- 1Biosciences and Biotechnology Division, Lawrence Livermore National Laboratory, Livermore, CA, United States
- 2Department of Biochemistry and Molecular & Cellular Biology, Georgetown University, Washington, DC, United States
- 3Materials Engineering Division, Lawrence Livermore National Laboratory, Livermore, CA, United States
Chloroplasts are critical organelles in plants and algae responsible for accumulating biomass through photosynthetic carbon fixation and cellular maintenance through metabolism in the cell. Chloroplasts are increasingly appreciated for their role in biomanufacturing, as they can produce many useful molecules, and a deeper understanding of chloroplast regulation and function would provide more insight for the biotechnological applications of these organelles. However, traditional genetic approaches to manipulate chloroplasts are slow, and generation of transgenic organisms to study their function can take weeks to months, significantly delaying the pace of research. To develop chloroplasts themselves as a quicker and more defined platform, we isolated chloroplasts from the green algae, Chlamydomonas reinhardtii, and examined their photosynthetic function after extraction. Combined with a metabolic modeling approach using flux-balance analysis, we identified key metabolic reactions essential to chloroplast function and leveraged this information into reagents that can be used in a “chloroplast media” capable of maintaining chloroplast photosynthetic function over time ex vivo compared to buffer alone. We envision this could serve as a model platform to enable more rapid design-build-test-learn cycles to study and improve chloroplast function in combination with genetic modifications and potentially as a starting point for the bottom-up design of a synthetic organelle-containing cell.
1 Introduction
Chloroplasts are critical organelles in plant and algal cells, playing key roles in metabolism and cellular maintenance. Beyond their well-known function in carbon fixation through photosynthesis, chloroplasts are increasingly recognized for their involvement in diverse metabolic pathways, such as lipid biosynthesis (Kunst et al., 1988) and amino acid production (Reyes-Prieto and Moustafa, 2012; Chen et al., 2018). These pathways make chloroplasts valuable not only for agriculture, where they can enhance crop yield (Galmes et al., 2014), but also for biomanufacturing, as they have been used to produce biofuels (Georgianna and Mayfield, 2012), pharmaceuticals (Khan et al., 2018), and high-value compounds such as vitamins and pigments (Gedi et al., 2017; Bock, 2014; Maliga and Bock, 2011).
Despite their importance, studying chloroplast function through genetic approaches is laborious and time-consuming, often requiring many weeks to generate mutants even within relatively simple and well-defined model organisms such as the single celled green algae Chlamydomonas reinhardtii (Wang et al., 2023). Additionally, dissecting specific biochemical pathways within chloroplasts can be challenging due to the complexity and “noise” of the cellular environment. These limitations hinder progress in both basic research and the development of biotechnological applications related to chloroplasts.
In vitro systems potentially offer a more controlled approach to study chloroplast function, removing the interference from other cellular processes. Maintaining chloroplast functionality outside of their native cellular context is difficult though, as they rapidly lose photosynthetic activity after isolation over the course of a few days (Green et al., 2005). However, there is evidence that they can be maintained in a prolonged viable state in non-native contexts. Notably, various sacoglossan sea slugs have demonstrated the ability to “steal” chloroplasts from their algal food sources, termed kleptoplasty, and maintain them in a photosynthetically active state for weeks to months inside their own cells (Cruz and Cartaxana, 2022). Intrigued by this, we posited that there must exist a set of conditions under which chloroplasts can artificially be kept photosynthetically functional outside of their host cell.
Here, we leveraged the model organism C. reinhardtii and computational modeling to simulate key metabolic networks within chloroplasts and develop an initial defined chemical medium for maintaining chloroplast function ex vivo. We performed flux balance analysis (FBA) using a system-scale model of the C. reinhardtii chloroplast (Bjerkelund Rokke et al., 2020) to analyze single-reaction knockout phenotypes. Through these simulations, we identified essential reactions and metabolites spanning several classes of molecules, notably amino acids, nucleotides, and magnesium ions. Noting the importance of protein synthesis in chloroplast function (Sundby et al., 1993a), we expanded the medium to involve components commonly used in in vitro transcription/translation buffers and showed improved maintenance of photosynthetic function of chloroplasts ex vivo compared to storage in sorbitol cushioned HEPES buffer alone.
2 Results
2.1 Photosynthetic function of extracted chloroplasts is temperature dependent
To determine whether functional chloroplast lifetimes can be modulated outside of their host cell, we extracted chloroplasts from C. reinhardtii and measured their photosynthetic function after extraction using pulse amplitude modulated (PAM) fluorometry (Figure 1A). Percoll/ficoll density gradients allowed for the efficient separation of intact chloroplasts from whole cells and thylakoid membranes (Mason et al., 2006) (Figure 1B) where strong membrane-bound chlorophyll a signal could be detected in individual chloroplasts. Because C. reinhardtii contain only a single chloroplast per cell, we were able to measure our recovery rate by counting the number of isolated chloroplasts, obtaining >70% recovery on average (Figure 1C).
