- 1Centre for Immunology and Allergy Research, Westmead Institute for Medical Research, Westmead, NSW, Australia
- 2School of Medical Sciences, Faculty of Medicine and Health, The University of Sydney, Sydney, NSW, Australia
Nanoparticles hold a great potential for therapeutic targeting due to their ability to improve the stability of encapsulated cargo and promote the transport of cargo across membranes to reach to the target site. Most commercially available nanomedicines are simple synthetic liposomes, however, there are numerous side effects due to their off-target delivery and rapid clearance from the bloodstream. Recently, attention has moved toward extracellular vesicles (EVs)–lipid bilayer enclosed particles released by cells (size ranging from 30 to 10,000 nm in diameter). EVs carry and transport lipids, proteins, and nucleic acids from their parental cells to recipient cells, hence they play a key role in intercellular communication. The ability of EVs to cross biological barriers including the blood brain barrier has generated significant attention to explore them as potential biomarkers and natural drug delivery vehicles for various therapeutics and small molecules. EVs have also been implicated in disease pathogenesis by transmitting pathogenic proteins between cells, making them promising biomarkers for disease diagnosis and monitoring. In this review, we will focus on the potential and challenges of EVs as biomarkers, drug delivery vehicles and next-generation therapeutics. Finally, we will explore misfolded protein disorders, amyloidosis, as a case study for how EVs may contribute to disease pathology and how EVs could be applied in the clinic as diagnostic and prognostic biomarkers of amyloid diseases.
Introduction
Nanoparticles have been considered as the holy grail for effective drug delivery vehicles. The ideal nanoparticle shuttles the drug-load or “cargo” safely to a desired target. The advantage of nanoparticles is their small size, which enables them to move freely throughout the body and access target sites where bigger materials cannot reach (van der Koog et al., 2022). In addition, nanoparticles can prevent undesirable interactions between the drug-load and non-target tissues, hence reduce toxicity and increase stability of the encapsulated drug in the circulation (van der Koog et al., 2022). Liposomes and micelles are among the first generation of nanoparticle-based drug delivery. These can contain gold, magnetic or other inorganic nanoparticles, which can be used for imaging and therapeutic functions. Despite the evident benefits of liposomes for drug delivery, clinical application has been limited due to their speedy clearance from the circulation, accumulation in off-target organs and initiating innate immune responses (Sercombe et al., 2015).
Recently, extracellular vesicles (EVs) have emerged as a next-generation nanoparticle-based therapy. EVs are lipid nanoparticles secreted from all cells, ranging in size from 30 to 4 µm and are comprised of biological entities such as proteins, mRNA, miRNA, and lipids (Kalluri and LeBleu, 2020). Their composition is closely linked to the contents and physiological status of their original cells, meaning that they can be used to detect abnormal cells (Kalluri and LeBleu, 2020). EVs obtain superior drug delivery capacity in comparison to liposomes due to their complex components, which can foster tissue targeting and reduce non-specific interactions (Mathieu et al., 2019). Additionally, EVs are stable in biological fluids and their composition protects against exogenous RNases and proteases (Boukouris and Mathivanan, 2015). This feature makes EVs an ideal diagnostic biomarker for numerous diseases. Moreover, EVs are promising candidates for further bioengineering, for instance to modify their content and serve as drug delivery vehicles.
In this review, we will focus on the potential of EVs in diagnostics and therapeutics. We will elaborate on the engineering strategies of EVs to maximise their therapeutic potential in drug delivery. We will also explore the challenges of translating EVs to the clinic and discuss the potential use of EVs in a clinical application–misfolded protein disease, amyloidosis. Amyloidosis presents with a wide range of non-specific clinical symptoms and there is no approach to predict which organs amyloid proteins deposit and cause damage. Recent studies demonstrate that EVs contain amyloid and promote its deposition (Yamaguchi et al., 2022). The role of EVs in amyloid disease pathology will be discussed as well as prospects for EVs as diagnostic and prognostic biomarkers.
1.1 Classification and biogenesis of EVs
EVs are currently classified into exosomes, microvesicles, microparticles, ectosomes, oncosomes, apoptotic bodies, and exomeres (Gurunathan et al., 2021). These classifications are defined by the mechanism of biogenesis of each subtype as well as their biophysical properties (Phan et al., 2022). Exosomes are a major subtype comprised of “small” vesicles (30–150 nm) derived from intracellular endosomal compartments. Exosomes form as intraluminal vesicles and are secreted by the merging of multivesicular bodies with the plasma membrane (Harding et al., 1983). Rab guanosine triphosphate (GTPases), including Rab7A, Rab11, Rab27A, Rab27B, and Rab35, were found to regulate the trafficking of multivesicular bodies to plasma membrane (Tai et al., 2018). Microvesicles are another major subtype of EVs comprised of “larger” vesicles (200 nm to 1–2 µm) (Doyle and Wang, 2019). Microvesicles are often referred to as shedding vesicles or ectosomes because they are produced at the plasma membrane and oncosomes when they contain oncogenic components (Desrochers et al., 2016). The biogenesis of microvesicles is mediated by ARF6 and RHOA, which rearrange the actin cytoskeleton (Li et al., 2012). Apoptotic bodies are another subtype of EVs, which are 1–5 µm in size and released from membrane blebs of cells undergoing programmed cell death (Caruso and Poon, 2018). Non-membranous nanoparticles (less than 50 nm) were recently identified and termed exomeres. However, the classification of EVs is challenging as there is a significant overlap in size range, no consensus biomarkers to define subtypes and the mechanisms for biogenesis of many EVs (e.g., exomeres) is unknown (Zhang et al., 2018). Therefore, all naturally released particles from cells containing a lipid bilayer that cannot replicate are broadly defined as EVs (Théry et al., 2018).
1.2 Composition of EVs
EVs are comprised of nucleic acids, transcriptional regulators, signalling proteins, and lipids, which are derived from their cell of origin (Figure 1). For instance, EVs secreted from lung epithelial cells contain lung surfactant proteins (Lee et al., 2017). EVs contain cell-specific proteins that can be used to determine their source and could serve as biomarkers of disease manifestation and severity. For example, EVs isolated from the serum of patients with pancreatic cancer contained high levels of glypican-1 (GPC1), a cell surface proteoglycan highly expressed in pancreatic cancer but not in normal pancreas or chronic pancreatitis (Kleeff et al., 1998). GPC1 positive EVs correlated with tumour burden and patient survival pre- and post-surgical tumour resection (Melo et al., 2015).
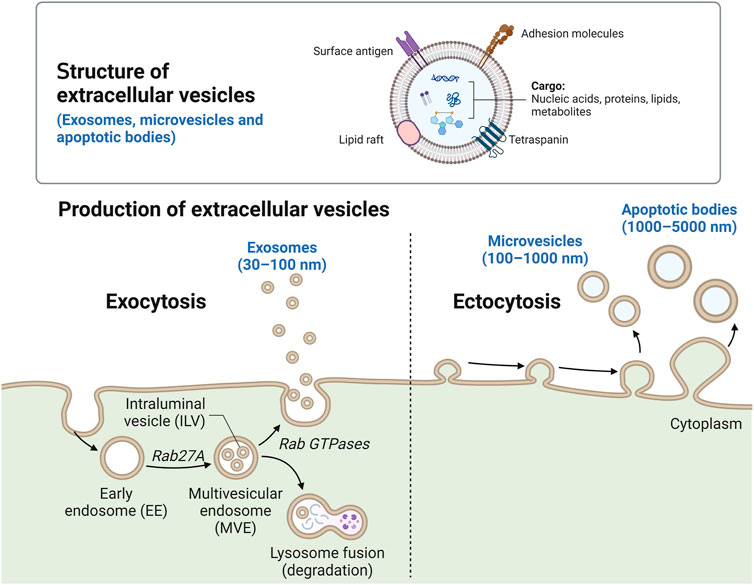
FIGURE 1. Schematic of EV molecular composition and biogenesis. The key molecular components of EVs include membrane proteins, tetraspanins, lipid rafts, surface antigens, nucleic acids, metabolites, and intracellular proteins. The biogenesis of three main types of EVs–exosomes (30–150 nm), microvesicles (100–1,000 nm) and apoptotic bodies (1,000–5,000 nm). Created with Biorender.
Exosomes, a common subtype of EVs, contain proteins needed for transport and fusion with other cell membranes (GTPases, Annexins and flotillin), tetraspanins (CD9, CD63, CD81 and CD82), heat shock proteins (Hsc70, Hsp90), and proteins associated with the development of multivesicular bodies (Alix, TSG101). Exosomes possess unique lipid-related proteins and phospholipases (Zhang et al., 2012), enriched with cholesterol, ceramide or other sphingolipids, and phosphoglycerides with long and saturated fatty-acyl chains (Trajkovic et al., 2008). There is less information for classification of other EVs such as microvesicles or apoptotic bodies. However, the most common markers for microvesicles are CD40 ligand, adenosine diphosphate ribosylation factor 6, several integrins and selectins (Pugholm et al., 2015). Annexin V, thrombospondin, and C3b are considered specific markers for apoptotic bodies (Savill et al., 1992).
1.3 EV functions
Given that EVs reflect the components of their parental cells that secrete them in response to local signalling and stimuli (Bazzan et al., 2021), EVs can play a significant role as (a) biological mediators, (b) diagnostic biomarkers and (c) drug delivery vehicles (Figure 2).
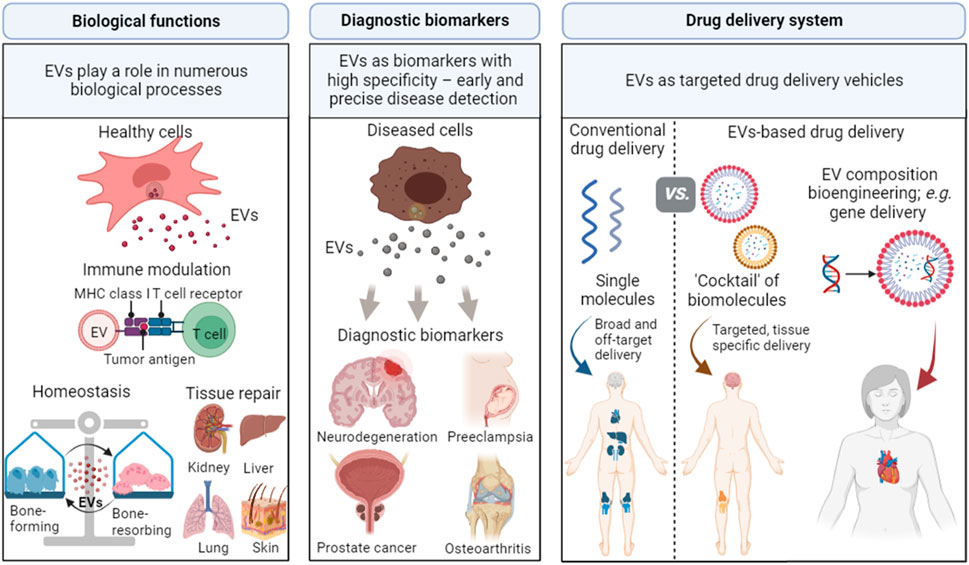
FIGURE 2. Applications of EVs in biological processes, diagnostic biomarkers, and drug delivery vehicles. EVs play important roles in biological processes as they can alter immune responses, maintain cell homeostasis, and promote tissue regeneration. EVs can be involved in specific disease processes and are promising biomarkers for numerous diseases, including neurodegeneration, preeclampsia, prostate cancer, and osteoarthritis. EVs can also be used as drug delivery vehicles due to their capability to target specific sites and cross biological barriers. Created with BioRender.com.
