- Center for Life Nano and Neuro-Science (CLN2S), Istituto Italiano di Tecnologia (IIT), Rome, Italy
For decades, 3D bioprinting has offered a revolutionising approach to combine living cells and biomaterials to engineer complex, yet functional constructs. However, traditional 3D bioprinting platforms fall short of the ability to pattern complex gradients of biomaterials, cells, and ultimately bio-physical properties to drive tissue formation and regeneration. Recently, 3D microfluidic-assisted bioprinting (3DMB) has risen as a new hybrid approach for the fabrication of physiologically relevant tissues, adopting a microfluidic chip as functional printhead to achieve hierarchical patterning of bioinks and precise control over the microscale architecture of printed constructs, enabling the creation of multi-layered tissues. This review explores recent advancements in graded biomaterial patterning using microfluidic-assisted spinning and novel 3D bioprinting technologies. The physiological hierarchical arrangement of human tissues and the crucial role of biomaterials in achieving ordered assembly is hereby discussed. Lastly, the integration of microfluidic-assisted techniques with new bioprinting platforms is highlighted, examining the latest advancements in tissue regeneration and disease modelling.
1 Introduction
To date, 3D bioprinting platforms are capable of orchestrating the three-dimensional arrangement of living cells and biomaterial inks in a layer-by-layer fashion, for tissue engineering and regenerative medicine (TERM) purposes. The most relevant and widely used 3D bioprinting approaches for the engineering of 3D constructs include inkjet-based (Angelopoulos et al., 2020; Prabhakaran et al., 2022), laser-assisted (Heinrich et al., 2019), and extrusion-based bioprinting (Jeong et al., 2020). The specifics of these 3D bioprinting technologies are beyond the scope of this review and can be found in details elsewhere (Chameettachal et al., 2019; Cidonio et al., 2019; Scognamiglio et al., 2020; Lima et al., 2022; Karvinen and Kellomäki, 2023; Sabzevari et al., 2023).
Typically, 3D bioprinting processes are used to generate three-dimensional structures but fail to precisely tune the spatial distribution of functional elements (Marcotulli et al., 2023), resulting ultimately incapable of mimicking the complex physiological hierarchical structure of complex human tissues (Figure 1A).
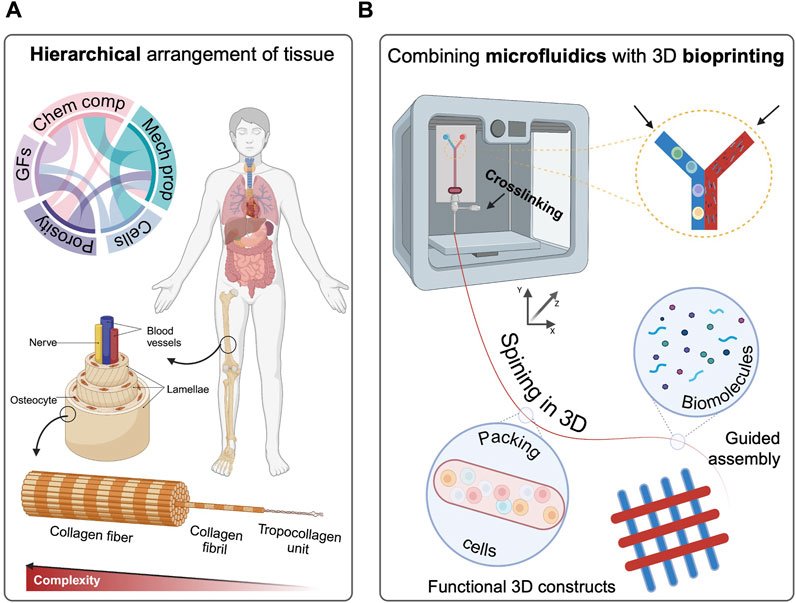
FIGURE 1. (A) Hierarchical physiological arrangement of tissue components, indicating organization level of the human body. (B) Combining microfluidic and EBB as a promising approach to mimic the natural architecture. Created with Biorender.com.
In recent years, the emerging of hybrid 3D bioprinting technologies such as the intersection of volumetric bioprinting and melt extrusion (Größbacher et al., 2023), fused deposition modelling (FDM) with integrated electrospinning (Micalizzi et al., 2023), the combination of inkjet printing and electrospinning (Xu et al., 2012) and developing computer-aided design fabrication techniques (Jung et al., 2016) have gained significant attention and demonstrated the potential to revolutionise the fabrication of complex tissue structures by addressing the limitations associated with conventional bioprinting methods (Idaszek et al., 2019; Kosik-Kozioł et al., 2019). Indeed, 3D microfluidic-assisted bioprinting (3DMB) is coming to the fore as a highly promising technology for the graded patterning of bioinks (cells and biomaterials) (Figure 1B), and the precise control over the ultimate tissue architecture and functionality (Davoodi et al., 2020; Swieszkowski et al., 2020). Herein, we explore the recent advancements in hierarchical patterning of biomaterials with microfluidic-assisted spinning and 3D bioprinting, highlighting the biomaterial inks explored so far, with particular emphasis on the promises treasured for future TERM applications.
2 Hierarchy in regeneration and modelling
Biological tissues are organised in ordered structures with multiple degrees of both functional and structural complexity, comprising cells residing within a tissue-specific extracellular matrix (ECM) (Rose and De Laporte, 2018). The complex macromolecular framework of ECM consists of a plethora of proteins, glycosaminoglycans, proteoglycans, and glycoproteins retained in a significant water volume (Salvatore et al., 2021). Biomimetic approaches aiming to recapitulate the architecture of human tissues hold extensive potential across various domains, encompassing drug screening (Wang et al., 2022c), disease modelling (Vanderburgh et al., 2017; Iafrate et al., 2022), organotypic models (Barrila et al., 2010), and tissue repair (Cidonio et al., 2021b; Szklanny et al., 2021). In this domain, hierarchically graded tissues refer to intricate biological structures in which the precise arrangement and coordination of diverse cell types, ECM, and functional components are regulated in a ordered patterning. This graded fashion extends across scales, ranging from the macro-to the micro-environment with stepwise changes that emulate the complexity of the native tissue (Fratzl, 2005; Zhang et al., 2022b).
2.1 Physiological hierarchical arrangement in human tissue
Living organisms present a remarkable level of organisation and structure, characterised by a graded arrangement that span from individual molecules to multicellular assemblies (Figure 1A). This hierarchy encompasses entities of varying dimensions and scales, interconnected through anisotropic relationships (Cidonio et al., 2021a). Within the highly organised framework of the human body, tissues can be typically classified into four major categories: epithelial, connective, muscle, and nervous tissues. Each tissue type serves unique purposes, resulting functional for the homeostasis of the human body (Betts et al., 2021). For instance, epithelial tissue is made of layered protective sheets of cells that cover surfaces of the body, line internal cavities and passages, and contribute to the formation of specific glands (Torras et al., 2018; Gómez-Gálvez et al., 2021; Mohammadi et al., 2023). In the complex stratified system of natural tissues, cells are found to engage in interactions with similar or other cell types, enabling essential communication that drives tissue morphogenesis and function (Song et al., 2019). Abundantly surrounding the cells, the ECM presents a diverse array of adhesion ligands, spatially arranged in 3D, that closely interact with cell surface receptors, transmitting signals (Tueni et al., 2023). The ECM translate biophysical signals and cues to cells, conditioning the mechanotransduction based on mechanical stiffness, and macromolecular composition. The structural features of ECM, such as the stratified organization and fibre arrangement, intensely impact cell behaviour, performance, and tissue function (Ratri et al., 2021). Notably, the anisotropic nature of ECM fibres, which exhibit hierarchical arrangement, can vary significantly across different tissues (Li et al., 2017).
