- 1Institute for Neurosciences of Montpellier, INSERM, University of Montpellier, Montpellier, France
- 2Department of Ophthalmology, Gui de Chauliac Hospital, Montpellier, France
- 3Sydney Medical School, The Save Sight Institute, The University of Sydney, Sydney, NSW, Australia
The cornea acts as the eye foremost protective layer and is essential for its focusing power. Corneal blindness may arise from physical trauma or conditions like dystrophies, keratitis, keratoconus, or ulceration. While conventional treatments involve medical therapies and donor allografts—sometimes supplemented with keratoprostheses—these options are not suitable for all corneal defects. Consequently, the development of bioartificial corneal tissue has emerged as a critical research area, aiming to address the global shortage of human cornea donors. Bioengineered corneas hold considerable promise as substitutes, with the potential to replace either specific layers or the entire thickness of damaged corneas. This review first delves into the structural anatomy of the human cornea, identifying key attributes necessary for successful corneal tissue bioengineering. It then examines various corneal pathologies, current treatments, and their limitations. Finally, the review outlines the primary approaches in corneal tissue engineering, exploring cell-free, cell-based, and scaffold-based options as three emerging strategies to address corneal failure.
1 Introduction
The cornea is a convex, transparent layer that covers the pupil, iris, and anterior chamber of the eye (Duke-Elder, 1958). Its primary role is to protect the underlying ocular structures, transmit light, and provide approximately two-thirds of the refractive power of the eye by focusing light onto the retina with minimal optical loss or scattering. Structurally, the cornea is composed of five distinct layers, and damage to any one of these can result in vision impairment (Maurice, 1957; Laibson, 1972).
Globally, over 10 million people are affected by corneal blindness due to various injuries or diseases. The most common treatment for vision restoration in such cases is corneal transplantation, or keratoplasty (KP), either partially or fully replacing the damaged cornea. However, KP faces significant challenges, including a shortage of donor corneas and the risk of immune rejection (Paton, 1954; Mannis and Krachmer, 1981; Aquavella, 2007). These challenges underscore the urgent need for innovative alternative solutions, such as bioengineered corneas, to replace native corneal tissue (Germain et al., 2000; Carlsson et al., 2003; Fagerholm et al., 2009).
2 Anatomy of the cornea
2.1 General description of the cornea
The adult human cornea, measuring approximately 550 µm in thickness, is divided into three main regions and has an oval shape, with vertical dimensions of 10–11 mm and horizontal dimensions of 11–12 mm (Ehlers et al., 1975; Doughty and Zaman, 2000; Piñero et al., 2008). Corneal thickness decreases with age and tapers from the periphery toward the center. The outermost layer is the epithelium, separated from the stroma by Bowman’s layer (BL), which is 8–12 µm thick. The middle section, composed of the stroma, accounts for the majority of the cornea’s thickness. Descemet’s membrane (DM) serves as the basement membrane between the stroma and the corneal endothelium (Figure 1) (Li et al., 1997; Hayashi et al., 2002; Reinstein et al., 2008). Each layer of the cornea features a distinct extracellular matrix (ECM) composition, with varying types of collagen fibers, as detailed in Table 1.
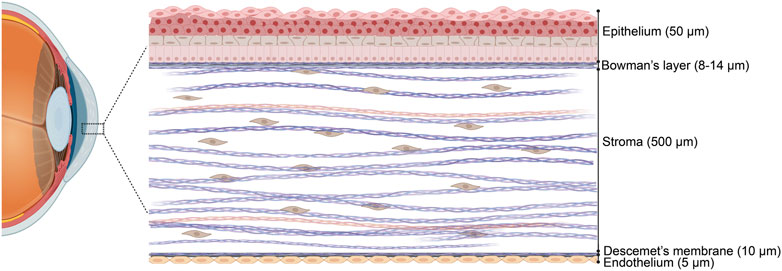
Figure 1. Schematic representation of a human cornea cross section in a hierarchical arrangement. Three cellular layers, namely, the epithelium, stroma, and endothelium, and two acellular basement membranes, namely, Bowman’s layer and Descemet’s membrane. Figure designed with Biorender (https://app.biorender.com).
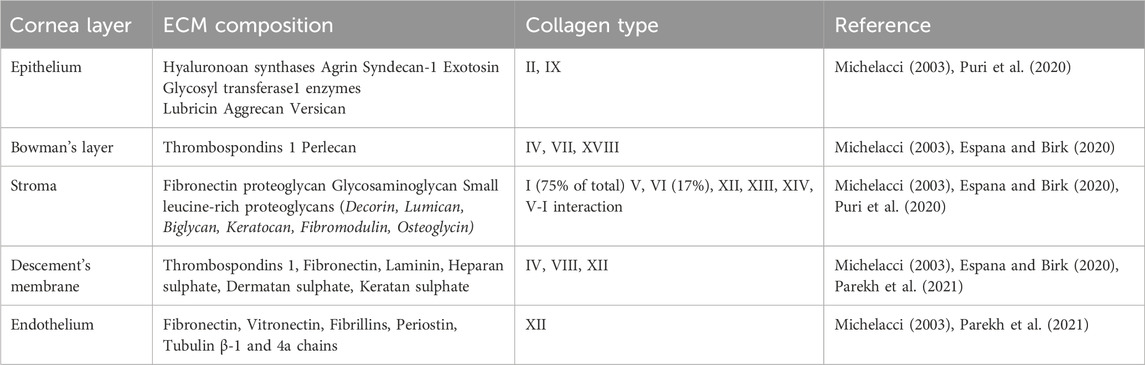
Table 1. Variations in ECM composition and collagen types across the different layers of the human adult cornea.
Even microscale deviations in the anterior corneal surface can result in significant changes to refractive power and visual function. The cornea’s light transmission efficiency ranges from 80% to 98% across wavelengths of 450–1,000 nm (Beems and Van Best, 1990). These high levels of light transmission in the visible spectrum (380–750 nm) are achieved despite the cornea’s dense collagen structure, a result of the highly organized packing and nanoscale architecture of the collagen fibers, which maintain transparency while allowing light to pass through (Maurice, 1957; Tseng et al., 2022).
Anatomically, the cornea can be divided into central and peripheral regions, each with unique structural and physiological characteristics. The central cornea is thinner but five times more innervated than the periphery (He et al., 2010; Bergmanson et al., 2019). From a mechanical perspective, the peripheral cornea is stiffer and displays a nonlinear stress-strain response, stiffening further under elevated strains. The elastic strength of the cornea, measured by Young’s modulus of elasticity, ranges from 82 to 530 kPa for the anterior cornea and from 28 to 162 kPa for the posterior stroma (Dias et al., 2013).
A thorough understanding of the architecture, cellular functions, and composition of each corneal layer is essential for successful tissue engineering or bioprinting efforts, providing a crucial foundation for experimental design and 3D modeling.
2.2 Corneal epithelium
The corneal epithelium originates from the surface ectoderm around the 5th to 6th week of embryonic development (Wulle and Richter, 1978). This outermost layer of the cornea, measuring 40–50 µm thick, is composed of 4–6 layers of nonsecretory stratified epithelial cells and is protected by a thin tear film that serves as a vital barrier against the external environment 20 three main types: basal cells forming the posterior layer, wing (or suprabasal) cells, and flat polygonal superficial cells with apical microvilli (Pellegrini et al., 1999; Delmonte and Kim, 2011; Lobo et al., 2016; Sridhar, 2018).
The maintenance of the corneal epithelium is explained by the X, Y, Z hypothesis proposed by Thoft and Friend, which describes a balance among three processes: basal epithelial cell proliferation (X), centripetal migration of peripheral cells (Y), and the loss of superficial epithelial cells (Z). This equilibrium ensures the consistent renewal of the corneal epithelium (Thoft and Friend, 1983).
The peripheral cells (Y) are known as limbal epithelial stem cell (LESCs), which reside exclusively in the limbal zone and are essential for the long-term maintenance and regeneration of the corneal epithelium after injury (Huang and Tseng, 1991). The heterogeneous limbal niche, located in the basal epithelial layer and within the palisades of Vogt, is rich in LESCs (Dua et al., 2005). LESCs maintain a steady cell population by proliferating and differentiating into transit amplifying cells (TACs), which then progressively migrate into the cornea to eventually differentiate into corneal epithelial cells (CECs) (Yoon et al., 2014).
2.3 Bowman’s layer
Bowman’s Layer (BL) is acellular and consists primarily of proteoglycans and randomly oriented collagen fibrils, predominantly type I, though types III, V, and XII are also present (Jacobsen et al., 1984; Marshall et al., 1991; Hayashi et al., 2002; Merindano et al., 2002). While several hypotheses exist regarding the function of BL, some remain controversial. For instance, Tong et al. proposed that BL plays a crucial mechanical role in maintaining corneal shape; however, this was challenged by studies indicating that BL is not essential for corneal stiffness (Tong et al., 2019; Torres-Netto et al., 2021).
Additionally, BL may serve as a barrier regulating the exchange of growth factors between the epithelium and stroma (Gordon et al., 1994; Mohan et al., 1997; Torricelli et al., 2015). This barrier function could have important implications for corneal homeostasis and wound healing, although further research is needed to fully understand these mechanisms.
2.4 Corneal stroma
The human corneal stroma forms the central core of the cornea, accounting for approximately 80%–85% of its total thickness. The unique structure and composition of the extracellular matrix (ECM) in the stroma are essential for maintaining light transmittance. Subtle variations in fibrillar diameter and spacing exist between the anterior and posterior stroma. In the anterior stroma, fibrils are more tightly packed, and the anterior and mid-stromal lamellae are heavily interwoven. In contrast, the posterior lamellae are less interwoven and become increasingly hydrated toward the central cornea (Meek and Knupp, 2015).
The transparency of the corneal stroma is largely attributed to the precise spatial arrangement of its narrow, densely packed collagen fibrils. The refractive index of these collagen fibrils closely matches that of the surrounding ground substance, resulting in minimal light scattering due to the small diameter of the fibrils—significantly smaller than the wavelength of visible light (Bron, 2001). This concept was initially proposed by Maurice, who demonstrated that the spatial organization of stromal collagen fibrils, oriented in specific directions, facilitates the forward propagation of secondary radiation. Known as the hexagonal crystalline lattice theory, this hypothesis emphasized the importance of fibril arrangement for corneal transparency (Maurice, 1957).
However, later studies questioned the existence of a long-range ordered crystalline lattice, instead describing the collagen fibril arrangement as a “short-range ordered” structure that enables optical interference effects (Farrell and Hart, 1969; Sayers et al., 1982; Worthington and Inouye, 1985). More recent research by Tseng et al. suggests that corneal transparency is influenced more by fibril diameter than by geometric arrangement into hexagonal lattices. According to their findings, the optical penetration of specific wavelengths is determined by the polydispersity of collagen fibrils rather than their spacing (Tseng et al., 2022).
The maintenance of this fibrillar arrangement is supported by collagen fibril-associated proteoglycans, which, along with matrix metalloproteinases and collagen, contribute to the cornea’s mechanical strength and transparency (Cheng and Pinsky, 2013). Type I collagen is the predominant collagen type in the corneal stroma, with smaller amounts of types V, VI, XII, XIII, XIV, and XXIV. The presence of type III collagen in the normal corneal stroma remains a subject of debate (Ihanamäki et al., 2004).
These collagen fibrils are heterotypic, comprising a single population jointly assembled from collagen types I and V. The surface of these fibrils is associated with leucine-rich proteoglycans and other fibril-associated collagens with interrupted triple helices, which help to link and integrate the fibrillar collagen with other components of the ECM (Chen et al., 2015).
While various models have offered different perspectives on corneal transparency, the prevailing framework focuses on a limited set of criteria that influence this property (Spadea et al., 2016): (i) the thickness of the corneal stroma, (ii) the density and diameter of collagen fibrils, (iii) the differential refractive index between fibrils and the surrounding matrix, and (iv) the spatial arrangement of the fibril array.
2.5 Descemet’s membrane
Descemet’s Membrane (DM) is a thin layer located at the posterior end of the cornea, primarily composed of laminins, nidogens, perlecan, and type IV collagen. It is divided into a non-banded layer adjacent to the stroma, approximately 0.3 µm thick, followed by an anterior striped zone that is 2–4 µm thick, and a posterior nebulous layer secreted by the neighboring endothelium (Johnson et al., 1982; Levy et al., 1995; Chaon et al., 2016; Price et al., 2021). As a basement membrane, DM is essential for anchoring the endothelium and facilitating the transfer of molecules and nutrients into the stroma. Additionally, DM plays a crucial role in maintaining corneal integrity by serving as an attachment site for corneal endothelial cells (Johnson et al., 1982; Levy et al., 1995; Saikia et al., 2018). Despite its supportive functions, DM has limited regenerative capacity in response to infections and corneal injuries (Marino et al., 2017).
Another recently identified layer, known as the “pre-DM” or “Dua’s layer,” has been reported in the posterior cornea. This well-defined, acellular layer is located just anterior to DM, separating at the level of the last row of stromal keratocytes. Dua’s layer consists of thin lamellae made up of tightly packed collagen bundles (Dua et al., 2013). Despite the initial controversy surrounding the discovery of the Dua’s layer, subsequent studies have provided evidence confirming its presence across different age groups, including pediatric patients. Although some aspects remain under investigation, the growing body of histological and clinical data has substantiated the anatomical relevance of the Dua’s layer in corneal research and surgical practice (Dua et al., 2023).
2.6 Corneal endothelium
The endothelium is a single, confluent layer of hexagonal, tightly packed squamous cells with discontinuous apical tight junctions, situated at the posterior of the cornea adjacent to DM (Bourne and Kaufman, 1976; Laing et al., 1976; Blatt et al., 1979). Its primary role is to regulate stromal hydration and facilitate the passage of nutrients from the aqueous humor into the cornea (Hedbys et al., 1963; Mishima, 1968; Mayes and Hodson, 1978).
3 Corneal pathologies and their current treatments
Corneal diseases rank as the fifth most common cause of blindness globally. As the outermost layer of the eye, the cornea is highly susceptible to various damaging factors, including mechanical, chemical, and thermal insults (Flaxman et al., 2017). According to a 2022 World Health Organization (WHO) report, 2.2 billion people worldwide experience vision impairment or blindness (www.who.int/news-room/fact-sheets/detail/blindness-and-visual-impairment). To improve transplantation outcomes, conventional full-thickness corneal transplants (penetrating keratoplasties) are increasingly being replaced by lamellar keratoplasties, where only specific layers of the cornea are transplanted, such as in deep anterior lamellar or endothelial keratoplasties (Ghezzi et al., 2015). When allogeneic corneal grafts are not viable, keratoprosthesis—replacement with an artificial cornea—can be employed, although this approach carries a high risk of complications, including glaucoma and infections (Iyer et al., 2018).
Corneal ulceration, a prevalent corneal pathology, typically involves epithelial defects with or without stromal loss, often accompanied by inflammation (Farghali et al., 2021). Rapid deterioration of corneal tissue and stromal necrosis can lead to perforation, resulting in blindness from scarring and astigmatism (Huerva et al., 2014). Corneal ulcers can be caused by bacterial (e.g., Staphylococcus, Streptococcus, Pseudomonas (Lakhundi et al., 2017)), fungal (e.g., Aspergillus, Fusarium (Sharma et al., 2022)), viral (e.g., Herpes simplex type 1 immune-related (Su et al., 2022)), or traumatic origins (Kang et al., 2020). In advanced cases, the disease often resists high-dose topical antibiotics and systemic corticosteroids (Palioura et al., 2016; Egrilmez and Yildirim-Theveny, 2020). Late-stage interventions include bandage or scleral lenses, amniotic membrane (AM) transplants, conjunctival flaps, autologous serum, tarsorrhaphy, or keratoplasty, depending on the extent of infection or perforation (Tuli et al., 2007; Bremond-Gignac et al., 2019).
Stromal or interstitial keratitis is defined as a nonulcerative and nonsuppurative inflammatory reaction accompanied by neovascularization and cellular penetration in the stroma. Etiologies of interstitial keratitis could be triggered as an immune response to autoimmune diseases (such as Cogan’s syndrome and Hodgkins disease), parasitic diseases (leishmaniasis, malaria, amoebiasis), viral diseases (herpex simplex I and II, influenza, measles), and bacterial diseases (syphilis, tuberculosis keratitis, lyme-associated keratitis, interstitial keratitis) (Schwartz et al., 1998). The primary cause of interstitial keratitis worldwide is syphilis bacterial infection. In the early stages, the disease responds well to high doses of topical steroids (Tu, 2022); however, in some cases, there is a risk of recurrence (Goegebuer et al., 2003; Vingopoulos et al., 2020; Li X. et al., 2023).
Corneal dystrophies, a group of genetic disorders affecting various corneal layers (Weiss et al., 2015, p. 2), include conditions like Fuchs’ endothelial dystrophy. This disease disrupts corneal hydration and can lead to bullous keratopathy, characterized by vision loss and corneal opacity (Kinoshita et al., 2018). Fuchs’ dystrophy progresses through three stages, influenced by mutations in genes like COL8A2, TCF4, and SLC4A11, affecting collagen and endothelial function (Riazuddin et al., 2010; Schmedt et al., 2012). Early management may involve hypertonic saline and bandages, but advanced cases often require corneal transplantation (Mataftsi et al., 2013; Koizumi et al., 2014; Yoshida et al., 2023). Although AM transplantation can alleviate symptoms and improve wound healing (Espana and Birk, 2020; Pires et al., 1999), more clinical trials are needed to assess long-term efficacy (Siu et al., 2014).
