- 1Biomedical and Chemical Engineering, Syracuse University, Syracuse, NY, United States
- 2BioInspired Syracuse, Institute for Living and Material Systems, Syracuse, NY, United States
The overuse of antibiotics to treat bacterial infections along with bacteria’s propensity to form biofilm communities has resulted in an alarming rise in drug-resistant microbes. Current approaches to infection surveillance and biofilm clearance in wounds are severely limited, requiring new biomaterials-based strategies to address this problem. To that end, a range of antimicrobial smart materials have been developed that change their properties in response to bacteria-induced external stimuli, providing tools with an additional level of complexity for defending against microbes. Researchers have tried to tackle this issue using materials that respond to the unique pH, temperature, and enzymatic changes that are induced by bacteria in wounds. These environmental responses are coupled with mechanisms to kill surrounding bacteria and/or to signal infection. For example, bacteria-responsive biomaterial solubilization (transition from non-solubilized solid material to solubilized liquid solution), swelling (volumetric increase due to absorption of surrounding media), de-swelling, degradation, or shape change can be coupled with drug release and/or activation or biofilm disruption, inhibition, or destruction. These materials provide a foundation for future work and improvements related to enhanced infection surveillance, increased specificity of infection response, and effective clearance of biofilms from wound surfaces.
1 Introduction
By 2050, it is projected that drug-resistant infections will cause 10,000,000 deaths each year, more than all cancers combined (O’Neill, 2016). A major contributor to drug-resistance and wound infection complications is biofilms (Bowler et al., 2020). Chronic wounds, including diabetic ulcers, affect >5 million patients per year in the US. The cost of healing one chronic wound is ∼$50,000, and diabetic ulcers are associated with ∼70% of foot amputations (Paquette and Falanga, 2002; Boulton et al., 2005). Of many factors that delay healing in chronic wounds, infection plays a significant role (Siddiqui and Bernstein, 2010; Ventola, 2015). To further complicate this clinical need, ∼50% of chronic wound patients with a limb threatening infection do not show systemic infection symptoms, delaying diagnosis and treatment (Lipsky et al., 2004). Burn wounds are also negatively impacted by infection. In the US, ∼10,000 people die each year from burn-related infections, and biofilms are associated with 60% of burn injury deaths (Smith et al., 1994; Norbury et al., 2016).
Despite the prevalence of wound infections, there is no expert consensus on the standard of care for assessment and treatment (Young, 2021). Infection assessment via wound swabbing or biopsies is susceptible to contamination with skin surface bacteria, may not effectively penetrate biofilms, and requires ∼48 h to obtain a diagnosis (Young, 2021). Due to the overuse and misuse of antibiotics that contribute to drug resistance (Kardas et al., 2005; English et al., 2010), systemic antibiotic intake is not standardly recommended for treating wound infections (Bowler et al., 2001). Treating infections typically involves painful debridement and local antimicrobials, such as iodine or silver, that could hinder healing (Goldenheim, 1993; Kramer, 1999; Khansa et al., 2019). Furthermore, bacteria within biofilms are less amenable to treatment (Miller et al., 2011). Thus, new approaches are needed to improve wound infection surveillance and biofilm clearance.
One of the many ways to combat the rise of antibiotic resistant ‘superbugs’ is the use of antimicrobial biomaterials (Fasiku et al., 2020). Current research on antimicrobial polymers provides a number of potential paths, including incorporation of antimicrobial agents into the bulk or as coatings (Jain et al., 2014). Within the field of polymer science, stimuli-responsive or ‘smart’ materials provide a platform for the inclusion of new functions into polymeric devices. Stimuli-responsive materials undergo large and abrupt changes in shape in response to environmental cues, including temperature, pH, or enzymes (Wei et al., 2017). These changes can be coupled with functions that aid in infection control, such as antimicrobial release or biofilm disruption and therefore could provide more precise tools for infection surveillance and treatment, Figures 1A–G. Smart biomaterials can be intrinsically antimicrobial based on their chemistry, they can have antimicrobial components incorporated into them, and/or they can be used to physically disrupt biofilms. There have been extensive efforts focused on smart materials that respond to externally-applied stimuli, including light (Yuan et al., 2022; Su et al., 2023), electric or magnetic fields (Zhu et al., 2019; Elbourne et al., 2020; Fragal et al., 2022), or ultrasound (LuTheryn et al., 2022; Fan et al., 2023), which show potential for improving infection control efforts. Here, we focus on smart biomaterials that independently respond to unique pH, temperature, and enzymatic environments induced by bacteria in wounds without the need for an external stimulus. Biomaterials that have been recently developed for infection control that undergo large and abrupt changes in the presence of bacteria are covered in this review and are summarized in Table 1.