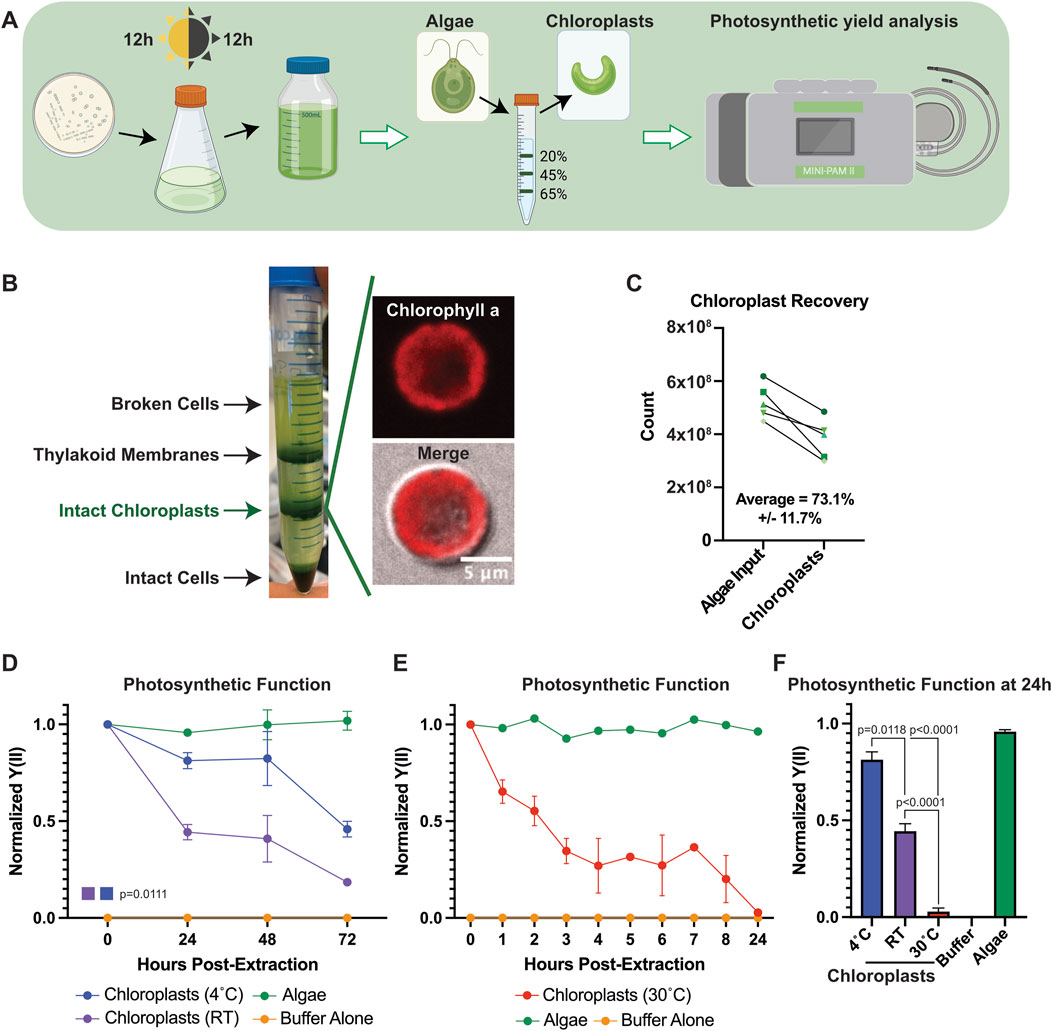
Figure 1. Chloroplast function ex vivo can be modulated by temperature. (A) Schematic describing the experimental pipeline for C. reinhardtii culture, chloroplast extraction, and photosynthetic measurements. (B) Image of representative chloroplast extraction with (inset) confocal micrographs of an extracted chloroplast. (C) Line graph showing chloroplast recovery from algal cells. (D) Line graphs showing normalized Y(II) values over time at 4°C and RT. P-values calculated using repeated measures 2-way ANOVA. (E) Line graphs showing normalized Y(II) values over time at 30°C. (F) Bar graph comparing normalized Y(II) values at 24 h post extraction at various temperatures. P-values calculated by Student’s t-test.
Chloroplasts have a limited functional lifespan after extraction from their host cell. Indeed, we observed results consistent with those reported in the literature (Green et al., 2005), where most chloroplast photosynthetic functionality was lost within 72 h of extraction in sorbitol cushioned HEPES buffer (HEPES buffer) (Figure 1D). We observed a sharp decline in the effective quantum yield of photosystem II (Y(II)) through PAM measurements within the first 24 h, before tapering off through 72 h, when stored at room temperature (RT). We observed a significant delay in the rate of Y(II) decline in extracted chloroplasts when stored at 4°C compared to RT (p = 0.0111), suggesting that chloroplast viability after removal from host cells could be influenced by temperature. Increasing the incubation temperature of extracted chloroplasts to 30°C accelerated the rate of Y(II) decline, demonstrating a similar loss of photosynthetic function within 8 h that is typically seen in 72 h at RT (Figure 1E), with the differences sharply highlighted at 24 h (Figure 1F), and we reasoned this could serve as a useful assay for measuring chloroplast function moving forward.
2.2 Computational analysis of C. Reinhardtii identifies metabolites essential to chloroplast function
Encouraged by our initial results, we sought to identify other conditions that could enhance metabolic robustness of chloroplasts or extend functional lifetimes when decoupled from their host cells to develop a chloroplast “growth media”. We performed computational system-level examination of chloroplasts using flux balance analysis (FBA) on a published genome-scale model (GEM) of algal chloroplasts from three algal species (Boyle and Morgan, 2009). GEMs use an organism’s annotated genome to generate a mathematical reconstruction of their metabolic networks in a stoichiometric matrix that can be probed to identify characteristics such as important pathways for growth, robustness to genetic and environmental perturbations, and tradeoffs between different system objectives (Bi et al., 2022). While the published GEM contained reactions from multiple algal species, we used only the reactions related to C. reinhardtii for our analyses, which contained 774 genes covering 764 metabolites and 788 total reactions.
To identify essential reactions, we performed systematic in silico single reaction knockouts of each metabolic reaction in the model, specifically focusing on identifying sets of reactions that are essential for growth or ATP generation in the chloroplast (Figure 2A). The process for system-level in silico knockout of reactions has been detailed as part of robustness analysis of a model’s metabolic network (Becker et al., 2007). In short, the process involves systematically setting the flux for each reaction in the model to a minimal threshold value and then using FBA to assess if the system can achieve a minimal value for the biological objective that is being analyzed. For our analyses we set the minimal threshold to zero production of chloroplast biomass. Our initial results (250 essential reactions, including the chloroplast biomass reaction) included both internal chloroplast biochemical reactions as well as transport processes that facilitate import of nutrients and export of waste material (Supplementary Table S1). To generate a list of essential C. reinhartii cytosolic metabolites that must be imported for operation of its chloroplast, we excluded the list of essential internal biochemical reactions and focused only on the essential transport reactions. Specifically, we looked at import, reasoning that any nutrients requiring import to chloroplasts from the host cell would also be required in a defined medium to support chloroplast maintenance and operation and obtained a list of imported metabolites.
