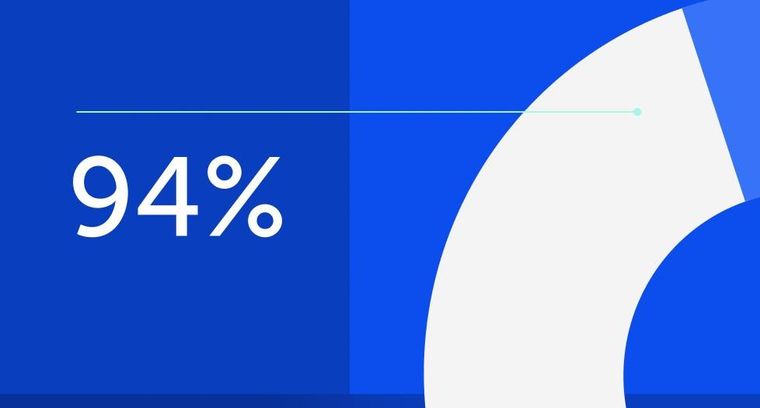
94% of researchers rate our articles as excellent or good
Learn more about the work of our research integrity team to safeguard the quality of each article we publish.
Find out more
ORIGINAL RESEARCH article
Front. Bird Sci., 06 December 2023
Sec. Bird Ecology and Behavior
Volume 2 - 2023 | https://doi.org/10.3389/fbirs.2023.1305453
Wings in birds vary tremendously in size and shape across species. Ecological needs are thought to shape variation across species in wing morphology over evolutionary times. For instance, demands for energetic efficiency in species that fly long distances have produced long and narrow wings with a high aspect ratio. Demand for maneuverability in cluttered habitats or to escape predators more easily have favored wings with large areas relative to body size producing a low wing loading. The association between ecological needs and wing morphology is complex, however, and could involve other ecological variables. Here, I draw attention to two ecological factors that have received little attention, namely, life history and sociality. Species at the slow end of the life history continuum emphasize adult survival over reproduction and are considered risk averse. I predicted that such species would benefit from low wing loading and low aspect ratio to increase maneuverability and the ability to escape predators. More solitary species cannot rely on others in their groups to decrease predation risk. I predicted that such species should also benefit from low wing loading and low aspect ratio. Using data from the literature on wing loading and aspect ratio in a large number of species, I examined these predictions in a phylogenetic framework including several potentially confounding ecological variables that have been previously linked to wing morphology such as habitat, lifestyle, and migration. As predicted, more solitary species tended to have low wing loading and low aspect ratio. Low wing loading was also more common in species at the slow end of the life history continuum, but aspect ratio was higher than predicted. Overall, the results support the idea that life history and sociality can predict variation across species in wing morphology and highlight the effect of predation risk on the evolution of wing morphology in birds.
Wings are a defining trait in many species of animals including insects, birds and some mammals. Generally, the ability to fly allows individuals to escape more easily from predators and poor conditions providing a clear evolutionary advantage. Volant vertebrates, for instance, tend to live longer than their ground counterparts of the same size (Healy et al., 2014). Wings in birds achieve this success despite tremendous variation in size and shape across species. To sustain flight in birds of different sizes, wing size varies allometrically with body size (Greenewalt, 1962; Rayner, 1988) although larger species tend to have relatively larger wings than predicted from their size. In addition to changes expected from body size variation, large variation in shape also occurs across species ranging from long and narrow wings in species of seabirds to short and broad wings in species like owls (Rayner, 1988). Variation in size and in shape across species beyond allometric considerations suggests that wing morphology is subject to selection pressures. The current thinking is that variation in ecological needs across species has shaped wing morphology over evolutionary times (Rayner, 1988; Dial, 2003; Grilli et al., 2017; Lapsansky et al., 2022).
The avian wing is a complex structure and several wing features could be shaped by evolutionary pressures. This includes overall wing length and area, but also more specific features like armwing and handwing length, wingtip shape along with traits related to the supporting locomotor apparatus like bone shape and muscle length (Biewener, 2011; Wang and Clarke, 2015; Baumgart et al., 2021). Two metrics, in particular, have often been used to characterize wing morphology (Norberg, 1990). Wing loading refers to the ratio between body mass and total wing area so that wing loading is low in species with large wings relative to their body size. Aspect ratio measures how pointy the wings are by relating wingspan to total wing area. Long, narrow wings thus have a high aspect ratio. Combinations of these two metrics have important consequences for flight type and maneuverability (Norberg, 1990; Dudley, 2002). Researchers have thus sought to relate variation across species in wing loading and aspect ratio to ecological needs associated with factors such as migration, lifestyle, and predation risk (Swaddle and Lockwood, 1998).