Within every multicellular organism, cells undergo proliferation, leading to the formation and sustenance of tissues that consist of numerous specialized cell types. In these organisms, hierarchical structures are used to enhance self-renewing of the tissues (Derényi and Szöllősi, 2017). Embedded within the intricate hierarchy of multicellular organisms lies a select group of tissue-specific stem cells, distinguished by their remarkable attributes of self-replication and the capacity for differentiation. As cells undergo proliferation, they progressively specialize and acquire distinct functions, orchestrating the tissue development process (West et al., 2021).
2.2 Biomaterial and hierarchical assembly
Biomaterials for TERM applications are typically designed to resemble 3D porous, fibrous, or permeable structures to facilitate the transport of body fluids and gases while promoting crucial cellular interactions, viability, and the deposition of the ECM. Importantly, biomaterials must minimise inflammation and toxicity, demonstrating biocompatible features while ensuring a controlled degradation rate to match the regenerative process (Khan et al., 2022; Bertsch et al., 2023; Liu et al., 2023). Moreover, biomaterials should enhance cell attachment, and proliferation, facilitating the secretion of the ECM and ultimately, tissue repair (Nikolova and Chavali, 2019). Biomaterials derived from plants, animals, microorganisms, and native tissues, hold tremendous potential as scaffolding templates, tailored for specific applications in TERM. These materials mimic the physiological and ordered microstructure of tissue, as well as accelerate cell attachment, growth, and a multitude of functions within the native environment (Huang et al., 2017).
Functionally graded materials (FGMs) are a fitting example of the engineering of ordered systems. FGMs are indeed a class of materials that demonstrate discrete variation in composition, structure or properties throughout the entire volume, imitating the natural transitions between different tissues (Ituarte et al., 2019). The precise patterning and strategic control over ultimate mechanical properties is of great interest for engineering new functional physiological structures. Single- or multi-material FGMs hold the possibility to address common challenges in fabricating hierarchical structures, namely delamination, and cracking (Li et al., 2020). Similarly, biphasic inks including foams and high internal phase emulsion (HIPE) have been introduced to the field of 3D printing, enabling this technology for unequalled control over the creation of the hierarchical constructs with graded pore size. Researchers have reported numerous advantages, due to their superior properties for the hierarchical porous scaffold, resulting in better vascularization, improved cell viability and enhanced oxygen and other crucial nutrients transportation (Search et al., 2023). A number of approaches for fabricating hierarchically porous scaffolds using biphasic inks have been reported in our previous work (Cidonio et al., 2021a).
Today, a new paradigm based on microfluidic technology has emerged as an unparalleled tool capable of providing precise control while ensuring the build-up of consistent and stable 3D structures. Microfluidic systems not only have shown the potential to provide control over placement of the different components, allowing for the production of spatially defined gradients, but also the ability to dynamically modulate flow rates and concentrations enabling real-time adjustments to the tissue construct spontaneously assembling (Pi et al., 2018). Capability to customise the micro-size channels allows microfluidic chips to deliver fibres or 3D structures for tissue engineering and drug delivery purposes. Additionally, microfluidic chips are typically cost-effective, and eco-friendly due to reduced material waste in constructing complex materials with functional properties (Du Chatinier et al., 2021; Prabhakar et al., 2021).
3 Microfluidic spinning technology
Currently, a number of methods are available for producing fibrous materials via spinning technique, including polymer melt (Zhong et al., 2021), dry (Felgueiras et al., 2019), wet (Araki and Miyayama, 2020), and electrospinning (Medeiros et al., 2022). However, the effects of extreme operating conditions such as high pressure, shear, and temperature make these approaches disadvantageous for cell encapsulation, and ultimately, biofabrication. Furthermore, traditional spinning methods impose limitations on the selection of raw materials and hinder the production of fibres with unique structures. Currently, microfluidic technology, as a typical wet-spinning process, has been found capable of generating cell-laden fibres with governable compositions and multiplex structures (Zhang et al., 2022a).
Microfluidic spinning is now used as a fabrication method that enables the precise control of fluids at micro-scale level. By dispersing one liquid into another miscible or immiscible solution, this approach generates individual continuous fibres through physical or chemical reactions within microfluidic channels (Yu et al., 2018). The microchannels design is playing a crucial role in achieving desired fibre characteristics. The formation of functionalised fibres is facilitated by diverse channel geometries, including nested capillaries and microfluidic chips, such as T-junction, flow-convergence, and coaxial structured chips (Tian et al., 2023). Microfluidic spinning currently offers mild and rapid reaction conditions, facilitating the engineering of cell-laden microfibres (Cheng et al., 2017). This technology allows precise control of the preparation process, enabling the production of unique structures like hollow tubes and parallel micro-groove arrays. These structures hold significant promise in TERM applications, such as reconstructing blood vessels (Guo et al., 2021), skeletal muscle (Zhao et al., 2021; Fornetti et al., 2023), bone (Kim et al., 2023), nerves (Chen et al., 2020), and lung (Mazio et al., 2023) tissue. The remarkable advantages of microfluidic spinning have garnered considerable attention from TERM, disease modelling, and drug screening fields, contributing to the generation of a library of biomaterials that can be wet-spun into micro-size fibres. Recently, Utagawa and others developed a rapid approach to generate a core-shell fibre, prepared from interfacial polyelectrolyte complexation (IPC), chitosan, DNA as core and Ca-alginate serving as shell (Figure 2A). The authors showed that the fibre shape can be changed by adjusting the concentration of DNA, providing an adequate environment for HepG2, 3T3, HUVECs, and 3T3 cells co-culturing (Utagawa et al., 2023).