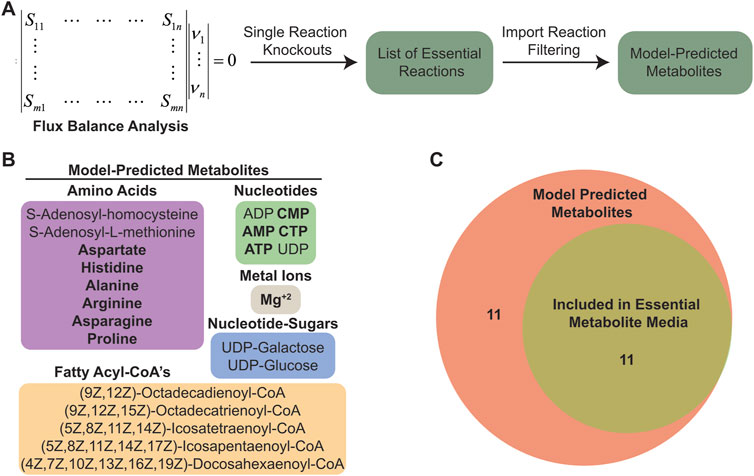
Figure 2. Flux balance analysis predicted a set of essential imported metabolites. (A) Schematic outlining the computational pipeline used to determine essential imported metabolites in C. reinhardtii chloroplasts. (B) List of essential imported metabolites predicted from C. reinhardtii chloroplast model. (C) Venn diagram showing number of metabolites included in EM media.
Among the model-predicted metabolites are various amino acids, fatty acid chains, nucleotides, nucleotide-sugars, cofactors, and critical biosynthesis intermediates (Figure 2B). In general, these compounds are necessary for DNA replication and protein production (amino acids, nucleotides, and Mg+2 ions), maintaining chloroplast membranes (Boudiere et al., 2014; Li-Beisson et al., 2015) (fatty acyl-CoA’s and nucleotide sugars), and generating defensive molecules such as antioxidants that are needed to negate the deleterious effects of ROS produced by photosynthesis (Hasanuzzaman et al., 2020) (adenylated amino acids). Since it is known that photosynthesis is an inherently damaging process resulting in high protein turnover (Sundby et al., 1993b), we focused on the metabolites involved in DNA and protein synthesis, and excluded the components more related to supporting membrane integrity as we assumed the demand for chloroplast membrane lipids would be substantially lower in non-dividing chloroplasts. From the model-predicted metabolite list, we chose 11 metabolites to include in an “essential metabolite chloroplast media” (EM) (Figure 2C; Supplementary Table S2).
2.3 Computationally identified metabolites prolong chloroplast photosynthetic function over time after extraction
To determine whether the identified metabolites could augment extracted chloroplast function, we incubated chloroplasts at 30°C either in HEPES buffer or EM media and took PAM readings over time (Figure 3A). While we saw an encouraging trend towards chloroplasts maintaining a higher photosynthetic yield (Y(II)) in the EM media, it was not significant (p = 0.2894). Closer examination of the EM media revealed a prevalence of amino acids, nucleotides, and importantly magnesium ions, which are essential for transcription and translation. Due to the high demand active photosynthesis places on chloroplasts, we reasoned that any media capable of supporting chloroplast function must also provide the raw materials needed to replace damaged proteins. Thus, we decided to use the PANOx-SP reaction buffer common to transcription/translation cell-free protein synthesis (CFPS) systems (Jewett and Swartz, 2004), which support those processes in vitro, as an “enhanced essential metabolite” (EEM) media (Supplementary Table S2). EEM media encompassed nearly all EM media components and provided additional amino acids, nucleotides, cofactors, salts, and energy rich components (Figure 3B). Incubation in EEM media showed a significantly slower decline in photosynthetic function over time compared to incubation in HEPES buffer (Figure 3C). To determine whether there were any morphological changes happening concurrently with photosynthetic decline, we imaged extracted chloroplasts on a confocal microscope after incubation at 30°C in HEPES buffer, EM, or EEM media. We categorized chloroplast morphology based on their shape and membrane appearance as either “intact”, “intermediate”, or “degraded” (Figure 3D). We were able to distinguish all three categories consistently between the different buffer conditions and quantified the population percentage of each category at 0 h and 8 h of incubation. The percentages between each group were consistent at the initial timepoint, however we saw differences emerge at 8 h (Figure 3E). Although both EM and EEM media had similar amounts of intact chloroplasts at 8 h, both groups performed better than HEPES buffer. Interestingly, we counted less degraded chloroplasts in EEM media compared to EM media (p = 0.0152) and HEPES buffer (p = 0.0026) at 8 h.