1.3.1 Biological functions
Due to their rich composition and capacity to interact with other cells, EVs function in many biological processes including the efficient removal and delivery of molecules between cells to modulate intercellular communication, cell maturation and adaption to environmental changes (Buzas, 2023). For example, in the context of the central nervous system, EVs derived from astrocytes appear to facilitate neuroprotection by shuttling neuroglobin to neurons, which exhibits antioxidant, anti-apoptotic and anti-inflammatory effects (Venturini et al., 2019). EVs derived from osteoblasts and osteoclasts also showed the ability to enhance both osteogenesis and osteoclastogenesis to maintain bone homeostasis (Pieters et al., 2019). EVs can control biological functions directly by triggering surface receptors on recipient cells or indirectly by fusing with the plasma membrane and releasing nucleic acids, genes, proteins or infectious agents in recipient cells. Through these actions, EVs have been shown to play an important role in stem cell maintenance (Ratajczak et al., 2006), tissue repair (Gatti et al., 2011), immune surveillance (Raposo et al., 1996), and blood coagulation (Del Conde et al., 2005).
In the context of the immune system, EVs have immunomodulatory effects, as evidenced by the ability to transfer antigens between antigen presenting cells (APCs). Indeed, EVs carry pre-formed functional peptide-MHC complexes from APCs such as dendritic cells to recipient cells, which stimulate maturation and differentiation in immature dendritic cells and monocytes (Wei et al., 2017; Schierer et al., 2018). EVs derived from dendritic cells also promote interferon-gamma (IFN- γ) production by naïve CD4+ T cells and trigger the differentiation into T helper 1, T helper 2 and regulatory T (Treg) cells (Kowal et al., 2016). EVs derived from T cells themselves have also emerged as critical immunomodulators. Small EVs derived from T cells were enriched with mitochondrial DNA (mtDNA), which primed dendritic cells to a specific antigen (Torralba et al., 2018). EVs derived from T cells are also capable of encapsulating immunomodulatory cytokines, enhancing cytokine stability in comparison to their free forms (Fitzgerald et al., 2018). The stabilizing effect of EVs on the encapsulated cargo has been exploited for drug delivery and biomarker applications (see sections 1.3.2 and 1.3.3).
EVs have also been implicated in triggering inflammatory pathways. Blood-derived EVs from patients with dermatomyositis contained double stranded DNA that stimulated the STING pathway to induce pro-inflammatory cytokine secretion (e.g., type I interferon) (Li et al., 2021). EVs have also been shown to influence the polarization of macrophages to enhance inflammation. EVs enriched with miRNA-155 are abnormally elevated in inflammatory bowel disease, acute lung injury, myocardial ischemia reperfusion injury and triggers macrophage polarization toward an inflammatory (M1) phenotype (Tang et al., 2022). The development of inhibitors that target EV-mediated inflammation and disease progression could be a new approach for treating inflammatory diseases.
A multifaceted role for EVs is reinforced with their ability to regenerate/repair tissue and damaged organs. EVs can transfer microRNAs, messenger RNAs and proteins, which stimulate mitogen-activated protein kinase, Wnt/β-catenin, PI3K/Akt, Notch, TGFβ/Smad, STAT and Hedgehog signalling, which have been linked to enhanced proliferation, migration and survival of recipient cells (Nagelkerke et al., 2021). Notably, EVs have also been shown to downregulate inflammation, attenuate cytokine storm, and promote angiogenesis, as well as trigger lineage-specific cell differentiation, which are critical to promote functional tissue repair/regeneration (Nagelkerke et al., 2021).
1.3.2 Potential of EVs as a biomarker
Since EVs can be secreted by all cells in the body in response to specific conditions (both physiological and pathological), EVs have been proposed as promising novel biomarkers for numerous diseases. EVs possess competitive advantages compared to traditional biomarkers, including (i) the ability to reflect disease progression and monitor treatment response via vesicle origin and their loaded cargo, (ii) their structure, which protects encapsulated cargo during long-term storage and freeze/thaw cycles; and (iii) the stability of EVs in body fluids (Lai et al., 2013; Tian et al., 2020). Therefore, EVs may have utility to predict flares in diseases that fluctuate over time such as, autoimmune diseases Sjögren’s syndrome, systemic lupus erythematosus and systemic sclerosis (Cecchettini et al., 2019).
In the context of diagnosis of autoimmune diseases, numerous tests can be conducted on biological fluids to examine autoimmune markers, such as autoantibodies and inflammatory signals. However, identical markers can be presented across numerous autoimmune disorders, which can make diagnosis uncertain and lack specificity (Castro and Gourley, 2010). Circulating EVs may uncover tissue-specific autoimmune-associated protein expression to improve diagnostic specificity (Cecchettini et al., 2019). An increase of platelet-derived EVs and endothelial microparticles was found in patients with Sjögren’s syndrome compared to healthy controls (Bartoloni et al., 2015). Another study found that 36 proteins associated with activation of the innate immune system (i.e., SIRPA and LSP1), were upregulated in EVs from the saliva of patients with Sjögren’s syndrome in comparison with healthy controls (Aqrawi et al., 2017). Other studies showed that hsa-miR-768-3p and hsa-miR-574-3p in the EVs derived from saliva can be utilized as biomarkers for inflammation and salivary gland dysfunction in Sjögren’s syndrome (Michael et al., 2010; Alevizos et al., 2011).
Due to their unique properties obtained from their originating cells, EVs can also be used to monitor disease progression and therapy in real time. In cancer, EVs represent relevant tumour constitutes during each stage of cancer management. For instance, EVs have been used recently as a predictive biomarker for outcome of checkpoint inhibitor therapy, anti-PD-1 (programmed death-1) (De Rubis et al., 2019). Additionally, cancer-derived EVs carrying functional programmed death-ligand 1 (PD-L1) on their surface could serve as biomarkers for therapy resistance (Chen et al., 2018).
1.3.3 Potential of EVs in drug delivery
EVs possess an inherent capability to target specific regions, tissues, and organs (Walker et al., 2019). Harnessing this capability would enable targeted delivery of therapeutics and thus reduce the side effects due to off target delivery and toxicity associated with conventional vehicles such as synthetic nanoparticles (liposomes, polymeric nanoparticles) (de Jong et al., 2020). It has been shown that the accumulation of liposomes at high doses in macrophages can influence their phagocytic activity, resulting in immunosuppression and increasing the risk of infections (Sercombe et al., 2015). In stark contrast to conventional delivery vehicles, EVs exhibit low toxicity and low immunogenicity, can penetrate blood vessels, and enter the extracellular matrix, and have intrinsic ability to cross physical barriers (e.g., blood-brain barrier) (Murphy et al., 2019a).
EVs contain unique composition that can interact specifically with selected target cells and allow the transfer of cargo to recipient cells. The protein contents and membrane protein influence the targeting behaviour of EVs. For instance, EVs expressing integrin α6 in complex with subunits β1 and β4 are preferentially targeted to S100-A4-positive fibroblasts and surfactant protein C-positive epithelial cells in the lungs (Hoshino et al., 2015). Meanwhile, EVs exhibiting subunit β5 are directed to Kupffer cells in the liver and EVs expressing β4 are targeted to CD31-positive endothelial cells in the brain (Hoshino et al., 2015). The lipid composition of EVs also alters their targeting behaviour, the strongest evidence being the targeting of macrophages via detection of phosphatidylserine on the surface of EVs. Phosphatidylserine-containing liposomes have been found to decrease EV uptake by competing with and thereby obstructing EV uptake by macrophages (Matsumoto et al., 2017).
Another important characteristic of EVs is their intrinsic capability to cross biological barriers such as the blood brain barrier, which is particularly difficult to breach with synthetic drug delivery vehicles. Alvarez-Erviti et al. demonstrated that intravenous injection of exosomes isolated from dendritic cells loaded with exogenous siRNA can knockdown BACE1, a gene implicated in Alzheimer’s disease, in particular areas of the brain (Alvarez-Erviti et al., 2011). Moreover, repeated administration of EVs did not decrease the efficacy of gene knockdown, as often seen for viral particles due to their detection by the immune system (van Haasteren et al., 2018). Further evidence showed that brain-derived EVs can be detected in peripheral blood, demonstrating that EVs can cross the blood brain barrier (Vandendriessche et al., 2020). Nevertheless, surface modifications for exosomes should be implemented to improve the targeting ability and achieve brain delivery after systemic administration (Tian et al., 2018). In addition, EVs derived from mammalian cells appear to be biocompatible across species. A previous study demonstrated that intravenous administration of bovine milk derived EVs to mice resulted in moderate cytokine release and no other adverse effects (Somiya et al., 2018). EVs are non-replicative and non-mutagenic, exhibiting low immunogenicity and low toxicity. Moreover, their biological barrier permeability and intrinsic cell targeting make EVs promising candidates for drug delivery systems.
Nevertheless, few aspects of the fundamental biology of EVs remain to be elucidated. There has been relatively little work considering the presence of “corona” on the surface of EVs that can alter interactions with recipient cells (Monopoli et al., 2012). Protein corona form readily on the EV surface (Palviainen et al., 2020), and the enzymatic removal of the corona from EVs has been shown to inhibit the cellular uptake of EVs (Escrevente et al., 2011). While corona clearly impacts on the fate of EV in biological systems and may have a pivotal implication on mechanisms of action of EVs, this area has only recently acknowledged in EV research and the studies on EV-corona remain in its infancy.
Additionally, the routes of EV uptake are highly divergent and depend on EV subtype and cell source (Mulcahy et al., 2014). It is possible that a specific uptake route may result in a greater amount of delivered cargo to the recipient cells than other routes. Elucidating this uptake pathway may increase the efficacy and efficiency of EV-based therapeutic strategies. Other challenges related to the translation of EVs based therapeutics to clinic including EV preparations, scalability, analytics, and regulation will be discussed below (Section 1.5).
1.4 Strategies to tailor EVs for different applications
EVs are surrounded by a hydrophobic lipid bilayer, which can be temporarily opened to enable the “insertion” of exogenous molecules within their core, allowing EVs’ to be enriched with the desired cargo such as therapeutic biomolecules (Murphy et al., 2019b). There are two main routes to encapsulate cargo into EVs, cell-based loading and non-cell-based loading (Han et al., 2021) (Figure 3). In cell-based loading, drugs or small biomolecules are first delivered into the parent cells and then packaged into EVs (Kanada et al., 2015). Whereas, in non-cell-based loading approaches, drugs or small biomolecules are directly inserted into EVs after their isolation from cells (Familtseva et al., 2019).