The need to fly long distances during migration or to forage for long periods on the wing is associated with low wing loading and high aspect ratio across birds (Minias et al., 2015; Vincze et al., 2019; Evans, 2021), two features that increase the energetic efficiency of flight (Norberg, 1990). Foraging under water, a substrate that offers more resistance to movement than air, has been linked to the evolution of relatively short wings with high loading as a means to reduce the cost of movements under water (Elliott et al., 2013). However, an aquatic environment might also reduce the need for maneuverability during flight due to lack of obstacles, which would relax selection against wings with a high loading (Lapsansky et al., 2022). Cluttered terrestrial habitats, by contrast, with more obstacles to flight should increase selection pressure for low wing loading to increase maneuverability (Van Hooydonck et al., 2009; Desrochers, 2010; Saino et al., 2017).
Adaptations related to wing morphology can also be viewed as the outcome of interactions with predators (Hedenstrom and Rosen, 2001). Low wing loading is thought to produce greater lift, which would facilitate escape from predators from stationary positions. Escape speed thus typically increases when birds carry less weight for a given wing size (Burns and Ydenberg, 2002; Krams, 2002) making birds with a higher wing loading more vulnerable to attacks (Bednekoff, 1996). In addition, low wing loading can also increase turning maneuverability when airborne (van den Hout et al., 2010) reducing the odds of capture by pursuing predators. Low aspect ratio is thought to increase flight performance during escape. Low aspect ratio reduces inertia, a crucial component to get airborne rapidly (van den Hout et al., 2010). Any ecological niche that reduces exposure to predators should relax selection for a particular wing morphology. For instance, in aquatic habitats, the ability to dive to escape predators might relax selection against high wing loading (Lapsansky et al., 2022).
Research on wing morphology thus far has identified many ecological correlates. Additional work, however, is needed to get a fuller picture. Recent studies, for instance, have shown that earlier conjectures about wing morphology are not necessarily supported in large-scale comparative analyses (Baumgart et al., 2021; Lapsansky et al., 2022), suggesting that the association between ecological needs and wing morphology might be more complex than once thought and could involve other ecological variables. Here, I draw attention to two ecological factors that have received little attention, namely, life history and sociality. Life history is typically viewed as a continuum of strategies involving trade-offs between reproduction and survival (Roff, 2002). At the slow end of the continuum, species tend to produce fewer offspring and live longer while species at the fast end have shorter lives but greater reproduction. Species at the slow end of the continuum are considered less likely to accept risk on themselves to ensure their own survival and future ability to invest in reproduction. At the fast end of the continuum, by contrast, species might accept more risk on themselves since the probability of reproducing in the future is small. Generally, species that emphasize adult survival and future reproduction tend to be more cautious. Indeed, species at the slow end of the continuum flee sooner when predators approach (Blumstein, 2006; Gotanda et al., 2009; Møller and Liang, 2012) and take fewer risks when raising young (Ghalambor and Martin, 2001). Wing morphology in species at the slow end of the life history continuum should show features that reduce the likelihood of capture by predators, such as low wing loading and low aspect ratio, to increase maneuverability during flight.
In terms of sociality, some species live alone while others occur in groups throughout their lives. Living in groups provides several anti-predator advantages (Caro, 2005; Beauchamp, 2014). For example, individuals in groups are more likely to detect predators early and also benefit from the dilution of risk among all group members. Supporting this conjecture, mortality is often lower in groups with more individuals (Cash et al., 1993; Clutton-Brock et al., 1999; Williams et al., 2003; Brown and Brown, 2004; Serrano et al., 2005) or in species that feed in groups rather than alone (Jullien and Clobert, 2000; Cruz-Angón et al., 2008; Srinivasan, 2019; Beauchamp, 2021). Death through predation is also lower in species forming larger groups (Shultz et al., 2004; Beauchamp, 2023a). Solitary species cannot rely on others to decrease the risk of predation. As for species at the slow end of the life history continuum, solitary species are likely to benefit from wings that offer more maneuverability to escape predators more easily.
I tested the prediction that life history and sociality predict variation in wing morphology across birds using a phylogenetic approach. This is important as wing morphology tends to be similar in more closely related species exposed to similar ecological factors (Rayner, 1988; Wang and Clarke, 2015). In addition, I adopted a multivariable approach to take into account the potential confounding effects of other ecological variables. Indeed, life history and sociality are associated with particular ecological niches whose features could on their own directly influence wing morphology. For instance, sociality as well as wing morphology are associated with body size and lifestyle (Greenewalt, 1962; Rayner, 1988; Beauchamp, 2022). Migrating species share traits with species at the slow end of the life history continuum such as high adult survival (Winger and Pegan, 2021). Migration is also expected to alter wing morphology (Minias et al., 2015; Vincze et al., 2019). Therefore, to isolate the association between wing morphology and life history or sociality, it is important to control for the possible effect of body size, lifestyle and migration.