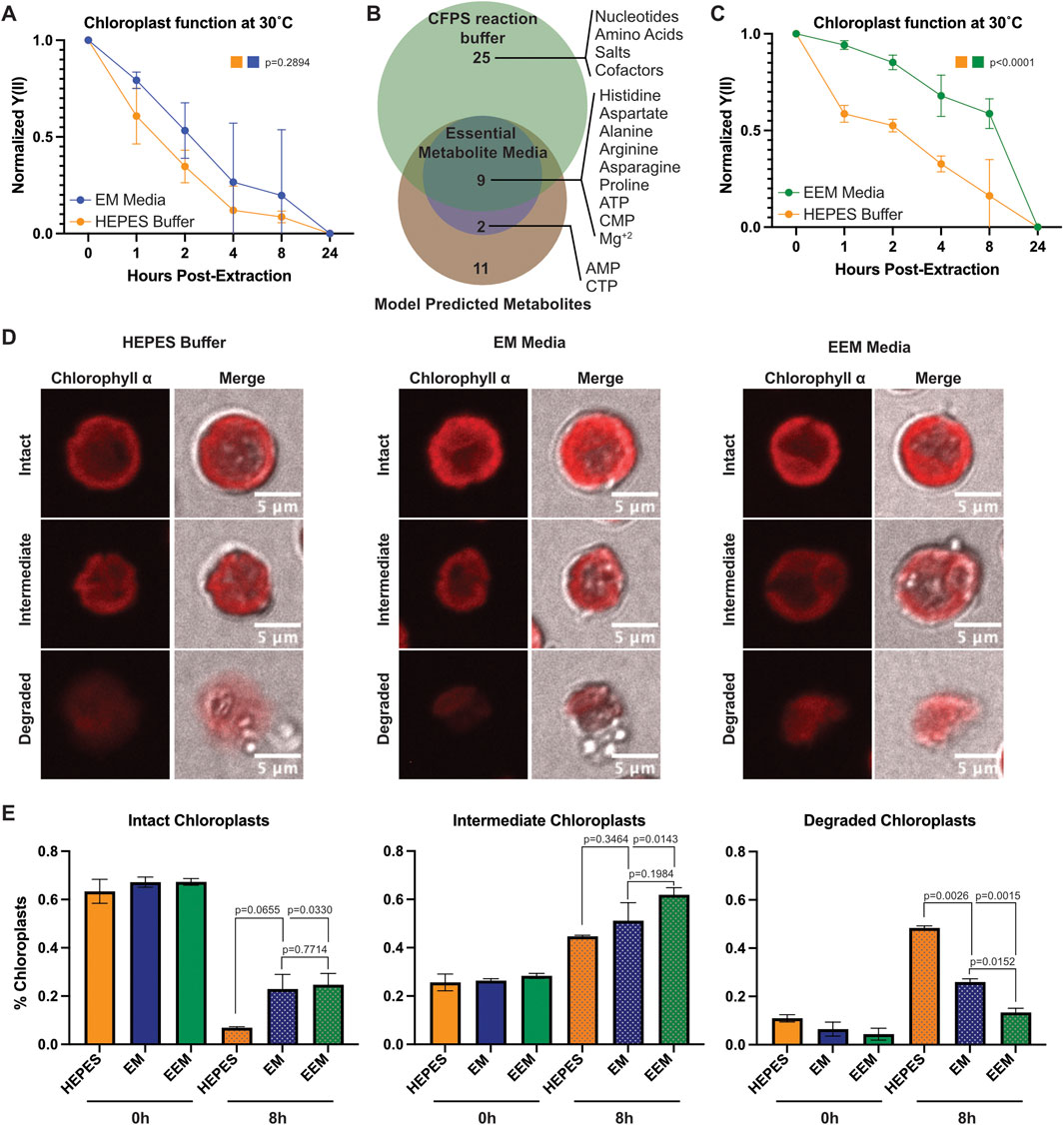
Figure 3. Metabolites supporting transcription and translation prolong extracted chloroplast photosynthetic function over time. (A) Line graph showing normalized Y(II) values over time from extracted chloroplasts at 30°C in HEPES buffer and EM media. N = 3 experiments. P-values calculated using repeated measures 2-way ANOVA. (B) Venn diagram showing overlap between predicted metabolites, EM media, and CFPS reaction buffer components. (C) Line graph showing normalized Y(II) values over time from extracted chloroplasts at 30°C in HEPES buffer and EEM media. N = 4 experiments. P-values calculated using repeated measures 2-way ANOVA. (D) Confocal micrographs showing representative intact, intermediate, and degraded chloroplasts in HEPES, EM media, and EEM media. (E) Bar graphs showing quantification of intact, intermediate, and degraded chloroplasts after incubation at 30°C for 0 h or 8 h. N = 2 experiments; 4 fields of view per group per experiment. P-values calculated by Student’s t-test.
3 Discussion
The world population is expected to grow beyond nine billion by 2050 (United Nations Department of Economic and Social Affairs and Population Division, 2024), increasing demands on food production and energy infrastructure. Improvements to existing crop yields and alternative energy sources utilizing bioenergy are prospective solutions to these challenges. Central to both processes are chloroplasts, which are mature plastids found in plants and algae that perform carbon fixation through photosynthesis, among other metabolic processes. Chloroplasts have become increasingly appreciated for their potential as biotechnology workhorses, proving instrumental in producing biomolecules for therapeutics (Specht et al., 2010) and lipids used in biofuel production (Nawkarkar et al., 2020; Cecchin et al., 2020). However, traditional methods of studying and manipulating chloroplasts within cells rely on labor-intensive techniques, often requiring many generations to achieve homoplasy, significantly slowing down research progress and hindering rapid experimental cycles.
Our study sought to approach this issue from a different angle and take the initial steps towards developing an ex vivo chloroplast platform. Isolated chloroplasts provide a defined system that minimizes cellular complexity, yet their rapid functional decline post-extraction remains a major challenge. We took inspiration from Sacoglassan sea slug kleptoplasty, which can maintain photosynthetically active chloroplasts from their algal sources for months after consumption (Cruz and Cartaxana, 2022). In fact, many species of unicellular organisms have displayed the ability to maintain active chloroplasts in completely non-native contexts (reviewed in (Miyagishima, 2023)), and we hypothesized that artificially defined environments could also prolong ex vivo chloroplast function.
Here, we successfully isolated chloroplasts from C. reinhardtii and identified a set of metabolites that could slow the decline of photosynthetic function after extraction. We used a C. reinhardtii chloroplast metabolic model and performed flux balance analysis (FBA) across the known metabolic reactions present in the model. Modeling single-reaction knockouts and optimizing for ATP production and biomass accumulation in the chloroplast, we identified a list of metabolic reactions and essential metabolites imported into chloroplasts and developed our initial media recipe. While our initial media (EM) did not significantly maintain chloroplast function at 30°C, we were encouraged by the findings. We noticed an abundance of transcription and translation related components in EM media, which led us to infer several key points: 1) These components are crucial since photosynthesis itself damages the photosynthetic machinery within chloroplasts (Aro et al., 1993; Tyystjarvi and Aro, 1996; Russell et al., 1995); 2) supporting protein synthesis and turnover is critical for maintaining function; and 3) CFPS reaction buffers could satisfy this requirement. CFPS enables protein production using cell extracts, and typical E. coli PANOx-SP (Jewett and Swartz, 2004) CFPS buffers contain most of the components in EM media, along with additional amino acids, nucleotides, salts, and cofactors. When we incubated extracted chloroplasts in EEM media at 30°C over time, we observed significant delays in chloroplast functional decline through PAM measurements. Together with our morphological analyses, our data showed that chloroplasts in EEM media were less degraded and maintained better membrane integrity, suggesting that EEM media better supported their structure and function over time.
