kent et al., 2022).
We determined that BWTE sampled in Cuba during a period of likely stopover of birds en route to wintering sites further south (Central and northern South America) suggests the possibility that some of the BWTE individuals that use the island as a stopover location may ultimately reach one of these two regions. The highest probability of transition for these birds was attributed to the Upper Midwest and Prairie breeding population. This aligns with the analysis of band recoveries made by Fernández-Ordóñez and Albert (2022) for birds recovered in Venezuela for BWTE and suggests a fall leapfrog migration pattern for this species.
Distinctions in transition probabilities were identified between Cuba and the rest of the insular Caribbean, with the highest transition probabilities associated with the Upper Midwest and Eastern BWTE breeding areas. This suggests the presence of various migratory routes within the Caribbean. However, we are not aware of any similar connectivity pattern described for BWTE in the Caribbean and note a tendency for researchers to treat the Caribbean as a single region (Cohen et al., 2014). Possibly, the route passing through the smaller Caribbean islands connects with central and eastern regions of Cuba but remains undetected due to regional biases in band capture and reporting probabilities. This underscores the need to incorporate samples from other Cuban areas when investigating BWTE migration.
4.2 Suggested patterns of the migration
We found compelling evidence indicating that both migration date and individual age significantly influenced feather isotopic composition. Specifically, based on δ2Hf, we observed that individuals tend to exhibit a more northerly origin as the migratory season progresses. Generally, there is a decreasing trend in the AIV prevalence with lower latitudes (Munster et al., 2007; Bevins et al., 2014). This gradient is associated with environmental factors such as temperature or host population density (Papp et al., 2017), as well as the temporal dynamics of virus circulation itself, with the highest prevalence typically occurring at the onset of the autumn migration (Olsen et al., 2006; Latorre-Margalef et al., 2009).
For BWTE, being among the first duck species to initiate migration, a decreased probability of infection by pathogens and parasites may result. Consequently, many individuals arrive in non-breeding regions without prior immunological experience (Garvon et al., 2016; Humphreys et al., 2020). This phenomenon would explain the species’ relatively high AIV prevalence later during the winter season in regions such as the Gulf Coast and Neotropics (Hanson et al., 2005; Ferro et al., 2010; González-Reiche et al., 2012; Hill et al., 2012). All of these findings underscore the critical importance of the initial phase of autumn migration for Cuba (Ferrer et al., 2014). During this phase, individuals tend to occupy molting areas to the south, increasing the likelihood of lacking prior immunological experience and, consequently, raising the possibility of acquiring the virus during the migration process.
There was a slight indication that adult BWTE individuals sampled during migration in Cuba occupied molting areas further south in North America than juveniles. Notably, higher AIV prevalence and viral shedding have been observed in juvenile (hatch-year) individuals within wild bird populations (Hill et al., 2012; Hoye et al., 2012; Avril et al., 2016). This outcome is linked to the fact that juveniles possess an immune system lacking immunity against AIV, in contrast to adults who may have acquired it in prior exposure. For the BWTE, adult males are among the first to migrate and can become abundant on the Gulf of Mexico coasts starting in late August (Bellrose, 1980; Thurber et al., 2020). However, as of the sampling initiation date in September, no discernible temporal variation pattern was observed in the age distribution of migrants.
4.3 Future lines of research
Part of our study was carried out during the winter season in the central region of Cuba, specifically in one of the country’s most significant wetlands, the Southern Sancti Spíritus Wetland (BirdLife International, 2020). This wetland stands out not only for its high concentration of BWTE but also for its recognition as a crucial area in the circulation of other viruses that utilize birds as hosts, such as West Nile virus (Pupo-Antunez et al., 2018). Nevertheless, there are other wetlands in the southern region of Cuba that are used by this species during winter. For instance, the Southern Los Palacios Wetland in the western region is noted for its remarkable richness and abundance of aquatic species (Aguilar et al., 2020). Furthermore, due to its geographical position as one of the primary entry points for migration and its proximity to poultry commercial farms (Ferrer et al., 2014; Montano et al., 2020), this area is important in terms of risk in AIV transfer between wild and domestic birds. In the future, it would be advantageous for studies to encompass additional areas like these to enhance our comprehension of the migratory connectivity of species utilizing Cuba as their winter habitat.