Neither the definition nor units of measurements of wing loading and aspect ratio is consistent in the avian literature. For the definition of wing loading, the main difference lies in the inclusion or exclusion of the area of the body between the wings (known as the root box). I opted to exclude the root box from wing loading measurements to reduce potential variation caused by the inclusion of measurements with or without the root box. Nevertheless, these two measurements are highly correlated and have similar ecological correlates (Vincze et al., 2019). Wing loading thus refers to body mass divided by the total area of the two wings excluding the root box (g cm-2). For aspect ratio, I also excluded the root box and defined wingspan (cm) as twice the wing length measured from the shoulder joint to the tip of the extended wing. Aspect ratio is the square of wingspan divided by the total area of the two wings (no units).
Using these definitions, I searched the avian literature for data on wing loading or aspect ratio. I first consulted large-scale published compilations (Banks, 1930; Poole, 1938; Hartman, 1961; Greenewalt, 1962; Greenewalt, 1975; Vincze et al., 2019; Evans, 2021; Lapsansky et al., 2022; Fu et al., 2023; Gill, 2023). I then made searches on Google Scholar for other sources using the following key words: wing loading, aspect ratio, wing morphology. I excluded studies that used other definitions of wing loading or aspect ratio, and relied only on studies that measured wing length and wing area directly from museum samples, recently dead birds or live birds. I also excluded all flightless species of birds. When sources distinguished between males and females, I opted for female subjects to increase homogeneity among samples (Lapsansky et al., 2022). This is because sexual selection can influence wing morphology and induce differences between males and females (Hedenström and Møller, 1992). Wing morphology can also change with age so I avoided samples obtained from juvenile birds. Average body mass was obtained from the different specimens measured in each study. When body mass was not provided, I used data from the literature (Dunning, 2008).
I used a published source for information on sociality (Beauchamp, 2022). The occurrence of flocking during foraging was the metric for sociality. Flocking was defined as the occurrence of roving groups including more than two adults searching for food, excluding family parties. I distinguished between species that regularly form flocks (flocking) and species that flock irregularly (occasional) or not at all (solitary). I pooled the dataset on sociality and the dataset on wing morphology to obtain a dataset including species with full information on sociality and at least one wing feature.
For each included species, I extracted information for other variables from the literature (Table 1). From Beauchamp (2022), I obtained data for annual adult survival and average clutch size. I used a published standardized dataset for lifestyle, habitat, and migration (Tobias et al., 2022). Lifestyle refers to the main substrate used during foraging (e.g. aquatic or aerial). Habitats are classified according to the extent of vegetation present. Migration status distinguishes between year-round residents and short- or long-distance migrants.
I used a phylogenetic generalized linear mixed model from the phyr package (Ives et al., 2019), which included random effects as well as a control for phylogenetic relatedness. The dependent variable was wing loading in log10 scale in one model and aspect ratio in log10 scale in the other. Given the strong correlation between annual adult survival, body mass, and clutch size, I used a phylogenetic PCA to extract independent components (Revell, 2012). For both wing loading and aspect ratio, the first extracted component showed a strong negative load for annual adult survival and a strong positive load for clutch size so that larger values on this axis represented faster life histories (Table 2). The set of independent variables in each model thus included body mass, the PCA component for pace of life history, habitat, lifestyle, migration, and sociality. I included study source as a random effect to account for idiosyncratic differences among studies in types of specimens included and measurement error. I also included species as a random effect to account for multiple measurements for each species. For phylogenetic relatedness, the variance–covariance distance matrix was considered for the included species in each model. The distance matrix was obtained from a 50% majority consensus tree constructed from a set of 1000 phylogenetic trees (Hackett et al., 2008). I used the ape package to obtain the consensus tree and branch lengths (Paradis et al., 2004). I assessed multicollinearity using variance inflating factors for the independent variables included in each phylogenetic regression model. All VIFs were below 4, indicating little or moderate multicollinearity (O’Brien, 2007).
For wing loading, the full dataset included 1373 measurements from 647 species belonging to 112 families. The top five families were Laridae, Accipitridae, Scolopacidae, Procellaridae, and Turdidae. Wing loading increased allometrically with body mass (Table 3) and with faster life histories (Figure 1). Wing loading was higher in aquatic species and lower in aerial species compared to other lifestyles (Table 3; Figure 2). Wing loading was higher in resident species, but was not related to habitat (Table 3; Figure 2). Controlling for these co-factors, wing loading was lower in more solitary species (Table 3; Figures 1 and 2).
Table 3 Results from a phylogenetic linear model for the effect of body mass, habitat, lifestyle, sociality, migration, and life history on wing loading in birds (n = 1373).
Figure 1 Association between residual wing loading and pace of life history in birds. The association is illustrated for various categories of sociality (flocking, occasional flocking, and solitary). Residuals are taken from the full phylogenetic model with all variables minus the pace of life history and sociality. Larger residuals indicate that wing loading is larger than predicted given the size of a species and its ecology.