However, like any computational approach, our results depend on the assumptions and accuracy of the underlying metabolic model. The model we used assumes an intact cell, with defined metabolic reactions in both cytosolic and chloroplast compartments. While the chloroplast genome encodes the most critical components of the photosynthetic machinery, most metabolic enzymes and proteins inside the chloroplast are nucleus encoded (Gallaher et al., 2018). Isolation of chloroplasts from the host would preclude protein resupply and ensure a limited functional lifetime tied to their rates of degradation. Isolated chloroplasts are translation competent (Leu et al., 1984; Mendiola-Morgenthaler et al., 1985) and could likely replace essential photosystem proteins such as D1 for a short duration, but indefinite sustained function ex vivo would ultimately require supplementation of nuclear-encoded factors. Nevertheless, we observed a measurable difference in photosynthetic function with EEM media over time at 30°C compared to HEPES buffer alone, suggesting that a purely biochemical formulation could still temporarily maintain function.
Our analysis identified several additional components absent in both the EM and EEM media that could possibly improve future media formulations, including adenosyl-containing amino acids, UDP-linked sugars, and fatty acid cofactors. S-adenosyl-homocysteine (AdoCys) can be converted into S-adenosyl-L-methionine (AdoMet), which serves as a universal methyl donor for methylation reactions and is a precursor for polyamine synthesis, which are critical for cell growth, division, and lipid accumulation (Tehlivets et al., 2013; Chiang et al., 1996). Although AdoCys and AdoMet are absent in the media, downstream polyamines like putrescine and spermidine are present in the EEM media, potentially compensating for this absence. External polyamines, such as putrescsine and spermidine, are known to influence C. Reinhardtii growth and may be taken up in limited amounts (Theiss et al., 2002; Freudenberg et al., 2022), though whether extracted chloroplasts retain these uptake mechanisms remains unexplored.
UDP-glucose, UDP-galactose, and the long- and very long-chain fatty acyl CoA’s we identified likely play important roles in lipid production, membrane maintenance, and fatty acid metabolism (Warakanont et al., 2019; Yang et al., 2015). UDP-glucose and UDP-galactose are interconvertible, with UDP-galactose serving as a key component of two photosynthetic membrane galactolipids: Monogalactosyldiacylglycerol (MGDG) and digalactosyldiacylglycerol (DGDG) (Boudiere et al., 2014; Li-Beisson et al., 2013). These lipids are highly conserved across photosynthetic organisms and can be produced entirely within chloroplasts via the “prokaryotic pathway” or in cooperation with the endoplasmic reticulum (ER) through the “eukaryotic pathway” which involves lipid trafficking between the chloroplast and ER. Both MGDG and DGDG serve critical structural and functional roles in thylakoid membranes and their associated photosystem complexes (Wang et al., 2014). Although extracted chloroplasts in EEM media maintained higher photosynthetic yield than the other conditions, we still observed a noticeable increase in the amount of degraded or membrane-damaged chloroplasts by 8 h and a sharp drop-off in photosynthetic yield by 24 h at 30°C. Although we did not explore the role of these lipid components, these findings suggest that membrane integrity may be involved in ex vivo chloroplast longevity, and we speculate that supplementation of lipid precursors could further prolong chloroplast function.
In this study, incubation of extracted chloroplasts in EEM media significantly delayed the decline of photosynthetic function compared to HEPES buffer and EM media. While the exact mechanism by which EEM media prolongs chloroplast function is unknown, we speculate the included components provide the necessary support for transcription, translation, and protein turnover within the chloroplast. Identifying media formulations that can sustain chloroplast functionality ex vivo for even brief periods could facilitate their direct manipulation and create a more efficient research and biomanufacturing (Miller et al., 2020) platform. Refining the media formulation by incorporating additional metabolites, such as fatty acid cofactors and UDP-linked sugars, and/or combining it with genetic modifications or direct protein supplementation may further extend chloroplast functional lifetimes and potentially enable faster design-build-test-learn cycles for chloroplast research and biotechnological applications. Additionally, advancements in extension of chloroplast functional lifetimes outside of their host cells may support efforts in constructing synthetic organelle-containing cells from the bottom up.
4 Materials and methods
4.1 Strains and growth conditions
Chlamydomonas reinhardtii strain CC400 cw15 mt+ was acquired from the Chlamydomonas Resource Center. C. reinhardtii was cultured in 250 mL flasks using high salt with acetate (HSA) media supplemented with Hutner’s trace elements (Chlamydomonas Resource Center) under 12 h:12 h light/dark cycles. Cultures were expanded to 500 mL bottles for chloroplast extractions under the same growth conditions and harvested after 4–5 days.
4.2 Chloroplast extraction
Chloroplasts were extracted from C. Reinhardtii cells according to Mason et al. (2006) with slight modifications. Briefly, 5 × 106−1 × 107 cells were harvested from a 500 mL culture by centrifugation at 4000 g for 10 min at 4°C, washed with 10 mL HEPES buffer (50 mM HEPES-KOH pH 7.5), pelleted, and resuspended in 2 mL HEPES buffer. The algae were diluted by adding 8–10 mL of isolation buffer (50 mM HEPES-KOH pH 7.5, 2 mM EDTA, 1 mM MgCl2, 300 mM Sorbitol, and 1% BSA), and lysed by a single pass through a 27-gauge needle. Lysed algae were centrifuged at 750 g for 2 min at 4°C and gently resuspended in 2 mL of isolation buffer using a paintbrush before layering on top of a pre-prepared Percoll/Ficoll gradient without isoascorbate. Gradients were centrifuged at 3265 g for 15 min at 4°C and the band at the 45%–65% interface was collected as intact chloroplasts before dilution with 50 mM HEPES-KOH pH 7.5 and used for downstream assays.