Keratoconus, a bilateral ectatic disease, causes progressive thinning and a cone-like protrusion of the cornea, leading to astigmatism and myopia (Claessens et al., 2022). Corneal cross-linking with ultraviolet light and riboflavin is effective in halting disease progression (Pagano et al., 2020; Aytekin and Pehlivan, 2021). Lamellar keratoplasty, which minimizes risks of endothelial rejection, is increasingly preferred over full-thickness keratoplasty for advanced cases (Liu et al., 2015; Feizi et al., 2023).
4 Biomaterial-based approaches for corneal tissue engineering
One of the earliest efforts to construct human cornea equivalents was achieved by Griffith et al., who used immortalized human corneal cells without relying on scaffolds or biomaterials (Griffith et al., 2000). Although these corneal equivalents replicated the main physical and physiological functions of the cornea, they presented several limitations. Advances in artificial cornea development highlighted the need to incorporate various scaffolds. Griffith and her team extensively researched crosslinked human collagen as a safe and stable corneal substitute (Li et al., 2003; Suuronen et al., 2004; Liu et al., 2008; Merrett et al., 2008). This research led to clinical trials involving the implantation of biosynthetic human recombinant collagen-based corneas in human patients, which successfully promoted corneal regeneration and restored vision (Fagerholm et al., 2009; 2014). This was a significant milestone in regenerative medicine and corneal tissue engineering.
Numerous investigations have focused on developing hemicorneal models as partial equivalents of the human cornea, designed to replicate specific layers or functions. These models are particularly useful for studying toxicity, drug delivery, and the fundamental biology of the human cornea without animal models. Hemicorneas can be constructed using human primary corneal LESCs and keratocytes anchored by scaffolds such as chitosan, glycosaminoglycan, human KP lenticules, collagen, and laminins (Builles et al., 2007; Barbaro et al., 2009). Innovative tissue-engineered corneas, which combine natural and synthetic biopolymers with primary corneal cells or stem cells, hold promise for future corneal repair applications.
Hydrogel scaffolds have attracted considerable attention in corneal regeneration due to their excellent biocompatibility, viscoelasticity, and water content, making them ideal substitutes for donor or artificial corneas (Vrehen et al., 2023; Wang M. et al., 2023). Biopolymers frequently studied for corneal construction include collagen, gelatin, methacrylated gelatin (GelMA), silk, hyaluronic acid (HA), and decellularized corneal tissues (Table 2). Collagen hydrogels, in particular, have shown promise as scaffolds for delivering LSCs and supporting full epithelial regeneration post-transplantation. Other bioengineered scaffolds, such as fibrin-agarose combined with allogeneic cells, are currently undergoing clinical trials as potential alternatives for ulcerated corneas (González-Andrades et al., 2017). However, the limited mechanical properties of natural polymers often necessitate combining them with synthetic hydrogels to create hybrid scaffolds with enhanced mechanical strength and customizable properties (Vrehen et al., 2023).
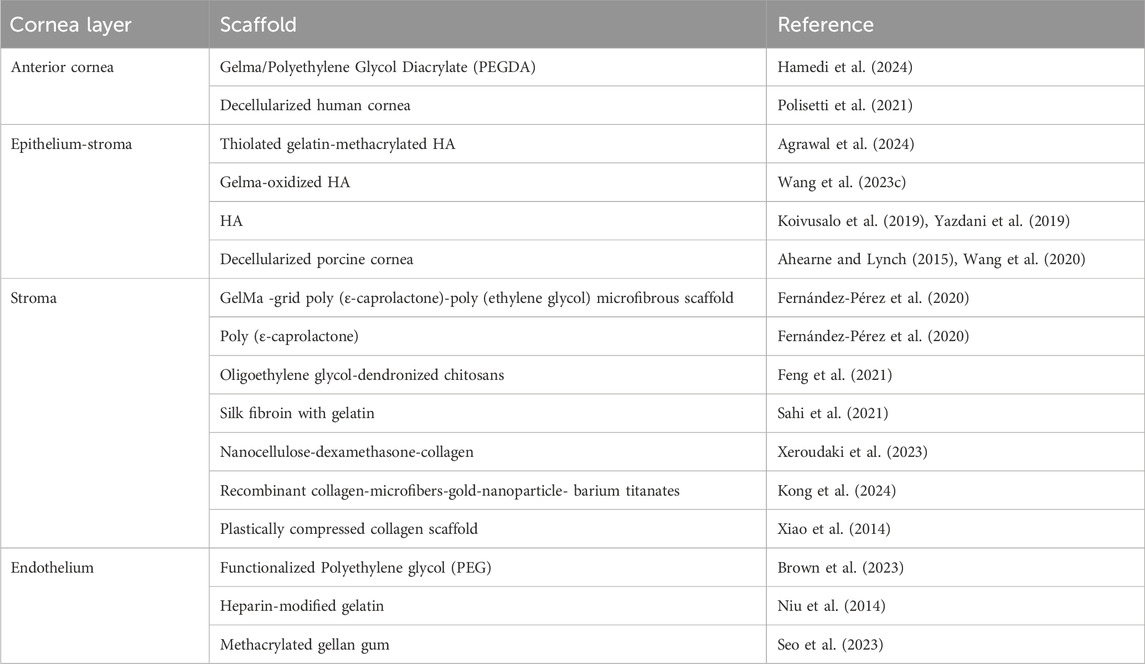
Table 2. Various types of scaffolds utilized in tissue engineering studies for the replacement of different layers of the cornea.
Electrospinning has emerged as a valuable technique for creating corneal scaffolds with interconnected nanofibers, typically less than 1,000 nm in diameter, closely mimicking native corneal architecture (Nosrati et al., 2020). Electrospun nanofibers, such as GelMA composite fibers, have demonstrated potential for corneal applications, as shown in a study by Arica et al., where GelMA prepolymer solutions were homogenized and nitrogen-washed to maintain an inert state before casting and photopolymerization (Figure 2A) (Arica et al., 2021). Electrospinning synthetic biocompatible polymers, including polyε-caprolactone, polylactic acid (PLA), and poly (lactic-co-glycolic acid) (PLGA), has also been explored to fabricate aligned scaffolds that support corneal cell proliferation and migration (Fernández-Pérez et al., 2020; Wang M. et al., 2023). Additionally, electrospun silk-gelatin scaffolds have been used to produce cornea analogs with comparable physicochemical properties (Sahi et al., 2021). This combination of electrospinning with natural and synthetic materials offers a promising approach for developing corneal scaffolds with improved mechanical strength and functionality.
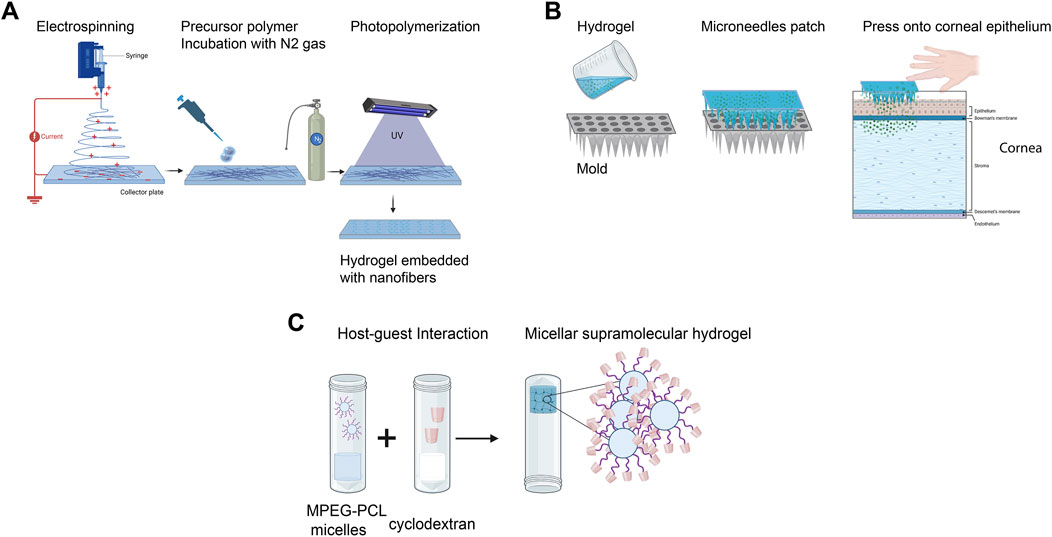
Figure 2. Bioengineered corneal scaffolds. (A) Fabrication of nanofibrous corneal scaffold nanofibers by electrospinning. (B) Utilization of hydrogel with microneedles patches for enhanced ocular drug delivery. (C) Development of micellar supramolecular hydrogel for efficient hydrophobic ocular drug delivery. (Adapted and modified from (Zhang et al., 2016; Arica et al., 2021; Shi et al., 2022; Wang et al., 2024). Figure designed with Biorender (https://app.biorender.com).
In conjunction with these regenerative strategies, integrating advanced drug delivery systems into bioengineered corneal constructs offers significant therapeutic benefits, including reduced inflammation and enhanced tissue healing. One such technology is microneedle injection, which has been widely investigated for efficient drug delivery. A recent study designed a minimally invasive dissolving microneedle array patch using poly D L-lactide and HA (Figure 2B). These acellular patches demonstrated high efficacy in treating fungal keratitis in vivo (Shi et al., 2022). Other hydrogels, such as nanowafers, have also been enhanced with nanodrug delivery systems (Yuan et al., 2015).
Recent advancements in micelle-laden hydrogels have shown promising results for treating glaucoma and other ocular disorders. These hydrogels utilize encapsulated PEG-PLA micelles within a triple-crosslinked hydrogel matrix, enabling prolonged and sustained drug release (Zhao et al., 2023). Constructing such micellar supramolecular hydrogels enhances precorneal retention time due to the hydrogel’s physical crosslinking and improves corneal drug penetration facilitated by the micellar structure. Briefly, supramolecular hydrogels are formed by integrating cyclodextrins (CDs) with monomethoxy PEG block polymers, which increase the delivery efficiency of hydrophobic drugs (Figure 2C) (Zhang et al., 2016).
5 Cell-based strategies for corneal tissue engineering
Regenerative medicine is emerging as a transformative approach with the potential to replace conventional pharmacological treatments across a wide range of diseases. It involves reviving or rejuvenating human tissues, or even organs, using different types of cells to restore normal function (Mousaei Ghasroldasht et al., 2022). Significant advancements in stem cell therapy have been made recently, with numerous approved and ongoing clinical trials. Commonly utilized cell types in these therapies include adult stem cells, mesenchymal stem cells (MSCs), embryonic stem cells (ESCs), and induced pluripotent stem cells (iPSCs) (Chen et al., 2022).
In corneal treatments, one major application of stem cell therapy is in addressing limbal stem cell deficiency (LSCD). The human limbus contains deep crypts surrounded by the palisades of Vogt, and disruptions in this limbal stem cell (LSC) niche framework lead to significant deficiencies (Ghareeb et al., 2020). LSCD staging is based on the extent of limbal involvement, and detecting precise changes requires advanced imaging tools like in vivo confocal microscopy and optical coherence tomography (OCT) (Banayan et al., 2018). Corneal epithelium reconstruction involves isolating and expanding cells from the limbal niche in vitro or ex vivo. For unilateral disease, a biopsy from the unaffected eye provides cells that are cultured and transplanted back into the patient, followed by localized immunosuppression to prevent immune reactions. This approach has shown significant improvements in corneal integrity for LSCD patients (Kolli et al., 2010; Ramírez et al., 2015).
A recent clinical approach expanded LSCs ex vivo under animal-free conditions to reduce patient risks. In this case, autologous LSCs were cultured on human AM under good manufacturing practice conditions, yielding positive results in reversing LSCD (Kolli et al., 2010). However, variations in AM properties limit its consistency, and alternatives with uniform characteristics are sought. Fibrin has been considered, though with lower success rates compared to AM (Rama et al., 2001). Despite success, LSCs alone have limitations in sustaining corneal epithelial homeostasis (Dua et al., 2009).
An alternative to LSCs is using oral mucosa (OM) tissue for patients with LSCD and other ocular surface disorders. OM is rich in stem cells resembling corneal epithelial structures and can form stratified cell layers on the corneal surface. Transplanted OM-derived cells maintain stemness while adopting an epithelial phenotype (Oliva et al., 2020; Gong et al., 2022). Clinical trials have shown that OM-cultured cell sheets improve epithelialization, visual acuity, and transparency in patients with severe ocular disorders (Nishida et al., 2004; Burillon et al., 2012; Kim et al., 2018b). This approach is an autologous-based therapy for corneal disorders (Bandeira et al., 2020). These sheets of autologous cells are treated and expanded ex vivo to be subsequently transplanted to the cornea of the patient. Various clinical trials revealed re-epithelialization of patient corneas after autologous OM-cultured epithelial cell sheet transplantation, with significant enhancement of visual strength and transparency. Treatment with OM epithelial transplantation supported by rigid contact lenses is also promising for restoring the vision of patients with severe ocular surface disorders (Sotozono et al., 2020).
Clinical studies of adipose-derived adult stem cells as implants in five patients with keratoconus yielded an optically transparent autologous stromal graft for up to 3 years without any noted complications (El Zarif et al., 2021). Another clinical cell therapy approach was used in a single-group study, which included 11 people with no CECs detected and who were diagnosed with bullous keratopathy. Injection of ROCK inhibitor-supplemented human CECs improved visual acuity and improved CEC density 168 days post-cellular injection (Kinoshita et al., 2018).
Recent efforts in cell-based therapies focus on autologous approaches using adult stem cells or iPSCs. Human adipose-derived stem cells (ADSCs) can differentiate into epithelial-like cells for autologous therapies. For instance, ADSCs implanted in patients with keratoconus produced transparent grafts, maintaining functionality for up to 3 years (El Zarif et al., 2021).
Other approaches, such as the use of corneal stromal stem cells (CSCs), restore transparency in models with induced scarring (Du et al., 2009). This population of cells has been successfully characterized as an individual population showing essential qualities of adult stem cells; these cells are localized just below the limbus basal membrane (Funderburgh et al., 2016). Although CSCs offer essential regenerative qualities, their scarcity and the challenge of harvesting limit their use. Moreover, achieving high numbers of CSCs by ex vivo subculturing and proliferation could be challenging (Yusoff et al., 2022). ADSCs, by contrast, are readily available, and transplantation trials have shown sustained transparency in animal models (Arnalich-Montiel et al., 2008). Additionally, proof-of-concept trials have also validated the use of bone marrow-derived MSCs transplantation as a safe and effective procedure, with results similar to those of ex vivo cultured allogenic LSC transplantation (Calonge et al., 2019). To sum up, it is much easier to harvest and culture CSCs (namely, limbal stromal MSCs) than the epithelial phenotype stem cells in this region. Furthermore, since these cells are mesenchymal in origin (lacking MHC type 2), they are non-immunogenic and can even be harvested and cultured from the limbal region of donor corneal tissue harvested from cadavers.
Cell-based approaches that are scaffold-free, have been also employed in cornea tissue engineering, these are techniques using cells in suspension, spheroid aggregates forms, or even as sheets (Shah et al., 2008). To date, many fabrication techniques that employ both advanced scaffold-based and scaffold-free methods for the repair of damaged cornea to native structure have been developed (Guérin et al., 2021). The self-assembly process of scaffold-free approaches, enable the formation of stable structures via cell-to-cell interactions, relying on non-covalent interactions to organize molecules (Athanasiou et al., 2013; Nune et al., 2013).
Corneal organoids, 3D models of corneal tissue, show potential in developmental studies, disease modeling, and possibly as replacement organs (Lancaster and Knoblich, 2014). These self-organizing organoids (Meyer-Blazejewska et al., 2010; Nakano et al., 2012; Susaimanickam et al., 2017; Wan et al., 2024) can derive from primary cells, adult stem cells, ESCs, or iPSCs, and are influenced by external factors such as growth factors and ECM substrates (Clevers, 2016).
The corneal organoids replicate the primary cellular components of the cornea in a stable system, offering a platform to study long-term cellular phenotypes associated with diseases such as dry eye disease, as well as developmental processes. These models facilitate the exploration of interactions between various cell types and the ECM (Foster et al., 2017). Notably, corneal organoid cultures have been shown to preserve the limbal epithelial niche for up to 1 month. Epithelial sheets that retain the limbal epithelial phenotype have been successfully engineered from a single organoid. Furthermore, intact organoids have been successfully engrafted onto the limbus in a rabbit model, underscoring their potential for treating ocular surface diseases associated with limbal stem cell deficiency (LSCD) (Higa et al., 2020). Corneal organoids also hold significant promise for applications in drug screening, gene editing, and tissue replacement (Foster et al., 2017). Additionally, these organoids can be used in cell therapy as living biobanks and have high potential in the development of gene therapies, optogenetics, and as a source of transplantable cells (Zhong et al., 2014; Susaimanickam et al., 2017; Chichagova et al., 2020; Lu and Le, 2024).
However, a major challenge in organoid development is maintaining proliferative capacity, which limits their usability in extended testing (Lancaster and Huch, 2019). Other limitations include metabolic build-up, reduced glucose and oxygen diffusion, incomplete maturation, eventual cell necrosis, and a decline in cell proliferation over time (Andrews and Kriegstein, 2022).
6 Bioprinting as a bioengineering tool for corneal tissue
Bioprinting is an advanced bioengineering technique characterized by the additive, layer-by-layer deposition of cells within biomaterials or bioinks into spatially interconnected and defined structures using automated 3D technologies (Wang et al., 2022b). These structures are typically designed using computer-aided design (CAD) models or generated through magnetic resonance imaging or computed tomography (Shelmerdine et al., 2018; Schmieg et al., 2022).
Bioinks are fluidic biomaterials used in bioprinting to incorporate living cells, either in suspension or aggregate form (Zhang et al., 2018). When selecting a bioink, key factors to consider include printability, biocompatibility, biodegradability, mechanical stability, integrity, and similarity to the native composition (Wang et al., 2022b).