Figure 2 The relationship between residual wing loading and ecological variables in birds (A: Sociality; B: Lifestyle; C: Migration; D: Habitat). Residuals are taken from the full phylogenetic model with all variables minus the target variable. Larger residuals indicate that wing loading is larger than predicted given the size of a species and its ecology. Grey-filled violin plots show the distribution of the data. Box and whisker plots show the mean and interquartile range, and the whiskers extend to 1.5 times the interquartile range (outliers are shown as dots). The sample size for each category is shown under each box. Results from post-hoc testing between pairs of means are shown under each box: means with different letters were significantly different after the Benjamini-Hochberg sequential adjustment procedure.
For aspect ratio, I obtained 798 measurements from 520 species belonging to 102 families. The top five families were Laridae, Scolopacidae, Accipitridae, Picidae, and Turdidae. Aspect ratio was not allometrically related to body mass (Table 4) and decreased with faster life histories (Figure 3). Aspect ratio was higher in more open habitats and in aerial species compared to other species (Table 4; Figure 4). Aspect ratio was lower in resident than in migratory species (Table 4; Figure 4). Controlling for these co-factors, aspect ratio was lower in more solitary species (Table 4; Figure 4).
Table 4 Results from a phylogenetic linear model for the effect of body mass, habitat, lifestyle, sociality, migration, and life history on aspect ratio in birds (n = 798).
Figure 3 Association between residual aspect ratio and pace of life history in birds. The association is illustrated for various categories of sociality (flocking, occasional flocking, and solitary). Residuals are taken from the full phylogenetic model with all variables minus the pace of life history and sociality. Larger residuals indicate that aspect ratio is larger than predicted given the size of a species and its ecology.
Figure 4 The relationship between residual aspect ratio and ecological variables in birds. (A: Sociality; B: Lifestyle; C: Migration; D: Habitat). Residuals are taken from the full phylogenetic model with all variables minus the target variable. Larger residuals indicate that aspect ratio is larger than predicted given the size of a species and its ecology. Grey-filled violin plots show the distribution of the data. Box and whisker plots show the mean and interquartile range, and the whiskers extend to 1.5 times the interquartile range (outliers are shown as dots). The sample size for each category is shown under each box. Results from post-hoc testing between pairs of means are shown under each box: means with different letters were significantly different after the Benjamini-Hochberg sequential adjustment procedure.
Using a phylogenetic approach, I examined whether life history and sociality predict variation in wing morphology across birds. In a large number of species occupying varied ecological niches, I found some support for the hypothesis after controlling for potentially confounding variables. More solitary species thus tended to have low wing loading and low aspect ratio, two features that increase maneuverability during flight. Species at the slow end of the life history continuum tended to have low wing loading but contrary to the prediction aspect ratio was larger than expected.
In the case of solitary species, which cannot rely on group members to reduce predation risk, greater maneuverability through low wing loading and low aspect ratio is expected to facilitate escape from predators. Lower predation risk in more social species, by contrast, appears to have relaxed selection against high wing loading and high aspect ratio. Sociality in birds is more common in open habitats and in aquatic species (Beauchamp, 2022). As habitat and lifestyle can have a direct influence on wing morphology (Rayner, 1988), the association between sociality and wing morphology might occur indirectly through the effect of habitat and lifestyle. Indeed, demands for maneuverability are considered lower in more open habitats with fewer obstacles and high wing loading is common in aquatic species (Rayner, 1988). Nevertheless, the association between sociality and wing morphology persisted after controlling for the effect of habitat and lifestyle.
The results fits with the idea that variation in predation risk induced by sociality can shape wing morphology. Empirical research generally supports an association between predation risk and wing morphology but little attention has focused on sociality as a correlate of wing morphology. For instance, across species of birds, high aspect ratio is associated with longer flight initiation distances from approaching threats as predicted for species with less maneuverable wings (Møller et al., 2013). When exposed to a temporary increase in predation risk, species of birds often (but not always) respond by decreasing wing loading (van den Hout et al., 2010; Zimmer et al., 2011; Pascual and Senar, 2015; Walters et al., 2017) presumably to facilitate escape from predators. Swaddle and Lockwood (1998) showed that predation risk for a number of small passerines exposed to one avian predator was lower for species with a more rounded wingtip shape. While I did not measure wingtip shape, rounded wingtip shape is associated with greater lift of the sort produced by low wing loading. None of these studies considered sociality as a correlate of wing morphology. Comparing two species of shorebirds, Burns and Ydenberg (2002) found that wing loading was lower in the species foraging in the riskier habitat and that low wing loading allowed greater acceleration from a stationary position, which would result in faster escape from predators. They suggested that sociality probably played a role because the species in the more exposed habitat was also more solitary and could not rely on others as easily to detect predators quickly. My results extend this observation to a large number of species with varied ecological niches.