4.3 Measurement of photosynthetic efficiency (MINI PAM-II)
The photochemical activity of Photosystem II (PSII) was measured using a pulse amplitude modulated (PAM) fluorometer (MINI-PAM-II, Heinz-Walz, Germany). Chloroplast concentrations were determined via hemocytometer and aliquoted into sterile Eppendorf tubes at a concentration of 1 × 106 chloroplasts/mL. Chloroplasts were pelleted at 600 g for 3 min at room temperature and resuspended in equal volumes of HEPES buffer, EM, or EEM media to maintain consistent concentrations across samples. The centrifugation and resuspension processes were repeated to eliminate residual extraction and storage buffers. Resuspended samples were incubated at pre-determined temperatures (4°C, 25°C, and 30°C) and protected from light with foil. Time “0” chloroplast measurements were taken immediately upon resuspension into designated buffer post-extraction. 200 μL aliquots of 1 × 106 chloroplasts/mL stock solution were pipetted into a MINI PAM-II chamber and read per manufacturer instructions. N = 3 experiments and n = 4 experiments were performed to compare HEPES buffer to EM media and HEPES buffer to EEM media, respectively. Repeated measures two-way ANOVA with Geisser-Greenhouse correction was used to determine statistical significance of changes in Y(II) values over time.
4.4 Microscopy and image analysis
Extracted chloroplasts were pelleted and gently resuspended in HEPES buffer, EM, or EEM media at a concentration of 1 × 106 chloroplasts/mL. 15 μL of the suspension was plated onto a No. 1.5 18-well glass slide (Ibidi #81821) and allowed to settle for 30 min. Differential interference contrast (DIC) and fluorescence (415 nm ex/640 nm em) images were captured using a 60x objective on a Nikon inverted confocal microscope. Morphology was classified into three categories: Intact (fully intact membrane with minimal degradation), intermediate (some visible membrane perforation), and degraded (no intact membrane). For each timepoint (n = 2 experiments per timepoint), 4 images containing 250–500 chloroplasts each were analyzed using ImageJ (ImageJ2 2.14.0/1.54f) and classifications were averaged across the images. Statistical analysis was performed using Student’s t-test to assess significance.
4.5 Flux balance analysis
Flux balance analysis (FBA) is a constraint-based reconstruction and analysis approach that uses a genome-scale metabolic reconstruction as its basis. The elementary functional information derived from annotated genomes is used with available knowledge of enzymology to reconstruct all metabolic pathways in an organism. Metabolism is represented mathematically as a stoichiometric matrix, S(m×n), where m is the number of metabolites and n the number of different reactions. By assuming mass balance and the system is in metabolic steady-state, the following set of linear equations govern the system’s behavior:
where Ci is the concentration of metabolite i. Other limitations are imposed on the system based on experimental studies, such as bounding the flux through a reaction, as well as constraints on the import of nutrients and export of waste products. These constraints are formulated as:
where bi and νj are the export/import flux of metabolite species i, and the flux through internal reaction j respectively. α, β, χ, and φ are the lower and upper limits for these fluxes. Finally, FBA employs linear programming to determine a feasible steady-state flux vector that optimizes an objective function, often chosen to be the production of biomass, i.e., cellular growth. For our analyses, FBA was used with a published system-scale model of algal chloroplasts (Bjerkelund Røkke et al., 2020) to analyze single gene and reaction knockout phenotypes in the model. While we only used the C. reinhardtii-related reactions in the model, since the developers of the system-scale model used Nannochloropsis gaditana as a base for all models, any reaction in the C. reinhardtii model that is also present in N. gaditana has a “Nano” designation.
For the single reaction knockouts, the activity of each reaction in the model is blocked and the model is tested for its ability to grow (i.e., produce biomass). When blocking the activity of a reaction leads to cessation of growth, that reaction is labeled an essential reaction for the analyzed environment. In case of single gene knockout, for inactivation of each gene, the reactions that are catalyzed by the product of translation of that protein are blocked and if this results in cessation of growth, the gene is deemed essential.
For our analysis identifying essential media nutrients, the list of essential transport reactions, particularly import reactions were used to predict an essential medium to support operation of isolated C. reinhardtii chloroplasts. The transport reactions in genome-scale models of metabolism typically are included in the models based on annotation of membrane surface proteins as well as known auxotrophies of a system. In some cases, when a biosynthetic pathway for a known component of biomass is missing in the metabolic reconstruction, the transport reactions are “gap-filled” into the models to ensure its ability to produce biomass.
Data availability statement
The original contributions presented in the study are included in the article/Supplementary Material, further inquiries can be directed to the corresponding author.
Author contributions
MM: Formal Analysis, Investigation, Methodology, Validation, Visualization, Writing–original draft, Writing–review and editing. AN: Data curation, Formal Analysis, Investigation, Methodology, Visualization, Writing–original draft, Writing–review and editing, Supervision. TM: Data curation, Formal Analysis, Methodology, Writing–review and editing, Investigation. CY: Methodology, Writing–review and editing, Supervision. MC: Resources, Supervision, Writing–review and editing, Methodology. SH-P: Conceptualization, Formal Analysis, Funding acquisition, Investigation, Methodology, Supervision, Visualization, Writing–original draft, Writing–review and editing.
Funding
The author(s) declare that financial support was received for the research and/or publication of this article. This research was supported by the Department of Energy Laboratory Directed Research and Development (LDRD) program at Lawrence Livermore National Laboratory, 23-LW-059.
Acknowledgments
Work at the Lawrence Livermore National Laboratory was performed under the auspices of the U.S. Department of Energy under Contract DE-AC52-07NA27344.
Conflict of interest
The authors declare that the research was conducted in the absence of any commercial or financial relationships that could be construed as a potential conflict of interest.
Generative AI statement
The authors declare that no Generative AI was used in the creation of this manuscript.
Publisher’s note
All claims expressed in this article are solely those of the authors and do not necessarily represent those of their affiliated organizations, or those of the publisher, the editors and the reviewers. Any product that may be evaluated in this article, or claim that may be made by its manufacturer, is not guaranteed or endorsed by the publisher.