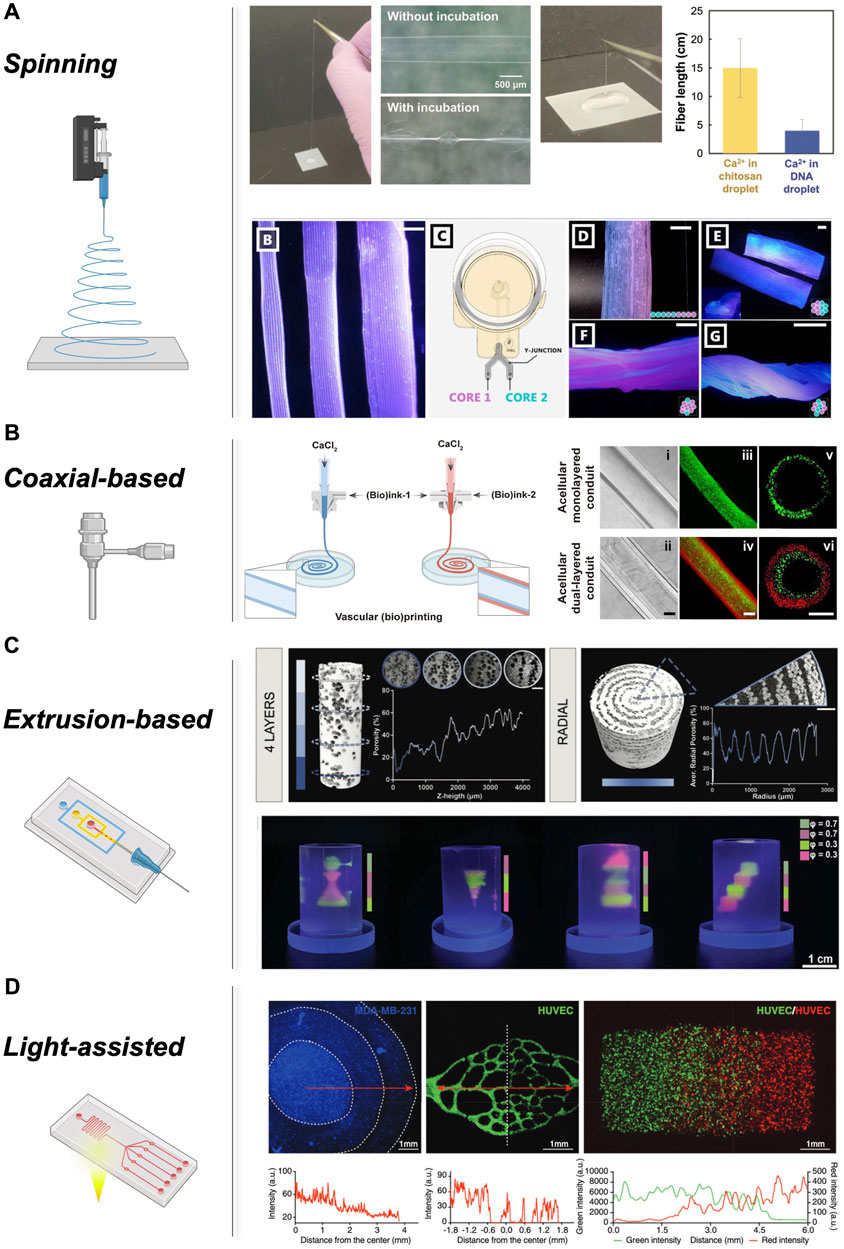
FIGURE 2. Different approaches in microfluidic 3D bioprinting: (A) Spun core-shell hydrogel fibre composed of chitosan/DNA IPC and the effect of Ca2+ presence in chitosan or DNA on fibre length. Adopted with permission from (Utagawa et al., 2023). Hydrogel fibres with different diameter, microfluidic printhead to fabricate multiple material core extrusion for single-layer, multi-layer and hydrogel bundle printing with gradual color transition, Multi-layered hydrogel bundles with variable core material fibres. Adopted from (Celikkin et al., 2023) under creative common attribution 4.0 license. (B) Microfluidic extrusion bioprinting process and different views of the final monolayered, dual-layered, and hollow tubes, using coaxial extruder. Adopted from (Wang et al., 2022a) under Creative Commons Attribution-Noncommercial license. (C) Micro-CT analysis for internal topographic of the porous FGMs, Multi-inlet microfluidic chip for alternating printing of GelMA, DexMA/nHA and DexMA emulsion. Complex structures with density gradient. Adopted with permission from (Marcotulli et al., 2023). (D) Light-assisted gradient bioprinting within hydrogels, indicating radial gradient in cell density, Encapsulating HUVECs, construct containing RFP-HUVECs and GFP-HUVECs and their fluorescence intensities. Adopted with permission from (Wang et al., 2022b).
3.1 Biomaterials to spin fibres
Material inks for rapid microfluidic-assisted spinning should be able to instantaneously assemble in continuous microfibres, while preserving biocompatible and biodegradable properties with adequate mechanical strength to support cell encapsulation and maturation (Zhao et al., 2022). A number of polymers have been placed under the spotlight as spinning biomaterials due to their biocompatibility and tuneable properties (Chen et al., 2022). Both natural and synthetic polymers can be adapted to be rapidly extruded using a microfluidic spinning platform. Typically, composite combinations of natural and synthetic polymers have been identified as ideal system for spinning. For TERM purposes, natural polymers, such as alginate, chitosan, hyaluronic acid (HA), collagen, and silk fibroin, are known for their biocompatibility and biodegradability. However, they generally exhibit weak mechanical strength. On the other hand, synthetic polymers offer strong mechanical properties and well-defined compositions but may suffer from limited biocompatibility. Synthetic polymers such as GelMa, polyethylene glycol (PEG), 4-hydroxybutyl acrylate (4-HBA), poly (ethylene glycol) dimethacrylates (PEGDMA), gelatin–hydroxyphenylpropionic acid (Gtn-HPA), poly (lactic-co-glycolic acid) (PLGA), and poly (caprolactone) (PCL) are highly versatile in mechanical strength, degradation rate, and cell interaction capabilities (Park et al., 2016; Gopinathan and Noh, 2018; Sung and Kim, 2020). Crucially, the material solution flowing through microfluidic channels must undergo instantaneous solidification, resulting in the microscale formation of fibres with precise geometrical structures (Perazzo et al., 2017; Zhao et al., 2021) with specific thixotropic properties. A number of methods are employed for solidification, including, chemical cross-linking (Andreazza et al., 2023), solvent exchange (Wu et al., 2022), ionic crosslinking (Mukherjee et al., 2023) and photo-crosslinking (Sang et al., 2023), with the last two being the most relevant.
3.2 Ionic crosslinking to rapidly gelate microfluidic-designed fibres
Ionic cross-linking is a popular approach to guide nearly instantaneous gelation of the spun fibre. This approach is based on the controlled interactions between negatively charged carboxyl groups, typically found in alginate-based materials, with multivalent cations present in cross-linking solutions (e.g., Ca2+, Al3+, and Mg2+) (Cidonio et al., 2020). Alginate (Zdiri et al., 2022), k-carrageenan (Kong and Ziegler, 2013), gellan gum (Gomes et al., 2023) and similar composites (Celikkin et al., 2023) are among the biocompatible polymers that have been spun into fibres. Microfluidic systems can be engineered to allow the divalent ions-rich solutions to be flushed coaxially with reactive biomaterials to facilitate rapid fibre formation (Fratini et al., 2023). However, the complete dependence on divalent ions for crosslinking holds certain disadvantages since ionic interactions are unregulated, and the loss of Ca2+ ions might result in an inconsistent gel matrix across different sections of the 3D printed object (Janarthanan et al., 2022).
3.3 Photocurable biomaterials for microfluidic spinning and processing
Photo cross-linkable polymers can be rapidly crosslinked upon exposure to specific wavelengths of light, enabling precise control over the crosslinking process (Xie et al., 2019; Thijssen et al., 2023). This technique is commonly employed for the crosslinking of methacrylated materials such as GelMA (Sharifi et al., 2021), PEGDA (Tan et al., 2008), and 4-HBA (Abdallah and Hadi Mohammed, 2023). In this process, a microfluidic system is utilized to facilitate the controlled flow of photopolymerization monomers and photo-initiators through the outlet. Herein, light is applied to trigger polymerization, resulting in the formation of micro-sized fibres or the rapid gelation of extruded materials. Typically, this method has been found to allow the fabrication of complex structures and facilitates the incorporation of bioactive molecules for enhanced cellular responses (Perazzo et al., 2017; Tian et al., 2023).
4 Microfluidic-assisted bioprinting for disease modelling and tissue regeneration
Microfluidic spinning holds tremendous advantages (Figure 2). The unparalleled ability to tune the fibre diameter, as well as the possibility to include multiple inlets comprising biomaterial and cells is appealing. However, single fibres are limited in recapitulating the complex physiological architecture of human tissues. 3D bioprinting has recently come to the rescue, with a number of different microfluidic-assisted approaches. Coaxial wet-spinning technology (Figure 2B) has been the first to explore the potential of single fibre spinning for the patterning of cellular and biochemical components (Han and Steckl, 2019). However, with the advent of microfluidic-based systems, a complex generation of hierarchical fibrous structures has been demonstrated. The possibility to engineer complex features thanks to the use of passive mixers and flow-focusing junctions is appealing and possible with microfluidic printheads for EBB (Figure 2C) and light-assisted (Figure 2D) platforms.