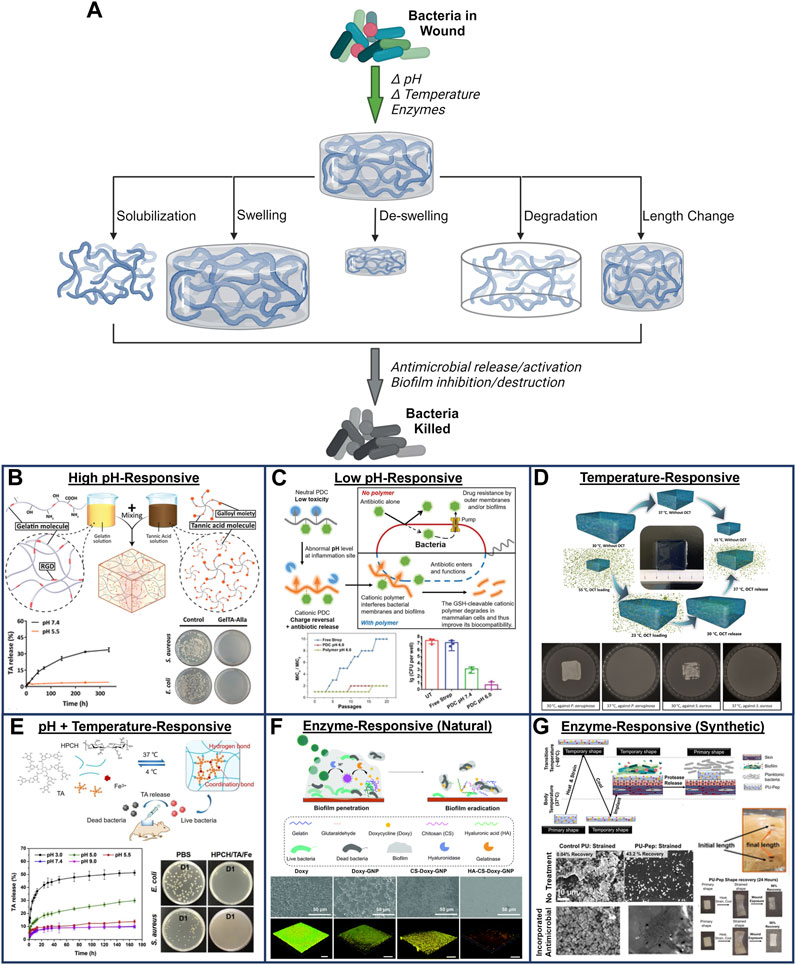
FIGURE 1. (A) Schematic representation of mechanisms for stimuli-responsive biomaterials to actively kill bacteria based on bacterial environmental cues. Created with Biorender.com. Representative bacterial environmentally-responsive systems. (B) Hydrogels that swell to release tannic acid at high pH to kill bacteria (from Ref. 36, © 2021 John Wiley & Sons, Inc.); (C) Cationic polymers that solubilize and release streptomycin at low pH to kill bacteria (from Ref. 40, © 2020 John Wiley & Sons, Inc.); (D) Lower critical solubility temperature hydrogels that de-swell at 37°C to release octenidine (from Ref. 49, © 2022 Royal Society of Chemistry); (E) Hydroxypropyl chitin hydrogels that crosslink at 37°C and release with tannic acid and ferric ions at low pH to kill bacteria (from Ref. 52, © 2020 Elsevier); (F) Gelatin nanoparticles coated with hyaluronic acid and chitosan. The chitosan swells at low pH to enable diffusion of bacterial hyaluronidase and gelatinase that degrades nanoparticles and triggers doxycycline release (from Ref. 57, © 2022 Royal Society of Chemistry); (G) Shape memory polyurethanes with poly (glutamic acid) in the backbone. Bacterial proteases degrade the poly (glutamic acid) to induce a shape change, which is a visible infection cue, prevents biofilm formation, and can be coupled with release of cinnamic acid to kill bacteria (from Ref. 62, © 2023 John Wiley & Sons, Inc.).
2 pH-responsive smart biomaterials
pH is a logarithmic measure of hydrogen ions used to indicate acidic (1–6.9), neutral 7), or basic (7.1–14) conditions. Human skin exhibits a slightly acidic pH of 4.2–5.6, which discourages bacteria overgrowth (Schmid-Wendtner and Korting, 2006). Interior tissues that are exposed during wounding are typically at pH 7.4, but wound pH can vary based on host immune response and the presence of bacteria. In general, mammalian cell healing is more efficient in acidic conditions, while bacteria prefer and promote alkaline environments (Weinrick et al., 2004; Shukla et al., 2007; Sharpe et al., 2009; Percival et al., 2014; Ono et al., 2015). Thus, infected wounds often have higher pH, while non-infected wounds have lower pH, providing an environmental cue for smart hydrogels.
2.1 Hydrogels based on polyanions and polycations
Hydrogels are water-soluble polymer networks that absorb large quantities of water. Hydrogels are typically biocompatible due to their high water content, enabling their extensive use in drug delivery and implantable biomaterials (Yang et al., 2018). Hydrogels are highly tunable and can be designed to change their solubility in water with changes in the environment, including pH. As solubility changes, hydrogel swelling is altered, which can be harnessed for environmentally-responsive drug delivery. pH-responsive hydrogels can be based on polyanions or polycations, which solubilize or swell to varying degrees based on the pKa of an ionic group in the polymer backbone. pH-responsive polyanions switch between a liquid polymer solution and a solid gel as the pH transitions from high to low. These solubility alterations occur as the polymer transitions between a charged, expanded state that is soluble at high pH and an uncharged, collapsed state that is insoluble at low pH. Polyanions can also be covalently crosslinked into insoluble 3D networks that swell (volumetric expansion due to absorption of surrounding media as chains expand) at high pH and de-swell (volumetric shrinking due to expulsion of media as chains collapse) at low pH. On the other hand, polycations move from a solution state at low pH to a solid gel state at high pH. Crosslinked polycations swell at low pH and de-swell and high pH. These properties can be employed in infection control to deliver antimicrobials upon swelling, de-swelling, or dissolution.
2.1.1 Polyanions
A recent example of pH-responsive hydrogels with controlled release of antimicrobials at basic conditions that mimic those of an infected wound is a keratin-based hydrogel that swells at high pH. Upon swelling, biocidal zinc oxide nanoplates are released, which resulted in a 6-log reduction in Staphylococcus aureus (Staphylococcus aureus) and E. coli (Escherichia coli) while maintaining high mammalian cytocompatibility (Villanueva et al., 2019). Ahmadian et al. developed a gelatin and tannic acid-based hydrogel system with low release of incorporated antimicrobials (tannic acid and allantoin) at low pH and higher release at physiological pH (7.4), Figure 1B (A et al., 2021). Combined release of both antimicrobials reduced E. coli and S. aureus growth.