Supplementary material
The Supplementary Material for this article can be found online at: https://www.frontiersin.org/articles/10.3389/fbioe.2025.1560200/full#supplementary-material
References
Aro, E. M., Virgin, I., and Andersson, B. (1993). Photoinhibition of Photosystem II. Inactivation, protein damage and turnover. Biochim. Biophys. Acta 1143, 113–134. doi:10.1016/0005-2728(93)90134-2
Becker, S. A., Feist, A. M., Mo, M. L., Hannum, G., Palsson, B. Ø., and Herrgard, M. J. (2007). Quantitative prediction of cellular metabolism with constraint-based models: the COBRA Toolbox. Nat. Protoc. 2, 727–738. doi:10.1038/nprot.2007.99
Bi, X., Liu, Y., Li, J., Du, G., Lv, X., and Liu, L. (2022). Construction of multiscale genome-scale metabolic models: frameworks and challenges. Biomolecules 12, 721. doi:10.3390/biom12050721
Bjerkelund Rokke, G., Hohmann-Marriott, M. F., and Almaas, E. (2020). An adjustable algal chloroplast plug-and-play model for genome-scale metabolic models. PLoS One 15, e0229408. doi:10.1371/journal.pone.0229408
Bjerkelund Røkke, G., Hohmann-Marriott, M. F., and Almaas, E. (2020). An adjustable algal chloroplast plug-and-play model for genome-scale metabolic models. Plos one 15, e0229408. doi:10.1371/journal.pone.0229408
Bock, R. (2014). Engineering chloroplasts for high-level foreign protein expression. Methods Mol. Biol. 1132, 93–106. doi:10.1007/978-1-62703-995-6_5
Boudiere, L., Michaud, M., Petroutsos, D., Rébeillé, F., Falconet, D., Bastien, O., et al. (2014). Glycerolipids in photosynthesis: composition, synthesis and trafficking. Biochim. Biophys. Acta 1837, 470–480. doi:10.1016/j.bbabio.2013.09.007
Boyle, N. R., and Morgan, J. A. (2009). Flux balance analysis of primary metabolism in Chlamydomonas reinhardtii. BMC Syst. Biol. 3, 4. doi:10.1186/1752-0509-3-4
Cecchin, M., Berteotti, S., Paltrinieri, S., Vigliante, I., Iadarola, B., Giovannone, B., et al. (2020). Improved lipid productivity in Nannochloropsis gaditana in nitrogen-replete conditions by selection of pale green mutants. Biotechnol. Biofuels 13, 78. doi:10.1186/s13068-020-01718-8
Chen, Y., Zhou, B., Li, J., Tang, H., Tang, J., and Yang, Z. (2018). Formation and change of chloroplast-located plant metabolites in response to light conditions. Int. J. Mol. Sci. 19, 654. doi:10.3390/ijms19030654
Chiang, P. K., Gordon, R. K., Tal, J., Zeng, G. C., Doctor, B. P., Pardhasaradhi, K., et al. (1996). S-Adenosylmetliionine and methylation. FASEB J. 10, 471–480. doi:10.1096/fasebj.10.4.8647346
Cruz, S., and Cartaxana, P. (2022). Kleptoplasty: getting away with stolen chloroplasts. PLoS Biol. 20, e3001857. doi:10.1371/journal.pbio.3001857
Freudenberg, R. A., Wittemeier, L., Einhaus, A., Baier, T., and Kruse, O. (2022). Advanced pathway engineering for phototrophic putrescine production. Plant Biotechnol. J. 20, 1968–1982. doi:10.1111/pbi.13879
Gallaher, S. D., Fitz-Gibbon, S. T., Strenkert, D., Purvine, S. O., Pellegrini, M., and Merchant, S. S. (2018). High-throughput sequencing of the chloroplast and mitochondrion of Chlamydomonas reinhardtii to generate improved de novo assemblies, analyze expression patterns and transcript speciation, and evaluate diversity among laboratory strains and wild isolates. Plant J. 93, 545–565. doi:10.1111/tpj.13788
Galmes, J., Conesa, M., Díaz-Espejo, A., Mir, A., Perdomo, J., Niinemets, Ü., et al. (2014). Rubisco catalytic properties optimized for present and future climatic conditions. Plant Sci. 226, 61–70. doi:10.1016/j.plantsci.2014.01.008
Gedi, M. A., Briars, R., Yuseli, F., Zainol, N., Darwish, R., Salter, A. M., et al. (2017). Component analysis of nutritionally rich chloroplasts: recovery from conventional and unconventional green plant species. J. Food Sci. Technol. 54, 2746–2757. doi:10.1007/s13197-017-2711-8
Georgianna, D. R., and Mayfield, S. P. (2012). Exploiting diversity and synthetic biology for the production of algal biofuels. Nature 488, 329–335. doi:10.1038/nature11479
Green, B. J., Fox, T. C., and Rumpho, M. E. (2005). Stability of isolated algal chloroplasts that participate in a unique molllusc/kleptoplast association. Symbiosis 40, 31–40.
Hasanuzzaman, M., Bhuyan, M., Zulfiqar, F., Raza, A., Mohsin, S., Mahmud, J., et al. (2020). Reactive oxygen species and antioxidant defense in plants under abiotic stress: revisiting the crucial role of a universal defense regulator. Antioxidants (Basel) 9, 681. doi:10.3390/antiox9080681
Jewett, M. C., and Swartz, J. R. (2004). Mimicking the Escherichia coli cytoplasmic environment activates long-lived and efficient cell-free protein synthesis. Biotechnol. Bioeng. 86, 19–26. doi:10.1002/bit.20026
Khan, M. O., Mehmood, M. A., Mukhtar, Z., and Ahmad, N. (2018). Chloroplasts as cellular factories for the cost-effective production of cellulases. Protein Pept. Lett. 25, 129–135. doi:10.2174/0929866525666180122115210
Kunst, L., Browse, J., and Somerville, C. (1988). Altered regulation of lipid biosynthesis in a mutant of Arabidopsis deficient in chloroplast glycerol-3-phosphate acyltransferase activity. Proc. Natl. Acad. Sci. U. S. A. 85, 4143–4147. doi:10.1073/pnas.85.12.4143
Leu, S., Mendiola-Morgenthaler, L., and Boschetti, A. (1984). Protein synthesis by isolated chloroplasts of Chlamydomonas reinhardii. FEBS Lett. 166, 23–27. doi:10.1016/0014-5793(84)80037-x
Li-Beisson, Y., Beisson, F., and Riekhof, W. (2015). Metabolism of acyl-lipids in Chlamydomonas reinhardtii. Plant J. 82, 504–522. doi:10.1111/tpj.12787