4.1 Coaxial-based 3D bioprinting technologies
Coaxial-based 3D bioprinting (Figure 2B) offers a continuous method for creating tubular micro-fibres generated from a concentric nozzle assembly that allows the simultaneous extrusion of two distinct solutions. By carefully selecting the hydrogel materials, the mechanical properties of the tubular structures, including toughness, stretchability, and compression resistance, can be precisely tailored to meet specific requirements (Millik et al., 2019; Liang et al., 2020; Bosch-Rué et al., 2021). Moreover, by increasing the number of coaxial orifices, vascular-like fibres can be easily fabricated. The coaxial technology is primarily relying on the use of instantaneous crosslinking of alginate (core) when exposed to divalent cations (sheet) while flowing. Indeed, Wang and co-workers utilized coaxial-driven 3D bioprinting to develop a mechanically stable double-layer hollow hydrogel (alginate and gelatin) for recreation of the vein and artery tissues. In this study, the core flow of CaCl2 enabled the in situ physical cross-linking of the alginate component in the (bio) ink. This cross-linking approach played a crucial role in preserving the tubular shape of the conduits. Furthermore, a bath containing a CaCl2/microbial transglutaminase (mTG) solution was utilized to reinforce the cross-linking of the bioprinted tubes. To mimic the structure and functionality of a native vein, a monolayered conduit was bioprinted with human umbilical vein smooth muscle cells (HUVSMCs) on its outer surface and perfused with HUVECs in the inner lumen. Following an additional week of incubation under similar culture conditions, a hollow venous conduit with a confluent endothelial layer inside and a thin, smooth muscle sheath on the outer surface was successfully constructed for further analysis (Wang et al., 2022a).
4.2 Microfluidic chip-driven extrusion-based bioprinting
Extrusion-based technology (Figure 2C) can be used in combination with microfluidic printheads to generate complex hierarchical structures. Recently, Marcotulli et al. demonstrated the printability of an oil-in-water emulsion using a new microfluidic printhead. The emulsion consisted of a cyclohexane-based organic phase dispersed in an aqueous phase composed of 20% w/v DexMA and 15% w/v Pluronic F-68, along with 1% w/v Irgacure 2,959 as a photoinitiator. Following the printing process, the samples were exposed to UV light at a wavelength of 365 nm to induce cross-linking. Thus, further architectural complexity was aided by the use of agarose-based support bath, demonstrating the ability of the new microfluidic-based technology in combination with FGMs to print highly complex physiologically-relevant structures (Marcotulli et al., 2023). More recently, extrusion-based microfluidic printing has been used to fabricate unique scaffolds infused with Chinese herbal medicine and a living microorganism for enhanced wound healing. The scaffold, composed of Panax notoginseng saponins (PNS) and microalgae Chlorella pyrenoidosa (MA), demonstrated controlled release of PNS, leading to improved cellular processes such as adhesion, proliferation, migration, and tube formation in vitro. Moreover, in vivo experiments with diabetic mice demonstrated the ability of scaffolds to alleviate local hypoxia, enhance angiogenesis, and accelerate wound closure after 14 days, highlighting the potential application for wound healing and tissue repair applications (Wang et al., 2023). Recently, a novel microfluidic-based bioprinting approach has been developed to house a highly efficient static mixer capable to spin multilayered microstructure fibres, demonstrating a continuous chaotic spinning with precise control over the internal structure of the deposited fibre (Chávez-Madero et al., 2020).
4.3 Microfluidic-driven light-assisted bioprinting
The versatile nature of microfluidic can be used to engineer new deposition approaches to aid light-based bioprinting (Figure 2D). Recently, Fournie et al. introduced a novel printhead design that utilizes hydrodynamic confinement for efficient microfluidic injection in the printing area. In this setup, an optical fibre is coupled to a 405-nm laser and integrated into the printhead. The adjustable power is used for photopolymerization during material injection. Unlike conventional approaches, the light is directly delivered within the printhead, near the fluidic delivery system, to guide rapid crosslinking (Fournié et al., 2023). Furthermore, Miri and others introduced a novel stereolithography bioprinting platform by incorporating a dynamic patterning system and a microfluidic device with pneumatic valves, where they achieved precise layer-by-layer bioprinting of 3D constructs. Utilizing vascular endothelial growth factor (VEGF)-loaded PEGDA and cell-laden GelMA, the authors successfully fabricated diverse hydrogel constructs which showed promise in neovascularization in a rat model (Miri et al., 2018). Creating complex tissue structures with gradients is a significant challenge in tissue engineering. Few methods have effectively achieved these gradients, which are crucial for functional engineered tissues. Thus, Wang and co-workers developed a composable-gradient Digital Light Processing (DLP)-based 3D bioprinting system that integrates a microfluidic mixer to generate continuous or discrete gradients of desired inks in real-time. In this work, various planar and 3D structures with gradients of materials, cell densities, growth factor concentrations, hydrogel stiffness, and porosities were successfully fabricated, highlighting the great, yet fully unexplored potential in biomedical application (Wang et al., 2022b).
5 Conclusion and future perspectives
To date, the complex hierarchical arrangement of the human body has not been yet recapitulated, slowing down the development of new therapies to regenerate damaged tissues. Microfluidic and 3D bioprinting can synergistically work towards the assembly of functional tissues, exploiting their combinatorial effect to generate complex and hierarchical constructs. Microfluidic spinning platforms can drive the ordered micro-assembly, resulting now capable of controlling mechanical flow, cells, drug and other molecules patterning, gradient building, and mixing. However, this approach fails to offer the fabrication of large and three-dimensional macro assemblies. Thus, the coupling of microfluidic chip as printheads with multi-axial computer-assisted manufacturing technology can rescue the current inability of modern biofabrication methodology to assemble hierarchically graded functional tissues. Nevertheless, microfluidic-assisted bioprinting add significant value to the creation of physiologically complex structures by providing spatial distribution of cells, biomaterials, and signalling factors (Peng et al., 2016), including engineered micro-fibrous vascularized tissues (Zhang et al., 2017). This precise control ensures that the manufactured structure closely resemble native tissues in terms of their organization and function.
Although microfluidic-assisted 3D bioprinting is now pacing to new unexplored frontiers in the patterning of cells and biomolecules, it is crucial to acknowledge the current limitations and challenges in terms of scalability, reproducibility, and long-term functionality of the fabricated constructs. Further research and development are needed to address these issues and optimize the integration of microfluidic spinning with biofabrication techniques for disease modelling and tissue regeneration. While microfluidic-assisted 3D bioprinting yet holds great promise, the ultimate clinical translation requires extensive validation and robustness testing in the years to come. Nevertheless, the innovative nature and the unparalleled potential of this technology to revolutionize tissue engineering and reduce animal testing should not be unnerved, facilitating and gardening new interest in the development of a better coupling of microfluidic and 3D bioprinting towards the engineer of hierarchical physiological tissues.
Author contributions
SM: Conceptualization, Data curation, Investigation, Visualization, Writing–original draft. GC: Conceptualization, Funding acquisition, Investigation, Project administration, Supervision, Visualization, Writing–original draft, Writing–review and editing.