An emerging and promising technology in this field is Freeform Reversible Embedding of Suspended Hydrogels (FRESH). This technique provides mechanical support through a thermoreversible gelatin slurry bath, creating an environment conducive to cellular migration and interaction (Corbett et al., 2019).
The primary strategies employed in 3D bioprinting include stereolithography, extrusion, laser-assisted, and inkjet bioprinting (Figure 3) (Matai et al., 2020). Stereolithography bioprinting is based on crosslinking a liquid photocrosslinkable bioink upon exposure to a specific light source, controlled by CAD, to produce a 3D model (Figure 3A) (Li W. et al., 2023). Extrusion-based bioprinting, the most common approach for corneal applications, creates structures through continuous deposition (Figure 3B) (Cooke and Rosenzweig, 2021). Laser-assisted bioprinting uses a focused laser beam to transfer a precise volume of bioink from a donor substrate to a receiver (Figure 3C) (Yang et al., 2021). Finally, inkjet bioprinting employs thermal or piezoelectric mechanisms to eject bioink droplets from the printhead, forming layer-by-layer structures (Figure 3D) (Kumar et al., 2021).
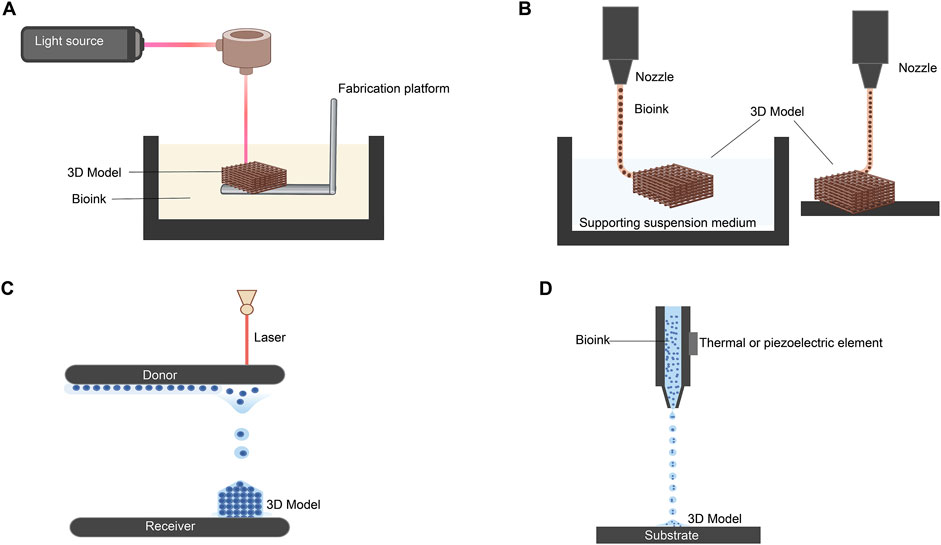
Figure 3. Most common strategies for 3D bioprinting. (A) Stereolithography-based 3D bioprinting. (B) Extrusion-based methods using suspended and direct extrusion bioprinting. (C) Laser-assisted bioprinting. (D) Inkjet bioprinting via piezoelectric and thermal elements. (Adapted and modified from (Cooke and Rosenzweig, 2021; Kumar et al., 2021; Yang et al., 2021; Li W. et al., 2023). Figure designed with Biorender (https://app.biorender.com).
6.1 Bioprinting of the corneal epithelium
Limbal epithelial stem cells demonstrate strong proliferative and migratory capabilities, allowing them to migrate from the limbus to the epithelial layer, where they differentiate into epithelial cells (Wang B. et al., 2023). This suggests the hypothetical possibility of forming the epithelial layer without the need for bioprinting. Bioengineered cell sheets have become the primary approach for generating a functional corneal epithelial layer in vitro (Bardag-Gorce et al., 2013; Kobayashi et al., 2013; Venugopal et al., 2020). Consequently, compared to research on the corneal stroma, far fewer studies have focused on 3D bioprinting for the corneal epithelium (Table 3).
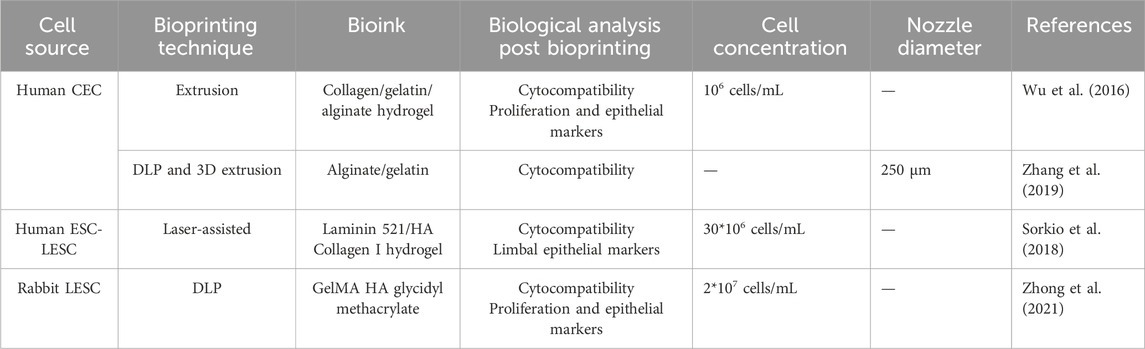
Table 3. Summary of recent significant studies on 3D bioprinting of corneal epithelium: a comparative analysis of bioprinting parameters, cell sources, technical approaches, bioinks, and key findings.
6.2 Bioprinting the corneal stroma
Numerous studies on corneal stroma bioprinting have been conducted using various bioprinting techniques, 3D software designs, and cell types (Table 4).
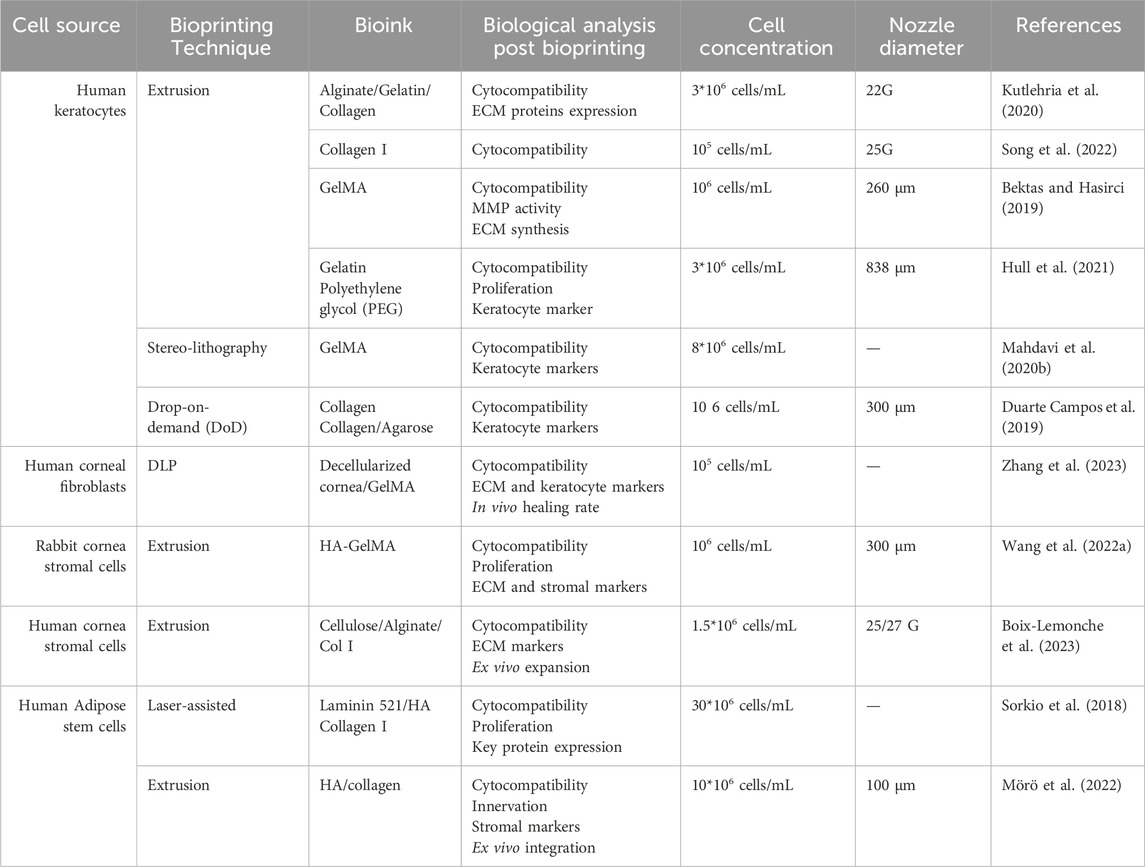
Table 4. Summary of recent significant studies on 3D bioprinting of corneal stroma: a comparative analysis of bioprinting parameters, cell sources, technical approaches, bioinks, and key findings.
Corneal stroma bioprinting primarily utilizes collagen bioink due to its biocompatibility (Osidak et al., 2020). However, to address the weak mechanical properties of collagen, it is often combined with other bioinks, such as agarose (Duarte Campos et al., 2019). Duarte Campos et al. employed the Drop-on-Demand (DoD) technique to achieve a corneal stroma structure while maintaining transparency (Duarte Campos et al., 2019). A limitation of this study is that the proposed bioprinted stroma lacks the organized collagen fibril architecture found in the native cornea, which impacts the biomechanical stability of the model.
In the work of Isaacson et al., corneal stroma was bioprinted using extrusion bioprinting with primary keratocytes embedded in alginate and collagen (Isaacson et al., 2018). However, the assessment of biological compatibility was limited to LIVE/DEAD viability assays and trypan blue staining. Additionally, the round morphology of bioprinted keratocytes post-printing indicates a lack of differentiation within the hydrogel.
Due to its favorable mechanical properties and functionality, GelMA has become widely used in bioprinting. Constructs bioprinted with GelMA loaded with corneal keratocytes showed high transparency and cell viability for up to 1 week, although cell proliferation declined significantly afterward (Bektas and Hasirci, 2019). Visible light-based stereolithography using GelMA also demonstrated optimal transparency immediately after bioprinting. Embedded corneal keratocytes retained high gene expression of specific markers and exhibited good viability 7 days post-bioprinting (Mahdavi et al., 2020a).
A team from Stanford and Carnegie Mellon Universities formulated and stabilized a variety of polymers to use as UNION bioinks. High stromal cell viability was observed 24 h post-printing, and the cell phenotype was maintained for 7 days using gelatin as a bioink (Hull et al., 2021). Recently, HA-based bioinks were developed for laser-assisted bioprinting, demonstrating promising physicochemical properties and high cellular compatibility (Sorkio et al., 2018). However, there has been no validation of corneal keratocyte phenotype or integration post-bioprinting. Other studies have achieved cell-laden structures with enhanced mechanical properties using HA bioink (Mörö et al., 2022).
6.3 Bioprinting of the corneal endothelium
Very few studies have attempted to bioprint corneal endothelial layers using solely extrusion-based bioprinters (Table 5). In parallel, the most effective approaches in corneal endothelial therapy currently involve artificial ROCK inhibitors, Descemet stripping only, and cellular and gene therapy techniques (Rocha-de-Lossada et al., 2021).
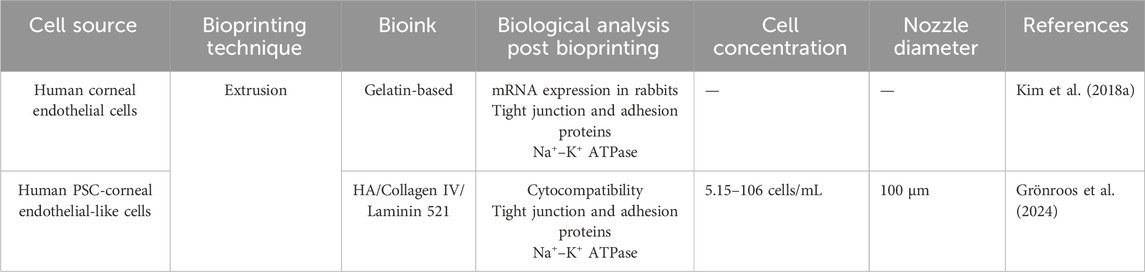
Table 5. Summary of recent significant studies on 3D bioprinting of corneal endothelium: a comparative analysis of bioprinting parameters, cell sources, technical approaches, bioinks, and key findings.
In recent studies, researchers have employed 3D extrusion bioprinting to deposit human corneal endothelial cells modified with RNase 5 plasmid vectors. This modification significantly enhanced cell survival and proliferation by activating cyclin and inhibiting apoptotic protein 4. These modified cells were bioprinted onto lyophilized AM and grafted into rabbit models, leading to notable improvements in edema and corneal thickness (Kim et al., 2018b). This approach underscores the potential of bioengineered 3D bioprinted corneal endothelial models for transplantation.
Additionally, extrusion-based 3D bioprinting has been used to develop integrated tissue from hiPSC-derived corneal endothelial cells, which exhibited essential endothelial characteristics and maintained cell viability for over 1 week. This was achieved using a specialized bioink composed of laminin, collagen IV, and HA (Grönroos et al., 2024).
7 Cell-free approaches for corneal tissue engineering
The use of stem cells as therapeutic agents has raised clinical safety concerns due to their heterogeneity and the potential for immune responses upon transplantation (Dua et al., 2009; Shi et al., 2012; Masterson et al., 2021; Oh et al., 2021). As a result, cell-free approaches are gaining traction in research, aiming to induce “cell homing” and recruit tissue-resident cells through bioactive molecules (Thalakiriyawa and Dissanayaka, 2024). Among these molecules are secretomes—such as cytokines, extracellular vesicles (EVs), and growth factors—produced by stem cells. EVs from various cellular sources have shown considerable promise in corneal repair and regeneration (Figure 4).
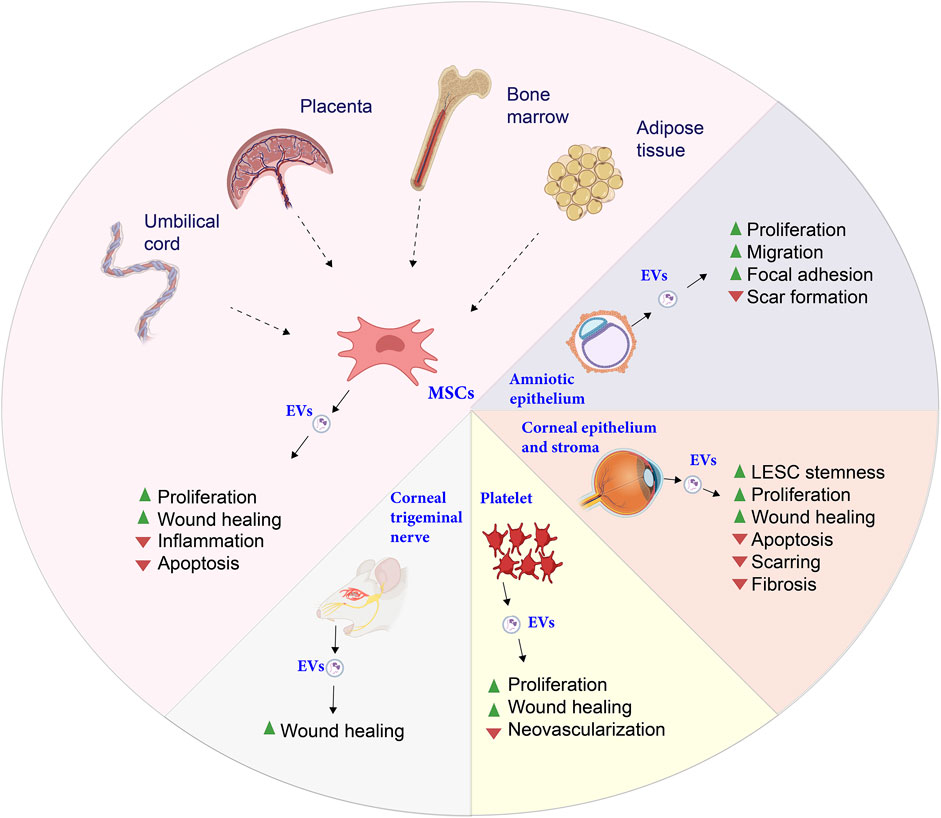
Figure 4. Summary of the known functions of extracellular vesicles (EVs) according to their origin. While most of them act positively on cell proliferation, each type of EVs modulate differently secondary cellular and physiological processes. Figure designed with Biorender (https://app.biorender.com).
Research on EVs derived from CSCs has demonstrated their regenerative and healing properties, including scar reduction, suppression of fibrosis markers, and enhancement of LESC properties via the Notch pathway (Shojaati et al., 2019; Santra et al., 2022; Wang et al., 2023e; Santra et al., 2024). EVs from human placental mesenchymal stem cells (MSCs) have also been found to enhance cell proliferation, exhibit anti-inflammatory effects, and facilitate wound healing in CECs (Tao et al., 2019). Similarly, EVs from bone marrow-derived MSCs promote corneal repair, protect endothelial and epithelial cells from apoptosis via caspase-3 inhibition, and support healing processes (Buono et al., 2021; Nuzzi et al., 2021; Saccu et al., 2022; Tati et al., 2024).
Moreover, EVs from placental and umbilical cord MSCs aid in wound healing by reducing inflammation and accelerating corneal repair (Tao et al., 2019; Liu et al., 2022). EVs from ADSCs are known to reduce inflammation in dry eye disease and support epithelial repair in diabetic models (Yu et al., 2020; Wang et al., 2023d; Wang et al., 2023 G.). EVs from other sources also contribute uniquely to corneal healing. For instance, EVs from corneal myofibroblasts enhance endothelial cell migration and proliferation (Yeung et al., 2022), while those from human amniotic epithelial cells stimulate epithelial and stromal cell proliferation, reduce scarring, and activate the focal adhesion pathway (Hu et al., 2022; Yi et al., 2024).