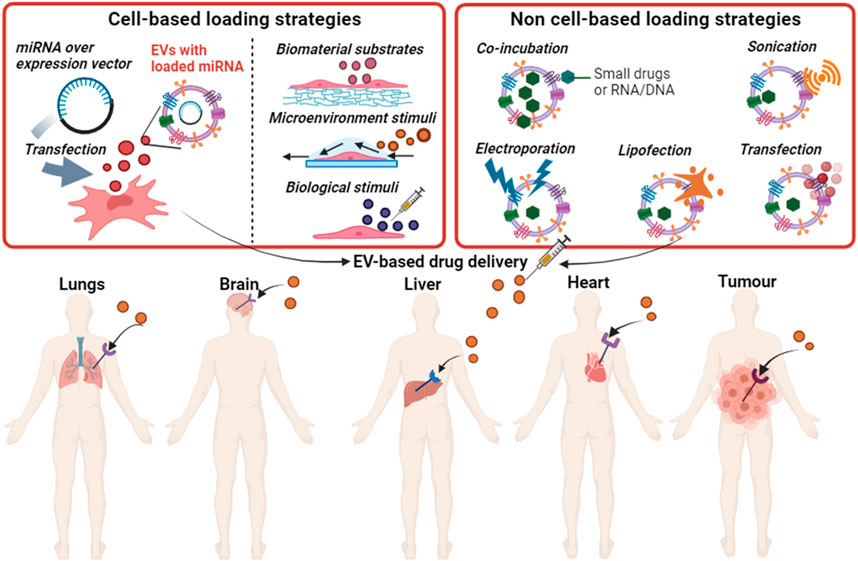
FIGURE 3. Loading strategies for small drugs or nucleic acids into EVs and the potential use of EVs in drug delivery to specific tissues. Produced in BioRender.com.
1.4.1 Cell-based loading of cargo
For the cell-based loading of cargo into EVs, numerous cellular preconditioning strategies have been applied to modify the composition of released EVs. Incubation and transfection of drugs are two commonly used strategies to load the cargo into the parent cells. For example, a small drug doxorubicin (DOX) has been successfully loaded into EVs derived from pancreatic cancer cells (PCCs), pancreatic stellate cells (PSCs) and macrophages by incubating these cells with DOX for 48 h (Kanchanapally et al., 2019). All the cells released the DOX-loaded EVs, but PCCs achieved the highest efficacy, which could be due to the increased efficiency of cancer cells to efflux drugs compared to other cell types (Borst, 2012). Direct transfection is another method used widely for loading miRNA into EVs (Han et al., 2021). One study showed that transfected HEK 293T cells with miR-21 released EVs enriched with miR-21, which decreased the viability of glioma cell lines (U87-MG, C6) (Monfared et al., 2019).
Additionally, it should be noted that preconditioning the parent cells in different environments can alter the cargo of released EVs. For example, ischemic preconditioning of mesenchymal stem cells (MSCs) resulted in the secretion of miR-22-enriched exosomes, which decreased cardiomyocyte apoptosis, fibrosis and enhanced cardiac function in a murine model of myocardial infarction (Feng et al., 2014a). Furthermore, cardiac stem cells preconditioned with heat shock released exosomes enriched in heat shock factor 1, which were delivered to cardiomyocytes and decreased apoptosis in a mouse model (Feng et al., 2014b). Culturing cells on different substrates or matrices may also affect the cargo within EVs. Indeed, a high abundance of the angiogenic factors VEGF-A and IL-8 were contained in EVs derived from human bone marrow-derived MSCs that were cultured in hollow-fibre bioreactors (Gobin et al., 2021). Another recent study showed that MSCs treated with bioglass, which provided calcium and phosphate ions to the cells, produced EVs with different composition compared to EVs derived from untreated MSCs (Wu et al., 2021). Specifically, the level of miR-342-5p was downregulated and miR-1290 was upregulated in EVs derived from bioglass treated MSCs, and these EVs showed an enhanced capability of promoting vascularization and intradermal angiogenesis of endothelial cells.
1.4.2 Non-cell-based loading of cargo
Exogenous nucleic acids (siRNA, miRNA, mRNA) or small drugs can be directly inserted into EVs using different approaches, such as electroporation, sonication, chemical transfection reagent (lipofection), co-incubation, or calcium chloride (Li et al., 2018). A recent study showed that an anti-KRASG12D siRNA was successfully loaded into MSC-derived EVs by electroporation and mice treated with the resulting EVs showed enhanced survival in a model of pancreatic cancer (Mendt et al., 2018). Another study successfully loaded siRNA into EVs by sonication, and these EVs induced a 50% knockdown of a target oncogene (Lamichhane et al., 2016). SiRNA was also loaded into EVs by lipofection, these EVs caused selective silencing of the RAD51 gene in recipient cells, which resulted in reproductive cancer cell death (Shtam et al., 2013). However, a major drawback of this approach is that the function of exogenous nucleic acids within EVs might be compromised when taken up by the recipient cells as electroporation can introduce precipitation and aggregation of siRNA; this might limit the application of this approach (Kooijmans et al., 2013).
Notably, the potential of EVs to change a paradigm in drug delivery is evident in a billion-dollar partnership between Evox Therapeutics Ltd. and Eli Lilly Co. to use exosome technology to deliver RNA-based therapeutics to the central nervous system. In summary, EVs are promising delivery vehicles for precision medicine that could enhance the therapeutic efficacy of different molecules, while reducing potential side effects and thus ultimately improving quality of life for patients.
1.5 Considerations for developing EVs for diagnostic and therapeutic approaches
Despite the huge potential of EVs as next-generation diagnostic and therapeutic agents, there are some challenges that hamper the current progress of EVs into clinical practice. These include: (i) lack of standardisation method to isolate, purify and characterise EVs, (ii) lack of reliable method to scale up EV production and lack of reliable EV source, (iii) lack of standardisation of pre-analytical and pre-isolation sources of variability during EV production, and (iv) insufficient guidelines for the regulation of EVs as therapeutics.
1.5.1 Standardising the method to isolate, purify and characterise EVs
There is currently no standardised protocol to isolate and purify large quantities of EVs for clinical use. Research laboratories have utilized different approaches to isolate, purify, quantify, and functionally characterise EVs (Sáenz-Cuesta et al., 2015). Since different sub/populations of EVs are generated depending on the isolation method used, it is vital to standardize protocols for EV isolation and characterisation (Phan et al., 2021). Furthermore, it must be noted that the final product of current isolation methods do not produce pure EVs but rather an EV-enriched formulation containing other components, including high-density lipoprotein (HDL) and low-density lipoprotein (LDL) (Webber and Clayton, 2013). Moreover, EVs derived from blood samples are likely to be contaminated with chylomicrons, which overlap the size distribution and density of EVs making them indistinguishable by current isolation and characterisation methods (Karimi et al., 2018).
1.5.2 Scalability of EVs and the choice of EV source
Most of the traditional methods to isolate EVs involve multiple steps and procedures, which are labour-intensive. The recent incorporation of tangential flow filtration (TFF) and chromatography-based methods have advanced large-scale production of EVs with current Good Manufacturing Practice (cGMP) (Watson et al., 2018). However, a fundamental understanding of how the substrates used for cell attachment and expansion (e.g., microcarriers) influence cell behaviour and EV composition are necessary to establish reliable protocols and consistency for EV production (de Almeida Fuzeta et al., 2020).
For EV-based therapeutics to progress into clinical applications, selection of an appropriate EV source is required. MSC are an attractive source due to their low immunogenicity and ability to regenerate tissue (Dörnen and Dittmar, 2021). Treatment of MSCs with N-methyldopamine and norepinephrine, is a potential strategy to increase the production of MSC-derived EVs and enhance EV yield (Wang et al., 2020). Nevertheless, the impacts of these strategies on EV composition, efficacy and biological functions associated with their therapeutic use are currently unknown.
1.5.3 Standardisation of the pre-analytical source of variability for EVs
Due to the complexity in EV isolation and the heterogeneity of EV samples, it is necessary to reduce pre-isolation and pre-analytical variables through consistent sample collection, storage conditions, and handling procedures.
Regarding the isolation of EVs from cell culture, serum-free medium or EV-free medium are recommended to avoid contaminants from growth supplements such as fetal bovine serum (FBS) (Lehrich et al., 2021). For the isolation or detection of EVs in blood, it is necessary to use a needle gauge that reduces shear forces to prevent platelet activation and secretion of platelet and erythrocyte-derived EVs (Coumans et al., 2017). It is also imperative to dispose the first few millilitres of blood collection, where the cell debris can contaminate the EV isolate (Coumans et al., 2017). The status of patients (fed/fasting) and blood collection time (morning/night) can also influence EV concentration and profile (Danielson et al., 2016). However, further research is required to define the optimal parameters before any recommendations are made (Danielson et al., 2016).
Most studies suggested using plasma for EV isolation from blood, as serum consists of platelet-derived EVs which can co-contaminate plasma-derived EVs (Witwer et al., 2013). Moreover, the results can be influenced by the selection of anticoagulant (heparin, citrate, EDTA) (Yokota et al., 1999). Precautions must be taken at every step of EV production, from initial centrifugation to reduce the processing time and prevent sample degradation due to temperature and enzymatic activities (Gandham et al., 2020).
The storage of EV preparations is challenging, since EVs may be unstable in the carrier media and their biological properties could be compromised by long-term storage (Sivanantham and Jin, 2022). Few studies demonstrated that −80°C is best to preserve EV contents however, repeated freeze-thawing can result in EV aggregation and lysis, causing overestimation of EV size, loss of cargo and biological functions (Jeyaram and Jay, 2017; Maroto et al., 2017; Welch et al., 2017; Théry et al., 2018). There is an urgent need to investigate how various reagents, including storage vials, involved in EV preparation and affected the biological functions of EVs and how to optimise EV stability (Théry et al., 2018).
1.5.4 Regulatory guidelines for EVs as therapeutics
Currently, there are no regulatory guidelines specific for EV-based therapeutics (Phan et al., 2022). However, the manufacturing and safety requirements for cell- and tissue-based therapeutics could serve as examples for the development of EV-based products (Soekmadji et al., 2020). It is anticipated that the development of EV-based therapeutics is highly dependent on the choice and characterisation of the origin of EVs (Soekmadji et al., 2020). Hence, the current considerations of safety and toxicity applied for cell-based therapies could also be employed for EV-based therapeutics (Lener et al., 2015).