Wing morphology was also associated with the pace of life history. In particular, wing loading was lower in species at the slow end of the life history continuum, providing support to the idea that more risk averse species tend to have low wing loading, which would increase maneuverability and the ability to escape from predators. Species at this end of the life history continuum occupy niches that might have on their own an impact on wing morphology. For instance, life history is associated with flocking tendencies in birds (Beauchamp, 2022) and migration (Winger and Pegan, 2021). However, the results show that life history is associated with wing morphology independently of flocking and other potentially confounding variables such as migration. Species at the slow end of the continuum flee sooner when predators approach (Blumstein, 2006; Gotanda et al., 2009; Møller and Liang, 2012), which is compatible with the idea that such species are risk averse. My results show that such species also have wing traits that can reduce predation risk. This is similar to the finding that species at the slow end of the life history continuum have larger eyes than expected from their size, which would facilitate the early detection of threats (Beauchamp, 2023b).
Contrary to expectation, aspect ratio was higher for species at the slow end of the life history continuum. High aspect ratio is typically associated with an aerial lifestyle, migration, and open habitats (Rayner, 1988; Vágási et al., 2016; Evans, 2021). Such wings reduce the cost of long flights and are suited to open habitats with fewer obstacles and less demands for maneuverability. I found support for all these associations suggesting that the effect of life history on aspect ratio was independent of these ecological factors. Species at the slow end of the life history continuum often live in environments with little predation pressure on adults such as islands (Covas, 2012). Relaxed predation pressure for such species might reduce investment in maintaining a strong flight apparatus and could explain why aspect ratio is high in such species (Gill, 2023). However, relaxed predation pressure would likely be associated with high wing loading as well, but I found that wing loading was actually lower in such species ruling out relaxed predation pressure. As pointed out by other researchers, the association between ecological needs and wing morphology is complex and more work is needed to get a full picture (Baumgart et al., 2021; Lapsansky et al., 2022).
I found support for expected associations between ecological needs and wing morphology. For instance, wing loading increased with body mass, was higher in aquatic species, and lower in aerial species or in migrating species (Rayner, 1988; Minias et al., 2015; Vincze et al., 2019; Evans, 2021; Lapsansky et al., 2022). Aspect ratio, as expected, was higher in aerial species, in migratory species, and in more open habitats (Rayner, 1988; Minias et al., 2015; Vincze et al., 2019; Evans, 2021). This suggests that these effects are robust across studies with different samples of species. In addition, the inclusion of species with varied lifestyles and from various habitats reduced possible inclusion bias.
My study has limitations. While the sample size here was relatively large, I could only sample a fraction of the total number of known avian species (< 10%). Because I sampled species with a known pace of life history, the study is biased toward species from countries with a long ornithological research history such as North America, Europe, and Australia. Therefore, a broader sample of species is needed to assess the robustness of the conclusions. I focused on two wing traits (wing loading and aspect ratio), which have been used frequently in comparative analyses of flight among species (Norberg, 1990). Nevertheless, such summary measurements may not fully capture the effect of ecology on wing morphology (Rayner, 1988; Baumgart et al., 2021). For the above measurements, I also ignored the area between the two wings as well as the tail, which are all involved in providing lift during flight (Pennycuick, 1989). Tail features, in particular, have been linked to ecological needs (Norberg and Boycott, 1979; Thomas and Balmford, 1995; Hedenström, 2002). Future studies could thus used other available measurements of wing morphology and also extend the analysis to the tail. Wing morphology is shaped by different environmental factors including habitat, lifestyle, and migration, which I considered in my analysis. However, I ignored the potential use of wings for sexual displays as systematic information on their occurrence was not widely available. Nevertheless, it is clear that wing features can be adapted to the production of sexual displays (Hedenström and Møller, 1992).
In conclusion, life history and sociality can predict variation across species in two aspects of wing morphology, wing loading and aspect ratio. This study highlights the importance of predation risk acting through life history and sociality in shaping wing morphology across species of birds.
The raw data supporting the conclusions of this article will be made available by the authors, without undue reservation.
GB: Conceptualization, Formal analysis, Methodology, Writing – original draft.
The author(s) declare that no financial support was received for the research, authorship, and/or publication of this article.
The author declares that the research was conducted in the absence of any commercial or financial relationships that could be construed as a potential conflict of interest.
All claims expressed in this article are solely those of the authors and do not necessarily represent those of their affiliated organizations, or those of the publisher, the editors and the reviewers. Any product that may be evaluated in this article, or claim that may be made by its manufacturer, is not guaranteed or endorsed by the publisher.
Banks E. (1930). The relation of weight to wing area in the flight of animals. J. Malayan Br. R Asiatic Soc 8, 334–360.
Baumgart S. L., Sereno P. C., Westneat M. W. (2021). Wing shape in waterbirds: Morphometric patterns associated with behavior, habitat, migration, and phylogenetic convergence. Integr. Org Biol. 3 (1), obab011. doi: 10.1093/iob/obab011
Beauchamp G. (2014). Social predation: How group living benefits predators and prey (New York: Academic Press).