EVs are now being utilized within electrospun scaffolds, marking a significant advancement in cell-free regenerative strategies for corneal repair. Electrospun nanofibrous scaffolds, particularly those composed of PLGA, have proven to be highly effective for the delivery of bioactive molecules. Recent studies have demonstrated that these scaffolds, when loaded with MSC-derived EVs, exhibit considerable therapeutic efficacy. In models of corneal and retinal injuries, PLGA nanofibrous scaffolds incorporating MSC-EVs have been shown to significantly enhance tissue regeneration, while reducing fibrosis and scar formation, suggesting their potential for ocular surface reconstruction (Wang et al., 2024).
This emerging approach is not limited to synthetic polymers; EVs have also been integrated into biological scaffolds, such as porcine-derived decellularized ECM and modified nanofibrous structures, further demonstrating their versatility in corneal tissue engineering (Hernandez et al., 2018). Additionally, while electrospun scaffolds have shown promising results, EVs have been incorporated into other biomaterials, such as MSC-EV-loaded chitosan hydrogels, which have similarly facilitated epithelial and stromal regeneration and reduced post-injury scar formation (Tang et al., 2022). The use of EVs within electrospun scaffolds and other biomaterial platforms represents a highly promising avenue in the development of advanced, cell-free therapies for corneal repair, offering both structural support and bioactivity necessary for effective tissue regeneration.
Despite these promising developments, EV-based therapies are still in their early stages, necessitating further research to fully understand their mechanisms of action and to comprehensively characterize EVs for use in corneal regeneration.
8 Overcoming current limitations
Despite the broad range of current treatments for corneal pathologies, sight-threatening conditions that require corneal grafts remain challenging to cure due to the limited availability of donor tissues. The risks associated with autologous tissue use and the lifelong immunosuppression required for allogenic grafts make bioengineered corneal tissue an appealing alternative. While emerging treatments, such as stem cell therapy and autologous oral mucosa transplantation, show promise, this discussion focuses on the essential features of a bioengineered cornea.
A key aspect of bioengineered corneas is sourcing cells. To reduce rejection risk, the cells should ideally originate from the patient. It is anticipated that two primary cell sources will be prominent in the coming years: oral mucosa cells and reprogrammed human iPSCs. While oral mucosa cells may lack the ability to transdifferentiate into keratocytes or endothelial cells, iPSCs present risks of genomic instability and require careful monitoring to ensure safety in bioengineered corneas. Furthermore, EVs have emerged as important components in cornea tissue engineering, facilitating cell communication, migration, and promoting tissue regeneration through their diverse cargo (proteins, RNA) (Hutcheon et al., 2019; McKay et al., 2019; 2020). However, no EV-based therapies have received FDA approval for therapeutic use up to this date (Cheng and Kalluri, 2023). As a relatively new field, current EV isolation techniques hold various limitations, including low yield, limited purity, sample heterogeneity, which are particularly problematic for producing clinical-grade EVs (Allelein et al., 2021). Additional challenges appear in direct incorporation of EVs into hydrogels or any type of scaffold due to variability in their loading efficiency between different batches. This issue could be resolved by employing the co-printing method outlined in Bari et al., where EVs are encapsulated within a hydrogel, providing a controlled and sustained release (Bari et al., 2021). There has been few research on loading therapeutic cargo onto EVs to treat occular diseases. Therefore, further research is essential to develop novel EV-related therapeutic strategies in ophthalmology.
As the field progresses, innovative solutions related to hydrogel composition, optimal cell sources, and clinically applicable methods will likely enhance clinical ophthalmology, particularly in cell therapy, organ bioprinting, and personalized medicine. Scaffold-free cell injection strategy might be promising due to the reduced inflammatory response and biomimetic properties. However, implementing this technique requires adherence to strict quality standards, alongside thorough in vivo studies, to effectively regulate complex matrix alignment of the cornea (Gouveia et al., 2017a; Syed-Picard et al., 2018). Moreover, cell injection alone is insufficient for regenerating severely damaged cornea, as it lacks the structural matrix support essential for proper cell localization and integration. For instance, injecting stromal cells into the corneal stroma can distort the native fibrillar architecture of the tissue (Alió del Barrio and Alió, 2018; Alió et al., 2019). While transparency and cell organization can be achieved, corneal models generated by scaffold-free approaches often lack long term stability, and mechanical robustness, which is crucial for withstanding the natural pressures of the eye (Guérin et al., 2021).
To achieve an optimal bioengineered cornea, it is essential to replicate the physiological properties of the native cornea and maintain a suitable microenvironment by utilizing suitable scaffolds. Hydration balance is a critical factor, with the native cornea maintaining a hydration limit of approximately 80% (Rinawati et al., 2018). Disruption of this balance characterizes various corneal diseases, including corneal edema (Yao et al., 2020).
The current research on scaffolds requires more focus on the nano-structural properties, cell-scaffold interaction, and scaffold degradation. Recent innovations such as implementation of curved templates has successfully facilitated the production of more accurate corneal curvatures while promoting the alignment of corneal keratocytes and collagen fibrils (Gouveia et al., 2017b). Additionally, Gouveia et al. developed self-lifting analogous tissue equivalents by using peptide amphiphile-coated surfaces with varying anisotropies (Gouveia et al., 2017a). Electrospun polyvinyl acetate and collagen scaffolds were also shown to mimic the cornea’s native structure. Although the scaffolds promoted cell adhesion and proliferation, poor mechanical properties, particularly inadequate strength for surgical suturing, limited their clinical application (Wu et al., 2018).
In bioengineered corneas, scaffold production consistency and scalability also present significant challenges once scaled up for larger clinical trials. Pore size and distribution within scaffolds are additional factors critical to the controlled release of therapeutic molecules. Pore characteristics also alter the scaffold’s rheological properties and mechanical stability, which are essential for supporting cellular activities and diffusion processes within the bioengineered scaffold (Fischer et al., 2019; Hady et al., 2022). Other issues also exist, and are related to the potential risk of inducing immune responses, heterogeneous cell distribution, and achieving a degradation rate that aligns with the cornea tissue formation (Ovsianikov et al., 2018). Polymers including PGA and PLGA are among the most commonly used in tissue-engineered corneas, and have been approved by the US FDA for clinical use. (Hu et al., 2004; Zhang et al., 2008; Deshpande et al., 2013; Arabpour et al., 2019). Additionally, acellular porcine corneal products gained approval of the FDA in 2015 (Zhang et al., 2015; Tran and Hou, 2023).
Even with the extensive research on biomaterials for corneal tissue regeneration, there is still no single agreement on the ideal scaffold or cell source. Clinical trials have yielded limited success and insufficient remodeling during scaffold degradation. While a significant portion of the trials focuses on evaluating various bio-based scaffolds, the majority of registered studies are dedicated to stem cell therapy and bioactive molecules. This highlights the prevailing interest in these two areas within the field of cornea regenerative medicine (Boroumand et al., 2024). Cornea reconstruction using synergism of scaffold-free and scaffold-based biomimetic approaches could advance scaffold functionalities, specifically for corneal stromal reconstruction (Jakab et al., 2010; Ovsianikov et al., 2018; Zurina et al., 2020). Mechanical stability, transparency, and integration with the host tissues, remain critical barriers to successful corneal tissue engineering, in both scaffold-free and biomaterial-based approaches. Thus, translational application is dependent on strategies that can support cell expansion, mimic the cornea ECM composition, and facilitates the overall cornea reconstruction process of a functional tissue.
While 3D bioprinting technology enables precise cellular placement within scaffolds, many current corneal bioprinting studies lack micropatterned cellular arrangements that closely mimic natural tissue. As a result, despite attempts to spatially arrange bioprinted cells, these studies have struggled to replicate the functionality and cellular diversity of the native human cornea (Brassard et al., 2021). Additional considerations, such as stiffness and mechanical properties, are crucial to enable successful suturing or clipping during transplantation (Fuest et al., 2020). Navigating these barriers necessitates a careful recapitulation of embryonic development and organization, allowing cellular self-assembly through patterned gene expression (Warmflash et al., 2014). This kinetic and spatial coordination is key for successful tissue generation and cellular turnover, as adult corneal homeostasis relies on the replication dynamics and spatial balance of corneal stem cells (Strinkovsky et al., 2021). Achieving a precise macroscale structure is influenced by microscale interactions that facilitate the self-organization and assembly of cells and tissues. Bioprinting and cell-specific aggregation are effective strategies for regulating the bottom-up assembly of tissues with complex biological characteristics (Brassard and Lutolf, 2019). A specific challenge in corneal tissue-engineered constructs is maintaining the orthogonal collagen fibrillary microarchitecture which is essential for corneal transparency. Addressing this issue may be possible by utilizing a technique initially developed for cartilage tissue engineering. This approach incorporates 4D bioprinting to remodel collagen fibers using magnetic streptavidin-coated iron nanoparticles, enabling real-time magnetic orientation during the printing process (Betsch et al., 2018).
Given the complexity of the cornea, developing an approved model would require combining advanced bioprinting technologies with other methods, including organoids and stem cells (Brassard and Lutolf, 2019; Jia et al., 2023). Moreover, creating a synergistic blend of 3D bioprinting and organoids would shift the focus to the targeted tissue’s structure and function, enabling the development of deliberate spatial designs that promote higher accuracy and faster organoid self-assembly (Ren et al., 2021). This integrated approach could enhance the production of functional, self-organized artificial tissues or organs. Recent studies have applied this concept to create promising centimeter-scale tissues that exhibit diverse, self-organized features of bioprinted organs, such as the heart, kidney, and intestines (Brassard et al., 2021; Humphreys, 2021; Lawlor et al., 2021).
Another important aspect of tissue engineering involves extensive animal model studies before clinical application. Anatomical studies of various species are crucial for designing engineered tissue models that are applicable to humans (Mukherjee et al., 2022). Selecting the ideal animal model requires an understanding of anatomical and pathophysiological similarities to humans to ensure that the model is fit for purpose (Liebschner, 2004; Ribitsch et al., 2020). Studies on corneas from different organisms help identify models with anatomy closest to the human cornea. Advances in human corneal surgery have often built on veterinary research; for example, studies on dog corneal ultrastructure have contributed to human surgical techniques (Kafarnik et al., 2023). Similarly, in tissue engineering, acellular scaffolds from bovine and porcine corneas have been used to support cellular function in bioengineered models. Extrapolating insights from animal studies is essential for optimizing tissue engineering strategies for human application (Ponce Márquez et al., 2009; Isidan et al., 2019). Collectively, these insights are vital for developing bioengineered corneas that can be used clinically.
Consequently, existing models have not advanced to clinical trials. Moving toward clinical trials would require substantial efforts to develop higher-performing models and a deeper understanding of corneal physiology and anatomy. Addressing issues related to host immune responses to bioartificial corneas and ensuring long-term compatibility is essential for this transition.
Author contributions
RA: Writing–original draft, Writing–review and editing. VD: Writing–original draft, Writing–review and editing. FM: Writing–original draft, Writing–review and editing.
Funding
The author(s) declare that financial support was received for the research, authorship, and/or publication of this article. This review was supported by the ATIP-Avenir program, Inserm, ANR (CE17 - Project TeFiCoPa), FRM (REP202110014140), and the Groupama Foundation.
Conflict of interest
The authors declare that the research was conducted in the absence of any commercial or financial relationships that could be construed as a potential conflict of interest.
Publisher’s note
All claims expressed in this article are solely those of the authors and do not necessarily represent those of their affiliated organizations, or those of the publisher, the editors and the reviewers. Any product that may be evaluated in this article, or claim that may be made by its manufacturer, is not guaranteed or endorsed by the publisher.
References
Agrawal, P., Tiwari, A., Chowdhury, S. K., Vohra, M., Gour, A., Waghmare, N., et al. (2024). Kuragel: a biomimetic hydrogel scaffold designed to promote corneal regeneration. iScience 27, 109641. doi:10.1016/j.isci.2024.109641
Ahearne, M., and Lynch, A. P. (2015). Early observation of extracellular matrix-derived hydrogels for corneal stroma regeneration. Tissue Eng. Part C. Methods 21, 1059–1069. doi:10.1089/ten.tec.2015.0008
Alió del Barrio, J. L., and Alió, J. L. (2018). Cellular therapy of the corneal stroma: a new type of corneal surgery for keratoconus and corneal dystrophies. Eye Vis 5, 28. doi:10.1186/s40662-018-0122-1
Alió, J. L., Alió Del Barrio, J. L., El Zarif, M., Azaar, A., Makdissy, N., Khalil, C., et al. (2019). Regenerative surgery of the corneal stroma for advanced keratoconus: 1-year outcomes. Am. J. Ophthalmol. 203, 53–68. doi:10.1016/j.ajo.2019.02.009
Allelein, S., Medina-Perez, P., Lopes, A. L. H., Rau, S., Hause, G., Kölsch, A., et al. (2021). Potential and challenges of specifically isolating extracellular vesicles from heterogeneous populations. Sci. Rep. 11, 11585. doi:10.1038/s41598-021-91129-y
Andrews, M. G., and Kriegstein, A. R. (2022). Challenges of organoid research. Annu. Rev. Neurosci. 45, 23–39. doi:10.1146/annurev-neuro-111020-090812
Arnalich-Montiel, F., Pastor, S., Blazquez-Martinez, A., Fernandez Delgado, J., Nistal, M., Alio, J. L., et al. (2008). Adipose-derived stem cells are a source for cell therapy of the corneal stroma. Stem Cells 26 (2), 570–579. doi:10.1634/stemcells.2007-0653
Aquavella, J. V. (2007). Challenges, opportunities and controversy in keratoplasty. Expert Rev. Ophthalmol. 2, 1–4. doi:10.1586/17469899.2.1.1
Arabpour, Z., Baradaran-Rafii, A., Bakhshaiesh, N. L., Ai, J., Ebrahimi-Barough, S., Esmaeili Malekabadi, H., et al. (2019). Design and characterization of biodegradable multi layered electrospun nanofibers for corneal tissue engineering applications. J. Biomed. Mater. Res. Part A 107, 2340–2349. doi:10.1002/jbm.a.36742
Arica, T. A., Guzelgulgen, M., Yildiz, A. A., and Demir, M. M. (2021). Electrospun GelMA fibers and p(HEMA) matrix composite for corneal tissue engineering. Mater Sci. Eng. C Mater Biol. Appl. 120, 111720. doi:10.1016/j.msec.2020.111720
Athanasiou, K., Eswaramoorthy, R., Hadidi, P., and Hu, J. (2013). Self-organization and the self-assembling process in tissue engineering. Annu. Rev. Biomed. Eng. 15, 115–136. doi:10.1146/annurev-bioeng-071812-152423
Aytekin, E., and Pehlivan, S. B. (2021). Corneal cross-linking approaches on keratoconus treatment. J. Drug Deliv. Sci. Technol. 63, 102524. doi:10.1016/j.jddst.2021.102524
Banayan, N., Georgeon, C., Grieve, K., Ghoubay, D., Baudouin, F., and Borderie, V. (2018). In vivo confocal microscopy and optical coherence tomography as innovative tools for the diagnosis of limbal stem cell deficiency. J. Fr. Ophtalmol. 41, e395–e406. doi:10.1016/j.jfo.2018.09.003
Bandeira, F., Goh, T.-W., Setiawan, M., Yam, G. H.-F., and Mehta, J. S. (2020). Cellular therapy of corneal epithelial defect by adipose mesenchymal stem cell-derived epithelial progenitors. Stem Cell Res. and Ther. 11, 14. doi:10.1186/s13287-019-1533-1
Barbaro, V., Ferrari, S., Fasolo, A., Ponzin, D., and Di Iorio, E. (2009). Reconstruction of a human hemicornea through natural scaffolds compatible with the growth of corneal epithelial stem cells and stromal keratocytes. Mol. Vis. 15, 2084–2093.
Bardag-Gorce, F., Oliva, J., Wood, A., Niihara, H., Makalinao, A., Sabino, S., et al. (2013). Microarray analysis of oral mucosal epithelial cell sheet. Tissue Eng. Regen. Med. 10, 362–370. doi:10.1007/s13770-013-1103-z
Bari, E., Scocozza, F., Perteghella, S., Sorlini, M., Auricchio, F., Torre, M. L., et al. (2021). 3D bioprinted scaffolds containing mesenchymal stem/stromal lyosecretome: next generation controlled release device for bone regenerative medicine. Pharmaceutics 13, 515. doi:10.3390/pharmaceutics13040515
Beems, E. M., and Van Best, J. A. (1990). Light transmission of the cornea in whole human eyes. Exp. Eye Res. 50, 393–395. doi:10.1016/0014-4835(90)90140-P
Bektas, C.K., and Hasirci, V. (2019). Cell loaded 3D bioprinted GelMA hydrogels for corneal stroma engineering. Biomater. Sci. 8, 438–449. doi:10.1039/C9BM01236B
Bergmanson, J., Burns, A., and Walker, M. (2019). Anatomical explanation for the central-peripheral thickness difference in human corneas. Investigative Ophthalmol. and Vis. Sci. 60, 4652.
Betsch, M., Cristian, C., Lin, Y.-Y., Blaeser, A., Schöneberg, J., Vogt, M., et al. (2018). Incorporating 4D into bioprinting: real-time magnetically directed collagen fiber alignment for generating complex multilayered tissues. Adv. Healthc. Mater. 7, 1800894. doi:10.1002/adhm.201800894
Blatt, H. L., Rao, G. N., and Aquavella, J. V. (1979). Endothelial cell density in relation to morphology. Investigative Ophthalmol. and Vis. Sci. 18, 856–859.