EV-based products are regulated broadly as biological medicinal products; the active substance of which is produced or isolated from a biological source (Silva et al., 2021). The subcategorization of EV-based products is likely to be defined by their active components and complexity (Lener et al., 2015; Silva et al., 2021). In 2018 and 2022, the committee for advanced therapy (CAT) suggested that EVs comprising recombinant RNAs should be considered gene therapy products because the effects of the EV directly correlated to these RNAs (Silva et al., 2021). EVs comprising recombinant proteins or peptides would belong to a different subcategory. Therefore, the knowledge of active substance and mode of action is required for regulatory classification of EV-based products and should be a central focus during product development (Gimona et al., 2021). The technology readiness levels (TRLs) scale, developed by NASA and employed by the US Department of Defence to enable clinical translation while mitigating risks of therapeutics, could be utilised to inform guidelines for the development of EV-based products (MARVEL, 2020), https://cordis.europa.eu/project/id/951768.
1.6 Potential application of EVs in amyloidosis
EVs have shown promise in both diagnostic and therapeutic applications for numerous complex diseases including cancer and autoimmune diseases. A recent study has suggested that EVs may directly contribute to disease pathology by promoting the deposition and aggregation of amyloid protein in amyloidosis (Yamaguchi et al., 2022). Moreover, it has been suggested that membrane proteins presented on the surface of EVs are responsible for directing amyloid proteins to specific organs where amyloids can initiate damage (Liu and Wang, 2023). Given the potential role for EVs in amyloid disease pathogenesis, it is possible that EVs could also have diagnostic and therapeutic applications in amyloidosis.
1.6.1 Amyloidosis
Amyloidosis is caused by deposition of misfolded and aggregated amyloid proteins in vital organ systems including the heart, kidney and liver (Merlini, 2017). Amyloid proteins are formed from soluble proteins, which undergo misfolding and consequently, assemble into insoluble fibrils (Almeida and Brito, 2020). These fibrils are non-branching and typically 8–12 nm in diameter (Juneja and Pati, 2020). The deposition of amyloid may be localised, with the deposits occurring in a single organ/tissue or systemic where numerous organs and tissues in the body are affected (Juneja and Pati, 2020).
Amyloidosis can present with a broad range of nonspecific and unpredictably progressive symptoms depending on where the amyloid fibrils deposit (Chee et al., 2010; Lu and Richards, 2019). Evaluation of two independent cohorts of patients with light chain amyloidosis reveal a wide range of affected organs in a majority of patients (% of patients indicated), including heart (70%), kidney (60%), liver (20%), gastrointestinal (15%), peripheral (15%) or autonomic nervous system (10%), and soft tissue (10%) (Wechalekar et al., 2013; Muchtar et al., 2019). There is an urgent need to develop an effective tool that can predict the targeted organ in early stage and prevent progressing pathology and organ dysfunction.
Amyloidosis can be formed from either an abnormal elevation in the concentration of proteins with an acquired or hereditary mutation, or precursor proteins with an intrinsic susceptibility to misfolding or proteolytic remodelling into amyloidogenic fragments (Chiti and Dobson, 2017). Amyloid diseases are classified based on the protein that forms amyloids. Currently, amyloids can be formed from 36 proteins in humans (Sipe et al., 2016).
The most popular types of amyloidosis are immunoglobulin light chain amyloidosis, transthyretin amyloidosis and reactive amyloidosis, the latter occurring secondary to a chronic inflammatory disorder such as infections or rheumatoid arthritis (Picken, 2020). With regards to naming conventions, the amyloid is termed A, followed by an abbreviation of the precursor protein, e.g., AL (amyloid derived from immunoglobulin light chain), ATTR (amyloid derived from transthyretin).
There is an unmet need for an early and accurate diagnosis and typing of amyloidosis. Many different types of amyloid diseases have a similar clinical presentation but require different treatments. For example, most AL treatments are based on suppressing the plasma cells responsible for producing toxic light chains with chemotherapy (bortezomib, cyclophosphamide) and immunosuppression (dexamethansone) (Bianchi et al., 2021) or more recently, anti-plasma cell monoclonal antibody (daratumumab) (Kastritis et al., 2021). In contrast, ATTR is treated with agents that stabilise circulating transthyretin tetramers (tafamidis, diflunisal) to prevent the formation of toxic monomers (Nuvolone et al., 2022) and recently, TTR gene silencing (patisiran, inotersen) to reduce total circulating transthyretin (Schmidt et al., 2022). To ensure patients receive the appropriate treatment, a comprehensive approach with biomarkers, multimodal imaging and invasive tissue biopsy is often required to screen, diagnose and differentiate amyloidosis (Jung et al., 2022).
While a monoclonal gammopathy of unknown significance (MGUS) is a potential biomarker for AL, MGUS occurs in 39% of people with ATTR (Phull et al., 2018), 3% of people over 50 years and is a known precursor for other serious indications like multiple myeloma (Kyle et al., 2018). Moreover, in some cases of AL, routine laboratory tests are insufficient to detect the light chains because the light chain burden is very low, and the titres are similar to healthy donors (Goldis et al., 2023). Without a clear biomarker to distinguish those with ATTR and MGUS (where the paraprotein is not contributing to pathology) from AL (where the paraprotein is causing pathology), patients are subjected to more invasive diagnostic procedures. Late and inaccurate diagnosis of amyloidosis resulted in a low rate of survival after diagnosis confirmation with 6 months median survival for late-stage AL amyloidosis (Sabbour et al., 2021).
1.6.2 The role of EVs in diagnosis of amyloidosis
Given that EVs are capable of transmitting cellular material specific to dysfunctional cells, tissues and organs, EVs are promising biomarkers for the diagnosis, typing and prognostication of amyloid diseases (Figure 4). The evaluation of EVs as diagnostic biomarkers in amyloidosis is a relatively recent research endeavour with the most extensive investigation in Alzheimer’s disease (AD). Amyloid-β (Aβ) and hyperphosphorylated tau play a role in the pathogenesis of AD and amyloid PET imaging is part of the diagnostic process (Rajmohan and Reddy, 2017). Previous studies demonstrated that EVs facilitated the accumulation of AD-associated toxic cargo. For example, neuronal-derived EVs (nEVs) were detected in the blood and carried Aβ, phosphorylated tau and synapse-related proteins, which could be used to diagnose AD (Li et al., 2022). Additionally, nEVs expressed in individuals in the symptomatic stages of AD contained higher levels of amyloid-β with 42 residues long (Aβ42) (Fiandaca et al., 2015; Goetzl et al., 2016; Winston et al., 2018; Jia et al., 2019; Zhao et al., 2020), tau phosphorylated at threonine 181 (p-tau-181) (Fiandaca et al., 2015; Goetzl et al., 2016; Winston et al., 2018; Jia et al., 2019), total tau (t-tau) (Fiandaca et al., 2015; Jia et al., 2019; Nam et al., 2020), and tau phosphorylated at serine 396 (p-S396-tau) (Fiandaca et al., 2015; Goetzl et al., 2016; Winston et al., 2018) compared to healthy controls.
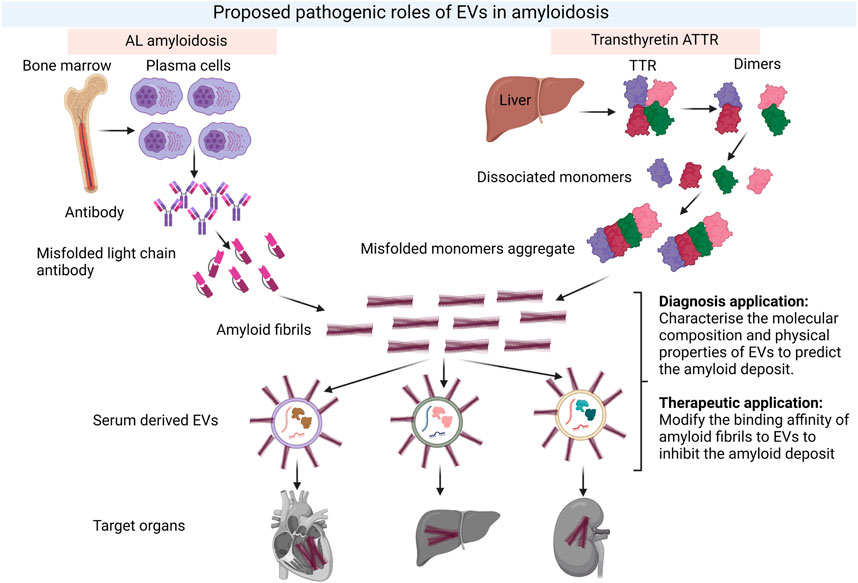
FIGURE 4. Proposed pathological role of EVs in amyloidosis and their potential in both diagnosis and therapeutics for amyloidosis. The formation of amyloid fibrils in two common types of amyloidosis, AL and ATTR and the potential roles of EVs in both diagnosis and therapeutic intervention. EVs could serve as early predictors of amyloid deposition in different tissues. Meanwhile, the composition of EVs could be modified to reduce binding affinity of amyloids and deposition. Created with BioRender.com.
nEVs have also shown potential as early biomarkers of AD. Fiandaca et al. demonstrated increased levels, relative to healthy participants, of p-tau-181, p-S396-tau, and Aβ42 in plasma-isolated nEVs as early as 10 years prior to the diagnosis of AD-related cognitive damage (Fiandaca et al., 2015). Another study performed longitudinal analysis on 887 plasma samples (over 3.5 ± 2.3 years before converting to AD) from 350 participants, including 128 participants developed AD by the final follow-up and 222 matched controls who continued cognitively normal (Kapogiannis et al., 2019). Individuals with future AD had higher levels of p-tau-181 and p-tau-231 in nEVs than matched controls (who remained cognitively normal over a same length of follow-up).
Similar to nEVs associating with AD, urinary derived EVs may have applications in renal amyloidosis. Urinary derived EVs (uEVs) have been shown to contain immunoglobulin light chain (LC) oligomers–a transition stage from monomers to amyloid fibrils (Šarić et al., 2014). Importantly, LC oligomers in uEVs were specifically detected in active patients with AL but not patients with multiple myeloma or MGUS (Ramirez-Alvarado et al., 2017). Ramirez-Alvarado and colleges also showed that LC oligomers could be found in uEVs derived from patient’s urine with unsolved renal progression despite hematologic complete response, which was defined as a normal serum free light chain ratio and undetectable monoclonal protein by serum and urine immunofixation (Ramirez-Alvarado et al., 2017). These data demonstrated the increased sensitivity of uEVs to detect amyloid LCs over conventional methods and the potential of uEVs as an improved measure of treatment response in AL. Based on these promising proof-of-concept studies, multiple strategies are being developed to overcome the technical barriers (sample standardization, inadequate starting material, etc.) to facilitate the utilization of uEVs as a biomarker for AL disease activity (Cooper et al., 2022).