Our establishment of migratory connectivity of BWTE using Cuba emphasizes opportunities for addressing various research issues associated with pathogen circulation (Altizer et al., 2011). Furthermore, our approach offers a pathway to scientifically reinforce risk-based surveillance solutions for early alert, prevention and control of these pathogens in Neotropical regions. The pattern of connectivity found in our study, together with knowledge of AIV infection rates at migration origin sites (Hobson et al., 2014), allows for a more accurate stratification of the risk of AIV incursion at destination and, consequently, better possibilities for risk-based planning towards resilience. The next phases of research should aim to integrate information on the infection dynamics of birds throughout their life cycle and their migratory behaviors, spanning both individual and population levels (Lebarbenchon et al., 2009; van Toor et al., 2018).
Through these combined analyses, we can identify critical areas along migratory flyways, including important stopover sites, which play a pivotal role in virus transmission and its potential movement across borders. Clearly, we have demonstrated the added value of risk assessment based on migratory connectivity deduced by stable isotope studies in BWTE.
Data availability statement
The raw data supporting the conclusions of this article will be made available by the authors, without undue reservation.
Ethics statement
Ethical approval was not required for the study involving animals in accordance with the local legislation and institutional requirements because the samples utilized in this study were obtained from the carcasses of a species targeted in sport hunting. The period of sampling aligned with the species’ hunting season in Cuba.
Author contributions
AR-O: Conceptualization, Data curation, Formal analysis, Investigation, Methodology, Software, Visualization, Writing – original draft. JK: Formal analysis, Investigation, Methodology, Software, Writing – review & editing. LM: Conceptualization, Supervision, Writing – review & editing. MC: Conceptualization, Supervision, Writing – review & editing. PA: Conceptualization, Supervision, Writing – review & editing. BD-H: Writing – review & editing. YA: Writing – review & editing. EG: Resources, Writing – review & editing. KH: Conceptualization, Funding acquisition, Supervision, Writing – review & editing.
Funding
The author(s) declare financial support was received for the research, authorship, and/or publication of this article. Funding to KH was provided by Environment and Climate Change Canada and a Discovery Grant for the Natural Sciences and Engineering Research Council (NSERC) of Canada (2017-04430).Funding to JK was provided by Natural Sciences and Engineering Research Council (NSERC PGSD), the Province of Ontario (QEII-GSST) and Western University.
Acknowledgments
Daniela Ventura and Elio Fontes assisted with field surveys and feather processing. Logistical support from the Museo General Municipal de la Sierpe’s management and Miguel Zuriaurre’s help in La Sierpe.
Conflict of interest
The authors declare that the research was conducted in the absence of any commercial or financial relationships that could be construed as a potential conflict of interest.
Publisher’s note
All claims expressed in this article are solely those of the authors and do not necessarily represent those of their affiliated organizations, or those of the publisher, the editors and the reviewers. Any product that may be evaluated in this article, or claim that may be made by its manufacturer, is not guaranteed or endorsed by the publisher.
Supplementary material
The Supplementary Material for this article can be found online at: https://www.frontiersin.org/articles/10.3389/fbirs.2024.1401625/full#supplementary-material
References
Acosta M., Mugica L., Denis D. (2002). Dinámica de los principales gremios de aves que habitan la arrocera Sur del Jíbaro, Sancti Spíritus, Cuba. El Pitirre 15, 25–30.