Boix-Lemonche, G., Nagymihaly, R.M., Niemi, E.M., Josifovska, N., Johansen, S., Moe, M.C., Scholz, H., and Petrovski, G. (2023). Intracorneal Implantation of 3D Bioprinted Scaffolds Containing Mesenchymal Stromal Cells Using Femtosecond-Laser-Assisted Intrastromal Keratoplasty. Macromolecular Bioscience 23, 2200422. doi:10.1002/mabi.202200422
Boroumand, S., Rahmani, M., Sigaroodi, F., Ganjoury, C., Parandakh, A., Bonakdar, A., et al. (2024). The landscape of clinical trials in corneal regeneration: a systematic review of tissue engineering approaches in corneal disease. J. Biomed. Mater. Res. Part B Appl. Biomaterials 112, e35449. doi:10.1002/jbm.b.35449
Bourne, W. M., and Kaufman, H. E. (1976). Specular microscopy of human corneal endothelium in vivo. Am. J. Ophthalmol. 81, 319–323. doi:10.1016/0002-9394(76)90247-6
Brassard, J. A., and Lutolf, M. P. (2019). Engineering stem cell self-organization to build better organoids. Cell Stem Cell 24, 860–876. doi:10.1016/j.stem.2019.05.005
Brassard, J. A., Nikolaev, M., Hübscher, T., Hofer, M., and Lutolf, M. P. (2021). Recapitulating macro-scale tissue self-organization through organoid bioprinting. Nat. Mater. 20, 22–29. doi:10.1038/s41563-020-00803-5
Bremond-Gignac, D., Daruich, A., Robert, M. P., and Chiambaretta, F. (2019). Recent innovations with drugs in clinical trials for neurotrophic keratitis and refractory corneal ulcers. Expert Opin. Investigational Drugs 28, 1013–1020. doi:10.1080/13543784.2019.1677605
Bron, A. J. (2001). The architecture of the corneal stroma. Br. J. Ophthalmol. 85, 379–381. doi:10.1136/bjo.85.4.379
Brown, K. D., Palmer, J., Finnigan, J., Gurr, P., Montenegro, J., Dusting, G., et al. (2023). Functionalising a PEG-based hydrogel scaffold improves human corneal endothelial cell culture for tissue-engineered endothelial keratoplasty. Investigative Ophthalmol. and Vis. Sci. 64, 3812.
Builles, N., Bechetoille, N., Justin, V., André, V., Barbaro, V., Di Iorio, E., et al. (2007). Development of a hemicornea from human primary cell cultures for pharmacotoxicology testing. Cell Biol. Toxicol. 23, 279–292. doi:10.1007/s10565-006-0191-0
Buono, L., Scalabrin, S., De Iuliis, M., Tanzi, A., Grange, C., Tapparo, M., et al. (2021). Mesenchymal stem cell-derived extracellular vesicles protect human corneal endothelial cells from endoplasmic reticulum stress-mediated apoptosis. Int. J. Mol. Sci. 22, 4930. doi:10.3390/ijms22094930
Burillon, C., Huot, L., Justin, V., Nataf, S., Chapuis, F., Decullier, E., et al. (2012). Cultured autologous oral mucosal epithelial cell sheet (CAOMECS) transplantation for the treatment of corneal limbal epithelial stem cell deficiency. Investigative Ophthalmol. and Vis. Sci. 53, 1325–1331. doi:10.1167/iovs.11-7744
Calonge, M., Pérez, I., Galindo, S., Nieto-Miguel, T., López-Paniagua, M., Fernández, I., et al. (2019). A proof-of-concept clinical trial using mesenchymal stem cells for the treatment of corneal epithelial stem cell deficiency. Transl. Res. 206, 18–40. doi:10.1016/j.trsl.2018.11.003
Carlsson, D. J., Li, F., Shimmura, S., and Griffith, M. (2003). Bioengineered corneas: how close are we? Curr. Opin. Ophthalmol. 14, 192–197. doi:10.1097/00055735-200308000-00004
Chaon, B., Maltry, A., and Hou, J. (2016). Comparison of central and peripheral Descemet’s membrane thickness in human donor corneas. Investigative Ophthalmol. and Vis. Sci. 57, 5267.
Chen, S., Mienaltowski, M. J., and Birk, D. E. (2015). Regulation of corneal stroma extracellular matrix assembly. Exp. Eye Res. 133, 69–80. doi:10.1016/j.exer.2014.08.001
Chen, X., Jiang, Y., Duan, Y., Zhang, X., and Li, X. (2022). Mesenchymal-stem-cell-based strategies for retinal diseases. Genes 13, 1901. doi:10.3390/genes13101901
Cheng, K., and Kalluri, R. (2023). Guidelines for clinical translation and commercialization of extracellular vesicles and exosomes based therapeutics. Extracell. Vesicle 2, 100029. doi:10.1016/j.vesic.2023.100029
Cheng, X., and Pinsky, P. M. (2013). Mechanisms of self-organization for the collagen fibril lattice in the human cornea. J. R. Soc. Interface 10, 20130512. doi:10.1098/rsif.2013.0512
Chichagova, V., Hilgen, G., Ghareeb, A., Georgiou, M., Carter, M., Sernagor, E., et al. (2020). Human iPSC differentiation to retinal organoids in response to IGF1 and BMP4 activation is line- and method-dependent. Stem Cells 38, 195–201. doi:10.1002/stem.3116
Claessens, J. L. J., Godefrooij, D. A., Vink, G., Frank, L. E., and Wisse, R. P. L. (2022). Nationwide epidemiological approach to identify associations between keratoconus and immune-mediated diseases. Br. J. Ophthalmol. 106, 1350–1354. doi:10.1136/bjophthalmol-2021-318804
Clevers, H. (2016). Modeling development and disease with organoids. Cell 165, 1586–1597. doi:10.1016/j.cell.2016.05.082
Cooke, M. E., and Rosenzweig, D. H. (2021). The rheology of direct and suspended extrusion bioprinting. Apl. Bioeng. 5, 011502. doi:10.1063/5.0031475
Corbett, D. C., Olszewski, E., and Stevens, K. (2019). A FRESH take on resolution in 3D bioprinting. Trends Biotechnol. 37, 1153–1155. doi:10.1016/j.tibtech.2019.09.003
Delmonte, D. W., and Kim, T. (2011). Anatomy and physiology of the cornea. J. Cataract and Refract. Surg. 37, 588–598. doi:10.1016/j.jcrs.2010.12.037
Deshpande, P., Ramachandran, C., Sefat, F., Mariappan, I., Johnson, C., McKean, R., et al. (2013). Simplifying corneal surface regeneration using a biodegradable synthetic membrane and limbal tissue explants. Biomaterials 34, 5088–5106. doi:10.1016/j.biomaterials.2013.03.064
Dias, J., Diakonis, V. F., Kankariya, V. P., Yoo, S. H., and Ziebarth, N. M. (2013). Anterior and posterior corneal stroma elasticity after corneal collagen crosslinking treatment. Exp. Eye Res. 116, 58–62. doi:10.1016/j.exer.2013.07.028
Doughty, M. J., and Zaman, M. L. (2000). Human corneal thickness and its impact on intraocular pressure measures: a review and meta-analysis approach. Surv. Ophthalmol. 44, 367–408. doi:10.1016/S0039-6257(00)00110-7
Du, Y., Carlson, E. C., Funderburgh, M. L., Birk, D. E., Pearlman, E., Guo, N., et al. (2009). Stem cell therapy restores transparency to defective murine corneas. Stem Cells 27, 1635–1642. doi:10.1002/stem.91
Dua, H. S., Shanmuganathan, V. A., Powell-Richards, A. O., Tighe, P. J., and Joseph, A. (2005). Limbal epithelial crypts: a novel anatomical structure and a putative limbal stem cell niche. Br. J. Ophthalmol. 89, 529–532. doi:10.1136/bjo.2004.049742
Dua, H. S., Miri, A., Alomar, T., Yeung, A. M., and Said, D. G. (2009). The role of limbal stem cells in corneal epithelial maintenance: testing the dogma. Ophthalmology 116, 856–863. doi:10.1016/j.ophtha.2008.12.017
Dua, H. S., Faraj, L. A., Said, D. G., Gray, T., and Lowe, J. (2013). Human corneal anatomy redefined: a novel pre-descemet’s layer (Dua’s Layer). Ophthalmology 120, 1778–1785. doi:10.1016/j.ophtha.2013.01.018
Dua, H. S., Freitas, R., Mohammed, I., Ting, D. S. J., and Said, D. G. (2023). The pre-Descemet’s layer (Dua’s layer, also known as the Dua-Fine layer and the pre-posterior limiting lamina layer): Discovery, characterisation, clinical and surgical applications, and the controversy. Prog. Retin Eye Res. 97, 101161. doi:10.1016/j.preteyeres.2022.101161
Duarte Campos, D. F., Rohde, M., Ross, M., Anvari, P., Blaeser, A., Vogt, M., et al. (2019). Corneal bioprinting utilizing collagen-based bioinks and primary human keratocytes. J. Biomed. Mater. Res. Part A 107, 1945–1953. doi:10.1002/jbm.a.36702
Egrilmez, S., and Yildirim-Theveny, Ş. (2020). Treatment-Resistant Bacterial Keratitis: Challenges and Solutions. Clin. Ophthalmol. 14, 287–297. doi:10.2147/OPTH.S181997
Ehlers, N., Bramsen, T., and Sperling, S. (1975). Applanation tonometry and central corneal thickness. Acta Ophthalmol. (Copenh) 53, 34–43. doi:10.1111/j.1755-3768.1975.tb01135.x
El Zarif, M., Alió, J. L., Alió del Barrio, J. L., De Miguel, M. P., Abdul Jawad, K., and Makdissy, N. (2021). Corneal Stromal Regeneration: A Review of Human Clinical Studies in Keratoconus Treatment. Front. Med. 8, 650724. doi:10.3389/fmed.2021.650724
Espana, E. M., and Birk, D. E. (2020). Composition, Structure and Function of the Corneal Stroma. Exp. Eye Res. 198, 108137. doi:10.1016/j.exer.2020.108137
Fagerholm, P., Lagali, N. S., Carlsson, D. J., Merrett, K., and Griffith, M. (2009). Corneal Regeneration Following Implantation of a Biomimetic Tissue-Engineered Substitute. Clin. Transl. Sci. 2, 162–164. doi:10.1111/j.1752-8062.2008.00083.x
Fagerholm, P., Lagali, N. S., Ong, J. A., Merrett, K., Jackson, W. B., Polarek, J. W., et al. (2014). Stable corneal regeneration four years after implantation of a cell-free recombinant human collagen scaffold. Biomaterials 35, 2420–2427. doi:10.1016/j.biomaterials.2013.11.079
Farghali, H. A., AbdElKader, N. A., AbuBakr, H. O., Ramadan, E. S., Khattab, M. S., Salem, N. Y., et al. (2021). Corneal Ulcer in Dogs and Cats: Novel Clinical Application of Regenerative Therapy Using Subconjunctival Injection of Autologous Platelet-Rich Plasma. Front. Veterinary Sci. 8, 641265. doi:10.3389/fvets.2021.641265
Farrell, R. A., and Hart, R. W. (1969). On the theory of the spatial organization of macromolecules in connective tissue. Bull. Math. Biophys. 31, 727–760. doi:10.1007/BF02477784
Feizi, S., Javadi, M. A., Karimian, F., Bayat, K., Bineshfar, N., and Esfandiari, H. (2023). Penetrating Keratoplasty Versus Deep Anterior Lamellar Keratoplasty for Advanced Stage of Keratoconus. Am. J. Ophthalmol. 248, 107–115. doi:10.1016/j.ajo.2022.11.019
Feng, L., Liu, R., Zhang, X., Li, J., Zhu, L., Li, Z., et al. (2021). Thermo-Gelling Dendronized Chitosans as Biomimetic Scaffolds for Corneal Tissue Engineering. ACS Appl. Mater. Interfaces 13, 49369–49379. doi:10.1021/acsami.1c16087
Fernández-Pérez, J., Kador, K. E., Lynch, A. P., and Ahearne, M. (2020). Characterization of extracellular matrix modified poly(ε-caprolactone) electrospun scaffolds with differing fiber orientations for corneal stroma regeneration. Mater. Sci. Eng. C 108, 110415. doi:10.1016/j.msec.2019.110415
Fischer, T., Hayn, A., and Mierke, C. T. (2019). Fast and reliable advanced two-step pore-size analysis of biomimetic 3D extracellular matrix scaffolds. Sci. Rep. 9, 8352. doi:10.1038/s41598-019-44764-5
Flaxman, S. R., Bourne, R. R. A., Resnikoff, S., Ackland, P., Braithwaite, T., Cicinelli, M. V., et al. (2017). Global causes of blindness and distance vision impairment 1990–2020: a systematic review and meta-analysis. Lancet Glob. Health 5, e1221–e1234. doi:10.1016/S2214-109X(17)30393-5
Foster, J. W., Wahlin, K., Adams, S. M., Birk, D. E., Zack, D. J., and Chakravarti, S. (2017). Cornea organoids from human induced pluripotent stem cells. Sci. Rep. 7, 41286. doi:10.1038/srep41286
Fuest, M., Yam, G. H.-F., Mehta, J. S., and Duarte Campos, D. F. (2020). Prospects and Challenges of Translational Corneal Bioprinting. Bioengineering 7, 71. doi:10.3390/bioengineering7030071
Funderburgh, J. L., Funderburgh, M. L., and Du, Y. (2016). Stem Cells in the Limbal Stroma. Ocul. Surf. 14, 113–120. doi:10.1016/j.jtos.2015.12.006
Germain, L., Carrier, P., Auger, F. A., Salesse, C., and Guérin, S. L. (2000). Can we produce a human corneal equivalent by tissue engineering? Prog. Retin. Eye Res. 19, 497–527. doi:10.1016/S1350-9462(00)00005-7
Ghareeb, A. E., Lako, M., and Figueiredo, F. C. (2020). Recent Advances in Stem Cell Therapy for Limbal Stem Cell Deficiency: A Narrative Review. Ophthalmol. Ther. 9, 809–831. doi:10.1007/s40123-020-00305-2
Ghezzi, C. E., Rnjak-Kovacina, J., and Kaplan, D. L. (2015). Corneal Tissue Engineering: Recent Advances and Future Perspectives. Tissue Eng. Part B Rev. 21, 278–287. doi:10.1089/ten.teb.2014.0397
Goegebuer, A., Ajay, L., Claerhout, I., and Kestelyn, P. (2003). Results of penetrating keratoplasty in syphilitic interstitial keratitis. Bull. De. la Soc. Belge. D’ophtalmologie, 35–39.
Gong, D., Yan, C., Yu, F., Yan, D., Wu, N., Chen, L., et al. (2022). Direct oral mucosal epithelial transplantation supplies stem cells and promotes corneal wound healing to treat refractory persistent corneal epithelial defects. Exp. Eye Res. 215, 108934. doi:10.1016/j.exer.2022.108934
González-Andrades, M., Mata, R., González-Gallardo, M. del C., Medialdea, S., Arias-Santiago, S., Martínez-Atienza, J., et al. (2017). A study protocol for a multicentre randomised clinical trial evaluating the safety and feasibility of a bioengineered human allogeneic nanostructured anterior cornea in patients with advanced corneal trophic ulcers refractory to conventional treatment. BMJ Open 7, e016487. doi:10.1136/bmjopen-2017-016487
Gordon, M. K., Foley, J. W., Birk, D. E., Fitch, J. M., and Linsenmayer, T. F. (1994). Type V collagen and Bowman’s membrane. Quantitation of mRNA in corneal epithelium and stroma. J. Biol. Chem. 269, 24959–24966. doi:10.1016/s0021-9258(17)31483-7
Gouveia, R. M., González-Andrades, E., Cardona, J. C., González-Gallardo, C., Ionescu, A. M., Garzon, I., et al. (2017a). Controlling the 3D architecture of Self-Lifting Auto-generated Tissue Equivalents (SLATEs) for optimized corneal graft composition and stability. Biomaterials 121, 205–219. doi:10.1016/j.biomaterials.2016.12.023
Gouveia, R. M., Koudouna, E., Jester, J., Figueiredo, F., and Connon, C. J. (2017b). Template Curvature Influences Cell Alignment to Create Improved Human Corneal Tissue Equivalents. Adv. Biosyst. 1, e1700135. doi:10.1002/adbi.201700135
Griffith, M., Osborne, R., Munger, R., Xiong, X., Doillon, C., Laycock, N., et al. (2000). Functional Human Corneal Equivalents Constructed from Cell Lines. Sci. (New York, N.Y.) 286, 2169–2172. doi:10.1126/science.286.5447.2169
Grönroos, P., Mörö, A., Puistola, P., Hopia, K., Huuskonen, M., Viheriälä, T., Ilmarinen, T., and Skottman, H. (2024). Bioprinting of human pluripotent stem cell derived corneal endothelial cells with hydrazone crosslinked hyaluronic acid bioink. Stem Cell Res Ther 15, 81. doi:10.1186/s13287-024-03672-w
Guérin, L.-P., Le-Bel, G., Desjardins, P., Couture, C., Gillard, E., Boisselier, É., et al. (2021). The Human Tissue-Engineered Cornea (hTEC): Recent Progress. Int. J. Mol. Sci. 22, 1291. doi:10.3390/ijms22031291
Hady, T. F., Hwang, B., Pusic, A. D., Waworuntu, R. L., Mulligan, M., Ratner, B., et al. (2022). Uniform 40-µm-pore diameter precision templated scaffolds promote a pro-healing host response by extracellular vesicle immune communication. J. Tissue Eng. Regen. Med. 15, 24–36. doi:10.1002/term.3160
Hamedi, E., Boroumand, S., Sigaroodi, F., Rahmani, M., Hosseinzadeh, S., Khani, M.-M., et al. (2024). Incorporation of GelMA/PEGDA into the Decellularized Cornea as a Potential Hybrid Scaffold for in situ Repairing of Deep Anterior Corneal Defects. J. Polym. Environ. 32, 4763–4776. doi:10.1007/s10924-024-03284-8
Hayashi, S., Osawa, T., and Tohyama, K. (2002). Comparative observations on corneas, with special reference to Bowman’s layer and Descemet’s membrane in mammals and amphibians. J. Morphol. 254, 247–258. doi:10.1002/jmor.10030
He, J., Bazan, N. G., and Bazan, H. E. P. (2010). Mapping the entire human corneal nerve architecture. Exp. Eye Res. 91, 513–523. doi:10.1016/j.exer.2010.07.007
Hedbys, B. O., Mishima, S., and Maurice, D. M. (1963). The inbibition pressure of the corneal stroma. Exp. Eye Res. 2, 99–111. doi:10.1016/s0014-4835(63)80001-9
Hernandez, M. J., Gaetani, R., Pieters, V. M., Ng, N. W., Chang, A. E., Martin, T. R., et al. (2018). Decellularized Extracellular Matrix Hydrogels as a Delivery Platform for MicroRNA and Extracellular Vesicle Therapeutics. Adv. Ther. 1, 1800032. doi:10.1002/adtp.201800032
Higa, K., Higuchi, J., Kimoto, R., Miyashita, H., Shimazaki, J., Tsubota, K., et al. (2020). Human corneal limbal organoids maintaining limbal stem cell niche function. Stem Cell Res. 49, 102012. doi:10.1016/j.scr.2020.102012
Hu, X., Wang, M., Chai, G., Zhang, Y., Li, W., Liu, W., et al. (2004). Reconstruction of rabbit corneal stroma using tissue engineering technique. Zhonghua Yan Ke Za Zhi 40, 517–521.