1.6.3 Proposed pathological function of EVs in amyloidosis
EVs have been proposed to promote the aggregation and deposition of amyloid proteins. A recent paper showed that aggregation of an inherited transthyretin variant V30M (TTR V30M), which causes familial ATTR, is enhanced on EV membranes (Yamaguchi et al., 2022). Moreover, EVs promoted the deposition of TTR V30M aggregation in specific tissues and organs. It was suggested that the unique membrane proteins presented on the surface of EVs were responsible for directing the deposition of TTR amyloids to specific tissues. However, a detailed analysis of TTR aggregation and EV uptake by recipient cells in affected organs are needed to fully elucidate the pathogenic role of EVs in familial ATTR and whether this pathway applies to non-familial (wild-type) ATTR.
Another recent study demonstrated that EVs derived from vascular smooth muscle cells (VSMCs) senescence are key mediators of medin accumulation (Whitehead et al., 2023). Medin is a 50-amino acid peptide that contributes to aortic medial amyloid (AMA)–the most widespread localized human amyloid. The release of EVs was promoted in the senescence of VSMCs via upregulation of sphingomyelin phosphodiesterase 3 (SMPD3). Senescence of VSMCs also enhanced the accumulation of medin in the extracellular matrix (ECM) by promoting medin aggregation on the surface of EVs and the binding of EVs to heparan sulfate proteoglycan 2 (HSPG2) in the ECM. Further study is needed to determine whether the interaction between EVs and HSPG2 impacts the role of HSPG2 in controlling ECM organization and stabilization via its interactions with various ECM components (Guilak et al., 2021). It remains to be determined whether the pathogenic mechanisms uncovered for EVs in familial ATTR and AMA are generalisable to other types of amyloidosis.
2 Conclusion and future outlook
Amyloidosis is a complex and heterogenous disease, which requires an early and accurate diagnosis as well as an effective treatment that not only removes the deposit of fibrils in organs but also promotes regeneration of the damaged tissues or organs. In this context, EVs are promising agents for both diagnosis and treatment of amyloidosis. While much of the focus on therapeutic applications for EVs has been directed toward drug delivery, emerging research demonstrating a role for EVs in disease process creates the exciting potential for interventions based on modifying EVs or their interactions with amyloid proteins. Understanding the composition of EVs involved in disease pathology such as promoting fibril formation or delivering amyloids to specific organs should be a research priority (Figure 4). Future research on EVs should focus on exploring the mechanism of EV uptake and their specific function as well as establishing regulatory guidelines for EV-based products. Overcoming the technical challenges of EV production and characterisation will enable their potential as biomarkers and therapeutic agents to be realised in amyloidosis and other complex diseases.
Author contributions
TP: Conceptualization, Writing–original draft, Writing–review and editing. JR: Conceptualization, Supervision, Writing–review and editing.
Funding
The author(s) declare financial support was received for the research, authorship, and/or publication of this article. This work was supported by The Peter Tosi Fellowship in Amyloidosis Research and a National Health and Medical Research Council, Australia (NHMRC) Ideas grant 1183619.
Conflict of interest
The authors declare that the research was conducted in the absence of any commercial or financial relationships that could be construed as a potential conflict of interest.
Publisher’s note
All claims expressed in this article are solely those of the authors and do not necessarily represent those of their affiliated organizations, or those of the publisher, the editors and the reviewers. Any product that may be evaluated in this article, or claim that may be made by its manufacturer, is not guaranteed or endorsed by the publisher.
References
Alevizos, I., Alexander, S., Turner, R. J., and Illei, G. G. (2011). MicroRNA expression profiles as biomarkers of minor salivary gland inflammation and dysfunction in Sjögren's syndrome. Arthritis Rheum. 63 (2), 535–544. doi:10.1002/art.30131
Almeida, Z. L., and Brito, R. M. M. (2020). Structure and aggregation mechanisms in amyloids. Molecules 25 (5), 1195. doi:10.3390/molecules25051195
Alvarez-Erviti, L., Seow, Y., Yin, H., Betts, C., Lakhal, S., and Wood, M. J. A. (2011). Delivery of siRNA to the mouse brain by systemic injection of targeted exosomes. Nat. Biotechnol. 29 (4), 341–345. doi:10.1038/nbt.1807
Aqrawi, L. A., Galtung, H. K., Vestad, B., Øvstebø, R., Thiede, B., Rusthen, S., et al. (2017). Identification of potential saliva and tear biomarkers in primary Sjögren’s syndrome, utilising the extraction of extracellular vesicles and proteomics analysis. Arthritis Res. Ther. 19 (1), 14–15. doi:10.1186/s13075-017-1228-x
Bartoloni, E., Alunno, A., Bistoni, O., Caterbi, S., Luccioli, F., Santoboni, G., et al. (2015). Characterization of circulating endothelial microparticles and endothelial progenitor cells in primary Sjögren’s syndrome: new markers of chronic endothelial damage? Rheumatology 54 (3), 536–544. doi:10.1093/rheumatology/keu320
Bazzan, E., Tinè, M., Casara, A., Biondini, D., Semenzato, U., Cocconcelli, E., et al. (2021). Critical review of the evolution of extracellular vesicles’ knowledge: from 1946 to today. Int. J. Mol. Sci. 22 (12), 6417. doi:10.3390/ijms22126417
Bianchi, G., Zhang, Y., and Comenzo, R. L. (2021). AL amyloidosis: current chemotherapy and immune therapy treatment strategies. JACC CardioOncol 3 (4), 467–487. doi:10.1016/j.jaccao.2021.09.003
Borst, P. (2012). Cancer drug pan-resistance: pumps, cancer stem cells, quiescence, epithelial to mesenchymal transition, blocked cell death pathways, persisters or what? Open Biol. 2 (5), 120066. doi:10.1098/rsob.120066
Boukouris, S., and Mathivanan, S. (2015). Exosomes in bodily fluids are a highly stable resource of disease biomarkers. Proteomics Clin. Appl. 9 (3-4), 358–367. doi:10.1002/prca.201400114
Buzas, E. I. (2023). The roles of extracellular vesicles in the immune system. Nat. Rev. Immunol. 23 (4), 236–250. doi:10.1038/s41577-022-00763-8
Caruso, S., and Poon, I. K. H. (2018). Apoptotic cell-derived extracellular vesicles: more than just debris. Front. Immunol. 9, 1486. doi:10.3389/fimmu.2018.01486
Castro, C., and Gourley, M. (2010). Diagnostic testing and interpretation of tests for autoimmunity. J. Allergy Clin. Immunol. 125 (2), S238–S247. doi:10.1016/j.jaci.2009.09.041
Cecchettini, A., Finamore, F., Puxeddu, I., Ferro, F., and Baldini, C. (2019). Salivary extracellular vesicles versus whole saliva: new perspectives for the identification of proteomic biomarkers in Sjögren’s syndrome. Clin. Exp. Rheumatol. 37 (118), 240–248.
Chee, C. E., Lacy, M. Q., Dogan, A., Zeldenrust, S. R., and Gertz, M. A. (2010). Pitfalls in the diagnosis of primary amyloidosis. Clin. Lymphoma Myeloma Leuk. 10 (3), 177–180. doi:10.3816/clml.2010.n.027
Chen, G., Huang, A. C., Zhang, W., Zhang, G., Wu, M., Xu, W., et al. (2018). Exosomal PD-L1 contributes to immunosuppression and is associated with anti-PD-1 response. Nature 560 (7718), 382–386. doi:10.1038/s41586-018-0392-8
Chiti, F., and Dobson, C. M. (2017). Protein misfolding, amyloid formation, and human disease: a summary of progress over the last decade. Annu. Rev. Biochem. 86 (1), 27–68. doi:10.1146/annurev-biochem-061516-045115
Cooper, S. A., Dick, C. J., Misra, P., Leung, N., Schinstock, C. A., and Ramirez-Alvarado, M. (2022). Pathologic light chain amyloidosis oligomer detection in urinary extracellular vesicles as a diagnostic tool for response and progression of disease. Front. Oncol. 12, 978198. doi:10.3389/fonc.2022.978198
Coumans, F. A. W., Brisson, A. R., Buzas, E. I., Dignat-George, F., Drees, E. E., El-Andaloussi, S., et al. (2017). Methodological guidelines to study extracellular vesicles. Circ. Res. 120 (10), 1632–1648. doi:10.1161/circresaha.117.309417
Danielson, K. M., Estanislau, J., Tigges, J., Toxavidis, V., Camacho, V., Felton, E. J., et al. (2016). Diurnal variations of circulating extracellular vesicles measured by nano flow cytometry. PLoS One 11 (1), e0144678. doi:10.1371/journal.pone.0144678
de Almeida Fuzeta, M., Bernardes, N., Oliveira, F. D., Costa, A. C., Fernandes-Platzgummer, A., Farinha, J. P., et al. (2020). Scalable production of human mesenchymal stromal cell-derived extracellular vesicles under serum-/xeno-free conditions in a microcarrier-based bioreactor culture system. Front. Cell Dev. Biol. 8, 553444. doi:10.3389/fcell.2020.553444
de Jong, B., Barros, E. R., Hoenderop, J. G. J., and Rigalli, J. P. (2020). Recent advances in extracellular vesicles as drug delivery systems and their potential in precision medicine. Pharmaceutics 12 (11), 1006. doi:10.3390/pharmaceutics12111006
Del Conde, I., Shrimpton, C. N., Thiagarajan, P., and Lopez, J. A. (2005). Tissue-factor-bearing microvesicles arise from lipid rafts and fuse with activated platelets to initiate coagulation. Blood 106 (5), 1604–1611. doi:10.1182/blood-2004-03-1095
De Rubis, G., Rajeev Krishnan, S., and Bebawy, M. (2019). Liquid biopsies in cancer diagnosis, monitoring, and prognosis. Trends Pharmacol. Sci. 40 (3), 172–186. doi:10.1016/j.tips.2019.01.006
Desrochers, L. M., Antonyak, M. A., and Cerione, R. A. (2016). Extracellular vesicles: satellites of information transfer in cancer and stem cell biology. Dev. Cell 37 (4), 301–309. doi:10.1016/j.devcel.2016.04.019
Dörnen, J., and Dittmar, T. (2021). The role of MSCs and cell fusion in tissue regeneration. Int. J. Mol. Sci. 22 (20), 10980. doi:10.3390/ijms222010980
Doyle, L. M., and Wang, M. Z. (2019). Overview of extracellular vesicles, their origin, composition, purpose, and methods for exosome isolation and analysis. Cells 8 (7), 727. doi:10.3390/cells8070727
Escrevente, C., Keller, S., Altevogt, P., and Costa, J. (2011). Interaction and uptake of exosomes by ovarian cancer cells. BMC Cancer 11 (1), 108. doi:10.1186/1471-2407-11-108
Familtseva, A., Jeremic, N., and Tyagi, S. C. (2019). Exosomes: cell-created drug delivery systems. Mol. Cell Biochem. 459 (1-2), 1–6. doi:10.1007/s11010-019-03545-4