In a synthetic approach, a methacrylic acid co-polymer with tunable pH response based on methacrylic acid content was employed to fabricate electrospun mesh scaffolds with pH sensitivity at low, neutral, and high pH (Miranda-Calderon et al., 2022). After loading the fibers with bactericidal agents, the authors show that drug release varies between the three chemistries based on pH, and antimicrobial properties can be tuned with both S. aureus and E. coli. This platform could allow for dressing selection based on real-time wound pH measurements.
2.1.2 Polycations
Although infection generally increases pH, bacteria in biofilms can survive in lower pH ranges than planktonic bacteria (Percival et al., 2014). Thus, infected wound pH may decrease over time as bacteria enter biofilms and the innate immune response takes over. To that end, the majority of pH-responsive antimicrobial wound dressings focus on polycations. Charged polycations are also inherently antimicrobial, which has been used extensively to complement released antimicrobial activity.
Antimicrobial peptides are often employed in polycation-based systems. Wang et al. recently reported an antimicrobial octapeptide that self-assembles into nanofibers at neutral pH and solubilizes in acidic conditions (Wang et al., 2019). The soluble peptide destabilizes bacteria membranes to aid in killing. Drug-loaded antimicrobial peptides promoted healing in a methicillin-resistant S. aureus (MRSA)-infected diabetic mouse wound model when applied 2 days after infection, indicating its potential efficacy as a chronic wound dressing. Another antimicrobial peptide was developed as an injectable wound dressing that forms a solid gel at physiological and high pH and solubilizes at pH 6 (Edirisinghe et al., 2023). By incorporating a non-natural AzAla tryptophan analog residue into the peptide sequence, antimicrobial properties were achieved against E. coli and S. aureus, which are activated upon solubilization at lower pH.
Using a synthetic approach, Ye et al. employed antimicrobial, pH responsive polycations that induce pores in bacterial membranes, Figure 1C (Ye et al., 2020). The polycation is neutral under physiological conditions but becomes positively charged to solubilize at low pH. Solubilization enabled controlled release of antibiotic streptomycin, which had enhanced transport through the resulting membrane pores, correlating with improved clearance of E. coli, P. aeruginosa (Pseudomonas aeruginosa), and MRSA (∼two to three log reductions vs streptomycin delivery on its own) in mouse thigh, lung, and peritoneum infections, respectively, when applied 1 h after bacterial inoculation.
2.2 Supramolecular hydrogels
2.2.1 Peptide-based supramolecular hydrogels
Supramolecular hydrogels form a sub-class of pH-responsive materials that reversibly solidify based on specific, non-covalent interactions between components. One example includes supramolecular assembly of peptides into 3D networks. Pal and Roy employed collagen-inspired peptide amphiphiles to form nanoparticles with encapsulated antimicrobial ferulic acid (Pal and Roy, 2022). The nanoparticles are stable at neutral pH but undergo a structural transformation into nanofibers at pH 8.5, triggering ferulic acid release. The released antimicrobial effectively killed E. coli and S. aureus, while the peptide nanofibers showed beneficial effects for mammalian cell healing in vitro assays. Another group synthesized three amphiphilic pentapeptides that form supramolecular hydrogels that are stable at neutral pH (Chen et al., 2022). These hydrogels solubilize in acidic pH to provide an antimicrobial effect by combined exposure to the inherently antimicrobial peptides and release of encapsulated oregano oil. The hydrogels enhanced S. aureus infected wound closure in a mouse model by reducing bacterial loads in the wounds.
2.2.2 Other supramolecular hydrogels
A supramolecular hydrogel based on carboxymethyl agarose complexed with Ag+ was developed that is de-swollen at neutral pH and swells at acidic pH (Huang et al., 2020). The hydrogels release more Ag + as they swell to significantly reduce S. aureus and E. coli CFU counts in vitro. In a S. aureus infected mouse wound model, the Ag + -containing hydrogels reduced bacteria load and enhanced closure rates. Another supramolecular hydrogel was developed based on sodium alginate/poly (N-vinyl caprolactam) with incorporated tannic acid, which served as both a gelation binder and an antimicrobial (Preman et al., 2020). The scaffolds exhibited increased tannic acid release at low pH and were effective against several strains of Gram-negative and Gram-positive bacteria in vitro while enhancing healing in an in vivo rat wound model.
3 Thermally-responsive smart biomaterials
Normal, non-infected dermal wound temperatures are reported within the range of 30.2°C–33.0°C (Gethin et al., 2021). Wound inflammation can increase temperatures by 1.5°C–2.2°C, while infected wound temperatures are 4°C–5°C higher (Chanmugam et al., 2017). After successful infection treatment, dermal wound temperatures decrease back to 1°C within normal levels. Based on these temperature differentials, many approaches to smart antimicrobial wound dressings rely on thermally-responsive materials.
3.1 Lower critcal solubility temperature polymers
Polymers with lower critical solubility temperatures (LCST) are in solution at low temperatures due to dominant water/polymer interactions (Bernstein et al., 1977). As temperature is increased, hydrophobic interactions dominate to drive de-solubilization processes to form a solid scaffold. This approach is commonly used for injectable biomaterials, where a polymer is in a liquid solution state at room temperature and then solidifies after injection and heating to body temperature (Gandhi et al., 2015). This mechanism is particularly useful for space-filling applications in irregularly-shaped wounds. LCST polymers can also be crosslinked to swell and de-swell at low and high temperatures, respectively, without loss of overall structure. These dissolution and swelling processes can be coupled with antimicrobial release to aid in infection control.