Hu, S., Wang, Z., Jin, C., Chen, Q., Fang, Y., Jin, J., et al. (2022). Human amniotic epithelial cell-derived extracellular vesicles provide an extracellular matrix-based microenvironment for corneal injury repair. J. Tissue Eng. 13, 20417314221122123. doi:10.1177/20417314221122123
Huang, A. J., and Tseng, S. C. (1991). Corneal epithelial wound healing in the absence of limbal epithelium. Invest Ophthalmol. Vis. Sci. 32, 96–105.
Huerva, V., Ascaso, F. J., and Grzybowski, A. (2014). Infliximab for Peripheral Ulcerative Keratitis Treatment. Medicine 93, e176. doi:10.1097/MD.0000000000000176
Hull, S. M., Lindsay, C. D., Brunel, L. G., Shiwarski, D. J., Tashman, J. W., Roth, J. G., et al. (2021). 3D Bioprinting using UNIversal Orthogonal Network (UNION) Bioinks. Adv. Funct. Mater 31, 2007983. doi:10.1002/adfm.202007983
Humphreys, B. D. (2021). Bioprinting better kidney organoids. Nat. Mater. 20, 128–130. doi:10.1038/s41563-020-00881-5
Hutcheon, A. E. K., Zieske, J. D., and Guo, X. (2019). 3D in vitro model for human corneal endothelial cell maturation. Exp. Eye Res. 184, 183–191. doi:10.1016/j.exer.2019.04.003
Ihanamäki, T., Pelliniemi, L. J., and Vuorio, E. (2004). Collagens and collagen-related matrix components in the human and mouse eye. Prog. Retin Eye Res. 23, 403–434. doi:10.1016/j.preteyeres.2004.04.002
Isaacson, A., Swioklo, S., and Connon, C. J. (2018). 3D bioprinting of a corneal stroma equivalent. Exp. Eye Res. 173, 188–193. doi:10.1016/j.exer.2018.05.010
Isidan, A., Liu, S., Li, P., Lashmet, M., Smith, L. J., Hara, H., et al. (2019). Decellularization methods for developing porcine corneal xenografts and future perspectives. Xenotransplantation 26, e12564. doi:10.1111/xen.12564
Iyer, G., Srinivasan, B., Agarwal, S., Talele, D., Rishi, E., Rishi, P., et al. (2018). Keratoprosthesis: Current global scenario and a broad Indian perspective. Indian J. Ophthalmol. 66, 620–629. doi:10.4103/ijo.IJO_22_18
Jacobsen, I. E., Jensen, O. A., and Prause, J. U. (1984). Structure and Composition of Bowman’s Membrane. Acta Ophthalmol. 62, 39–53. doi:10.1111/j.1755-3768.1984.tb06755.x
Jakab, K., Norotte, C., Marga, F., Murphy, K., Vunjak-Novakovic, G., and Forgacs, G. (2010). Tissue engineering by self-assembly and bio-printing of living cells. Biofabrication 2, 022001. doi:10.1088/1758-5082/2/2/022001
Jia, S., Bu, Y., Lau, D.-S. A., Lin, Z., Sun, T., Lu, W. W., et al. (2023). Advances in 3D bioprinting technology for functional corneal reconstruction and regeneration. Front. Bioeng. Biotechnol. 10, 1065460. doi:10.3389/fbioe.2022.1065460
Johnson, D. H., Bourne, W. M., and Campbell, R. J. (1982). The ultrastructure of Descemet’s membrane. I. Changes with age in normal corneas. Arch. Ophthalmol. 100, 1942–1947. doi:10.1001/archopht.1982.01030040922011
Kafarnik, C., Faraj, L. A., Ting, D. S. J., Goh, J. N., Said, D. G., and Dua, H. S. (2023). Ex vivo demonstration of canine corneal pre-Descemet’s anatomy using pneumodissection as for the big bubble technique for deep anterior lamellar keratoplasty. Sci. Rep. 13, 5922. doi:10.1038/s41598-022-24438-5
Kang, Y., Zhang, H., Hu, M., Ma, Y., Chen, P., Zhao, Z., et al. (2020). Alterations in the Ocular Surface Microbiome in Traumatic Corneal Ulcer Patients. Invest Ophthalmol. Vis. Sci. 61, 35. doi:10.1167/iovs.61.6.35
Kim, K.W., Lee, S.J., Park, S.H., and Kim, J.C. (2018a). Ex Vivo Functionality of 3D Bioprinted Corneal Endothelium Engineered with Ribonuclease 5-Overexpressing Human Corneal Endothelial Cells. Adv Healthc Mater 7, e1800398. doi:10.1002/adhm.201800398
Kim, Y. J., Lee, H. J., Ryu, J. S., Kim, Y. H., Jeon, S., Oh, J. Y., et al. (2018b). Prospective Clinical Trial of Corneal Reconstruction With Biomaterial-Free Cultured Oral Mucosal Epithelial Cell Sheets. Cornea 37, 76–83. doi:10.1097/ICO.0000000000001409
Kinoshita, S., Koizumi, N., Ueno, M., Okumura, N., Imai, K., Tanaka, H., et al. (2018). Injection of Cultured Cells with a ROCK Inhibitor for Bullous Keratopathy. N. Engl. J. Med. 378, 995–1003. doi:10.1056/NEJMoa1712770
Kobayashi, T., Kan, K., Nishida, K., Yamato, M., and Okano, T. (2013). Corneal regeneration by transplantation of corneal epithelial cell sheets fabricated with automated cell culture system in rabbit model. Biomaterials 34, 9010–9017. doi:10.1016/j.biomaterials.2013.07.065
Koivusalo, L., Kauppila, M., Samanta, S., Parihar, V. S., Ilmarinen, T., Miettinen, S., et al. (2019). Tissue adhesive hyaluronic acid hydrogels for sutureless stem cell delivery and regeneration of corneal epithelium and stroma. Biomaterials 225, 119516. doi:10.1016/j.biomaterials.2019.119516
Koizumi, N., Okumura, N., Ueno, M., and Kinoshita, S. (2014). New Therapeutic Modality for Corneal Endothelial Disease Using Rho-Associated Kinase Inhibitor Eye Drops. Cornea 33, S25–S31. doi:10.1097/ICO.0000000000000240
Kolli, S., Ahmad, S., Lako, M., and Figueiredo, F. (2010). Successful Clinical Implementation of Corneal Epithelial Stem Cell Therapy for Treatment of Unilateral Limbal Stem Cell Deficiency. Stem Cells 28, 597–610. doi:10.1002/stem.276
Kong, B., Liu, R., Hu, X., Li, M., Zhou, X., Zhao, Y., et al. (2024). Cornea-Inspired Ultrasound-Responsive Adhesive Hydrogel Patches for Keratitis Treatment. Adv. Funct. Mater. 34, 2310544. doi:10.1002/adfm.202310544
Kumar, P., Ebbens, S., and Zhao, X. (2021). Inkjet printing of mammalian cells – Theory and applications. Bioprinting 23, e00157. doi:10.1016/j.bprint.2021.e00157
Kutlehria, S., Cong Dinh, T., Bagde, A., Patel, N., Gebeyehu, A., and Singh, M. (2020). High-throughput 3D bioprinting of corneal stromal equivalents. J Biomed Mater Res B Appl Biomater 108, 2981–2994. doi:10.1002/jbm.b.34628
Laibson, P. R. (1972). Cornea and Sclera. Archives Ophthalmol. 88, 553–574. doi:10.1001/archopht.1972.01000030555018
Laing, R. A., Sandstrom, M. M., Berrospi, A. R., and Leibowitz, H. M. (1976). Changes in the corneal endothelium as a function of age. Exp. Eye Res. 22, 587–594. doi:10.1016/0014-4835(76)90003-8
Lakhundi, S., Siddiqui, R., and Khan, N. A. (2017). Pathogenesis of microbial keratitis. Microb. Pathog. 104, 97–109. doi:10.1016/j.micpath.2016.12.013
Lancaster, M. A., and Huch, M. (2019). Disease modelling in human organoids. Dis. Model Mech. 12, dmm039347. doi:10.1242/dmm.039347
Lancaster, M. A., and Knoblich, J. A. (2014). Organogenesis in a dish: Modeling development and disease using organoid technologies. Science 345, 1247125. doi:10.1126/science.1247125
Lawlor, K. T., Vanslambrouck, J. M., Higgins, J. W., Chambon, A., Bishard, K., Arndt, D., et al. (2021). Cellular extrusion bioprinting improves kidney organoid reproducibility and conformation. Nat. Mater. 20, 260–271. doi:10.1038/s41563-020-00853-9
Levy, S. G., McCartney, A. C. E., Sawada, H., Dopping-Hepenstal, P. J. C., Alexander, R. A., and Moss, J. (1995). Descemet’s membrane in the iridocorneal-endothelial syndrome: Morphology and composition. Exp. Eye Res. 61, 323–333. doi:10.1016/S0014-4835(05)80127-7
Li, H. F., Petroll, W. M., Møller-Pedersen, T., Maurer, J. K., Cavanagh, H. D., and Jester, J. V. (1997). Epithelial and corneal thickness measurements by in vivo confocal microscopy through focusing (CMTF). Curr. Eye Res. 16, 214–221. doi:10.1076/ceyr.16.3.214.15412
Li, F., Carlsson, D., Lohmann, C., Suuronen, E., Vascotto, S., Kobuch, K., et al. (2003). Cellular and nerve regeneration within a biosynthetic extracellular matrix for corneal transplantation. Proc. Natl. Acad. Sci. 100, 15346–15351. doi:10.1073/pnas.2536767100
Li, W., Wang, M., Ma, H., Chapa-Villarreal, F. A., Lobo, A. O., and Zhang, Y. S. (2023a). Stereolithography apparatus and digital light processing-based 3D bioprinting for tissue fabrication. iScience 26, 106039. doi:10.1016/j.isci.2023.106039
Li, X., Zhou, Y., Ma, H., and Wu, M. (2023b). A case report of interstitial keratitis and secondary glaucoma after cataract surgery that may be related to late congenital syphilis. BMC Ophthalmol. 23, 190. doi:10.1186/s12886-023-02852-1
Liebschner, M. A. K. (2004). Biomechanical considerations of animal models used in tissue engineering of bone. Biomaterials 25, 1697–1714. doi:10.1016/s0142-9612(03)00515-5
Liu, W., Merrett, K., Griffith, M., Fagerholm, P., Dravida, S., Heyne, B., et al. (2008). Recombinant human collagen for tissue engineered corneal substitutes. Biomaterials 29, 1147–1158. doi:10.1016/j.biomaterials.2007.11.011
Liu, H., Chen, Y., Wang, P., Li, B., Wang, W., Su, Y., et al. (2015). Efficacy and Safety of Deep Anterior Lamellar Keratoplasty vs. Penetrating Keratoplasty for Keratoconus: A Meta-Analysis. PLOS ONE 10, e0113332. doi:10.1371/journal.pone.0113332
Liu, X., Li, X., Wu, G., Qi, P., Zhang, Y., Liu, Z., et al. (2022). Umbilical Cord Mesenchymal Stem Cell-Derived Small Extracellular Vesicles Deliver miR-21 to Promote Corneal Epithelial Wound Healing through PTEN/PI3K/Akt Pathway. Stem Cells Int. 2022, 1–15. doi:10.1155/2022/1252557
Lobo, E. P., Delic, N. C., Richardson, A., Raviraj, V., Halliday, G. M., Di Girolamo, N., et al. (2016). Self-organized centripetal movement of corneal epithelium in the absence of external cues. Nat. Commun. 7, 12388. doi:10.1038/ncomms12388
Lu, C., and Le, Q. (2024). Advances in Organoid Technology: A Focus on Corneal Limbal Organoids. Stem Cell Rev. Rep. 20, 1227–1235. doi:10.1007/s12015-024-10706-6
Mahdavi, S. S., Abdekhodaie, M. J., Mashayekhan, S., Baradaran-Rafii, A., and Djalilian, A. R. (2020a). Bioengineering Approaches for Corneal Regenerative Medicine. Tissue Eng. Regen. Med. 17, 567–593. doi:10.1007/s13770-020-00262-8
Mahdavi, s. S., Abdekhodaie, M., Kumar, H., Mashayekhan, S., Baradaran- Rafii, A., and Kim, K. (2020b). Stereolithography 3D Bioprinting Method for Fabrication of Human Corneal Stroma Equivalent. Annals of Biomedical Engineering 48, 1955–1970. doi:10.1007/s10439-020-02537-6
Mannis, M. J., and Krachmer, J. H. (1981). Keratoplasty: a historical perspective. Surv. Ophthalmol. 25, 333–338. doi:10.1016/0039-6257(81)90159-4
Marino, G. K., Santhiago, M. R., Santhanam, A., Torricelli, A. A. M., and Wilson, S. E. (2017). Regeneration of Defective Epithelial Basement Membrane and Restoration of Corneal Transparency After Photorefractive Keratectomy. J. Refract. Surg. 33, 337–346. doi:10.3928/1081597X-20170126-02
Marshall, G. E., Konstas, A. G., and Lee, W. R. (1991). Immunogold fine structural localization of extracellular matrix components in aged human cornea. Graefe’s Arch. Clin. Exp. Ophthalmol. 229, 157–163. doi:10.1007/BF00170550
Masterson, C. H., Tabuchi, A., Hogan, G., Fitzpatrick, G., Kerrigan, S. W., Jerkic, M., et al. (2021). Intra-vital imaging of mesenchymal stromal cell kinetics in the pulmonary vasculature during infection. Sci. Rep. 11, 5265. doi:10.1038/s41598-021-83894-7
Mataftsi, A., Bourtoulamaiou, A., Anastasilakis, K., Ziakas, N. G., and Dimitrakos, S. A. (2013). Management of bullous keratopathy-related ulcer with autologous serum. Eye Contact Lens 39, e19–e20. doi:10.1097/ICL.0b013e31825f6386
Matai, I., Kaur, G., Seyedsalehi, A., McClinton, A., and Laurencin, C. T. (2020). Progress in 3D bioprinting technology for tissue/organ regenerative engineering. Biomaterials 226, 119536. doi:10.1016/j.biomaterials.2019.119536
Maurice, D. M. (1957). The structure and transparency of the cornea. J. Physiol. 136, 263–286. doi:10.1113/jphysiol.1957.sp005758
Mayes, K. R., and Hodson, S. (1978). Some effects of hydrostatic pressure on corneal hydration during specular microscopy. Exp. Eye Res. 26, 141–145. doi:10.1016/0014-4835(78)90111-2
McKay, T. B., Karamichos, D., Hutcheon, A. E. K., Guo, X., and Zieske, J. D. (2019). Corneal Epithelial–Stromal Fibroblast Constructs to Study Cell–Cell Communication in vitro. Bioengineering 6, 110. doi:10.3390/bioengineering6040110
McKay, T. B., Guo, X., Hutcheon, A. E. K., Karamichos, D., and Ciolino, J. B. (2020). Methods for Investigating Corneal Cell Interactions and Extracellular Vesicles in vitro. Curr. Protoc. Cell Biol. 89, e114. doi:10.1002/cpcb.114
Meek, K. M., and Knupp, C. (2015). Corneal structure and transparency. Prog. Retin Eye Res. 49, 1–16. doi:10.1016/j.preteyeres.2015.07.001
Merindano, M. D., Costa, J., Canals, M., Potau, J. M., and Ruano, D. (2002). A comparative study of Bowman’s layer in some mammals: Relationships with other constituent corneal structures. Eur. J. Anat., 133–139.