Li-Beisson, Y., Shorrosh, B., Beisson, F., Andersson, M. X., Arondel, V., Bates, P. D., et al. (2013). Acyl-lipid metabolism. Arab. Book 11, e0161. doi:10.1199/tab.0161
Maliga, P., and Bock, R. (2011). Plastid biotechnology: food, fuel, and medicine for the 21st century. Plant Physiol. 155, 1501–1510. doi:10.1104/pp.110.170969
Mason, C. B., Bricker, T. M., and Moroney, J. V. (2006). A rapid method for chloroplast isolation from the green alga Chlamydomonas reinhardtii. Nat. Protoc. 1, 2227–2230. doi:10.1038/nprot.2006.348
Mendiola-Morgenthaler, L., Leu, S., and Boschetti, A. (1985). Isolation of biochemically active chloroplasts from Chlamydomonas. Plant Sci. 38, 33–39. doi:10.1016/0168-9452(85)90076-7
Miller, T. E., Beneyton, T., Schwander, T., Diehl, C., Girault, M., McLean, R., et al. (2020). Light-powered CO2 fixation in a chloroplast mimic with natural and synthetic parts. Science 368, 649–654. doi:10.1126/science.aaz6802
Miyagishima, S. Y. (2023). Taming the perils of photosynthesis by eukaryotes: constraints on endosymbiotic evolution in aquatic ecosystems. Commun. Biol. 6, 1150. doi:10.1038/s42003-023-05544-0
Nawkarkar, P., Chugh, S., Sharma, S., Jain, M., Kajla, S., and Kumar, S. (2020). Characterization of the chloroplast genome facilitated the transformation of parachlorella kessleri-I, A potential marine alga for biofuel production. Curr. Genomics 21, 610–623. doi:10.2174/1389202921999201102164754
Reyes-Prieto, A., and Moustafa, A. (2012). Plastid-localized amino acid biosynthetic pathways of Plantae are predominantly composed of non-cyanobacterial enzymes. Sci. Rep. 2, 955. doi:10.1038/srep00955
Russell, A. W., Critchley, C., Robinson, S. A., Franklin, L. A., Seaton, G., Chow, W. S., et al. (1995). Photosystem II regulation and dynamics of the chloroplast D1 protein in arabidopsis leaves during photosynthesis and photoinhibition. Plant Physiol. 107, 943–952. doi:10.1104/pp.107.3.943
Specht, E., Miyake-Stoner, S., and Mayfield, S. (2010). Micro-algae come of age as a platform for recombinant protein production. Biotechnol. Lett. 32, 1373–1383. doi:10.1007/s10529-010-0326-5
Sundby, C., McCaffery, S., and Anderson, J. M. (1993a). Turnover of the photosystem II D1 protein in higher plants under photoinhibitory and nonphotoinhibitory irradiance. J. Biol. Chem. 268, 25476–25482. doi:10.1016/s0021-9258(19)74416-0
Sundby, C., McCaffery, S., and Anderson, J. M. (1993b). Turnover of the photosystem II D1 protein in higher plants under photoinhibitory and nonphotoinhibitory irradiance. J. Biol. Chem. 268, 25476–25482. doi:10.1016/s0021-9258(19)74416-0
Tehlivets, O., Malanovic, N., Visram, M., Pavkov-Keller, T., and Keller, W. (2013). S-adenosyl-L-homocysteine hydrolase and methylation disorders: yeast as a model system. Biochim. Biophys. Acta 1832, 204–215. doi:10.1016/j.bbadis.2012.09.007
Theiss, C., Bohley, P., and Voigt, J. (2002). Regulation by polyamines of ornithine decarboxylase activity and cell division in the unicellular green alga Chlamydomonas reinhardtii. Plant Physiol. 128, 1470–1479. doi:10.1104/pp.010896
Tyystjarvi, E., and Aro, E. M. (1996). The rate constant of photoinhibition, measured in lincomycin-treated leaves, is directly proportional to light intensity. Proc. Natl. Acad. Sci. U. S. A. 93, 2213–2218. doi:10.1073/pnas.93.5.2213
United Nations Department of Economic and Social Affairs, Population Division (2024). World population prospects 2024: summary of results. UN DESA/POP/2024.
Wang, L., Patena, W., Van Baalen, K. A., Xie, Y., Singer, E. R., Gavrilenko, S., et al. (2023). A chloroplast protein atlas reveals punctate structures and spatial organization of biosynthetic pathways. Cell 186, 3499–3518.e14. doi:10.1016/j.cell.2023.06.008
Wang, S., Uddin, M. I., Tanaka, K., Yin, L., Shi, Z., Qi, Y., et al. (2014). Maintenance of chloroplast structure and function by overexpression of the rice Monogalactosyldiacylglycerol synthase gene leads to enhanced salt tolerance in tobacco. Plant Physiol. 165, 1144–1155. doi:10.1104/pp.114.238899
Warakanont, J., Li-Beisson, Y., and Benning, C. (2019). LIP4 is involved in triacylglycerol degradation in Chlamydomonas reinhardtii. Plant Cell Physiol. 60, 1250–1259. doi:10.1093/pcp/pcz037
Keywords: chloroplast, Chlamydomonas reinhardtii, metabolic modeling, photosynthesis, flux balance analysis (FBA), media formulation
Citation: Mohagheghi M, Navid A, Mossington T, Ye C, Coleman MA and Hoang-Phou S (2025) Developing a media formulation to sustain ex vivo chloroplast function. Front. Bioeng. Biotechnol. 13:1560200. doi: 10.3389/fbioe.2025.1560200
Received: 14 January 2025; Accepted: 19 March 2025;
Published: 09 April 2025.
Edited by:
Shailendra Pratap Singh, Banaras Hindu University, IndiaReviewed by:
Rajneesh Singhal, Michigan State University, United StatesShaobo Yang, Dana–Farber Cancer Institute, United States
Copyright © 2025 Mohagheghi, Navid, Mossington, Ye, Coleman and Hoang-Phou. This is an open-access article distributed under the terms of the Creative Commons Attribution License (CC BY). The use, distribution or reproduction in other forums is permitted, provided the original author(s) and the copyright owner(s) are credited and that the original publication in this journal is cited, in accordance with accepted academic practice. No use, distribution or reproduction is permitted which does not comply with these terms.
*Correspondence: Steven Hoang-Phou, aG9hbmdwaG91MUBsbG5sLmdvdg==