Feng, Y., Huang, W., Meng, W., Jegga, A. G., Wang, Y., Cai, W., et al. (2014b). Heat shock improves Sca-1+ stem cell survival and directs ischemic cardiomyocytes toward a prosurvival phenotype via exosomal transfer: a critical role for HSF1/miR-34a/HSP70 pathway. Stem Cells 32 (2), 462–472. doi:10.1002/stem.1571
Feng, Y., Huang, W., Wani, M., Yu, X., and Ashraf, M. (2014a). Ischemic preconditioning potentiates the protective effect of stem cells through secretion of exosomes by targeting Mecp2 via miR-22. PLoS One 9 (2), e88685. doi:10.1371/journal.pone.0088685
Fiandaca, M. S., Kapogiannis, D., Mapstone, M., Boxer, A., Eitan, E., Schwartz, J. B., et al. (2015). Identification of preclinical Alzheimer's disease by a profile of pathogenic proteins in neurally derived blood exosomes: a case-control study. Alzheimers Dement. 11 (6), 600–607. doi:10.1016/j.jalz.2014.06.008
Fitzgerald, W., Freeman, M. L., Lederman, M. M., Vasilieva, E., Romero, R., and Margolis, L. (2018). A system of cytokines encapsulated in ExtraCellular vesicles. Sci. Rep. 8 (1), 8973. doi:10.1038/s41598-018-27190-x
Gandham, S., Su, X., Wood, J., Nocera, A. L., Alli, S. C., Milane, L., et al. (2020). Technologies and standardization in research on extracellular vesicles. Trends Biotechnol. 38 (10), 1066–1098. doi:10.1016/j.tibtech.2020.05.012
Gatti, S., Bruno, S., Deregibus, M. C., Sordi, A., Cantaluppi, V., Tetta, C., et al. (2011). Microvesicles derived from human adult mesenchymal stem cells protect against ischaemia-reperfusion-induced acute and chronic kidney injury. Nephrol. Dial. Transpl. 26 (5), 1474–1483. doi:10.1093/ndt/gfr015
Gimona, M., Brizzi, M. F., Choo, A. B. H., Dominici, M., Davidson, S. M., Grillari, J., et al. (2021). Critical considerations for the development of potency tests for therapeutic applications of mesenchymal stromal cell-derived small extracellular vesicles. Cytotherapy 23 (5), 373–380. doi:10.1016/j.jcyt.2021.01.001
Gobin, J., Muradia, G., Mehic, J., Westwood, C., Couvrette, L., Stalker, A., et al. (2021). Hollow-fiber bioreactor production of extracellular vesicles from human bone marrow mesenchymal stromal cells yields nanovesicles that mirrors the immuno-modulatory antigenic signature of the producer cell. Stem Cell Res. Ther. 12 (1), 127. doi:10.1186/s13287-021-02190-3
Goetzl, E. J., Mustapic, M., Kapogiannis, D., Eitan, E., Lobach, I. V., Goetzl, L., et al. (2016). Cargo proteins of plasma astrocyte-derived exosomes in Alzheimer's disease. Faseb J. 30 (11), 3853–3859. doi:10.1096/fj.201600756r
Goldis, R., Kaplan, B., Kukuy, O. L., Arad, M., Magen, H., Shavit-Stein, E., et al. (2023). Diagnostic challenges and solutions in systemic amyloidosis. Int. J. Mol. Sci. 24 (5), 4655. doi:10.3390/ijms24054655
Guilak, F., Hayes, A. J., and Melrose, J. (2021). Perlecan in pericellular mechanosensory cell-matrix communication, extracellular matrix stabilisation and mechanoregulation of load-bearing connective tissues. Int. J. Mol. Sci. 22 (5), 2716. doi:10.3390/ijms22052716
Gurunathan, S., Kang, M. H., and Kim, J. H. (2021). A comprehensive review on factors influences biogenesis, functions, therapeutic and clinical implications of exosomes. Int. J. Nanomedicine 16, 1281–1312. doi:10.2147/ijn.s291956
Han, Y., Jones, T. W., Dutta, S., Zhu, Y., Wang, X., Narayanan, S. P., et al. (2021). Overview and update on methods for cargo loading into extracellular vesicles. Process. (Basel) 9 (2), 356. doi:10.3390/pr9020356
Harding, C., Heuser, J., and Stahl, P. (1983). Receptor-mediated endocytosis of transferrin and recycling of the transferrin receptor in rat reticulocytes. J. Cell Biol. 97 (2), 329–339. doi:10.1083/jcb.97.2.329
Hoshino, A., Costa-Silva, B., Shen, T. L., Rodrigues, G., Hashimoto, A., Tesic Mark, M., et al. (2015). Tumour exosome integrins determine organotropic metastasis. Nature 527 (7578), 329–335. doi:10.1038/nature15756
Jeyaram, A., and Jay, S. M. (2017). Preservation and storage stability of extracellular vesicles for therapeutic applications. Aaps J. 20 (1), 1. doi:10.1208/s12248-017-0160-y
Jia, L., Qiu, Q., Zhang, H., Chu, L., Du, Y., Zhang, J., et al. (2019). Concordance between the assessment of Aβ42, T-tau, and P-T181-tau in peripheral blood neuronal-derived exosomes and cerebrospinal fluid. Alzheimers Dement. 15 (8), 1071–1080. doi:10.1016/j.jalz.2019.05.002
Juneja, R., and Pati, H. P. (2020). Approach to the diagnosis of amyloidosis. Indian J. Hematol. Blood Transfus. 36 (2), 246–253. doi:10.1007/s12288-019-01208-4
Jung, M. H., Chang, S., Han, E. J., and Youn, J. C. (2022). Multimodal imaging and biomarkers in cardiac amyloidosis. Diagn. (Basel) 12 (3), 627. doi:10.3390/diagnostics12030627
Kalluri, R., and LeBleu, V. S. (2020). The biology, function, and biomedical applications of exosomes. Science 367 (6478), eaau6977. doi:10.1126/science.aau6977
Kanada, M., Bachmann, M. H., Hardy, J. W., Frimannson, D. O., Bronsart, L., Wang, A., et al. (2015). Differential fates of biomolecules delivered to target cells via extracellular vesicles. Proc. Natl. Acad. Sci. U. S. A. 112 (12), E1433–E1442. doi:10.1073/pnas.1418401112
Kanchanapally, R., Deshmukh, S. K., Chavva, S. R., Tyagi, N., Srivastava, S. K., Patel, G. K., et al. (2019). Drug-loaded exosomal preparations from different cell types exhibit distinctive loading capability, yield, and antitumor efficacies: a comparative analysis. Int. J. Nanomedicine 14, 531–541. doi:10.2147/ijn.s191313
Kapogiannis, D., Mustapic, M., Shardell, M. D., Berkowitz, S. T., Diehl, T. C., Spangler, R. D., et al. (2019). Association of extracellular vesicle biomarkers with alzheimer disease in the Baltimore longitudinal study of aging. JAMA Neurol. 76 (11), 1340–1351. doi:10.1001/jamaneurol.2019.2462
Karimi, N., Cvjetkovic, A., Jang, S. C., Crescitelli, R., Hosseinpour Feizi, M. A., Nieuwland, R., et al. (2018). Detailed analysis of the plasma extracellular vesicle proteome after separation from lipoproteins. Cell Mol. Life Sci. 75 (15), 2873–2886. doi:10.1007/s00018-018-2773-4
Kastritis, E., Palladini, G., Minnema, M. C., Wechalekar, A. D., Jaccard, A., Lee, H. C., et al. (2021). Daratumumab-based treatment for immunoglobulin light-chain amyloidosis. N. Engl. J. Med. 385 (1), 46–58. doi:10.1056/nejmoa2028631
Kleeff, J., Ishiwata, T., Kumbasar, A., Friess, H., Büchler, M. W., Lander, A. D., et al. (1998). The cell-surface heparan sulfate proteoglycan glypican-1 regulates growth factor action in pancreatic carcinoma cells and is overexpressed in human pancreatic cancer. J. Clin. Invest. 102 (9), 1662–1673. doi:10.1172/jci4105
Kooijmans, S. A. A., Stremersch, S., Braeckmans, K., de Smedt, S. C., Hendrix, A., Wood, M. J., et al. (2013). Electroporation-induced siRNA precipitation obscures the efficiency of siRNA loading into extracellular vesicles. J. Control Release 172 (1), 229–238. doi:10.1016/j.jconrel.2013.08.014
Kowal, J., Arras, G., Colombo, M., Jouve, M., Morath, J. P., Primdal-Bengtson, B., et al. (2016). Proteomic comparison defines novel markers to characterize heterogeneous populations of extracellular vesicle subtypes. Proc. Natl. Acad. Sci. U. S. A. 113 (8), E968–E977. doi:10.1073/pnas.1521230113
Kyle, R. A., Larson, D. R., Therneau, T. M., Dispenzieri, A., Kumar, S., Cerhan, J. R., et al. (2018). Long-term follow-up of monoclonal gammopathy of undetermined significance. N. Engl. J. Med. 378 (3), 241–249. doi:10.1056/nejmoa1709974
Lai, R. C., Yeo, R. W. Y., Tan, K. H., and Lim, S. K. (2013). Exosomes for drug delivery - a novel application for the mesenchymal stem cell. Biotechnol. Adv. 31 (5), 543–551. doi:10.1016/j.biotechadv.2012.08.008
Lamichhane, T. N., Jeyaram, A., Patel, D. B., Parajuli, B., Livingston, N. K., Arumugasaamy, N., et al. (2016). Oncogene knockdown via active loading of small RNAs into extracellular vesicles by sonication. Cell Mol. Bioeng. 9 (3), 315–324. doi:10.1007/s12195-016-0457-4
Lee, H., Zhang, D., Wu, J., Otterbein, L. E., and Jin, Y. (2017). Lung epithelial cell–derived microvesicles regulate macrophage migration via MicroRNA-17/221–induced integrin β1 recycling. J. Immunol. 199 (4), 1453–1464. doi:10.4049/jimmunol.1700165
Lehrich, B. M., Liang, Y., and Fiandaca, M. S. (2021). Foetal bovine serum influence on in vitro extracellular vesicle analyses. J. Extracell. Vesicles 10 (3), e12061. doi:10.1002/jev2.12061
Lener, T., Gimona, M., Aigner, L., Börger, V., Buzas, E., Camussi, G., et al. (2015). Applying extracellular vesicles based therapeutics in clinical trials - an ISEV position paper. J. Extracell. Vesicles 4, 30087. doi:10.3402/jev.v4.30087
Li, B., Antonyak, M. A., Zhang, J., and Cerione, R. A. (2012). RhoA triggers a specific signaling pathway that generates transforming microvesicles in cancer cells. Oncogene 31 (45), 4740–4749. doi:10.1038/onc.2011.636
Li, S. P., Lin, Z. x., Jiang, X. y., and Yu, X. y. (2018). Exosomal cargo-loading and synthetic exosome-mimics as potential therapeutic tools. Acta Pharmacol. Sin. 39 (4), 542–551. doi:10.1038/aps.2017.178
Li, T. R., Yang, Q., Hu, X., and Han, Y. (2022). Biomarkers and tools for predicting alzheimer's disease in the preclinical stage. Curr. Neuropharmacol. 20 (4), 713–737. doi:10.2174/1570159x19666210524153901
Li, Y., Bax, C., Patel, J., Vazquez, T., Ravishankar, A., Bashir, M. M., et al. (2021). Plasma-derived DNA containing-extracellular vesicles induce STING-mediated proinflammatory responses in dermatomyositis. Theranostics 11 (15), 7144–7158. doi:10.7150/thno.59152
Liu, Y.-J., and Wang, C. (2023). A review of the regulatory mechanisms of extracellular vesicles-mediated intercellular communication. Cell Commun. Signal. 21 (1), 77. doi:10.1186/s12964-023-01103-6
Lu, R., and Richards, T. A. (2019). AL amyloidosis: unfolding a complex disease. J. Adv. Pract. Oncol. 10 (8), 813–825. doi:10.6004/jadpro.2019.10.8.4
Maroto, R., Zhao, Y., Jamaluddin, M., Popov, V. L., Wang, H., Kalubowilage, M., et al. (2017). Effects of storage temperature on airway exosome integrity for diagnostic and functional analyses. J. Extracell. Vesicles 6 (1), 1359478. doi:10.1080/20013078.2017.1359478
MARVEL (2020). Evolving reversible iMmunocapture by membrane sensing peptides: towARds scalable extracellular VEsicLes isolation. European Commission. H2020-EU.1.2.2. Available at: https://cordis.europa.eu/project/id/951768.