Pan et al. combined a commonly used thermo-responsive polymer, poly (N-isopropylacrylamide) (PNIPAAm) with an antifouling polymer, poly (2-methacryloyloxyethyl phosphorylcholine) (PMPC) to obtain a dual-function hydrogel, Figure 1D (Pan et al., 2022). They took advantage of higher swelling at low temperature to efficiently load the hydrogels with an antimicrobial, octenidine, via diffusion. Upon heating to 37°C, the resulting de-swelling induced a 25-fold increase in octenidine release as compared with testing at normal skin temperatures of 30°C. This antimicrobial release resulted in a 3-log reduction in E. coli, P. aeruginosa, S. aureus, and Staphylococcus epidermidis.
3.2 Thermally-actuated shape memory polymers
Beyond thermo-responsive hydrogels, researchers have employed thermally-actuated shape memory polymers (SMPs) to disrupt biofilms. SMPs are type of ‘smart’ material that can be programmed and stored in a temporary shape and then return to their original, permanent state after application of an external stimulus, such as heat. Gu et al. grew S. aureus biofilms on strained polyurethane SMPs and then heated them to 40°C to induce shape recovery (i.e., shrinking) (Gu et al., 2016). The shape recovery process reduced S. aureus biomass from 5.4 to 0.07 μm3/μm2, and later studies showed that shape recovery with subsequent application of tobramycin reduced dispersed biofilm cell counts by 2,479X in comparison to static controls (Gu et al., 2016; Lee et al., 2018). These effects were attributed to the SMP shape change, which physically disrupted the biofilm structure to increase bacterial activity.
3.3 Combined pH- and thermo-responsive systems
Many researchers combine pH- and thermo-responsive materials for smart wound dressing development. In one approach, a composite hydrogel was developed based on hydroxypropyl chitin and tannic acid, Figure 1E (Ma et al., 2020). Upon heating to 37°C, the hydrogel transitions from a liquid solution into a solid, physically crosslinked gel, which aids in wound delivery by enabling space-filling of the wound volume by the liquid prior to gelation. The tannic acid is specifically released in acidic environments to provide antimicrobial protection in conjunction with incorporated ferric ion particles. The composite hydrogels were effective in vitro against E. coli and S. aureus, and they showed some benefits in healing in a mouse infected wound model. Using synthetic polymers, Haidari et al. combined PNIPAAm with poly (acrylic acid) (a polyanion) into a hydrogel loaded with silver nanoparticles (AgNPs) (Haidari et al., 2022). The materials exhibit a LCST of ∼36°C, which the authors posit could enhance swelling at elevated infected dermal wound temperatures. The hydrogels had limited Ag+ ion release at acidic pH and increased release at pH > 7.4. The AgNP release at higher pH resulted in correspondingly high antimicrobial efficacy against S. aureus both in vitro and in vivo.
4 Enzyme-responsive smart biomaterials
Bacteria release a number of proteases and other enzymes during infection to inactivate immune factors, break down tissues, and slow healing (Supuran et al., 2001; Lindsay et al.). Researchers have harnessed these processes to develop enzymatically-responsive biomaterials that respond to and kill bacteria.
4.1 Enzymatically-responsive natural polymers
An enzyme-responsive nanosystem was fabricated using mesoporous ruthenium nanoparticles as nanocarriers for a prodrug. The nanocarriers were encapsulated within hyaluronic acid (HA) (Liu et al., 2019). Hyaluronidase secreted by bacteria degrades the HA to trigger release of the prodrug, which then activates an antibiotic on the outer surface of the hydrogels. The nanosystem exhibited bactericidal and antibiofilm activity against drug-resistant S. aureus and P. aeruginosa. In a different approach with higher specificity towards biofilms, Wang and Shukla designed an antibiotic-loaded gelatin nanoparticle that is coated with HA and chitosan, Figure 1F. (Wang and Shukla) The chitosan becomes charged in the acidic biofilm environment, promoting swelling. As the hydrogel swells, hyaluronidase and gelatinase that are release by bacteria can more easily diffuse into the system. These enzymes degrade the HA and gelatin to provide biofilm-responsive antibiotic release. Nanoparticles were able to penetrate Vibrio vulnificus biofilms, eliminate the biofilm matrix, and kill bacteria with higher efficacy as compared with free drug treatment. Another system harnessed bacteria’s capability to permeabilize eukaryotic cell phospholipid vesicle bilayer members using secreted α-hemolysin and phospholipase. In brief, antimicrobials and a self-quenching dye were encapsulated within cell-mimicking phospholipid vesicles, which were incorporated into a gelatin scaffold (Zhou et al., 2018). Upon exposure to S. aureus or P. aeruginosa, the vesicle membranes are damaged by bacterial proteases, which provides mechanisms to both kill bacteria via released antimicrobials and to enable visual surveillance of infection via dye color change.
4.2 Enzymatically-responsive synthetic polymers
In efforts to provide synthetic scaffolds that selectively release antimicrobials in the presence of bacteria, poly (caprolactone) and poly (ethylene succinate) nanofibers were fabricated with a biocide (Abdali et al., 2019). The synthetic polymers are degraded by lipase and acids secreted by bacteria, which induces release of the incorporated biocide and subsequent reduction of S. aureus and E. coli.
These described approaches show promise, but most of the targeted enzymes are also produced by mammalian cells, limiting specificity of the systems towards bacteria. Towards increasing specificity, a synthetic hydrogel system was designed with groups that are cleaved by β-lactamase that is produced by bacteria (Yu et al., 2020; Alkekhia et al., 2022). The hydrogels were biostable in physiological conditions and in common enzymes (i.e., collagenase and lipase) but degrade in the presence of β-lactamase-producing bacteria. This degradation process was coupled with the release of fluorescent nanoparticles in vitro and ex vivo models and can be used in the future to design β-lactamase-responsive drug delivery and/or diagnostic tools.