Funding
The author(s) declare that no financial support was received for the research, authorship, and/or publication of this article.
Acknowledgments
GC acknowledges funding from Orthoregenerative Network No. 22-214.
Conflict of interest
The authors declare that the research was conducted in the absence of any commercial or financial relationships that could be construed as a potential conflict of interest.
The author GC declared that they were an editorial board member of Frontiers at the time of submission. This had no impact on the peer review process and the final decision.
Publisher’s note
All claims expressed in this article are solely those of the authors and do not necessarily represent those of their affiliated organizations, or those of the publisher, the editors and the reviewers. Any product that may be evaluated in this article, or claim that may be made by its manufacturer, is not guaranteed or endorsed by the publisher.
References
Abdallah, Z. S., and Hadi Mohammed, A. (2023). Crosslinked poly(hydroxybutyl acrylate-co-acrylamide) based hydrogels: synthesis, characterization, and performance evaluation in heavy metal removal. Polimery 68, 86–92. doi:10.14314/polimery.2023.2.2
Andreazza, R., Morales, A., Pieniz, S., and Labidi, J. (2023). Gelatin-based hydrogels: potential biomaterials for remediation. Polymers 15, 1026. doi:10.3390/polym15041026
Angelopoulos, I., Allenby, M. C., Lim, M., and Zamorano, M. (2020). Engineering inkjet bioprinting processes toward translational therapies. Biotechnol. Bioeng. 117, 272–284. doi:10.1002/bit.27176
Araki, J., and Miyayama, M. (2020). Wet spinning of cellulose nanowhiskers; fiber yarns obtained only from colloidal cellulose crystals. Polymer 188, 122116. doi:10.1016/j.polymer.2019.122116
Barrila, J., Radtke, A. L., Crabbé, A., Sarker, S. F., Herbst-Kralovetz, M. M., Ott, C. M., et al. (2010). Organotypic 3D cell culture models: using the rotating wall vessel to study host–pathogen interactions. Nat. Rev. Microbiol. 8, 791–801. doi:10.1038/nrmicro2423
Bertsch, C., Maréchal, H., Gribova, V., Lévy, B., Debry, C., Lavalle, P., et al. (2023). Biomimetic bilayered scaffolds for tissue engineering: from current design strategies to medical applications. Adv. Healthc. Mat. 12, 2203115. doi:10.1002/adhm.202203115
Betts, J. G., Young, K. A., and Wise, J. A. (2021). Anatomy and Physiology 2e. 2e ed. Place of publication not identified: OpenStax.
Bosch-Rué, E., Delgado, L. M., Gil, F. J., and Perez, R. A. (2021). Direct extrusion of individually encapsulated endothelial and smooth muscle cells mimicking blood vessel structures and vascular native cell alignment. Biofabrication 13, 015003. doi:10.1088/1758-5090/abbd27
Celikkin, N., Presutti, D., Maiullari, F., Volpi, M., Promovych, Y., Gizynski, K., et al. (2023). Combining rotary wet-spinning biofabrication and electro-mechanical stimulation for the in vitro production of functional myo-substitutes. Biofabrication 15, 045012. doi:10.1088/1758-5090/ace934
Chameettachal, S., Yeleswarapu, S., Sasikumar, S., Shukla, P., Hibare, P., Bera, A. K., et al. (2019). 3D bioprinting: recent trends and challenges. J. Indian Inst. Sci. 99, 375–403. doi:10.1007/s41745-019-00113-z
Chávez-Madero, C., De León-Derby, M. D., Samandari, M., Ceballos-González, C. F., Bolívar-Monsalve, E. J., Mendoza-Buenrostro, C., et al. (2020). Using chaotic advection for facile high-throughput fabrication of ordered multilayer micro- and nanostructures: continuous chaotic printing. Biofabrication 12, 035023. doi:10.1088/1758-5090/ab84cc
Chen, S., Wu, C., Liu, A., Wei, D., Xiao, Y., Guo, Z., et al. (2020). Biofabrication of nerve fibers with mimetic myelin sheath-like structure and aligned fibrous niche. Biofabrication 12, 035013. doi:10.1088/1758-5090/ab860d
Chen, Z., Lv, Z., Zhang, Z., Zhang, Y., and Cui, W. (2022). Biomaterials for microfluidic technology. Mat. futur. 1, 012401. doi:10.1088/2752-5724/ac39ff
Cheng, J., Jun, Y., Qin, J., and Lee, S.-H. (2017). Electrospinning versus microfluidic spinning of functional fibers for biomedical applications. Biomaterials 114, 121–143. doi:10.1016/j.biomaterials.2016.10.040
Cidonio, G., Costantini, M., Pierini, F., Scognamiglio, C., Agarwal, T., and Barbetta, A. (2021a). 3D printing of biphasic inks: beyond single-scale architectural control. J. Mat. Chem. C 9, 12489–12508. doi:10.1039/D1TC02117F
Cidonio, G., Glinka, M., Dawson, J. I., and Oreffo, R. O. C. (2019). The cell in the ink: improving biofabrication by printing stem cells for skeletal regenerative medicine. Biomaterials 209, 10–24. doi:10.1016/j.biomaterials.2019.04.009
Cidonio, G., Glinka, M., Kim, Y.-H., Dawson, J. I., and Oreffo, R. O. C. (2021b). “Nanocomposite clay-based bioinks for skeletal tissue engineering,” in Computer-aided tissue engineering methods in molecular biology. Editors A. Rainer, and L. Moroni (New York, NY: Springer US), 63–72. doi:10.1007/978-1-0716-0611-7_6
Cidonio, G., Glinka, M., Kim, Y.-H., Kanczler, J. M., Lanham, S. A., Ahlfeld, T., et al. (2020). Nanoclay-based 3D printed scaffolds promote vascular ingrowth ex vivo and generate bone mineral tissue in vitro and in vivo. Biofabrication 12, 035010. doi:10.1088/1758-5090/ab8753
Davoodi, E., Sarikhani, E., Montazerian, H., Ahadian, S., Costantini, M., Swieszkowski, W., et al. (2020). Extrusion and microfluidic-based bioprinting to fabricate biomimetic tissues and organs. Adv. Mat. Technol. 5, 1901044. doi:10.1002/admt.201901044
Derényi, I., and Szöllősi, G. J. (2017). Hierarchical tissue organization as a general mechanism to limit the accumulation of somatic mutations. Nat. Commun. 8, 14545. doi:10.1038/ncomms14545
Du Chatinier, D. N., Figler, K. P., Agrawal, P., Liu, W., and Zhang, Y. S. (2021). The potential of microfluidics-enhanced extrusion bioprinting. Biomicrofluidics 15, 041304. doi:10.1063/5.0033280
Felgueiras, H. P., Tavares, T. D., and Amorim, M. T. P. (2019). Biodegradable, spun nanocomposite polymeric fibrous dressings loaded with bioactive biomolecules for an effective wound healing: a review. IOP Conf. Ser. Mat. Sci. Eng. 634, 012033. doi:10.1088/1757-899X/634/1/012033
Fornetti, E., De Paolis, F., Fuoco, C., Bernardini, S., Giannitelli, S. M., Rainer, A., et al. (2023). A novel extrusion-based 3D bioprinting system for skeletal muscle tissue engineering. Biofabrication 15, 025009. doi:10.1088/1758-5090/acb573