Beauchamp G. (2021). Flocking in birds increases annual adult survival in a global analysis. Oecologia. 197, 387–394. doi: 10.1007/s00442-021-05023-5
Beauchamp G. (2022). Flocking in birds is associated with diet, foraging substrate, timing of activity, and life history. Behav. Ecol. Sociobiol. 76, 74. doi: 10.1007/s00265-022-03183-9
Beauchamp G. (2023a). Susceptibility to predation varies with body mass, foraging niche, and anti-predator responses among bird species. Birds. 4, 73–84. doi: 10.3390/birds4010006
Beauchamp G. (2023b). Life history and sociality predict variation in eye size across birds. Birds. 4, 284–294. doi: 10.3390/birds4030024
Bednekoff P. A. (1996). Translating mass dependent flight performance into predation risk: an extension of Metcalfe & Ure. Proc. R. Soc. London Ser. B: Biol. Sci. 263, 887–889. doi: 10.1098/rspb.1996.0131
Biewener A. A. (2011). Muscle function in avian flight: achieving power and control. Philos. Trans. R. Soc. B: Biol. Sci. 366, 1496–1506. doi: 10.1098/rstb.2010.0353
Blumstein D. T. (2006). Developing an evolutionary ecology of fear: how life history and natural history traits affect disturbance tolerance in birds. Anim. Behav. 71, 389–399. doi: 10.1016/j.anbehav.2005.05.010
Brown C. R., Brown M. B. (2004). Group size and ectoparasitism affect daily survival probability in a colonial bird. Behav. Ecol. Sociobiol. 56, 498–511. doi: 10.1007/s00265-004-0813-6
Burns J. G., Ydenberg R. C. (2002). The effects of wing loading and gender on the escape flights of least sandpipers (Calidris minutilla) and western sandpiper (Calidris mauri). Behav. Ecol. Sociobiol. 52, 128–136. doi: 10.1007/s00265-002-0494-y
Caro T. M. (2005). Antipredator defenses in birds and mammals (Chicago: University of Chicago Press).
Cash K. J., McKee M. H., Wrona F. J. (1993). Short- and long-term consequences of grouping and group foraging in the free-living flatworm Dugesia tigrina. J. Anim. Ecol. 62, 529–535. doi: 10.2307/5202
Clutton-Brock T. H., Gaynor D., McIlrath G. M., Maccoll A. D. C., Kansky R., Chadwick P., et al. (1999). Predation, group size and mortality in a cooperative mongoose, Suricata suricatta. J. Anim. Ecol. 68, 672–683. doi: 10.1046/j.1365-2656.1999.00317.x
Covas R. (2012). Evolution of reproductive life histories in island birds worldwide. Proc. R Soc. Lond B: Biol. Sci. 279, 1531–1537. doi: 10.1098/rspb.2011.1785
Cruz-Angón A., Sillett T. S., Greenberg R. (2008). An experimental study of habitat selection by birds in a coffee plantation. Ecology. 89, 921–927. doi: 10.1890/07-0164.1
Desrochers A. (2010). Morphological response of songbirds to 100 years of landscape change in North America. Ecology. 91, 1577–1582. doi: 10.1890/09-2202.1
Dial K. P. (2003). Evolution of avian locomotion: Correlates of flight style, locomotor modules, nesting biology, body size, development, and the origin of flapping flight. Auk. 120, 941–952. doi: 10.1642/0004-8038(2003)120[0941:EOALCO]2.0.CO;2
Dudley R. (2002). Mechanisms and implications of animal flight maneuverability. Integr. Comp. Biol. 42, 135–140. doi: 10.1093/icb/42.1.135
Elliott K. H., Ricklefs R. E., Gaston A. J., Hatch S. A., Speakman J. R., Davoren G. K. (2013). High flight costs, but low dive costs, in auks support the biomechanical hypothesis for flightlessness in penguins. Proc. Natl. Acad. Sci. 110, 9380–9384. doi: 10.1073/pnas.1304838110
Evans S. W. (2021). The wing morphology traits of resident birds that spend a large amount of time per day flying are similar to those of migrant birds. J. Ornithol. 162, 765–778. doi: 10.1007/s10336-021-01870-4
Fu H., Su M., Chu J. J., Margaritescu A., Claramunt S. (2023). New methods for estimating the total wing area of birds. Ecol. Evol. 13, e10480. doi: 10.1002/ece3.10480
Ghalambor C. K., Martin T. E. (2001). Fecundity-survival trade-offs and parental risk-taking in birds. Science. 292, 494–497. doi: 10.1126/science.1059379
Gotanda K. M., Turgeon K., Kramer D. L. (2009). Body size and reserve protection affect flight initiation distance in parrotfishes. Behav. Ecol. Sociobiol. 63, 1563–1572. doi: 10.1007/s00265-009-0750-5
Greenewalt C. W. (1962). Dimensional relationships for flying animals. Smithsonian Miscellaneous Collections. 144, 1–46.