In a different approach, our group synthesized bacterial protease-responsive SMPs based on a segmented polyurethane with incorporated poly (glutamic acid), which is degraded by bacterial proteases to trigger shape recovery, Figure 1G (Ramezani and Monroe, 2023). Strained SMPs underwent visible shape recovery within ≤24 h in response to multiple bacteria strains (S. aureus, S. epidermidis, and E. coli), while remaining stable during exposure to mammalian fibroblasts. Shape recovery of strained samples prevented biofilm formation on the sample surfaces, and resulting attached planktonic bacteria had enhanced vulnerability to applied antimicrobials. Samples with physically incorporated antimicrobials simultaneously prevented biofilm formation and killed isolated bacteria. The shape change could serve as a combined infection surveillance tool and biofilm inhibitor to enable earlier and more effective infection treatment.
5 Discussion
Bacteria-responsive ‘smart’ systems are ever-expanding and highly promising in the wake of the antibiotic resistance crisis. pH and thermo-responsive approaches are more fully developed and show efficacy in vivo infection models, with the added benefit of simplified designs that do not rely on biological components. However, pH and temperature fluctuations during infection are generally small and unreliable, limiting specificity of these methods. Newer protease-responsive biomaterials could enhance specificity towards bacteria vs mammalian cells but are less developed. These materials can benefit from tools and information gained during the rise of mammalian enzyme-responsive biomaterials, such as the rapid synthesis and screening of peptide derivatives (Lin et al., 2021). In general, many antimicrobial approaches use antibiotics or antimicrobials that have limited efficacy against biofilms. Thus, future efforts should continue to consider the unique biofilm environment.
While bacterial infections are a significant clinical concern, fungi (yeast) infect billions of people every year with ∼150 million severe and life threatening cases, resulting in ∼1.7 million deaths annually (Bongomin et al., 2017; Houšť et al., 2020). With some exceptions within the biomaterials field, research on fungal infections is limited, and very few classes of antifungal drugs are available (Vera-González et al., 2020). New fungal species have emerged with resistance to currently available antifungals, and the Centers for Disease Control ranks drug-resistant Candida yeasts as a “serious antibiotic resistance threat” in the same threat level class as MRSA and multidrug-resistant P. aeruginosa (CDC, 2019). Based on these increasing threats to human health, it is essential that future efforts in smart biomaterials also turn their attention to fungal infections.
Some of the research highlighted here provides mechanisms for infection surveillance through color or shape change. However, infection assessment options are still limited. Future generations of bacteria-responsive biomaterials can provide valuable tools in complex wound dressings with simultaneous monitoring, surveillance, and treatment capabilities (Mostafalu et al., 2018; Ullah et al., 2023). These efforts will require multidisciplinary work between biomaterials scientists, electrical and computer engineers, biologists, and clinicians.
Author contributions
MM: Supervision, Writing–original draft, Writing–review and editing. DF: Conceptualization, Methodology, Writing–original draft.
Funding
The author(s) declare that no financial support was received for the research, authorship, and/or publication of this article.
Conflict of interest
The authors declare that the research was conducted in the absence of any commercial or financial relationships that could be construed as a potential conflict of interest.
Publisher’s note
All claims expressed in this article are solely those of the authors and do not necessarily represent those of their affiliated organizations, or those of the publisher, the editors and the reviewers. Any product that may be evaluated in this article, or claim that may be made by its manufacturer, is not guaranteed or endorsed by the publisher.
References
Abdali, Z., Logsetty, S., and Liu, S. (2019). Bacteria-responsive single and core–shell nanofibrous membranes based on polycaprolactone/poly(ethylene succinate) for on-demand release of biocides. ACS Omega 4, 4063–4070. doi:10.1021/acsomega.8b03137
Ahmadian, Z., Correia, A., Hasany, M., Figueiredo, P., Dobakhti, F., Eskandari, M. R., et al. (2021). A hydrogen-bonded extracellular matrix-mimicking bactericidal hydrogel with radical scavenging and hemostatic function for pH-responsive wound healing acceleration. Adv. Healthc. Mat. 10, 2001122. doi:10.1002/adhm.202001122
Alkekhia, D., LaRose, C., and Shukla, A. (2022). β-Lactamase-Responsive hydrogel drug delivery platform for bacteria-triggered cargo release. ACS Appl. Mat. Interfaces. 14, 27538–27550. doi:10.1021/acsami.2c02614
Bernstein, R. E., Cruz, C. A., Paul, D. R., and Barlow, J. W. (1977). LCST behavior in polymer blends. Macromolecules 10, 681–686. doi:10.1021/ma60057a037
Bongomin, F., Gago, S., Oladele, R. O., and Denning, D. W. (2017). Global and multi-national prevalence of fungal diseases—estimate precision. J. Fungi . 3, 57. doi:10.3390/jof3040057
Boulton, A. J. M., Vileikyte, L., Ragnarson-Tennvall, G., and Apelqvist, J. (2005). The global burden of diabetic foot disease. Lancet London, Engl. 366, 1719–1724. doi:10.1016/S0140-6736(05)67698-2
Bowler, P., Murphy, C., and Wolcott, R. (2020). Biofilm exacerbates antibiotic resistance: is this a current oversight in antimicrobial stewardship? Antimicrob. Resist. Infect. Control. 9, 162. doi:10.1186/s13756-020-00830-6
Bowler, P. G., Duerden, B. I., and Armstrong, D. G. (2001). Wound microbiology and associated approaches to wound management. Clin. Microbiol. Rev. 14, 244–269. doi:10.1128/CMR.14.2.244-269.2001
CDC (2019). Antibiotic resistance threats in the United States 2019. https://www.cdc.gov/drugresistance/pdf/threats-report/2019-ar-threats-report-508.pdf.