Aguilar S., Manica L. T., Acosta M., Castro R., Hernández Z., González A., et al. (2020). Spatio-temporal patterns of waterbird assemblages in Cuba’s South Coast Wetlands: Conservation implications. Wetlands 40, 407–419. doi: 10.1007/s13157-019-01178-3
Altizer S., Bartel R., Han B. A. (2011). Animal migration and infectious disease risk. Science. 331, 296–302. doi: 10.1126/science.1194694
Arnold T. W. (2010). Uninformative parameters and model selection using Akaike's Information Criterion. J. Wildl. Manage. 74, 1175–1178. doi: 10.1111/j.1937–2817.2010.tb01236.x
Avril A., Grosbois V., Latorre-Margalef N., Gaidet N., Tolf C., Olsen B., et al. (2016). Capturing individual-level parameters of influenza A virus dynamics in wild ducks using multistate models. J. Appl. Ecol. 53, 1289–1297. doi: 10.1111/1365-2664.12699
Bauer S., Lisovski S., Hahn S. (2016). Timing is crucial for consequences of migratory connectivity. Oikos. 125, 605–612. doi: 10.1111/oik.02706
Bessell P. R., Robinson R. A., Golding N., Searle K. R., Handel I. G., Boden L. A., et al. (2016). Quantifying the risk of introduction of West nile virus into great britain by migrating passerine birds. Transbound Emerg. 63, e347–e359. doi: 10.1111/tbed.2016.63.issue-5
Bevins S. N., Pedersen K., Lutman M. W., Baroch J. A., Schmit B. S., Kohler D., et al. (2014). Large-scale avian influenza surveillance in wild birds throughout the United States. PLoS One 9, e104360. doi: 10.1371/journal.pone.0104360
BirdLife International (2020) Important Bird Areas factsheet: Humedal Sur de Sancti Spíritus. Available online at: http://www.birdlife.org (Accessed October 19, 2020).
BirdLife International and Handbook of the Birds of the World (2024) Bird Species Distribution Maps of the World. Version 2020.1. 2020. Available online at: http://datazone.birdlife.org/species/requestdis (Accessed January 13, 2024).
Bowen G. J. (2024) Gridded maps of the isotopic composition of meteoric waters. Available online at: http://www.waterisotopes.org (Accessed January 13, 2024).
Bowen G. J., Wassenaar L. I., Hobson K. A. (2005). Global application of stable hydrogen and oxygen isotopes to wildlife forensics. Oecologia. 143, 337–348. doi: 10.1007/s00442-004-1813-y
Breban R., Drake J. M., Stallknecht D. E., Rohani P. (2009). The role of environmental transmission in recurrent avian influenza epidemics. PLoS Comput. Biol. 5, e1000346. doi: 10.1371/journal.pcbi.1000346
Brown A. J., Victor G., Hartley D., Oura C. (2018). A review of eight high-priority, economically important viral pathogens of poultry within the caribbean region. Vet. Sci. 5, 1–12. doi: 10.3390/vetsci5010014
Cameron A. R. (2012). The consequences of risk-based surveillance: Developing output-based standards for surveillance to demonstrate freedom from disease. Prev. Vet. Med. 105, 280–286. doi: 10.1016/j.prevetmed.2012.01.009
Campbell C. J., Fitzpatrick M. C., Vander Zanden H. B., Nelson D. M. (2020) Advancing interpretation of stable isotope assignment maps: comparing andsummarizing origins of known-provenance migratory bats. Anim. Migr. 7, 27–41. doi: 10.1515/ami-2020-0004
Cohen E. B., Hostetler J. A., Hallworth M. T., Rushing C. S., Sillett T. S., Marra P. P. (2017). Quantifying the strength of migratory connectivity. Methods Ecol. Evol. 9, 513–524. doi: 10.1111/2041-210X.12916
Cohen E. B., Hostetler J. A., Royle J. A., Marra P. P. (2014). Estimating migratory connectivity of birds when re-encounter probabilities are heterogeneous. Ecol. Evol. 4, 259–270. doi: 10.1002/ece3.1059
CWHC (2024) Highly Pathogenic Avian Influenza - wildlife dashboard. Available online at: https://www.cwhc-rcsf.ca/avian_influenza.php (Accessed February 26, 2024).