Greenewalt C. W. (1975). The flight of birds: The significant dimensions, their departure from the requirements for dimensional similarity, and the effect on flight aerodynamics of that departure. Tr Amer Philo Soc 65, 1–67. doi: 10.2307/1006161
Grilli M. G., Lambertucci S. A., Therrien J.-F., Bildstein K. L. (2017). Wing size but not wing shape is related to migratory behavior in a soaring bird. J. Avian Biol. 48, 669–678. doi: 10.1111/jav.01220
Hackett S. J., Kimball R. T., Reddy S., Bowie R. C. K., Braun E. L., Braun M. J., et al. (2008). A phylogenomic study of birds reveals their evolutionary history. Science. 320, 1763–1768. doi: 10.1126/science.1157704
Hartman F. A. (1961). “Locomotor mechanisms of birds,” in Smithsonian Miscellaneous Collections (Washington and Smithsonian Institution), vol. 143.
Healy K., Guillerme T., Finlay S., Kane A., Kelly S. B. A., McClean D., et al. (2014). Ecology and mode-of-life explain lifespan variation in birds and mammals. Proc. R Soc. Lond B: Biol. Sci. 281, 20140298. doi: 10.1098/rspb.2014.0298
Hedenström A. (2002). Aerodynamics, evolution and ecology of avian flight. Trends Ecol. Evol. 17, 415–422. doi: 10.1016/S0169-5347(02)02568-5
Hedenström A., Møller A. P. (1992). Morphological adaptations to song flight in passerine birds: a comparative study. Proc. R Soc. Lond B: Biol. Sci. 247, 183–187. doi: 10.1098/rspb.1992.0026
Hedenstrom A., Rosen M. (2001). Predator versus prey: on aerial hunting and escape strategies in birds. Behav. Ecol. 12, 150–156. doi: 10.1093/beheco/12.2.150
Ives A., Dinnage R., Nell L. A., Helmus M., Li D. (2023) phyr: Model Based Phylogenetic Analysis, version 1.1.2. Available at: https://daijiang.github.io/phyr/.
Jullien M., Clobert J. (2000). The survival value of flocking in neotropical birds: Reality or fiction? Ecology 81, 3416–3430. doi: 10.1890/0012-9658(2000)081[3416:TSVOFI]2.0.CO;2
Krams I. (2002). Mass-dependent take-off ability in wintering great tits (Parus major): comparison of top-ranked males and subordinate juvenile females. Behav. Ecol. Sociobiol. 51, 345–351. doi: 10.1007/s00265-002-0452-8
Lapsansky A. B., Warrick D. R., Tobalske B. W. (2022). High wing-loading correlates with dive performance in birds, suggesting a strategy to reduce buoyancy. Integr. Comp. Biol. 62, 878–889. doi: 10.1093/icb/icac117
Minias P., Meissner W., Włodarczyk R., Ożarowska A., Piasecka A., Kaczmarek K., et al. (2015). Wing shape and migration in shorebirds: a comparative study. Ibis. 157, 528–535. doi: 10.1111/ibi.12262
Møller A. P., Liang W. (2012). Tropical birds take small risks. Behav. Ecol. 24, 267–272. doi: 10.1093/beheco/ars163
Møller A. P., Vágási C. I., Pap P. L. (2013). Risk-taking and the evolution of mechanisms for rapid escape from predators. J. Evol. Biol. 26, 1143–1150. doi: 10.1111/jeb.12147
Norberg U. M., Boycott B. B. (1979). Morphology of the wings, legs and tail of three coniferous forest tits, the goldcrest, and the treecreeper in relation to locomotor pattern and feeding station selection. Philos. Trans. R Soc. B-Biol Sci. 287, 131–165. doi: 10.1098/rstb.1979.0054
O’Brien R. M. (2007). A caution regarding rules of thumb for variance inflation factors. Qual. Quantity. 41, 673–690. doi: 10.1007/s11135-006-9018-6
Paradis E., Claude J., Strimmer K. (2004). APE: analyses of phylogenetics and evolution in R language. Bioinformatics. 20, 289–290. doi: 10.1093/bioinformatics/btg412
Pascual J., Senar J. C. (2015). Resident but not transient Eurasian Siskins reduce body mass in response to increasing predation risk: a natural experiment. J. Ornithol. 156, 451–456. doi: 10.1007/s10336-014-1143-8
Pennycuick C. J. (1989). Bird flight performance: a practical calculation manual (Oxford: Oxford University Press).