Chanmugam, A., Langemo, D., Thomason, K., Haan, J., Altenburger, E. A., Tippett, A., et al. (2017). Relative temperature maximum in wound infection and inflammation as compared with a control subject using long-wave infrared thermography. Adv. Skin. Wound Care 30, 406–414. doi:10.1097/01.asw.0000522161.13573.62
Chen, H., Cheng, J., Cai, X., Han, J., Chen, X., You, L., et al. (2022). pH-switchable antimicrobial supramolecular hydrogels for synergistically eliminating biofilm and promoting wound healing. ACS Appl. Mat. Interfaces. 14, 18120–18132. doi:10.1021/acsami.2c00580
Edirisinghe, D. I., D’Souza, A., Ramezani, M., Carroll, R. J., Chicón, Q., Muenzel, C. L., et al. (2023). Antibacterial and cytocompatible pH-responsive peptide hydrogel. Molecules 28, 4390. doi:10.3390/molecules28114390
Elbourne, A., Cheeseman, S., Atkin, P., Truong, N. P., Syed, N., Zavabeti, A., et al. (2020). Antibacterial liquid metals: biofilm treatment via magnetic activation. ACS Nano 14, 802–817. doi:10.1021/acsnano.9b07861
English, B. K., and Gaur, A. H. (2010). “The use and abuse of antibiotics and the development of antibiotic resistance,” in Hot top. Infect. Immun. Child. VI. Editors A. Finn, N. Curtis, and A. J. Pollard (New York, NY, USA: Springer New York), 73–82. doi:10.1007/978-1-4419-0981-7_6
Fan, J., Xuan, M., Zhao, P., Loznik, M., Chen, J., Kiessling, F., et al. (2023). Ultrasound responsive microcapsules for antibacterial nanodrug delivery. Nano Res. 16, 2738–2748. doi:10.1007/s12274-022-4919-9
Fasiku, V. O., Hassan, D., Owonubi, S. J., Mukwevho, E., and Lolu Olajide, J. (2020). “Antibiotic polymer for biomedical applications (chapter 3),” in Antibiot. Mater. Healthc., Editors V. Kokkarachedu, V. Kanikireddy, and H. Sadiku (Cambridge, Massachusetts, United States: Academic Press), 33–49. doi:10.1016/B978-0-12-820054-4.00003-3
Fragal, E. H., Fragal, V. H., Silva, E. P., Paulino, A. T., da Silva Filho, E. C., Mauricio, M. R., et al. (2022). Magnetic-responsive polysaccharide hydrogels as smart biomaterials: synthesis, properties, and biomedical applications. Carbohydr. Polym. 292, 119665. doi:10.1016/j.carbpol.2022.119665
Gandhi, A., Paul, A., Sen, S. O., and Sen, K. K. (2015). Studies on thermoresponsive polymers: phase behaviour, drug delivery and biomedical applications. Asian J. Pharm. Sci. 10, 99–107. doi:10.1016/j.ajps.2014.08.010
Gethin, G., Ivory, J. D., Sezgin, D., Muller, H., O’Connor, G., and Vellinga, A. (2021). What is the “normal” wound bed temperature? A scoping review and new hypothesis. Wound Repair Regen. 29, 843–847. doi:10.1111/wrr.12930
Goldenheim, P. D. (1993). An appraisal of povidone-iodine and wound healing. Postgrad. Med. J. 69 (Suppl. 3), S97–S105. http://europepmc.org/abstract/MED/8290466.
Gu, H., Lee, S. W., Buffington, S. L., Henderson, J. H., and Ren, D. (2016). On-demand removal of bacterial biofilms via shape memory activation. ACS Appl. Mat. Interfaces. 8, 21140–21144. doi:10.1021/acsami.6b06900
Haidari, H., Vasilev, K., Cowin, A. J., and Kopecki, Z. (2022). Bacteria-activated dual pH- and temperature-responsive hydrogel for targeted elimination of infection and improved wound healing. ACS Appl. Mat. Interfaces. 14, 51744–51762. doi:10.1021/acsami.2c15659
Houšť, J., Spížek, J., and Havlíček, V. (2020). Antifungal drugs. Metab 10, 106. doi:10.3390/metabo10030106
Huang, W.-C., Ying, R., Wang, W., Guo, Y., He, Y., Mo, X., et al. (2020). A macroporous hydrogel dressing with enhanced antibacterial and anti-inflammatory capabilities for accelerated wound healing. Adv. Funct. Mat. 30. doi:10.1002/adfm.202000644
Jain, A., Duvvuri, L. S., Farah, S., Beyth, N., Domb, A. J., and Khan, W. (2014). Antimicrobial polymers. Adv. Healthc. Mat. 3, 1969–1985. doi:10.1002/adhm.201400418
Kardas, P., Devine, S., Golembesky, A., and Roberts, C. (2005). A systematic review and meta-analysis of misuse of antibiotic therapies in the community. Int. J. Antimicrob. Agents. 26, 106–113. doi:10.1016/j.ijantimicag.2005.04.017
Khansa, I., Schoenbrunner, A. R., Kraft, C. T., and Janis, J. E. (2019). Silver in wound care-friend or foe? a comprehensive review. Plast. Reconstr. Surg. Glob. Open. 7, e2390. doi:10.1097/GOX.0000000000002390
Kramer, S. A. (1999). Effect of povidone-iodine on wound healing: a review. J. Vasc. Nurs. 17, 17–23. doi:10.1016/S1062-0303(99)90004-3
Lee, S. W., Gu, H., Kilberg, J. B., and Ren, D. (2018). Sensitizing bacterial cells to antibiotics by shape recovery triggered biofilm dispersion. Acta Biomater. 81, 93–102. doi:10.1016/j.actbio.2018.09.042
Lin, Y., Penna, M., Spicer, C. D., Higgins, S. G., Gelmi, A., Kim, N., et al. (2021). High-throughput peptide derivatization toward supramolecular diversification in microtiter plates. ACS Nano 15, 4034–4044. doi:10.1021/acsnano.0c05423
Lindsay, S., Oates, A., and Bourdillon, K. (2017). The detrimental impact of extracellular bacterial proteases on wound healing. Int. Wound J. 14, 1237–1247. doi:10.1111/iwj.12790
Lipsky, B. A., Berendt, A. R., Embil, J., and de Lalla, F. (2004). Diagnosing and treating diabetic foot infections. Diabetes. Metab. Res. Rev. 20, S56–S64. doi:10.1002/dmrr.441