Fournié, V., Venzac, B., Trevisiol, E., Foncy, J., Roul, J., Assie-Souleille, S., et al. (2023). A microfluidics-assisted photopolymerization method for high-resolution multimaterial 3D printing. Addit. Manuf. 72, 103629. doi:10.1016/j.addma.2023.103629
Fratini, C., Weaver, E., Moroni, S., Irwin, R., Dallal Bashi, Y. H., Uddin, S., et al. (2023). Combining microfluidics and coaxial 3D-bioprinting for the manufacturing of diabetic wound healing dressings. Biomater. Adv. 153, 213557. doi:10.1016/j.bioadv.2023.213557
Fratzl, P. (2005). “Hierarchical structure and mechanical adaptation of biological materials,” in Learning from nature How to design new implantable biomaterialsis: from biomineralization Fundamentals to biomimetic Materials and processing routes NATO science series II: mathematics, physics and chemistry. Editors R. L. Reis, and S. Weiner (Dordrecht: Springer Netherlands), 15–34. doi:10.1007/1-4020-2648-X_2
Gomes, D., Batista-Silva, J. P., Sousa, A., and Passarinha, L. A. (2023). Progress and opportunities in Gellan gum-based materials: a review of preparation, characterization and emerging applications. Carbohydr. Polym. 311, 120782. doi:10.1016/j.carbpol.2023.120782
Gómez-Gálvez, P., Vicente-Munuera, P., Anbari, S., Buceta, J., and Escudero, L. M. (2021). The complex three-dimensional organization of epithelial tissues. Development 148, dev195669. doi:10.1242/dev.195669
Gopinathan, J., and Noh, I. (2018). Recent trends in bioinks for 3D printing. Biomater. Res. 22, 11. doi:10.1186/s40824-018-0122-1
Größbacher, G., Bartolf-Kopp, M., Gergely, C., Bernal, P. N., Florczak, S., De Ruijter, M., et al. (2023). Volumetric printing across melt electrowritten scaffolds fabricates multi-material living constructs with tunable architecture and mechanics. Adv. Mat. 35, 2300756. doi:10.1002/adma.202300756
Guo, Y., Yan, J., Xin, J. H., Wang, L., Yu, X., Fan, L., et al. (2021). Microfluidic-directed biomimetic Bulbine torta -like microfibers based on inhomogeneous viscosity rope-coil effect. Lab. Chip 21, 2594–2604. doi:10.1039/D1LC00252J
Han, D., and Steckl, A. J. (2019). Coaxial electrospinning formation of complex polymer fibers and their applications. ChemPlusChem 84, 1453–1497. doi:10.1002/cplu.201900281
Heinrich, M. A., Liu, W., Jimenez, A., Yang, J., Akpek, A., Liu, X., et al. (2019). 3D bioprinting: from benches to translational applications. Small, 1805510. doi:10.1002/smll.201805510
Huang, G., Li, F., Zhao, X., Ma, Y., Li, Y., Lin, M., et al. (2017). Functional and biomimetic materials for engineering of the three-dimensional cell microenvironment. Chem. Rev. 117, 12764–12850. doi:10.1021/acs.chemrev.7b00094
Iafrate, L., Benedetti, M. C., Donsante, S., Rosa, A., Corsi, A., Oreffo, R. O. C., et al. (2022). Modelling skeletal pain harnessing tissue engineering. Vitro Models 1, 289–307. doi:10.1007/s44164-022-00028-7
Idaszek, J., Costantini, M., Karlsen, T. A., Jaroszewicz, J., Colosi, C., Testa, S., et al. (2019). 3D bioprinting of hydrogel constructs with cell and material gradients for the regeneration of full-thickness chondral defect using a microfluidic printing head. Biofabrication 11, 044101. doi:10.1088/1758-5090/ab2622
Ituarte, I. F., Boddeti, N., Hassani, V., Dunn, M. L., and Rosen, D. W. (2019). Design and additive manufacture of functionally graded structures based on digital materials. Addit. Manuf. 30, 100839. doi:10.1016/j.addma.2019.100839
Janarthanan, G., Kim, J. H., Kim, I., Lee, C., Chung, E.-J., and Noh, I. (2022). Manufacturing of self-standing multi-layered 3D-bioprinted alginate-hyaluronate constructs by controlling the cross-linking mechanisms for tissue engineering applications. Biofabrication 14, 035013. doi:10.1088/1758-5090/ac6c4c
Jeong, H.-J., Nam, H., Jang, J., and Lee, S.-J. (2020). 3D bioprinting strategies for the regeneration of functional tubular tissues and organs. Bioengineering 7, 32. doi:10.3390/bioengineering7020032
Jung, J. W., Lee, J.-S., and Cho, D.-W. (2016). Computer-aided multiple-head 3D printing system for printing of heterogeneous organ/tissue constructs. Sci. Rep. 6, 21685. doi:10.1038/srep21685
Karvinen, J., and Kellomäki, M. (2023). Design aspects and characterization of hydrogel-based bioinks for extrusion-based bioprinting. Bioprinting 32, e00274. doi:10.1016/j.bprint.2023.e00274
Khan, K., Gasbarrino, K., Mahmoud, I., Dufresne, L., Daskalopoulou, S. S., Schwertani, A., et al. (2022). Bioactive scaffolds in stem cell-based therapies for myocardial infarction: a systematic review and meta-analysis of preclinical trials. Stem Cell. Rev. Rep. 18, 2104–2136. doi:10.1007/s12015-021-10186-y
Kim, M. K., Paek, K., Woo, S.-M., and Kim, J. A. (2023). Bone-on-a-Chip: biomimetic models based on microfluidic technologies for biomedical applications. ACS Biomater. Sci. Eng. acsbiomaterials, 9, 3058–3073. doi:10.1021/acsbiomaterials.3c00066
Kong, L., and Ziegler, G. R. (2013). Fabrication of κ-carrageenan fibers by wet spinning: addition of ι-carrageenan. Food Hydrocoll. 30, 302–306. doi:10.1016/j.foodhyd.2012.06.011
Kosik-Kozioł, A., Costantini, M., Mróz, A., Idaszek, J., Heljak, M., Jaroszewicz, J., et al. (2019). 3D bioprinted hydrogel model incorporating β -tricalcium phosphate for calcified cartilage tissue engineering. Biofabrication 11, 035016. doi:10.1088/1758-5090/ab15cb
Li, Q., Qu, F., Han, B., Wang, C., Li, H., Mauck, R. L., et al. (2017). Micromechanical anisotropy and heterogeneity of the meniscus extracellular matrix. Acta Biomater. 54, 356–366. doi:10.1016/j.actbio.2017.02.043
Li, Y., Feng, Z., Hao, L., Huang, L., Xin, C., Wang, Y., et al. (2020). A review on functionally graded materials and structures via additive manufacturing: from multi-scale design to versatile functional properties. Adv. Mat. Technol. 5, 1900981. doi:10.1002/admt.201900981
Liang, Q., Gao, F., Zeng, Z., Yang, J., Wu, M., Gao, C., et al. (2020). Coaxial scale-up printing of diameter-tunable biohybrid hydrogel microtubes with high strength, perfusability, and endothelialization. Adv. Funct. Mat. 30, 2001485. doi:10.1002/adfm.202001485
Lima, T. D. P. L., Canelas, C. A. D. A., Concha, V. O. C., Costa, F. A. M. D., and Passos, M. F. (2022). 3D bioprinting technology and hydrogels used in the process. J. Funct. Biomater. 13, 214. doi:10.3390/jfb13040214