Mathieu, M., Martin-Jaular, L., Lavieu, G., and Théry, C. (2019). Specificities of secretion and uptake of exosomes and other extracellular vesicles for cell-to-cell communication. Nat. Cell Biol. 21 (1), 9–17. doi:10.1038/s41556-018-0250-9
Matsumoto, A., Takahashi, Y., Nishikawa, M., Sano, K., Morishita, M., Charoenviriyakul, C., et al. (2017). Role of phosphatidylserine-derived negative surface charges in the recognition and uptake of intravenously injected B16bl6-derived exosomes by macrophages. J. Pharm. Sci. 106 (1), 168–175. doi:10.1016/j.xphs.2016.07.022
Melo, S. A., Luecke, L. B., Kahlert, C., Fernandez, A. F., Gammon, S. T., Kaye, J., et al. (2015). Glypican-1 identifies cancer exosomes and detects early pancreatic cancer. Nature 523 (7559), 177–182. doi:10.1038/nature14581
Mendt, M., Kamerkar, S., Sugimoto, H., McAndrews, K. M., Wu, C. C., Gagea, M., et al. (2018). Generation and testing of clinical-grade exosomes for pancreatic cancer. JCI Insight 3 (8), e99263. doi:10.1172/jci.insight.99263
Merlini, G. (2017). AL amyloidosis: from molecular mechanisms to targeted therapies. Hematol. Am. Soc. Hematol. Educ. Program 2017 (1), 1–12. doi:10.1182/asheducation-2017.1.1
Michael, A., Bajracharya, S., Yuen, P., Zhou, H., Star, R., Illei, G., et al. (2010). Exosomes from human saliva as a source of microRNA biomarkers. Oral Dis. 16 (1), 34–38. doi:10.1111/j.1601-0825.2009.01604.x
Monfared, H., Jahangard, Y., Nikkhah, M., Mirnajafi-Zadeh, J., and Mowla, S. J. (2019). Potential therapeutic effects of exosomes packed with a miR-21-sponge construct in a rat model of glioblastoma. Front. Oncol. 9, 782. doi:10.3389/fonc.2019.00782
Monopoli, M. P., Åberg, C., Salvati, A., and Dawson, K. A. (2012). Biomolecular coronas provide the biological identity of nanosized materials. Nat. Nanotechnol. 7 (12), 779–786. doi:10.1038/nnano.2012.207
Muchtar, E., Gertz, M. A., Kyle, R. A., Lacy, M. Q., Dingli, D., Leung, N., et al. (2019). A modern primer on light chain amyloidosis in 592 patients with mass spectrometry-verified typing. Mayo Clin. Proc. 94 (3), 472–483. doi:10.1016/j.mayocp.2018.08.006
Mulcahy, L. A., Pink, R. C., and Carter, D. R. (2014). Routes and mechanisms of extracellular vesicle uptake. J. Extracell. Vesicles 3. doi:10.3402/jev.v3.24641
Murphy, D. E., de Jong, O. G., Brouwer, M., Wood, M. J., Lavieu, G., Schiffelers, R. M., et al. (2019a). Extracellular vesicle-based therapeutics: natural versus engineered targeting and trafficking. Exp. Mol. Med. 51 (3), 32–12. doi:10.1038/s12276-019-0223-5
Murphy, D. E., de Jong, O. G., Brouwer, M., Wood, M. J., Lavieu, G., Schiffelers, R. M., et al. (2019b). Extracellular vesicle-based therapeutics: natural versus engineered targeting and trafficking. Exp. Mol. Med. 51 (3), 32–12. doi:10.1038/s12276-019-0223-5
Nagelkerke, A., Ojansivu, M., van der Koog, L., Whittaker, T. E., Cunnane, E. M., Silva, A. M., et al. (2021). Extracellular vesicles for tissue repair and regeneration: evidence, challenges and opportunities. Adv. Drug Deliv. Rev. 175, 113775. doi:10.1016/j.addr.2021.04.013
Nam, E., Lee, Y. B., Moon, C., and Chang, K. A. (2020). Serum tau proteins as potential biomarkers for the assessment of alzheimer's disease progression. Int. J. Mol. Sci. 21 (14), 5007. doi:10.3390/ijms21145007
Nuvolone, M., Girelli, M., and Merlini, G. (2022). Oral therapy for the treatment of transthyretin-related amyloid cardiomyopathy. Int. J. Mol. Sci. 23 (24), 16145. doi:10.3390/ijms232416145
Palviainen, M., Saraswat, M., Varga, Z., Kitka, D., Neuvonen, M., Puhka, M., et al. (2020). Extracellular vesicles from human plasma and serum are carriers of extravesicular cargo—implications for biomarker discovery. PLOS ONE 15 (8), e0236439. doi:10.1371/journal.pone.0236439
Phan, T. H., Divakarla, S. K., Yeo, J. H., Lei, Q., Tharkar, P., Pansani, T. N., et al. (2021). New multiscale characterization methodology for effective determination of isolation-structure-function relationship of extracellular vesicles. Front. Bioeng. Biotechnol. 9, 669537. doi:10.3389/fbioe.2021.669537
Phan, T. H., Kim, S. Y., Rudge, C., and Chrzanowski, W. (2022). Made by cells for cells – extracellular vesicles as next-generation mainstream medicines. J. Cell Sci. 135 (1), jcs259166. doi:10.1242/jcs.259166
Phull, P., Sanchorawala, V., Connors, L. H., Doros, G., Ruberg, F. L., Berk, J. L., et al. (2018). Monoclonal gammopathy of undetermined significance in systemic transthyretin amyloidosis (ATTR). Amyloid 25 (1), 62–67. doi:10.1080/13506129.2018.1436048
Picken, M. M. (2020). The pathology of amyloidosis in classification: a review. Acta Haematol. 143 (4), 322–334. doi:10.1159/000506696
Pieters, B. C. H., Cappariello, A., van den Bosch, M. H. J., van Lent, P. L. E. M., Teti, A., and van de Loo, F. A. J. (2019). Macrophage-derived extracellular vesicles as carriers of alarmins and their potential involvement in bone homeostasis. Front. Immunol. 10, 1901. doi:10.3389/fimmu.2019.01901
Pugholm, L. H., Revenfeld, A. L. S., Søndergaard, E. K. L., and Jørgensen, M. M. (2015). Antibody-based assays for phenotyping of extracellular vesicles. BioMed Res. Int. 2015, 1–15. doi:10.1155/2015/524817
Rajmohan, R., and Reddy, P. H. (2017). Amyloid-beta and phosphorylated tau accumulations cause abnormalities at synapses of alzheimer's disease neurons. J. Alzheimers Dis. 57 (4), 975–999. doi:10.3233/jad-160612
Ramirez-Alvarado, M., Barnidge, D. R., Murray, D. L., Dispenzieri, A., Marin-Argany, M., Dick, C. J., et al. (2017). Assessment of renal response with urinary exosomes in patients with AL amyloidosis: a proof of concept. Am. J. Hematol. 92 (6), 536–541. doi:10.1002/ajh.24717
Raposo, G., Nijman, H. W., Stoorvogel, W., Liejendekker, R., Harding, C. V., Melief, C. J., et al. (1996). B lymphocytes secrete antigen-presenting vesicles. J. Exp. Med. 183 (3), 1161–1172. doi:10.1084/jem.183.3.1161
Ratajczak, J., Miekus, K., Kucia, M., Zhang, J., Reca, R., Dvorak, P., et al. (2006). Embryonic stem cell-derived microvesicles reprogram hematopoietic progenitors: evidence for horizontal transfer of mRNA and protein delivery. Leukemia 20 (5), 847–856. doi:10.1038/sj.leu.2404132
Sabbour, H., Hasan, K. Y., Al Badarin, F., Alibazoglu, H., Rivard, A. L., Romany, I., et al. (2021). From clinical clues to final diagnosis: the return of detective work to clinical medicine in cardiac amyloidosis. Front. Cardiovasc. Med. 8, 644508. doi:10.3389/fcvm.2021.644508
Sáenz-Cuesta, M., Arbelaiz, A., Oregi, A., Irizar, H., Osorio-Querejeta, I., Muñoz-Culla, M., et al. (2015). Methods for extracellular vesicles isolation in a hospital setting. Front. Immunol. 6, 50. doi:10.3389/fimmu.2015.00050
Šarić, A., Chebaro, Y. C., Knowles, T. P. J., and Frenkel, D. (2014). Crucial role of nonspecific interactions in amyloid nucleation. Proc. Natl. Acad. Sci. 111 (50), 17869–17874. doi:10.1073/pnas.1410159111
Savill, J., Hogg, N., Ren, Y., and Haslett, C. (1992). Thrombospondin cooperates with CD36 and the vitronectin receptor in macrophage recognition of neutrophils undergoing apoptosis. J. Clin. investigation 90 (4), 1513–1522. doi:10.1172/jci116019
Schierer, S., Ostalecki, C., Zinser, E., Lamprecht, R., Plosnita, B., Stich, L., et al. (2018). Extracellular vesicles from mature dendritic cells (DC) differentiate monocytes into immature DC. Life Sci. Alliance 1 (6), e201800093. doi:10.26508/lsa.201800093
Schmidt, H. H., Wixner, J., Planté-Bordeneuve, V., Muñoz-Beamud, F., Lladó, L., Gillmore, J. D., et al. (2022). Patisiran treatment in patients with hereditary transthyretin-mediated amyloidosis with polyneuropathy after liver transplantation. Am. J. Transpl. 22 (6), 1646–1657. doi:10.1111/ajt.17009
Sercombe, L., Veerati, T., Moheimani, F., Wu, S. Y., Sood, A. K., and Hua, S. (2015). Advances and challenges of liposome assisted drug delivery. Front. Pharmacol. 6, 286. doi:10.3389/fphar.2015.00286
Shtam, T. A., Kovalev, R. A., Varfolomeeva, E. Y., Makarov, E. M., Kil, Y. V., and Filatov, M. V. (2013). Exosomes are natural carriers of exogenous siRNA to human cells in vitro. Cell Commun. Signal. 11 (1), 88. doi:10.1186/1478-811x-11-88
Silva, A. K. A., Morille, M., Piffoux, M., Arumugam, S., Mauduit, P., Larghero, J., et al. (2021). Development of extracellular vesicle-based medicinal products: a position paper of the group “Extracellular Vesicle translatiOn to clinicaL perspectiVEs – EVOLVE France”. Adv. Drug Deliv. Rev. 179, 114001. doi:10.1016/j.addr.2021.114001