Merrett, K., Fagerholm, P., McLaughlin, C. R., Dravida, S., Lagali, N., Shinozaki, N., et al. (2008). Tissue-Engineered Recombinant Human Collagen-Based Corneal Substitutes for Implantation: Performance of Type I versus Type III Collagen. Investigative Ophthalmol. and Vis. Sci. 49, 3887–3894. doi:10.1167/iovs.07-1348
Meyer-Blazejewska, E. A., Kruse, F. E., Bitterer, K., Meyer, C., Hofmann-Rummelt, C., Wünsch, P. H., et al. (2010). Preservation of the limbal stem cell phenotype by appropriate culture techniques. Invest Ophthalmol. Vis. Sci. 51, 765–774. doi:10.1167/iovs.09-4109
Michelacci, Y. M. (2003). Collagens and proteoglycans of the corneal extracellular matrix. Braz J. Med. Biol. Res. 36, 1037–1046. doi:10.1590/S0100-879X2003000800009
Mohan, R. R., Liang, Q., Kim, W.-J., Helena, M. C., Baerveldt, F., and Wilson, S. E. (1997). Apoptosis in the Cornea: Further Characterization of Fas/Fas Ligand System. Exp. Eye Res. 65, 575–589. doi:10.1006/exer.1997.0371
Mörö, A., Samanta, S., Honkamäki, L., Rangasami, V. K., Puistola, P., Kauppila, M., et al. (2022). Hyaluronic acid based next generation bioink for 3D bioprinting of human stem cell derived corneal stromal model with innervation. Biofabrication 15, 015020. doi:10.1088/1758-5090/acab34
Mousaei Ghasroldasht, M., Seok, J., Park, H.-S., Liakath Ali, F. B., and Al-Hendy, A. (2022). Stem Cell Therapy: From Idea to Clinical Practice. Int. J. Mol. Sci. 23, 2850. doi:10.3390/ijms23052850
Mukherjee, P., Roy, S., Ghosh, D., and Nandi, S. K. (2022). Role of animal models in biomedical research: a review. Laboratory Animal Res. 38, 18. doi:10.1186/s42826-022-00128-1
Nakano, T., Ando, S., Takata, N., Kawada, M., Muguruma, K., Sekiguchi, K., et al. (2012). Self-Formation of Optic Cups and Storable Stratified Neural Retina from Human ESCs. Cell Stem Cell 10, 771–785. doi:10.1016/j.stem.2012.05.009
Nishida, K., Yamato, M., Hayashida, Y., Watanabe, K., Yamamoto, K., Adachi, E., et al. (2004). Corneal Reconstruction with Tissue-Engineered Cell Sheets Composed of Autologous Oral Mucosal Epithelium. N. Engl. J. Med. 351, 1187–1196. doi:10.1056/NEJMoa040455
Niu, G., Choi, J.-S., Wang, Z., Skardal, A., Giegengack, M., and Soker, S. (2014). Heparin-modified gelatin scaffolds for human corneal endothelial cell transplantation. Biomaterials 35, 4005–4014. doi:10.1016/j.biomaterials.2014.01.033
Nosrati, H., Ashrafi-Dehkordi, K., Alizadeh, Z., Sanami, S., and Banitalebi-Dehkordi, M. (2020). Biopolymer-based scaffolds for corneal stromal regeneration: A review. Polim. Med. 50, 57–64. doi:10.17219/pim/127653
Nune, Dr. M., Kumaraswamy, P., Krishnan, U., and Sethuraman, S. (2013). Self-Assembling Peptide Nanofibrous Scaffolds for Tissue Engineering: Novel Approaches and Strategies for Effective Functional Regeneration. Curr. protein and peptide Sci. 14, 70–84. doi:10.2174/1389203711314010010
Nuzzi, R., Buono, L., Scalabrin, S., De Iuliis, M., and Bussolati, B. (2021). Effect of Stem Cell-Derived Extracellular Vesicles on Damaged Human Corneal Endothelial Cells. Stem Cells Int. 2021, 1–12. doi:10.1155/2021/6644463
Oh, J. Y., Kim, E., Yun, Y. I., and Lee, R. H. (2021). Mesenchymal stromal cells for corneal transplantation: Literature review and suggestions for successful clinical trials. Ocular Surf. 20, 185–194. doi:10.1016/j.jtos.2021.02.002
Oliva, J., Bardag-Gorce, F., and Niihara, Y. (2020). Clinical Trials of Limbal Stem Cell Deficiency Treated with Oral Mucosal Epithelial Cells. Int. J. Mol. Sci. 21, 411. doi:10.3390/ijms21020411
Osidak, E. O., Kozhukhov, V. I., Osidak, M. S., and Domogatsky, S. P. (2020). Collagen as Bioink for Bioprinting: A Comprehensive Review. Int. J. Bioprint 6, 270. doi:10.18063/ijb.v6i3.270
Ovsianikov, A., Khademhosseini, A., and Mironov, V. (2018). The Synergy of Scaffold-Based and Scaffold-Free Tissue Engineering Strategies. Trends Biotechnol. 36, 348–357. doi:10.1016/j.tibtech.2018.01.005
Pagano, L., Gadhvi, K. A., Borroni, D., Iselin, K. C., Vinciguerra, R., Tzamalis, A., et al. (2020). Bilateral Keratoconus Progression: Immediate Versus Delayed Sequential Bilateral Corneal Cross-linking. J. Refract. Surg. 36, 552–556. doi:10.3928/1081597X-20200629-01
Palioura, S., Henry, C. R., Amescua, G., and Alfonso, E. C. (2016). Role of steroids in the treatment of bacterial keratitis. Clin. Ophthalmol. 10, 179–186. doi:10.2147/OPTH.S80411
Parekh, M., Ramos, T., O’Sullivan, F., Meleady, P., Ferrari, S., Ponzin, D., et al. (2021). Human corneal endothelial cells from older donors can be cultured and passaged on cell-derived extracellular matrix. Acta Ophthalmol. 99, e512–e522. doi:10.1111/aos.14614
Paton, R. (1954). Corneal Transplantation: A Review of 365 Operations. A.M.A. Archives Ophthalmol. 52, 871–916. doi:10.1001/archopht.1954.00920050877009
Pellegrini, G., O, G., P, P., A, L., S, B., P, R., et al. (1999). Location and clonal analysis of stem cells and their differentiated progeny in the human ocular surface. J. cell Biol. 145, 769–782. doi:10.1083/jcb.145.4.769
Pires, R. T., Tseng, S. C., Prabhasawat, P., Puangsricharern, V., Maskin, S. L., Kim, J. C., et al. (1999). Amnio1tic membrane transplantation for symptomatic bullous keratopathy. Arch. Ophthalmol. 117 (10), 1291–1297. doi:10.1001/archopht.117.10.1291
Piñero, D. P., Plaza Puche, A. B., and Alió, J. L. (2008). Corneal diameter measurements by corneal topography and angle-to-angle measurements by optical coherence tomography: Evaluation of equivalence. J. Cataract and Refract. Surg. 34, 126–131. doi:10.1016/j.jcrs.2007.10.010
Polisetti, N., Schmid, A., Schlötzer-Schrehardt, U., Maier, P., Lang, S. J., Steinberg, T., et al. (2021). A decellularized human corneal scaffold for anterior corneal surface reconstruction. Sci. Rep. 11, 2992. doi:10.1038/s41598-021-82678-3
Ponce Márquez, S., Martínez, V. S., McIntosh Ambrose, W., Wang, J., Gantxegui, N. G., Schein, O., et al. (2009). Decellularization of bovine corneas for tissue engineering applications. Acta Biomater. 5, 1839–1847. doi:10.1016/j.actbio.2009.02.011
Price, M. O., Mehta, J. S., Jurkunas, U. V., and Price, F. W. (2021). Corneal endothelial dysfunction: Evolving understanding and treatment options. Prog. Retin. Eye Res. 82, 100904. doi:10.1016/j.preteyeres.2020.100904
Puri, S., Coulson-Thomas, Y. M., Gesteira, T. F., and Coulson-Thomas, V. J. (2020). Distribution and Function of Glycosaminoglycans and Proteoglycans in the Development, Homeostasis and Pathology of the Ocular Surface. Front. Cell Dev. Biol. 8, 731. doi:10.3389/fcell.2020.00731
Rama, P., Bonini, S., Lambiase, A., Golisano, O., Paterna, P., De Luca, M., et al. (2001). Autologous fibrin-cultured limbal stem cells permanently restore the corneal surface of patients with total limbal stem cell deficiency. Transplantation 72, 1478–1485. doi:10.1097/00007890-200111150-00002
Ramírez, B. E., Sánchez, A., Herreras, J. M., Fernández, I., García-Sancho, J., Nieto-Miguel, T., et al. (2015). Stem Cell Therapy for Corneal Epithelium Regeneration following Good Manufacturing and Clinical Procedures. BioMed Res. Int. 2015, 1–19. doi:10.1155/2015/408495
Reinstein, D. Z., Archer, T. J., Gobbe, M., Silverman, R. H., and Coleman, D. J. (2008). Epithelial Thickness in the Normal Cornea: Three-dimensional Display With Artemis Very High-frequency Digital Ultrasound. J. Refract Surg. 24, 571–581. doi:10.3928/1081597x-20080601-05
Ren, Y., Yang, X., Ma, Z., Sun, X., Zhang, Y., Li, W., et al. (2021). Developments and Opportunities for 3D Bioprinted Organoids. Int. J. Bioprint 7, 364. doi:10.18063/ijb.v7i3.364
Riazuddin, S. A., Vithana, E. N., Seet, L.-F., Liu, Y., Al-Saif, A., Koh, L. W., et al. (2010). Missense mutations in the sodium borate cotransporter SLC4A11 cause late-onset Fuchs corneal dystrophya. Hum. Mutat. 31, 1261–1268. doi:10.1002/humu.21356
Ribitsch, I., Baptista, P. M., Lange-Consiglio, A., Melotti, L., Patruno, M., Jenner, F., et al. (2020). Large Animal Models in Regenerative Medicine and Tissue Engineering: To Do or Not to Do. Front. Bioeng. Biotechnol. 8, 972. doi:10.3389/fbioe.2020.00972
Rinawati, M., Triastuti, J., and Pursetyo, K. T. (2018). Characterization of elasticity and hydration of composite hydrogel based on collagen-iota carrageenan as a corneal tissue engineering. IOP Conf. Ser. Earth Environ. Sci. 137, 012042. doi:10.1088/1755-1315/137/1/012042
Rocha-de-Lossada, C., Rachwani-Anil, R., Borroni, D., Sánchez-González, J.-M., Esteves-Marques, R., Soler-Ferrández, F.-L., et al. (2021). New Horizons in the Treatment of Corneal Endothelial Dysfunction. J. Ophthalmol. 2021, 1–11. doi:10.1155/2021/6644114
Saccu, G., Menchise, V., Gai, C., Bertolin, M., Ferrari, S., Giordano, C., et al. (2022). Bone Marrow Mesenchymal Stromal/Stem Cell-Derived Extracellular Vesicles Promote Corneal Wound Repair by Regulating Inflammation and Angiogenesis. Cells 11, 3892. doi:10.3390/cells11233892
Sahi, A. K., Varshney, N., Poddar, S., Gundu, S., and Mahto, S. K. (2021). Fabrication and Characterization of Silk Fibroin-Based Nanofibrous Scaffolds Supplemented with Gelatin for Corneal Tissue Engineering. Cells Tissues Organs 210, 173–194. doi:10.1159/000515946
Saikia, P., Medeiros, C. S., Thangavadivel, S., and Wilson, S. E. (2018). Basement membranes in the cornea and other organs that commonly develop fibrosis. Cell Tissue Res. 374, 439–453. doi:10.1007/s00441-018-2934-7
Santra, M., Weng, L., Louise Geary, M., Yang, tiangbing, Jhanji, V., and Yam, G. (2022). Corneal stromal stem cell-derived extracellular vesicles transport TGF β3 to mediate anti-fibrosis effect on corneal scarring. Investigative Ophthalmol. and Vis. Sci. 63, 100–A0198.
Santra, M., Geary, M. L., Jhanji, V., Funderburgh, M., and Yam, G. (2024). A Cell-free Treatment with Quality-Controlled Corneal Stromal Stem Cell-Derived Extracellular Vesicles on Corneal Opacities. Investigative Ophthalmol. and Vis. Sci. 65, 518.
Sayers, Z., Koch, M. H., Whitburn, S. B., Meek, K. M., Elliott, G. F., and Harmsen, A. (1982). Synchrotron x-ray diffraction study of corneal stroma. J. Mol. Biol. 160, 593–607. doi:10.1016/0022-2836(82)90317-5
Schmedt, T., Silva, M. M., Ziaei, A., and Jurkunas, U. (2012). Molecular bases of corneal endothelial dystrophies. Exp. Eye Res. 95, 24–34. doi:10.1016/j.exer.2011.08.002
Schmieg, B., Gretzinger, S., Schuhmann, S., Guthausen, G., and Hubbuch, J. (2022). Magnetic resonance imaging as a tool for quality control in extrusion-based bioprinting. Biotechnol. J. 17, 2100336. doi:10.1002/biot.202100336
Schwartz, G. S., Harrison, A. R., and Holland, E. J. (1998). Etiology of immune stromal (interstitial) keratitis. Cornea 17, 278–281. doi:10.1097/00003226-199805000-00007
Seo, J. S., Tumursukh, N.-E., Choi, J. H., Song, Y., Jeon, G., Kim, N. E., et al. (2023). Modified gellan gum-based hydrogel with enhanced mechanical properties for application as a cell carrier for cornea endothelial cells. Int. J. Biol. Macromol. 236, 123878. doi:10.1016/j.ijbiomac.2023.123878
Shah, A., Brugnano, J., Sun, S., Vase, A., and Orwin, E. (2008). The Development of a Tissue-Engineered Cornea: Biomaterials and Culture Methods. Pediatr. Res. 63, 535–544. doi:10.1203/PDR.0b013e31816bdf54
Sharma, N., Bagga, B., Singhal, D., Nagpal, R., Kate, A., Saluja, G., et al. (2022). Fungal keratitis: A review of clinical presentations, treatment strategies and outcomes. Ocular Surf. 24, 22–30. doi:10.1016/j.jtos.2021.12.001
Shelmerdine, S. C., Simcock, I. C., Hutchinson, J. C., Aughwane, R., Melbourne, A., Nikitichev, D. I., et al. (2018). 3D printing from microfocus computed tomography (micro-CT) in human specimens: education and future implications. Br. J. Radiology 91, 20180306. doi:10.1259/bjr.20180306
Shi, Y., Su, J., Roberts, A. I., Shou, P., Rabson, A. B., and Ren, G. (2012). How mesenchymal stem cells interact with tissue immune responses. Trends Immunol. 33, 136–143. doi:10.1016/j.it.2011.11.004
Shi, H., Zhou, J., Wang, Y., Zhu, Y., Lin, D., Lei, L., et al. (2022). A Rapid Corneal Healing Microneedle for Efficient Ocular Drug Delivery. Small 18, 2104657. doi:10.1002/smll.202104657
Shojaati, G., Khandaker, I., Funderburgh, M. L., Mann, M. M., Basu, R., Stolz, D. B., et al. (2019). Mesenchymal Stem Cells Reduce Corneal Fibrosis and Inflammation via Extracellular Vesicle-Mediated Delivery of miRNA. Stem Cells Transl. Med. 8, 1192–1201. doi:10.1002/sctm.18-0297
Siu, G. D. J.-Y., Young, A. L., and Jhanji, V. (2014). Alternatives to corneal transplantation for the management of bullous keratopathy. Curr. Opin. Ophthalmol. 25, 347–352. doi:10.1097/ICU.0000000000000062
Song, Y., Hua, S., Sayyar, S., Chen, Z., Chung, J., Liu, X., Yue, Z., Angus, C., Filippi, B., Beirne, S., Wallace, G., Sutton, G., and You, J. (2022). Corneal bioprinting using a high concentration pure collagen I transparent bioink. Bioprinting 28, e00235. doi:10.1016/j.bprint.2022.e00235
Sorkio, A., Koch, L., Koivusalo, L., Deiwick, A., Miettinen, S., Chichkov, B., et al. (2018). Human stem cell based corneal tissue mimicking structures using laser-assisted 3D bioprinting and functional bioinks. Biomaterials 171, 57–71. doi:10.1016/j.biomaterials.2018.04.034
Sotozono, C., Inatomi, T., Nakamura, T., Ueta, M., Imai, K., Fukuoka, H., et al. (2020). Oral Mucosal Epithelial Transplantation and Limbal-Rigid Contact Lens: A Therapeutic Modality for the Treatment of Severe Ocular Surface Disorders. Cornea 39, S19–S27. doi:10.1097/ICO.0000000000002566
Spadea, L., Maraone, G., Verboschi, F., Vingolo, E. M., and Tognetto, D. (2016). Effect of corneal light scatter on vision: a review of the literature. Int. J. Ophthalmol. 9, 459–464. doi:10.18240/ijo.2016.03.24
Sridhar, M. S. (2018). Anatomy of cornea and ocular surface. Indian J. Ophthalmol. 66, 190–194. doi:10.4103/ijo.IJO_646_17
Strinkovsky, L., Havkin, E., Shalom-Feuerstein, R., and Savir, Y. (2021). Spatial correlations constrain cellular lifespan and pattern formation in corneal epithelium homeostasis. eLife 10, e56404. doi:10.7554/eLife.56404
Su, Y., Li, G., Xu, J., Zheng, J., Jiao, J., Zhang, J., et al. (2022). Immune-related keratitis is a rare complication associated with nivolumab treatment in a patient with advanced colorectal cancer: A case report. Front. Oncol. 12, 1021713. doi:10.3389/fonc.2022.1021713
Susaimanickam, P. J., Maddileti, S., Pulimamidi, V. K., Boyinpally, S. R., Naik, R. R., Naik, M. N., et al. (2017). Generating minicorneal organoids from human induced pluripotent stem cells. Development 144, 2338–2351. doi:10.1242/dev.143040
Suuronen, E. J., Nakamura, M., Watsky, M. A., Stys, P. K., Müller, L. J., Munger, R., et al. (2004). Innervated human corneal equivalents as in vitro models for nerve-target cell interactions. FASEB J. 18, 170–172. doi:10.1096/fj.03-0043fje
Syed-Picard, F. N., Du, Y., Hertsenberg, A. J., Palchesko, R., Funderburgh, M. L., Feinberg, A. W., et al. (2018). Scaffold-free tissue engineering of functional corneal stromal tissue. J. Tissue Eng. Regen. Med. 12, 59–69. doi:10.1002/term.2363
Tang, Q., Lu, B., He, J., Chen, X., Fu, Q., Han, H., et al. (2022). Exosomes-loaded thermosensitive hydrogels for corneal epithelium and stroma regeneration. Biomaterials 280, 121320. doi:10.1016/j.biomaterials.2021.121320
Tao, H., Chen, X., Cao, H., Zheng, L., Li, Q., Zhang, K., et al. (2019). Mesenchymal Stem Cell-Derived Extracellular Vesicles for Corneal Wound Repair. Stem Cells Int. 2019, 1–9. doi:10.1155/2019/5738510
Tati, V., Muthukumar V, S., and Shukla, S. (2024). Mesenchymal vs. epithelial extracellular vesicles in corneal epithelial repair, apoptosis, and immunomodulation: An in vitro study. Exp. Eye Res. 247, 110027. doi:10.1016/j.exer.2024.110027
Thalakiriyawa, D. S., and Dissanayaka, W. L. (2024). Advances in Regenerative Dentistry Approaches: An Update. Int. Dent. J. 74, 25–34. doi:10.1016/j.identj.2023.07.008
Thoft, R. A., and Friend, J. (1983). The X, Y, Z hypothesis of corneal epithelial maintenance. Investigative Ophthalmol. and Vis. Sci. 24, 1442–1443.