Liu, S., Yu, J.-M., Gan, Y.-C., Qiu, X.-Z., Gao, Z.-C., Wang, H., et al. (2023). Biomimetic natural biomaterials for tissue engineering and regenerative medicine: new biosynthesis methods, recent advances, and emerging applications. Mil. Med. Res. 10, 16. doi:10.1186/s40779-023-00448-w
Marcotulli, M., Tirelli, M. C., Volpi, M., Jaroszewicz, J., Scognamiglio, C., Kasprzycki, P., et al. (2023). Microfluidic 3D printing of emulsion ink for engineering porous functionally graded materials. Adv. Mat. Technol. 8, 2201244. doi:10.1002/admt.202201244
Mazio, C., Scognamiglio, L. S., Passariello, R., Panzetta, V., Casale, C., Urciuolo, F., et al. (2023). Easy-to-Build and reusable microfluidic device for the dynamic culture of human bronchial cystic fibrosis epithelia. ACS Biomater. Sci. Eng. 9, 2780–2792. doi:10.1021/acsbiomaterials.2c01460
Medeiros, G. B., Lima, F. D. A., De Almeida, D. S., Guerra, V. G., and Aguiar, M. L. (2022). Modification and functionalization of fibers formed by electrospinning: a review. Membranes 12, 861. doi:10.3390/membranes12090861
Micalizzi, S., Russo, L., Giacomelli, C., Montemurro, F., Maria, C. de, Nencioni, M., et al. (2023). Multimaterial and multiscale scaffold for engineering enthesis organ. Int. J. Bioprinting 9, 763. doi:10.18063/ijb.763
Millik, S. C., Dostie, A. M., Karis, D. G., Smith, P. T., McKenna, M., Chan, N., et al. (2019). 3D printed coaxial nozzles for the extrusion of hydrogel tubes toward modeling vascular endothelium. Biofabrication 11, 045009. doi:10.1088/1758-5090/ab2b4d
Miri, A. K., Nieto, D., Iglesias, L., Goodarzi Hosseinabadi, H., Maharjan, S., Ruiz-Esparza, G. U., et al. (2018). Microfluidics-enabled multimaterial maskless stereolithographic bioprinting. Adv. Mat. 30, 1800242. doi:10.1002/adma.201800242
Mohammadi, S., Jabbari, F., and Babaeipour, V. (2023). Bacterial cellulose-based composites as vehicles for dermal and transdermal drug delivery: a review. Int. J. Biol. Macromol. 242, 124955. doi:10.1016/j.ijbiomac.2023.124955
Mukherjee, K., Dutta, P., and Giri, T. K. (2023). Al3+/Ca2+ cross-linked hydrogel matrix tablet of etherified tara gum for sustained delivery of tramadol hydrochloride in gastrointestinal milieu. Int. J. Biol. Macromol. 232, 123448. doi:10.1016/j.ijbiomac.2023.123448
Nikolova, M. P., and Chavali, M. S. (2019). Recent advances in biomaterials for 3D scaffolds: a review. Bioact. Mat. 4, 271–292. doi:10.1016/j.bioactmat.2019.10.005
Park, J.-H., Han, C.-M., Lee, E.-J., and Kim, H.-W. (2016). Preparation of highly monodispersed porous-channeled poly(caprolactone) microspheres by a microfluidic system. Mat. Lett. 181, 92–98. doi:10.1016/j.matlet.2016.06.020
Peng, W., Unutmaz, D., and Ozbolat, I. T. (2016). Bioprinting towards physiologically relevant tissue models for pharmaceutics. Trends Biotechnol. 34, 722–732. doi:10.1016/j.tibtech.2016.05.013
Perazzo, A., Nunes, J. K., Guido, S., and Stone, H. A. (2017). Flow-induced gelation of microfiber suspensions. Proc. Natl. Acad. Sci. 114, E8557-E8564. doi:10.1073/pnas.1710927114
Pi, Q., Maharjan, S., Yan, X., Liu, X., Singh, B., Van Genderen, A. M., et al. (2018). Digitally tunable microfluidic bioprinting of multilayered cannular tissues. Adv. Mat. 30, 1706913. doi:10.1002/adma.201706913
Prabhakar, P., Sen, R. K., Dwivedi, N., Khan, R., Solanki, P. R., Srivastava, A. K., et al. (2021). 3D-Printed microfluidics and potential biomedical applications. Front. Nanotechnol. 3, 609355. doi:10.3389/fnano.2021.609355
Prabhakaran, P., Palaniyandi, T., Kanagavalli, B., Ram Kumar, V., Hari, R., Sandhiya, V., et al. (2022). Prospect and retrospect of 3D bio-printing. Acta histochem. 124, 151932. doi:10.1016/j.acthis.2022.151932
Ratri, M. C., Brilian, A. I., Setiawati, A., Nguyen, H. T., Soum, V., and Shin, K. (2021). Recent advances in regenerative tissue fabrication: tools, materials, and microenvironment in hierarchical aspects. Adv. NanoBiomed Res. 1, 2000088. doi:10.1002/anbr.202000088
Rose, J. C., and De Laporte, L. (2018). Hierarchical design of tissue regenerative constructs. Adv. Healthc. Mat. 7, 1701067. doi:10.1002/adhm.201701067
Sabzevari, A., Rayat Pisheh, H., Ansari, M., and Salati, A. (2023). Progress in bioprinting technology for tissue regeneration. J. Artif. Organs. doi:10.1007/s10047-023-01394-z
Salvatore, L., Gallo, N., Natali, M. L., Terzi, A., Sannino, A., and Madaghiele, M. (2021). Mimicking the hierarchical organization of natural collagen: toward the development of ideal scaffolding material for tissue regeneration. Front. Bioeng. Biotechnol. 9, 644595. doi:10.3389/fbioe.2021.644595
Sang, S., Mao, X., Cao, Y., Liu, Z., Shen, Z., Li, M., et al. (2023). 3D bioprinting using synovium-derived MSC-laden photo-cross-linked ECM bioink for cartilage regeneration. ACS Appl. Mat. Interfaces 15, 8895–8913. doi:10.1021/acsami.2c19058
Scognamiglio, C., Soloperto, A., Ruocco, G., and Cidonio, G. (2020). Bioprinting stem cells: building physiological tissues one cell at a time. Am. J. Physiol.-Cell Physiol. 319, C465–C480. doi:10.1152/ajpcell.00124.2020
Search, J., Mahjoubnia, A., Chen, A. C., Deng, H., Tan, A. J., Chen, S., et al. (2023). 3D-printing of selectively porous, freestanding structures via humidity-induced rapid phase change. Addit. Manuf. 68, 103514. doi:10.1016/j.addma.2023.103514
Sharifi, S., Sharifi, H., Akbari, A., and Chodosh, J. (2021). Systematic optimization of visible light-induced crosslinking conditions of gelatin methacryloyl (GelMA). Sci. Rep. 11, 23276. doi:10.1038/s41598-021-02830-x
Song, X., Tetik, H., Jirakittsonthon, T., Parandoush, P., Yang, G., Lee, D., et al. (2019). Biomimetic 3D printing of hierarchical and interconnected porous hydroxyapatite structures with high mechanical strength for bone cell culture. Adv. Eng. Mat. 21, 1800678. doi:10.1002/adem.201800678
Sung, Y. K., and Kim, S. W. (2020). Recent advances in polymeric drug delivery systems. Biomater. Res. 24, 12. doi:10.1186/s40824-020-00190-7
Swieszkowski, W., Dokmeci, M. R., and Khademhosseini, A. (2020). Microfluidics in biofabrication. Biofabrication 12, 030201. doi:10.1088/1758-5090/ab7e75