Liu, Y., Lin, A., Liu, J., Chen, X., Zhu, X., Gong, Y., et al. (2019). Enzyme-responsive mesoporous ruthenium for combined chemo-photothermal therapy of drug-resistant bacteria. ACS Appl. Mat. Interfaces 11, 26590–26606. doi:10.1021/acsami.9b07866
LuTheryn, G., Hind, C., Campbell, C., Crowther, A., Wu, Q., Keller, S. B., et al. (2022). Bactericidal and anti-biofilm effects of uncharged and cationic ultrasound-responsive nitric oxide microbubbles on Pseudomonas aeruginosa biofilms. Front. Cell. Infect. Microbiol. 12, 956808. doi:10.3389/fcimb.2022.956808
Ma, M., Zhong, Y., and Jiang, X. (2020). Thermosensitive and pH-responsive tannin-containing hydroxypropyl chitin hydrogel with long-lasting antibacterial activity for wound healing. Carbohydr. Polym. 236, 116096. doi:10.1016/j.carbpol.2020.116096
Miller, C. N., Carville, K., Newall, N., Kapp, S., Lewin, G., Karimi, L., et al. (2011). Assessing bacterial burden in wounds: comparing clinical observation and wound swabs. Int. Wound J. 8, 45–55. doi:10.1111/j.1742-481X.2010.00747.x
Miranda-Calderon, L., Yus, C., Landa, G., Mendoza, G., Arruebo, M., and Irusta, S. (2022). Pharmacokinetic control on the release of antimicrobial drugs from pH-responsive electrospun wound dressings. Int. J. Pharm. 624, 122003. doi:10.1016/j.ijpharm.2022.122003
Mostafalu, P., Tamayol, A., Rahimi, R., Ochoa, M., Khalilpour, A., Kiaee, G., et al. (2018). Smart bandage for monitoring and treatment of chronic wounds. Small 14, 1703509. doi:10.1002/SMLL.201703509
Norbury, W., Herndon, D. N., Tanksley, J., Jeschke, M. G., and Finnerty, C. C. (2016). Infection in burns. Surg. Infect. (Larchmt). 17, 250–255. doi:10.1089/sur.2013.134
O’Neill, J. (2016). Tackling drug-resistant infections globally: final report and recommendations. https://apo.org.au/node/63983.
Ono, S., Imai, R., Ida, Y., Shibata, D., Komiya, T., and Matsumura, H. (2015). Increased wound pH as an indicator of local wound infection in second degree burns. Burns 41, 820–824. doi:10.1016/j.burns.2014.10.023
Pal, V. K., and Roy, S. (2022). Bioactive peptide nano-assemblies with pH-triggered shape transformation for antibacterial therapy. ACS Appl. Nano Mat. 5, 12019–12034. doi:10.1021/acsanm.2c03250
Pan, F., Zhang, S., Altenried, S., Zuber, F., Chen, Q., and Ren, Q. (2022). Advanced antifouling and antibacterial hydrogels enabled by controlled thermo-responses of a biocompatible polymer composite. Biomater. Sci. 10, 6146–6159. doi:10.1039/D2BM01244H
Paquette, D., and Falanga, V. (2002). Leg ulcers. Clin. Geriatr. Med. 18, 77–88. doi:10.1016/s0749-0690(03)00035-1
Percival, S. L., McCarty, S., Hunt, J. A., and Woods, E. J. (2014). The effects of pH on wound healing, biofilms, and antimicrobial efficacy. Wound Repair Regen. Off. Publ. Wound heal. Soc. And. Eur. Tissue Repair Soc. 22, 174–186. doi:10.1111/wrr.12125
Preman, N. K., Prabhu, A., Shaikh, S. B., Barki, R. R., Bhandary, Y. P., Rekha, P. D., et al. (2020). Bioresponsive supramolecular hydrogels for hemostasis, infection control and accelerated dermal wound healing. J. Mat. Chem. B 8, 8585–8598. doi:10.1039/D0TB01468K
Ramezani, M., and Monroe, M. B. B. (2023). Bacterial protease-responsive shape memory polymers for infection surveillance and biofilm inhibition in chronic wounds. J. Biomed. Mat. Res. Part A. 111, 921–937. doi:10.1002/jbm.a.37527
Schmid-Wendtner, M.-H., and Korting, H. C. (2006). The pH of the skin surface and its impact on the barrier function. Skin. Pharmacol. Physiol. 19, 296–302. doi:10.1159/000094670
Sharpe, J. R., Harris, K. L., Jubin, K., Bainbridge, N. J., and Jordan, N. R. (2009). The effect of pH in modulating skin cell behaviour. Br. J. Dermatol. 161, 671–673. doi:10.1111/j.1365-2133.2009.09168.x
Shukla, V. K., Shukla, D., Tiwary, S. K., Agrawal, S., and Rastogi, A. (2007). Evaluation of pH measurement as a method of wound assessment. J. Wound Care. 16, 291–294. doi:10.12968/jowc.2007.16.7.27062
Siddiqui, A. R., and Bernstein, J. M. (2010). Chronic wound infection: facts and controversies. Clin. Dermatol. 28, 519–526. doi:10.1016/j.clindermatol.2010.03.009
Smith, D. J. J., Thomson, P. D., Garner, W. L., and Rodriguez, J. L. (1994). Burn wounds: infection and healing. Am. J. Surg. 167, 46S–48S. doi:10.1016/0002-9610(94)90011-6
Su, Y., Andrabi, S. M., Shahriar, S. M. S., Wong, S. L., Wang, G., and Xie, J. (2023). Triggered release of antimicrobial peptide from microneedle patches for treatment of wound biofilms. J. Control. Release. 356, 131–141. doi:10.1016/j.jconrel.2023.02.030
Supuran, C. T., Scozzafava, A., and Mastrolorenzo, A. (2001). Bacterial proteases: current therapeutic use and future prospects for the development of new antibiotics. Expert Opin. Ther. Pat. 11, 221–259. doi:10.1517/13543776.11.2.221
Ullah, I., Wagih, M., Sun, Y., Li, Y., Hajdu, K., Courson, R., et al. (2023). Wirelessly powered drug-free and anti-infective smart bandage for chronic wound care. IEEE Trans. Biomed. Circuits Syst., 1–16. doi:10.1109/TBCAS.2023.3277318
Ventola, C. L. (2015). The antibiotic resistance crisis: part 1: causes and threats. P T 40, 277–283.