Tong, C. M., van Dijk, K., and Melles, G. R. J. (2019). Update on Bowman layer transplantation. Curr. Opin. Ophthalmol. 30, 249–255. doi:10.1097/ICU.0000000000000570
Torres-Netto, E. A., Hafezi, F., Spiru, B., Gilardoni, F., Hafezi, N. L., Gomes, J. A. P., et al. (2021). Contribution of Bowman layer to corneal biomechanics. J. Cataract and Refract. Surg. 47, 927–932. doi:10.1097/j.jcrs.0000000000000543
Torricelli, A. A. M., Marino, G. K., Santhanam, A., Wu, J., Singh, A., and Wilson, S. E. (2015). Epithelial basement membrane proteins perlecan and nidogen-2 are up-regulated in stromal cells after epithelial injury in human corneas. Exp. Eye Res. 134, 33–38. doi:10.1016/j.exer.2015.03.016
Tran, T. M., and Hou, J. H. (2023). Clinical applications of bioengineered tissue-cellular products for management of corneal diseases. Curr. Opin. Ophthalmol. 34, 311–323. doi:10.1097/ICU.0000000000000961
Tseng, S. H., Yang, C.-Y., Li, J.-H., Jeng, Y.-M., Ranasinghesagara, J. C., and Venugopalan, V. (2022). Corneal transparency and scleral opacity arises from the nanoarchitecture of the constituent collagen fibrils. Biomed. Opt. Express 13, 1485–1496. doi:10.1364/BOE.444832
Tu, E. Y. (2022). “Interstitial Keratitis,” in Albert and jakobiec’s principles and practice of ophthalmology. Editors D. M. Albert, J. W. Miller, D. T. Azar, and L. H. Young (Cham: Springer International Publishing), 361–375. doi:10.1007/978-3-030-42634-7_328
Tuli, S. S., Schultz, G. S., and Downer, D. M. (2007). Science and Strategy for Preventing and Managing Corneal Ulceration. Ocular Surf. 5, 23–39. doi:10.1016/S1542-0124(12)70050-2
Venugopal, B., Shenoy, S. J., Mohan, S., Anil Kumar, P. R., and Kumary, T. V. (2020). Bioengineered corneal epithelial cell sheet from mesenchymal stem cells-A functional alternative to limbal stem cells for ocular surface reconstruction. J. Biomed. Mater Res. B Appl. Biomater. 108, 1033–1045. doi:10.1002/jbm.b.34455
Vingopoulos, F., Karagiotis, T., and Palioura, S. (2020). Bilateral interstitial keratitis, erythema nodosum and atrial fibrillation as presenting signs of polyarteritis nodosa. Am. J. Ophthalmol. Case Rep. 18, 100619. doi:10.1016/j.ajoc.2020.100619
Vrehen, A. F., Rutten, M. G. T. A., and Dankers, P. Y. W. (2023). Development of a Fully Synthetic Corneal Stromal Construct via Supramolecular Hydrogel Engineering. Adv. Healthc. Mater. 12, 2301392. doi:10.1002/adhm.202301392
Wan, X., Gu, J., Zhou, X., Le, Q., Wang, J., Xin, C., et al. (2024). Establishment of human corneal epithelial organoids for ex vivo modelling dry eye disease. Cell Prolif., e13704. doi:10.1111/cpr.13704
Wang, F., Shi, W., Li, H., Wang, H., Sun, D., Zhao, L., et al. (2020). Decellularized porcine cornea-derived hydrogels for the regeneration of epithelium and stroma in focal corneal defects. Ocular Surf. 18, 748–760. doi:10.1016/j.jtos.2020.07.020
Wang, R., Deng, S., Wu, Y., Wei, H., Jing, G., Zhang, B., Liu, F., Tian, H., Chen, X., and Tian, W. (2022a). Remodelling 3D printed GelMA-HA corneal scaffolds by cornea stromal cells. Colloid and Interface Science Communications 49, 100632. doi:10.1016/j.colcom.2022.100632
Wang, Y., Wang, J., Ji, Z., Yan, W., Zhao, H., Huang, W., et al. (2022b). Application of Bioprinting in Ophthalmology. Int. J. Bioprint 8, 552. doi:10.18063/ijb.v8i2.552
Wang, B., Guo, H., Han, Z., Wu, S., Liu, J., Lin, Z., et al. (2023a). NRG1 Regulates Proliferation, Migration and Differentiation of Human Limbal Epithelial Stem Cells. Curr. Issues Mol. Biol. 45, 10121–10130. doi:10.3390/cimb45120632
Wang, G., Zeng, L., Gong, C., Gong, X., Zhu, T., and Zhu, Y. (2023b). Extracellular vesicles derived from mouse adipose-derived mesenchymal stem cells promote diabetic corneal epithelial wound healing through NGF/TrkA pathway activation involving dendritic cells. Exp. Eye Res. 231, 109484. doi:10.1016/j.exer.2023.109484
Wang, L., Li, A., Zhang, D., Zhang, M., Ma, L., Li, Y., et al. (2023c). Injectable double-network hydrogel for corneal repair. Chem. Eng. J. 455, 140698. doi:10.1016/j.cej.2022.140698
Wang, L., Wang, X., Chen, Q., Wei, Z., Xu, X., Han, D., et al. (2023d). MicroRNAs of extracellular vesicles derived from mesenchymal stromal cells alleviate inflammation in dry eye disease by targeting the IRAK1/TAB2/NF-κB pathway. Ocular Surf. 28, 131–140. doi:10.1016/j.jtos.2023.03.002
Wang, L., Xu, X., Chen, Q., Wei, Y., Wei, Z., Jin, Z.-B., et al. (2023e). Extracellular Vesicle MicroRNAs From Corneal Stromal Stem Cell Enhance Stemness of Limbal Epithelial Stem Cells by Targeting the Notch Pathway. Investigative Ophthalmol. and Vis. Sci. 64, 42. doi:10.1167/iovs.64.12.42
Wang, M., Li, Y., Wang, H., Li, M., Wang, X., Liu, R., et al. (2023f). Corneal regeneration strategies: From stem cell therapy to tissue engineered stem cell scaffolds. Biomed. and Pharmacother. 165, 115206. doi:10.1016/j.biopha.2023.115206
Wang, J., Wang, X., Ma, X., Pan, T., Fu, Q., Li, X., et al. (2024). Mesenchymal Stem Cells Derived Extracellular Vesicles Modified-Plga Electrospinning Nanofibrous Scaffolds for Corneal and Retinal Repair. Mater. Des., 113389. doi:10.2139/ssrn.4813617
Warmflash, A., Sorre, B., Etoc, F., Siggia, E. D., and Brivanlou, A. H. (2014). A method to recapitulate early embryonic spatial patterning in human embryonic stem cells. Nat. Methods 11, 847–854. doi:10.1038/nmeth.3016
Weiss, J. S., Møller, H. U., Aldave, A. J., Seitz, B., Bredrup, C., Kivelä, T., et al. (2015). IC3D classification of corneal dystrophies--edition 2. Cornea 34, 117–159. doi:10.1097/ICO.0000000000000307
Worthington, C. R., and Inouye, H. (1985). X-ray diffraction study of the cornea. Int. J. Biol. Macromol. 7, 2–8. doi:10.1016/0141-8130(85)90057-1
Wu, Z., Su, X., Xu, Y., Kong, B., Sun, W., and Mi, S. (2016). Bioprinting three-dimensional cell-laden tissue constructs with controllable degradation. Sci Rep 6, 24474. doi:10.1038/srep24474
Wu, Z., Kong, B., Liu, R., Sun, W., and Mi, S. (2018). Engineering of Corneal Tissue through an Aligned PVA/Collagen Composite Nanofibrous Electrospun Scaffold. Nanomaterials 8, 124. doi:10.3390/nano8020124
Wulle, K. G., and Richter, J. (1978). Electron microscopy of the early embryonic development of the human corneal epithelium. Albr. Graefes Arch. für Klin. Exp. Ophthalmol. 209, 39–49. doi:10.1007/bf00419161
Xeroudaki, M., Rafat, M., Moustardas, P., Mukwaya, A., Tabe, S., Bellisario, M., et al. (2023). A double-crosslinked nanocellulose-reinforced dexamethasone-loaded collagen hydrogel for corneal application and sustained anti-inflammatory activity. Acta Biomater. 172, 234–248. doi:10.1016/j.actbio.2023.10.020
Xiao, X., Pan, S., Liu, X., Zhu, X., Connon, C. J., Wu, J., et al. (2014). In vivo study of the biocompatibility of a novel compressed collagen hydrogel scaffold for artificial corneas. J. Biomed. Mater. Res. Part A 102, 1782–1787. doi:10.1002/jbm.a.34848
Yang, H., Yang, K.-H., Narayan, R. J., and Ma, S. (2021). Laser-based bioprinting for multilayer cell patterning in tissue engineering and cancer research. Essays Biochem. 65, 409–416. doi:10.1042/EBC20200093
Yao, J., Ma, J., Zhao, J., Qi, P., Li, M., Lin, L., et al. (2020). Corneal hydration assessment indicator based on terahertz time domain spectroscopy. Biomed. Opt. Express, BOE 11, 2073–2084. doi:10.1364/BOE.387826
Yazdani, M., Shahdadfar, A., Jackson, C. J., and Utheim, T. P. (2019). Hyaluronan-Based Hydrogel Scaffolds for Limbal Stem Cell Transplantation: A Review. Cells 8, 245. doi:10.3390/cells8030245
Yeung, V., Zhang, T. C., Yuan, L., Parekh, M., Cortinas, J. A., Delavogia, E., et al. (2022). Extracellular Vesicles Secreted by Corneal Myofibroblasts Promote Corneal Epithelial Cell Migration. Int. J. Mol. Sci. 23, 3136. doi:10.3390/ijms23063136
Yi, S., Kim, J., Kim, M. J., Yae, C. G., Kim, K. H., and Kim, H. K. (2024). Development of human amniotic epithelial cell-derived extracellular vesicles as cell-free therapy for dry eye disease. Ocular Surf. 34, 370–380. doi:10.1016/j.jtos.2024.09.006
Yoon, J. J., Ismail, S., and Sherwin, T. (2014). Limbal stem cells: Central concepts of corneal epithelial homeostasis. World J. Stem Cells 6, 391–403. doi:10.4252/wjsc.v6.i4.391
Yoshida, M., Yokokura, S., Hariya, T., Kobayashi, W., Hashimoto, K., and Nakazawa, T. (2023). Ripasudil Eyedrops Ameliorated Bullous Keratopathy Complicated with Cytomegalovirus Corneal Endotheliitis: A Case Report. Ocular Immunol. Inflamm. 31, 207–210. doi:10.1080/09273948.2021.1988114
Yu, C., Chen, P., Xu, J., Liu, Y., Li, H., Wang, L., et al. (2020). hADSCs derived extracellular vesicles inhibit NLRP3inflammasome activation and dry eye. Sci. Rep. 10, 14521. doi:10.1038/s41598-020-71337-8
Yuan, X., Marcano, D. C., Shin, C. S., Hua, X., Isenhart, L. C., Pflugfelder, S. C., et al. (2015). Ocular Drug Delivery Nanowafer with Enhanced Therapeutic Efficacy. ACS Nano 9, 1749–1758. doi:10.1021/nn506599f
Yusoff, N. Z. binte M., Riau, A. K., Yam, G. H. F., binte Halim, N. S. H., and Mehta, J. S. (2022). Isolation and Propagation of Human Corneal Stromal Keratocytes for Tissue Engineering and Cell Therapy. Cells 11, 178. doi:10.3390/cells11010178
Zhang, Y. Q., Zhang, W. J., Liu, W., Hu, X. J., Zhou, G. D., Cui, L., et al. (2008). Tissue Engineering of Corneal Stromal Layer with Dermal Fibroblasts: Phenotypic and Functional Switch of Differentiated Cells in Cornea. Tissue Eng. Part A 14, 295–303. doi:10.1089/tea.2007.0200
Zhang, M.-C., Liu, X., Jin, Y., Jiang, D.-L., Wei, X.-S., and Xie, H.-T. (2015). Lamellar Keratoplasty Treatment of Fungal Corneal Ulcers With Acellular Porcine Corneal Stroma. Am. J. Transplant. 15, 1068–1075. doi:10.1111/ajt.13096
Zhang, Z., He, Z., Liang, R., Ma, Y., Huang, W., Jiang, R., et al. (2016). Fabrication of a Micellar Supramolecular Hydrogel for Ocular Drug Delivery. Biomacromolecules 17, 798–807. doi:10.1021/acs.biomac.5b01526
Zhang, Z., Jin, Y., Yin, J., Xu, C., Xiong, R., Christensen, K., et al. (2018). Evaluation of bioink printability for bioprinting applications. Appl. Phys. Rev. 5, 041304. doi:10.1063/1.5053979
Zhang, B., Xue, Q., Hu, H., Yu, M., Gao, L., Luo, Y., Li, Y., Li, J., Ma, L., Yao, Y., and Yang, H. (2019). Integrated 3D bioprinting-based geometry-control strategy for fabricating corneal substitutes. J Zhejiang Univ Sci B 20, 945–959. doi:10.1631/jzus.B1900190
Zhang, M., Yang, F., Han, D., Zhang, S.-Y., Dong, Y., Li, X., Ling, L., Deng, Z., Cao, X., Tian, J., Ye, Q., and Wang, Y. (2023). 3D bioprinting of corneal decellularized extracellular matrix: GelMA composite hydrogel for corneal stroma engineering. Int J Bioprint 9, 774. doi:10.18063/ijb.774
Zhao, J., Xiong, J., Ning, Y., Zhao, J., Wang, Z., Long, L., et al. (2023). A triple crosslinked micelle-hydrogel lacrimal implant for localized and prolonged therapy of glaucoma. Eur. J. Pharm. Biopharm. 185, 44–54. doi:10.1016/j.ejpb.2023.02.011
Zhong, X., Gutierrez, C., Xue, T., Hampton, C., Vergara, M. N., Cao, L.-H., et al. (2014). Generation of three-dimensional retinal tissue with functional photoreceptors from human iPSCs. Nat. Commun. 5, 4047. doi:10.1038/ncomms5047
Zhong, Z., Balayan, A., Tian, J., Xiang, Y., Hwang, H.H., Wu, X., Deng, X., Schimelman, J., Sun, Y., Ma, C., Santos, A.D., You, S., Tang, M., Yao, E., Shi, X., Steinmetz, N.F., Deng, S.X., and Chen, S. (2021). Bioprinting of dual ECM scaffolds encapsulating limbal stem/progenitor cells in active and quiescent statuses. Biofabrication 13, 044101. doi:10.1088/1758-5090/ac1992
Keywords: bioartificial cornea, tissue engineering, cornea, corneal pathologies, 3D bioprinting, hydrogel scaffolds
Citation: Al Monla R, Daien V and Michon F (2024) Advanced bioengineering strategies broaden the therapeutic landscape for corneal failure. Front. Bioeng. Biotechnol. 12:1480772. doi: 10.3389/fbioe.2024.1480772
Received: 14 August 2024; Accepted: 30 October 2024;
Published: 13 November 2024.
Edited by:
Nuno Araújo-Gomes, University of Twente, NetherlandsReviewed by:
Yolanda Diebold, University of Valladolid, SpainArantxa Acera, University of the Basque Country, Spain
Copyright © 2024 Al Monla, Daien and Michon. This is an open-access article distributed under the terms of the Creative Commons Attribution License (CC BY). The use, distribution or reproduction in other forums is permitted, provided the original author(s) and the copyright owner(s) are credited and that the original publication in this journal is cited, in accordance with accepted academic practice. No use, distribution or reproduction is permitted which does not comply with these terms.
*Correspondence: Frederic Michon, ZnJlZGVyaWMubWljaG9uQGluc2VybS5mcg==