Szklanny, A. A., Machour, M., Redenski, I., Chochola, V., Goldfracht, I., Kaplan, B., et al. (2021). 3D bioprinting of engineered tissue flaps with hierarchical vessel networks (VesselNet) for direct host-to-implant perfusion. Adv. Mat. 33, 2102661. doi:10.1002/adma.202102661
Tan, G., Wang, Y., Li, J., and Zhang, S. (2008). Synthesis and characterization of injectable photocrosslinking poly (ethylene glycol) diacrylate based hydrogels. Polym. Bull. 61, 91–98. doi:10.1007/s00289-008-0932-8
Thijssen, Q., Quaak, A., Toombs, J., De Vlieghere, E., Parmentier, L., Taylor, H., et al. (2023). Volumetric printing of thiol-ene photo-cross-linkable poly(ε-caprolactone): a tunable material platform serving biomedical applications. Adv. Mat. 35, 2210136. doi:10.1002/adma.202210136
Tian, L., Ma, J., Li, W., Zhang, X., and Gao, X. (2023). Microfiber fabricated via microfluidic spinning toward tissue engineering applications. Macromol. Biosci. 23, 2200429. doi:10.1002/mabi.202200429
Torras, N., García-Díaz, M., Fernández-Majada, V., and Martínez, E. (2018). Mimicking epithelial tissues in three-dimensional cell culture models. Front. Bioeng. Biotechnol. 6, 197. doi:10.3389/fbioe.2018.00197
Tueni, N., Allain, J.-M., and Genet, M. (2023). On the structural origin of the anisotropy in the myocardium: multiscale modeling and analysis. J. Mech. Behav. Biomed. Mat. 138, 105600. doi:10.1016/j.jmbbm.2022.105600
Utagawa, Y., Ino, K., Takinoue, M., and Shiku, H. (2023). Fabrication and cell culture applications of core-shell hydrogel fibers composed of chitosan/DNA interfacial polyelectrolyte complexation and calcium alginate: straight and beaded core variations. Adv. Healthc. Mat., 2302011. doi:10.1002/adhm.202302011
Vanderburgh, J., Sterling, J. A., and Guelcher, S. A. (2017). 3D printing of tissue engineered constructs for in vitro modeling of disease progression and drug screening. Ann. Biomed. Eng. 45, 164–179. doi:10.1007/s10439-016-1640-4
Wang, D., Maharjan, S., Kuang, X., Wang, Z., Mille, L. S., Tao, M., et al. (2022a). Microfluidic bioprinting of tough hydrogel-based vascular conduits for functional blood vessels. Sci. Adv. 8, eabq6900. doi:10.1126/sciadv.abq6900
Wang, M., Li, W., Mille, L. S., Ching, T., Luo, Z., Tang, G., et al. (2022b). Digital light processing based bioprinting with composable gradients. Adv. Mat. 34, 2107038. doi:10.1002/adma.202107038
Wang, X., Jia, J., Niu, M., Li, W., and Zhao, Y. (2023). Living Chinese herbal scaffolds from microfluidic bioprinting for wound healing. Research 6, 0138. doi:10.34133/research.0138
Wang, Y., Kankala, R. K., Ou, C., Chen, A., and Yang, Z. (2022c). Advances in hydrogel-based vascularized tissues for tissue repair and drug screening. Bioact. Mat. 9, 198–220. doi:10.1016/j.bioactmat.2021.07.005
West, J., Schenck, R. O., Gatenbee, C., Robertson-Tessi, M., and Anderson, A. R. A. (2021). Normal tissue architecture determines the evolutionary course of cancer. Nat. Commun. 12, 2060. doi:10.1038/s41467-021-22123-1
Wu, M., Chen, X., Xu, J., and Zhang, H. (2022). Freeze-thaw and solvent-exchange strategy to generate physically cross-linked organogels and hydrogels of curdlan with tunable mechanical properties. Carbohydr. Polym. 278, 119003. doi:10.1016/j.carbpol.2021.119003
Xie, H., Yang, K.-K., and Wang, Y.-Z. (2019). Photo-cross-linking: a powerful and versatile strategy to develop shape-memory polymers. Prog. Polym. Sci. 95, 32–64. doi:10.1016/j.progpolymsci.2019.05.001
Xu, T., Binder, K. W., Albanna, M. Z., Dice, D., Zhao, W., Yoo, J. J., et al. (2012). Hybrid printing of mechanically and biologically improved constructs for cartilage tissue engineering applications. Biofabrication 5, 015001. doi:10.1088/1758-5082/5/1/015001
Yu, Y., Shang, L., Guo, J., Wang, J., and Zhao, Y. (2018). Design of capillary microfluidics for spinning cell-laden microfibers. Nat. Protoc. 13, 2557–2579. doi:10.1038/s41596-018-0051-4
Zdiri, K., Cayla, A., Elamri, A., Erard, A., and Salaun, F. (2022). Alginate-based bio-composites and their potential applications. J. Funct. Biomater. 13, 117. doi:10.3390/jfb13030117
Zhang, M., Peng, X., Fan, P., Zhou, Y., and Xiao, P. (2022a). Recent progress in preparation and application of fibers using microfluidic spinning technology. Macromol. Chem. Phys. 223, 2100451. doi:10.1002/macp.202100451
Zhang, S., Ju, W., Chen, X., Zhao, Y., Feng, L., Yin, Z., et al. (2022b). Hierarchical ultrastructure: an overview of what is known about tendons and future perspective for tendon engineering. Bioact. Mat. 8, 124–139. doi:10.1016/j.bioactmat.2021.06.007
Zhang, Y. S., Pi, Q., and Genderen, A. M. van (2017). Microfluidic bioprinting for engineering vascularized tissues and organoids. JoVE J. Vis. Exp., e55957. doi:10.3791/55957
Zhao, G., Zhou, H., Jin, G., Jin, B., Geng, S., Luo, Z., et al. (2022). Rational design of electrically conductive biomaterials toward excitable tissues regeneration. Prog. Polym. Sci. 131, 101573. doi:10.1016/j.progpolymsci.2022.101573
Zhao, M., Liu, H., Zhang, X., Wang, H., Tao, T., and Qin, J. (2021). A flexible microfluidic strategy to generate grooved microfibers for guiding cell alignment. Biomater. Sci. 9, 4880–4890. doi:10.1039/D1BM00549A
Keywords: microfluidic, biofabrication, hierarchical constructs, gradients, functionally graded materials (FGM), bioprinting
Citation: Mohammadi S and Cidonio G (2023) Unravelling hierarchical patterning of biomaterial inks with 3D microfluidic-assisted spinning: a paradigm shift in bioprinting technologies. Front. Front. Biomater. Sci. 2:1279061. doi: 10.3389/fbiom.2023.1279061
Received: 17 August 2023; Accepted: 06 October 2023;
Published: 17 October 2023.
Edited by:
Valeria Chiono, Polytechnic University of Turin, ItalyReviewed by:
Amir K. Miri, New Jersey Institute of Technology, United StatesCarmelo De Maria, University of Pisa, Italy
Copyright © 2023 Mohammadi and Cidonio. This is an open-access article distributed under the terms of the Creative Commons Attribution License (CC BY). The use, distribution or reproduction in other forums is permitted, provided the original author(s) and the copyright owner(s) are credited and that the original publication in this journal is cited, in accordance with accepted academic practice. No use, distribution or reproduction is permitted which does not comply with these terms.
*Correspondence: Gianluca Cidonio, Z2lhbmx1Y2EuY2lkb25pb0BpaXQuaXQ=