Dochtermann N. A., Jenkins S. H. (2011). Developing multiple hypotheses in behavioral ecology. Behav. Ecol. Sociobiol. 65, 37–45. doi: 10.1007/s00265-010-1039-4
Edwards P. M. B., Smith C. A. (2021). bbsBayes: an R package for hierarchical bayesian analysis of North American breeding bird survey data. J. Open Res. Softw. 9, 1–19. doi: 10.5334/jors.329
Fernández-Ordóñez J. C., Albert S. K. (2022). A century of bird band recoveries in Venezuela yield insights into migratory ecology. Southwest. Nat. 67, 39–51. doi: 10.1894/0038–4909-67.1.39
Ferrer E., Alfonso P., Ippoliti C., Abeledo M., Calistri P., Blanco P., et al. (2014). Development of an active risk-based surveillance strategy for avian influenza in Cuba. Prev. Vet. Med. 116, 161–167. doi: 10.1016/j.prevetmed.2014.05.012
Ferro P. J., Budke C. M., Peterson M. J., Cox D., Roltsch E., Merendino T., et al. (2010). Multiyear surveillance for avian influenza virus in waterfowl from wintering grounds, Texas coast, USA. Emerg. Infect. Dis. 16, 1224. doi: 10.3201/eid1608.091864
Garvon J. M., Fedynich A. M., Peterson M. J., Pence D. B. (2011). Helminth community dynamics in populations of blue-winged teal (Anas discors) using two distinctMigratory corridors. J. Parasitol. Res. 2011, 306257. doi: 10.1155/2011/306257
Garvon J. M., Mott J. B., Jacobs S. S., Fedynich A. M. (2016). Blood parasites of blue-winged teal (Anas discors) from two migratory corridors, in the Southern USA. J. Wildl. Dis. 52, 725–729. doi: 10.7589/2016-01-010
González-Reiche A. S., Morales-Betoulle M. E., Alvarez D., Betoulle J.-L., Muller M. L., Sosa S. M., et al. (2012). Influenza A viruses from wild birds in Guatemala Belong to the North American lineage. PLoS One 7, e32873. doi: 10.1371/journal.pone.0032873
Gunnarsson G., Latorre-Margalef N., Hobson K. A., Van Wilgenburg S. L., Elmberg J., Olsen B., et al. (2012). Disease dynamics and bird migration—Linking mallards anas platyrhynchos and subtype diversity of the influenza A virus in time and space. PLoS One 7, e35679. doi: 10.1371/journal.pone.0035679
Hanson B. A., Swayne D. E., Senne D. A., Lobpries D. S., Hurst J., Stallknecht D. E. (2005). Avian influenza viruses and paramyxoviruses in wintering and resident ducks in Texas. J. Wildl. Dis. 41, 624–628. doi: 10.7589/0090-3558-41.3.624
Hill N. J., Takekawa J. Y., Cardona C. J., Meixell B. W., Ackerman J. T., Runstadler J. A., et al. (2012). Cross-seasonal patterns of avian influenza virus in breeding and wintering migratory birds: A flyway perspective. Vector-Borne Zoonotic Dis. 12, 243–253. doi: 10.1089/vbz.2010.0246
Hobson K. A., Norris D. R., Kardynal K. J., Yohannes E. (2019). Animal Migration: A Context for Using New Techniques and Approaches, in Tracking animal migration with stable isotopes. 2nd ed. Eds. Hobson K. A., Wassenaar L. I. (London: Academic Press), 1–23.
Hobson K. A., Van Wilgenburg S. L., Faaborg J., Toms J. D., Rengifo C., Llanes Sosa A., et al. (2014). Connecting breeding and wintering grounds of Neotropical migrant songbirds using stable hydrogen isotopes: a call for an isotopic atlas of migratory connectivity. J. Field Ornithol. 85, 237–257. doi: 10.1111/jofo.12065
Hobson K. A., Wunder M. B., Van Wilgenburg S. L., Clark R. G., Wassenaar L. I. (2009). A method for investigating population declines of migratory birds using stable isotopes: Origins of harvested lesser scaup in North America. PLoS One 4, e7915. doi: 10.1371/journal.pone.0007915
Hood G. M. (2010) PopTools version 3.2.3. Available online at: https://poptools.software.informer.com/3.2/ (Accessed October 14, 2020).