Poole E. L. (1938). Weights and wing areas in North American Birds. Auk. 55, 511–517. doi: 10.2307/4078421
Rayner J. M. V. (1988). Form and function in avian flight. Curr. Ornithol. 5, 1–66. doi: 10.1007/978-1-4615-6787-5_1
Revell L. J. (2012). phytools: An R package for phylogenetic comparative biology (and other things). Methods Ecol. Evol. 3, 217–223. doi: 10.1111/j.2041-210X.2011.00169.x
Saino N., Ambrosini R., Caprioli M., Liechti F., Romano A., Rubolini D., et al. (2017). Wing morphology, winter ecology, and fecundity selection: evidence for sex-dependence in barn swallows (Hirundo rustica). Oecologia. 184, 799–812. doi: 10.1007/s00442-017-3918-0
Serrano D., Oro D., Ursúa E., Tella J. L. (2005). Colony size selection determines adult survival and dispersal preferences: Allee effects in a colonial bird. Am. Nat. 166, E22–E31. doi: 10.1086/431255
Shultz S., Noë R., McGraw W. S., Dunbar R. I. M. (2004). A community-level evaluation of the impact of prey behavioural and ecological characteristics on predator diet composition. Proc. R Soc. Lond B Biol. Sci. 271, 725–732. doi: 10.1098/rspb.2003.2626
Srinivasan U. (2019). Morphological and behavioral correlates of long-term bird survival in selectively logged forest. Front. Ecol. Evol. 7, 17. doi: 10.3389/fevo.2019.00017
Swaddle J. P., Lockwood R. (1998). Morphological adaptations to predation risk in Passerines. J. Avian Biol. 29, 172–176. doi: 10.2307/3677195
Thomas A. L. R., Balmford A. (1995). How natural selection shapes birds’ tails. Am. Nat. 146, 848–868. doi: 10.1086/285828
Tobias J. A., Sheard C., Pigot A. L., Devenish A. J. M., Yang J. X., Sayol F., et al. (2022). AVONET: morphological, ecological and geographical data for all birds. Ecol. Lett. 25, 581–597. doi: 10.1111/ele.13898
Vágási C. I., Pap P. L., Vincze O., Osváth G., Erritzøe J., Møller A. P. (2016). Morphological adaptations to migration in birds. Evol. Biol. 43, 48–59. doi: 10.1007/s11692-015-9349-0
van den Hout P. J., Mathot K. J., Maas L. R. M., Piersma T. (2010). Predator escape tactics in birds: linking ecology and aerodynamics. Behav. Ecol. 21, 16–25. doi: 10.1093/beheco/arp146
Van Hooydonck B., Herrel A., Gabela A., Podos J. (2009). Wing shape variation in the medium ground finch (Geospiza fortis): an ecomorphological approach. Biol. J. Linn. Soc 98, 129–138. doi: 10.1111/j.1095-8312.2009.01269.x
Vincze O., Vágási C. I., Pap P. L., Palmer C., Møller A. P. (2019). Wing morphology, flight type and migration distance predict accumulated fuel load in birds. J. Exp. Biol. 222, 183517. doi: 10.1242/jeb.183517
Walters B. T., Cheng T. N. N., Doyle J., Guglielmo C. G., Clinchy M., Zanette L. Y. (2017). Too important to tamper with: predation risk affects body mass and escape behaviour but not escape ability. Func Ecol. 31, 1405–1417. doi: 10.1111/1365-2435.12851
Wang X., Clarke J. A. (2015). The evolution of avian wing shape and previously unrecognized trends in covert feathering. Proc. R Soc. Lond B: Biol. Sci. 282, 20151935. doi: 10.1098/rspb.2015.1935
Williams C. K., Lutz R. S., Applegate R. D. (2003). Optimal group size and northern bobwhite coveys. Anim. Behav. 66, 377–387. doi: 10.1006/anbe.2003.2215
Winger B. M., Pegan T. M. (2021). Migration distance is a fundamental axis of the slow-fast continuum of life history in boreal birds. Ornithology. 138, 1–18. doi: 10.1093/ornithology/ukab043
Keywords: annual adult survival, aspect ratio, flocking, phylogenetic linear mixed model, wing loading
Citation: Beauchamp G (2023) Is wing morphology across birds associated with life history and sociality? Front. Bird Sci. 2:1305453. doi: 10.3389/fbirs.2023.1305453
Received: 01 October 2023; Accepted: 21 November 2023;
Published: 06 December 2023.
Edited by:
Thomas Oliver Mérö, Hungarian Academy of Science, HungaryReviewed by:
Sang-im Lee, Daegu Gyeongbuk Institute of Science and Technology (DGIST), Republic of KoreaCopyright © 2023 Beauchamp. This is an open-access article distributed under the terms of the Creative Commons Attribution License (CC BY). The use, distribution or reproduction in other forums is permitted, provided the original author(s) and the copyright owner(s) are credited and that the original publication in this journal is cited, in accordance with accepted academic practice. No use, distribution or reproduction is permitted which does not comply with these terms.
*Correspondence: Guy Beauchamp, Z3V5Z2lsbGVzYmVhdWNoYW1wQGdtYWlsLmNvbQ==
Disclaimer: All claims expressed in this article are solely those of the authors and do not necessarily represent those of their affiliated organizations, or those of the publisher, the editors and the reviewers. Any product that may be evaluated in this article or claim that may be made by its manufacturer is not guaranteed or endorsed by the publisher.
Research integrity at Frontiers
Learn more about the work of our research integrity team to safeguard the quality of each article we publish.