Vera-González, N., Bailey-Hytholt, C. M., Langlois, L., de Camargo Ribeiro, F., de Souza Santos, E. L., Junqueira, J. C., et al. (2020). Anidulafungin liposome nanoparticles exhibit antifungal activity against planktonic and biofilm <i>Candida albicans</i>. J. Biomed. Mat. Res. Part A. 108, 2263–2276. doi:10.1002/jbm.a.36984
Villanueva, M. E., Cuestas, M. L., Pérez, C. J., Campo Dall′ Orto, V., and Copello, G. J. (2019). Smart release of antimicrobial ZnO nanoplates from a pH-responsive keratin hydrogel. J. Colloid Interface Sci. 536, 372–380. doi:10.1016/j.jcis.2018.10.067
Wang, J., Chen, X.-Y., Zhao, Y., Yang, Y., Wang, W., Wu, C., et al. (2019). pH-switchable antimicrobial nanofiber networks of hydrogel eradicate biofilm and rescue stalled healing in chronic wounds. ACS Nano 13, 11686–11697. doi:10.1021/acsnano.9b05608
Wang, Y., and Shukla, A. (2022). Bacteria-responsive biopolymer-coated nanoparticles for biofilm penetration and eradication. Biomater. Sci. 10, 2831–2843. doi:10.1039/d2bm00361a
Wei, M., Gao, Y., Li, X., and Serpe, M. J. (2017). Stimuli-responsive polymers and their applications. Polym. Chem. 8, 127–143. doi:10.1039/c6py01585a
Weinrick, B., Dunman, P. M., McAleese, F., Murphy, E., Projan, S. J., Fang, Y., et al. (2004). Effect of mild acid on gene expression in Staphylococcus aureus. J. Bacteriol. 186, 8407–8423. doi:10.1128/JB.186.24.8407-8423.2004
Yang, K., Han, Q., Chen, B., Zheng, Y., Zhang, K., Li, Q., et al. (2018). Antimicrobial hydrogels: promising materials for medical application. Int. J. Nanomedicine. 13, 2217–2263. doi:10.2147/IJN.S154748
Ye, M., Zhao, Y., Wang, Y., Yodsanit, N., Xie, R., and Gong, S. (2020). pH-responsive polymer–drug conjugate: an effective strategy to combat the antimicrobial resistance. Adv. Funct. Mat. 30, 2002655. doi:10.1002/adfm.202002655
Young, L. (2021). Identifying infection in chronic wounds. Wound Pract. Res. J. Aust. Wound Manag. Assoc., doi:10.3316/informit.655721133752326
Yu, C., Alkekhia, D., and Shukla, A. (2020). β-Lactamase responsive supramolecular hydrogels with host–guest self-healing capability. ACS Appl. Polym. Mat. 2, 55–65. doi:10.1021/acsapm.9b00879
Yuan, Z., Gottsacker, C., He, X., Waterkotte, T., and Park, Y. C. (2022). Repetitive drug delivery using Light-Activated liposomes for potential antimicrobial therapies. Adv. Drug Deliv. Rev. 187, 114395. doi:10.1016/j.addr.2022.114395
Zhou, J., Yao, D., Qian, Z., Hou, S., Li, L., Jenkins, A. T. A., et al. (2018). Bacteria-responsive intelligent wound dressing: simultaneous in situ detection and inhibition of bacterial infection for accelerated wound healing. Biomaterials 161, 11–23. doi:10.1016/j.biomaterials.2018.01.024
Keywords: antimicrobial, smart materials, stimuli-responsive, wound infection, thermoresponsive, pH responsive, enzyme responsive
Citation: Monroe MBB and Fikhman DA (2023) Mini-review antimicrobial smart materials: the future’s defense against wound infections. Front. Front. Biomater. Sci. 2:1285386. doi: 10.3389/fbiom.2023.1285386
Received: 29 August 2023; Accepted: 31 October 2023;
Published: 24 November 2023.
Edited by:
Silviya Petrova Zustiak, Saint Louis University, United StatesReviewed by:
Akram Abbasi, Brown University, United StatesCopyright © 2023 Monroe and Fikhman. This is an open-access article distributed under the terms of the Creative Commons Attribution License (CC BY). The use, distribution or reproduction in other forums is permitted, provided the original author(s) and the copyright owner(s) are credited and that the original publication in this journal is cited, in accordance with accepted academic practice. No use, distribution or reproduction is permitted which does not comply with these terms.
*Correspondence: Mary Beth Browning Monroe, bWJtb25yb2VAc3lyLmVkdQ==