Hoye B. J., Fouchier R. A. M., Klaassen M. (2012). Host behavior and physiology underpin individual variation in avian influenza virus infection in migratory Bewick’s swans. Proc. R. Soc B 279, 529–534. doi: 10.1098/rspb.2011.0958
Humphreys J. M., Ramey A. M., Douglas D. C., Mullinax J. M., Soos C., Link P., et al. (2020). Waterfowl occurrence and residence time as indicators of H5 and H7 avian influenza in North American Poultry. Sci. Rep. 10, 2592. doi: 10.1038/s41598–020-59077–1
Ippoliti C., Candeloro L., Savini L., Conte A., Gilbert M., Ayala J., et al. (2019). Combining multicriteria decision analysis and network-based model to assess the vulnerability of commercial Cuban poultry to avian influenza viruses. Front. Vet. Sci. doi: 10.3389/conf.fvets.2019.05.00110
Jourdain E., Gauthier-Clerc M., Bicout D. J., Sabatier P. (2007). Bird migration routes and risk for pathogen dispersion into Western Mediterranean Wetlands. Emerg. Infect. Dis. 13, 365–372. doi: 10.3201/eid1303.060301
Kent C. M., Ramey A. M., Ackerman J. T., Bahl J., Bevins S. N., Bowman A. S., et al. (2022). Spatiotemporal changes in influenza A virus prevalence among wild waterfowl inhabiting the continental United States throughout the annual cycle. Sci. Rep. 12, 1–10. doi: 10.1038/s41598-022-17396-5
Kilpatrick A. M., Chmura A. A., Gibbons D. W., Fleischer R. C., Marra P. P., Daszak P. (2006). Predicting the global spread of H5N1 avian influenza. PNAS. 103, 19368–19373. doi: 10.1073/pnas.0609227103
Kobayashi T., Tateishi R., Alsaaideh B., Sharma R. C., Wakaizumi T., Miyamoto D., et al. (2017). Production of global land cover data – GLCNMO2013. J. Geogr. Geol. 9, 1–15. doi: 10.5539/jgg.v9n3p1
Laake J. (2013) “RMark: An R Interface for Analysis of Capture-Recapture Data with MARK.” AFSC Processed Rep (Seattle, WA: Alaska Fish. Sci. Cent., NOAA, Natl. Mar. Fish. Serv.). Available online at: https://apps-afsc.fisheries.noaa.gov/Publications/ProcRpt/PR2013–01.pdf (Accessed February 28, 2024).
Latorre-Margalef N., Gunnarsson G., Munster V. J., Fouchier R. A. M., Osterhaus A. D. M. E., Elmberg J., et al. (2009). Effects of influenza A virus infection on migrating mallard ducks. Proc. R. Soc B. 276, 1029–1036. doi: 10.1098/rspb.2008.1501
Lebarbenchon C., Albespy F., Brochet A. L., Grandhomme V., Renaud F., Fritz H., et al. (2009). Spread of avian influenza viruses by common teal (Anas crecca) in Europe. PLoS One 4, e7289. doi: 10.1371/journal.pone.0007289
Lyons J. E., Winn B., Keyes T., Kalasz K. S. (2017). Post-breeding migration and connectivity of red knots in the Western Atlantic. J. Wildl. Manage. 82, 383–396. doi: 10.1002/jwmg.21389
Montano D., Percedo M., Vioel Rodríguez S., Fonseca O., Centelles Y., Ley O., et al. (2020). Influenza aviar. Oportunidades de mejora del sistema de vigilancia activa basado en riesgo en Cuba. Rev. Salud. Anim. 42, 1–10.