Sipe, J. D., Benson, M. D., Buxbaum, J. N., Ikeda, S. i., Merlini, G., Saraiva, M. J. M., et al. (2016). Amyloid fibril proteins and amyloidosis: chemical identification and clinical classification International Society of Amyloidosis 2016 Nomenclature Guidelines. Amyloid 23 (4), 209–213. doi:10.1080/13506129.2016.1257986
Sivanantham, A., and Jin, Y. (2022). Impact of storage conditions on EV integrity/surface markers and cargos. Life (Basel) 12 (5), 697. doi:10.3390/life12050697
Soekmadji, C., Li, B., Huang, Y., Wang, H., An, T., Liu, C., et al. (2020). The future of Extracellular Vesicles as Theranostics - an ISEV meeting report. J. Extracell. Vesicles 9 (1), 1809766. doi:10.1080/20013078.2020.1809766
Somiya, M., Yoshioka, Y., and Ochiya, T. (2018). Biocompatibility of highly purified bovine milk-derived extracellular vesicles. J. Extracell. vesicles 7 (1), 1440132. doi:10.1080/20013078.2018.1440132
Tai, Y. L., Chen, K., Hsieh, J., and Shen, T. (2018). Exosomes in cancer development and clinical applications. Cancer Sci. 109 (8), 2364–2374. doi:10.1111/cas.13697
Tang, D., Cao, F., Yan, C., Fang, K., Ma, J., Gao, L., et al. (2022). Extracellular vesicle/macrophage Axis: potential targets for inflammatory disease intervention. Front. Immunol. 13, 705472. doi:10.3389/fimmu.2022.705472
Théry, C., Witwer, K. W., Aikawa, E., Alcaraz, M. J., Anderson, J. D., Andriantsitohaina, R., et al. (2018). Minimal information for studies of extracellular vesicles 2018 (MISEV2018): a position statement of the International Society for Extracellular Vesicles and update of the MISEV2014 guidelines. J. Extracell. Vesicles 7 (1), 1535750. doi:10.1080/20013078.2018.1535750
Tian, J., Casella, G., Zhang, Y., Rostami, A., and Li, X. (2020). Potential roles of extracellular vesicles in the pathophysiology, diagnosis, and treatment of autoimmune diseases. Int. J. Biol. Sci. 16 (4), 620–632. doi:10.7150/ijbs.39629
Tian, T., Zhang, H. X., Fan, S., Zhu, Y. L., Qi, C., et al. (2018). Surface functionalized exosomes as targeted drug delivery vehicles for cerebral ischemia therapy. Biomaterials 150, 137–149. doi:10.1016/j.biomaterials.2017.10.012
Torralba, D., Baixauli, F., Villarroya-Beltri, C., Fernández-Delgado, I., Latorre-Pellicer, A., Acín-Pérez, R., et al. (2018). Priming of dendritic cells by DNA-containing extracellular vesicles from activated T cells through antigen-driven contacts. Nat. Commun. 9 (1), 2658. doi:10.1038/s41467-018-05077-9
Trajkovic, K., Hsu, C., Chiantia, S., Rajendran, L., Wenzel, D., Wieland, F., et al. (2008). Ceramide triggers budding of exosome vesicles into multivesicular endosomes. Science 319 (5867), 1244–1247. doi:10.1126/science.1153124
Vandendriessche, C., Bruggeman, A., Van Cauwenberghe, C., and Vandenbroucke, R. E. (2020). Extracellular vesicles in Alzheimer’s and Parkinson’s disease: small entities with large consequences. Cells 9 (11), 2485. doi:10.3390/cells9112485
van der Koog, L., Gandek, T. B., and Nagelkerke, A. (2022). Liposomes and extracellular vesicles as drug delivery systems: a comparison of composition, pharmacokinetics, and functionalization. Adv. Healthc. Mater. 11 (5), 2100639. doi:10.1002/adhm.202100639
van Haasteren, J., Hyde, S. C., and Gill, D. R. (2018). Lessons learned from lung and liver in-vivo gene therapy: implications for the future. Expert Opin. Biol. Ther. 18 (9), 959–972. doi:10.1080/14712598.2018.1506761
Venturini, A., Passalacqua, M., Pelassa, S., Pastorino, F., Tedesco, M., Cortese, K., et al. (2019). Exosomes from astrocyte processes: signaling to neurons. Front. Pharmacol. 10, 1452. doi:10.3389/fphar.2019.01452
Walker, S., Busatto, S., Pham, A., Tian, M., Suh, A., Carson, K., et al. (2019). Extracellular vesicle-based drug delivery systems for cancer treatment. Theranostics 9 (26), 8001–8017. doi:10.7150/thno.37097
Wang, J., Bonacquisti, E. E., Brown, A. D., and Nguyen, J. (2020). Boosting the biogenesis and secretion of mesenchymal stem cell-derived exosomes. Cells 9 (3), 660. doi:10.3390/cells9030660
Watson, D. C., Yung, B. C., Bergamaschi, C., Chowdhury, B., Bear, J., Stellas, D., et al. (2018). Scalable, cGMP-compatible purification of extracellular vesicles carrying bioactive human heterodimeric IL-15/lactadherin complexes. J. Extracell. Vesicles 7 (1), 1442088. doi:10.1080/20013078.2018.1442088
Webber, J., and Clayton, A. (2013). How pure are your vesicles? J. Extracell. Vesicles 2 (1), 19861. doi:10.3402/jev.v2i0.19861
Wechalekar, A. D., Schonland, S. O., Kastritis, E., Gillmore, J. D., Dimopoulos, M. A., Lane, T., et al. (2013). A European collaborative study of treatment outcomes in 346 patients with cardiac stage III AL amyloidosis. Blood 121 (17), 3420–3427. doi:10.1182/blood-2012-12-473066
Wei, G., Jie, Y., Haibo, L., Chaoneng, W., Dong, H., Jianbing, Z., et al. (2017). Dendritic cells derived exosomes migration to spleen and induction of inflammation are regulated by CCR7. Sci. Rep. 7, 42996. doi:10.1038/srep42996
Welch, J. L., Madison, M. N., Margolick, J. B., Galvin, S., Gupta, P., Martínez-Maza, O., et al. (2017). Effect of prolonged freezing of semen on exosome recovery and biologic activity. Sci. Rep. 7 (1), 45034. doi:10.1038/srep45034
Whitehead, M., Yusoff, S., Ahmad, S., Schmidt, L., Mayr, M., Madine, J., et al. (2023). Vascular smooth muscle cell senescence accelerates medin aggregation via small extracellular vesicle secretion and extracellular matrix reorganization. Aging Cell 22 (2), e13746. doi:10.1111/acel.13746
Winston, C. N., Goetzl, E. J., Baker, L. D., Vitiello, M. V., and Rissman, R. A. (2018). Growth hormone-releasing hormone modulation of neuronal exosome biomarkers in mild cognitive impairment. J. Alzheimers Dis. 66 (3), 971–981. doi:10.3233/jad-180302
Witwer, K. W., Buzás, E. I., Bemis, L. T., Bora, A., Lässer, C., Lötvall, J., et al. (2013). Standardization of sample collection, isolation and analysis methods in extracellular vesicle research. J. Extracell. Vesicles 2. doi:10.3402/jev.v2i0.20360
Wu, Z., He, D., and Li, H. (2021). Bioglass enhances the production of exosomes and improves their capability of promoting vascularization. Bioact. Mater. 6 (3), 823–835. doi:10.1016/j.bioactmat.2020.09.011
Yamaguchi, H., Kawahara, H., Kodera, N., Kumaki, A., Tada, Y., Tang, Z., et al. (2022). Extracellular vesicles contribute to the metabolism of transthyretin amyloid in hereditary transthyretin amyloidosis. Front. Mol. Biosci. 9, 839917. doi:10.3389/fmolb.2022.839917
Yokota, M., Tatsumi, N., Nathalang, O., Yamada, T., and Tsuda, I. (1999). Effects of heparin on polymerase chain reaction for blood white cells. J. Clin. Lab. Anal. 13 (3), 133–140. doi:10.1002/(sici)1098-2825(1999)13:3<133::aid-jcla8>3.0.co;2-0
Zhang, H., Freitas, D., Kim, H. S., Fabijanic, K., Li, Z., Chen, H., et al. (2018). Identification of distinct nanoparticles and subsets of extracellular vesicles by asymmetric flow field-flow fractionation. Nat. Cell Biol. 20 (3), 332–343. doi:10.1038/s41556-018-0040-4
Zhang, X., Wang, X., Zhu, H., Kranias, E. G., Tang, Y., Peng, T., et al. (2012). Hsp20 functions as a novel cardiokine in promoting angiogenesis via activation of VEGFR2. PloS one 7 (3), e32765. doi:10.1371/journal.pone.0032765
Keywords: extracellular vesicles, autoimmune, amyloidosis, diagnosis, therapeutics, drug delivery vehicles
Citation: Phan TH and Reed JH (2024) Extracellular vesicles as next-generation therapeutics and biomarkers in amyloidosis: a new frontier. Front. Front. Biomater. Sci. 2:1343658. doi: 10.3389/fbiom.2023.1343658
Received: 24 November 2023; Accepted: 29 December 2023;
Published: 12 January 2024.
Edited by:
Dietmar Werner Hutmacher, Queensland University of Technology, AustraliaReviewed by:
Manuel M. Mazo, University of Navarra, SpainCarlo Francesco Morasso, Scientific Clinical Institute Maugeri (ICS Maugeri), Italy
Olwyn Roxanne Mahon, University of Limerick, Ireland
Copyright © 2024 Phan and Reed. This is an open-access article distributed under the terms of the Creative Commons Attribution License (CC BY). The use, distribution or reproduction in other forums is permitted, provided the original author(s) and the copyright owner(s) are credited and that the original publication in this journal is cited, in accordance with accepted academic practice. No use, distribution or reproduction is permitted which does not comply with these terms.
*Correspondence: Thanh Huyen Phan, dGhhbmgucGhhbkBzeWRuZXkuZWR1LmF1