Muff S., Nilsen E. B., O’Hara R. B., Nater C. R. (2022). Rewriting results sections in the language of evidence. Trends Ecol. Evol. 37, 203–2010. doi: 10.1016/j.tree.2021.10.009
Munster V. J., Baas C., Lexmond P., Waldenström J., Wallensten A., Fransson T., et al. (2007). Spatial, temporal, and species variation in prevalence of influenza A viruses in wild migratory birds. PLoSPathog. 3, e61. doi: 10.1371/journal.ppat.0030061
Newman S. H., Hill N. J., Spragens K. A., Janies V., Voronkin I. O., Prosser D. J., et al. (2012). Eco-virological approach for assessing the role of wild birds in the spread of avian influenza H5N1 along the central asian flyway. PLoS One 7, e30636. doi: 10.1371/journal.pone.0030636
Olsen B., Munster V. J., Wallensten A., Waldenström J., Osterhaus A. D. M. E., Fouchier R. A. M. (2006). Global patterns of influenza A virus in wild birds. Science. 312, 384–388. doi: 10.1126/science.1122438
Palumbo M. D., Tozer D. C., Hobson K. A. (2019). Origins of harvested Mallards from Lake St. Clair, Ontario: a stable isotope approach. Avian Conserv. Ecol. 14. doi: 10.5751/ACE-01389–140203
Papp Z., Clark R. G., Parmley E. J., Leighton F. A., Waldner C., Soos C. (2017). The ecology of avian influenza viruses in wild dabbling ducks (Anas spp.) in Canada. PLoS One 12, e0176297. doi: 10.1371/journal.pone.0176297
Prosser D. J., Takekawa J. Y., Newman S. H., Yan B., Douglas D. C., Hou Y., et al. (2009). Satellite-marked waterfowl reveal migratory connection between H5N1 outbreak areas in China and Mongolia. Ibis 151, 568–576. doi: 10.1111/j.1474-919X.2009.00932.x
Pupo-Antunez M., Vázquez Y., Andonova M., Vázquez S., Morier L., Castex M. (2018). Field study: Searching for West Nile virus in Cuba. J. Emerg. Dis. Virol. 4. doi: 10.16966/2473–1846.142
Pyle P. (2008). Identification Guide to North American Birds. Part 2 (California: Slate Creek Press).
Ramey A. M., Poulson R. L., González-Reiche A. S., Wilcox B. R., Walther P., Link P., et al. (2014). Evidence for seasonal patterns in the relative abundance of avian influenza virus subtypes in blue-winged teal (Anas discors). J. Wildl. Dis. 50, 916–922. doi: 10.7589/2013-09-232
Ramey A. M., Walther P., Link P., Poulson R. L., Wilcox B. R., Newsome G. M., et al. (2016). Optimizing surveillance for South American origin influenza A viruses along the United States Gulf Coast through genomic characterization of isolates from blue-winged teal. Transbound Emerg. Dis. 63, 194–202. doi: 10.1111/tbed.12244
R Core Team (2023). R: A language and environment for statistical computing (Vienna, Austria: R Foundation for Statistical Computing). Available at: https://www.R-project.org/.
Reed E. T., Kardynal K. J., Horrocks J. A., Hobson K. A. (2018). Shorebird hunting in Barbados: Using stable isotopes to link the harvest at a migratory stopover site with sources of production. Condor. 120, 357–370. doi: 10.1650/CONDOR-17-127.1
Rohwer F. C., Johnson W. P., Loos E. R. (2020). Blue-winged Teal (Spatula discors), version 1.0., in Birds of the World. Eds. Poole A. F., Gill F. B. (Ithaca, NY: Cornell Lab of Ornithology).
Rubenstein D. R., Chamberlain C. P., Holmes R. T., Ayres M. P., Waldbauer J. R., Graves G. R., et al. (2002). Linking breeding and wintering ranges of a migratory songbird using stable isotopes. Science. 295, 1062–1065. doi: 10.1126/science.1067124
Sharp B. (1972). Eastward migration of blue-winged teal. J. Wildl. Manage. 36, 1273–1277. doi: 10.2307/3799262
Smith M. M., Ramey A. M. (2015). Prevalence and genetic diversity of hematozoa in South American waterfowl and evidence for intercontinental redistribution of parasites by migratory birds. Int. J. Parasitol. Parasites Wildl. 4, 22–28. doi: 10.1016/j.ijppaw.2014.12.007
Szymanski M. L., Dubovsky J. A. (2013). Distribution and derivation of the Blue-winged Teal (Anas discors) harvest 1970–2003 (Washington, D.C: U.S. Department of Interior, Fish and Wildlife Service, Biological Technical Publication FWS/BTP-R6017–2013).
Thurber B. G., Roy C., Zimmerling J. R. (2020). Long-term changes in the autumn migration phenology of dabbling ducks in southern Ontario and implications for waterfowl management. Wildl. Biol. 2020, 1–11. doi: 10.2981/wlb.00668
USDA (2024) Wild bird avian influenza surveillance. Available online at: https://www.aphis.usda.gov/aphis/maps/animal-health/wild-bird-avian-flu-surveillance (Accessed February 26, 2024).
Vander Zanden H. B., Wunder M. B., Hobson K. A., Van Wilgenburg S. L., Wassenaar L. I., Welker J. M., et al. (2014). Contrasting assignment of migratory organisms to geographic origins using long-term versus year-specific precipitation isotope maps, Methods Ecol. Evol. 5, 891–900. doi: 10.1111/2041–210X.12229
van Dijk J. G., Meissner W., Klaassen M. (2014). Improving provenance studies in migratory birds when using feather hydrogen stable isotopes. J. Avian Biol. 45, 103–108. doi: 10.1111/j.1600-048X.2013.00232.x
van Toor M. L., Avril A., Wu G., Holan S. H., Waldenström J. (2018). As the duck flies—Estimating the dispersal of low-pathogenic avian influenza viruses by migrating mallards. Front. Ecol. Evol. 6. doi: 10.3389/fevo.2018.00208
Van Wilgenburg S. L., Hobson K. A. (2011). Combining stable-isotope (δD) and band recovery data to improve probabilistic assignment of migratory birds to origin. Ecol. Appl. 21, 1340–1351. doi: 10.1890/09-2047.1
WAHIS (2024) WAHIS: Sistema Mundial de Información Zoosanitaria. Available online at: https://wahis.woah.org/#/home (Accessed March 13, 2024).
Wassenaar L. I., Hobson K. A. (2003). Comparative equilibration and online technique for determination of non- exchangeable hydrogen of keratins for use in animal migration studies. Isotopes Environ. Health Stud. 39, 211–217. doi: 10.1080/1025601031000096781
Wassenaar L. I., Hobson K. A., Sisti L. (2015). An online temperature-controlled vacuum-equilibration preparation system for the measurement of δ2H values of non-exchangeable-H and of δ18O values in organic materials by isotope-ratio mass spectrometry. Rapid. Commun. Mass Spectrom. 29, 397–407. doi: 10.1002/rcm.7118
Webster M. S., Marra P. P., Haig S. M., Bensch S., Holmes R. T. (2002). Links between worlds: unravelling migratory connectivity. Trends Ecol. Evol. 17, 76–83. doi: 10.1016/S0169-5347(01)02380-1
WOAH (2024) Resolution on global control of high pathogenicity avian influenza. Available online at: https://www.woah.org/app/uploads/2023/05/a-r28-tech-item-hpai-1.pdf (Accessed March 1, 2024). 90 GS/FR – May 2023.
Keywords: isotopic signature, banding data, deuterium, spatula discors, surveillance programs, prevalence, Caribbean, flyways
Citation: Rodríguez-Ochoa A, Kusack JW, Mugica L, Cruz MA, Alfonso P, Delgado-Hernández B, Abreu Y, García E and Hobson KA (2024) Migratory connectivity of Blue-winged Teal: risk implications for avian influenza virus introduction to Cuba. Front. Bird Sci. 3:1401625. doi: 10.3389/fbirs.2024.1401625
Received: 15 March 2024; Accepted: 10 May 2024;
Published: 20 June 2024.
Edited by:
Frank Wong, Australian Animal Health Laboratory (CSIRO), AustraliaReviewed by:
Scott Rush, Mississippi State University, United StatesSun-Woo Yoon, Andong National University, Republic of Korea
Copyright © 2024 Rodríguez-Ochoa, Kusack, Mugica, Cruz, Alfonso, Delgado-Hernández, Abreu, García and Hobson. This is an open-access article distributed under the terms of the Creative Commons Attribution License (CC BY). The use, distribution or reproduction in other forums is permitted, provided the original author(s) and the copyright owner(s) are credited and that the original publication in this journal is cited, in accordance with accepted academic practice. No use, distribution or reproduction is permitted which does not comply with these terms.
*Correspondence: Alejandro Rodríguez-Ochoa, cmRndWV6b2Nob2E4OUBnbWFpbC5jb20=