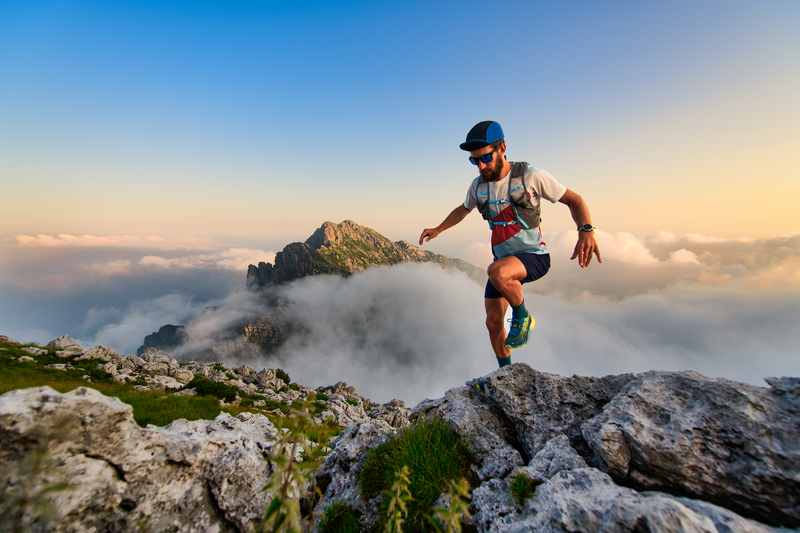
95% of researchers rate our articles as excellent or good
Learn more about the work of our research integrity team to safeguard the quality of each article we publish.
Find out more
MINI REVIEW article
Front. Bioeng. Biotechnol. , 18 July 2024
Sec. Biomaterials
Volume 12 - 2024 | https://doi.org/10.3389/fbioe.2024.1450267
This article is part of the Research Topic Environmentally-Responsive Biomaterials for Major Diseases Treatment View all 9 articles
Treating brain diseases presents significant challenges due to neuronal degeneration, inflammation, and the intricate nature of the brain. Stimuli-responsive hydrogels, designed to closely resemble the brain’s extracellular matrix, have emerged as promising candidates for controlled drug delivery and tissue engineering. These hydrogels have the unique ability to encapsulate therapeutic agents and release them in a controlled manner when triggered by environmental stimuli. This property makes them particularly suitable for delivering drugs precisely to targeted areas of the brain, while minimizing collateral damage to healthy tissue. Their preclinical success in treating various brain diseases in animal studies underscores their translational potential for human brain disease treatment. However, a deeper understanding of their long-term behavior, biodistribution, and biocompatibility within the brain remains crucial. Furthermore, exploring novel hydrogel systems and therapeutic combinations is paramount for advancing towards more effective treatments. This review summarizes the latest advancements in this field over the past 5 years, specifically highlighting preclinical progress with novel stimuli-responsive hydrogels for treating brain diseases.
Brain diseases are one of the primary neurological disorders with high incidence, including traumatic brain injury (TBI), Alzheimer’s disease, Parkinson’s disease, dementia, epilepsy, schizophrenia, stroke, depression, and glioblastoma (GBM) (Feigin et al., 2020). While their etiologies vary, they primarily involve progressive neuronal degeneration and inflammation. In most cases, the treatments to brain disease are limited to symptom reduction and palliative care. Curative therapies that can reverse the illness are lacking. Main challenges for brain disease treatment lie in the lack of structural support allowing repopulation of brain tissue from cell loss and the barriers for efficient drug delivery and release caused by blood-brain barriers (BBB) or other biological environment in brain tissues (Terstappen et al., 2021).
Stimuli-responsive hydrogels, characterized by their three-dimensional cross-linked polymer structure, possess unique properties that render them suitable for addressing various challenges in brain disease treatment. These hydrogels dynamically adapt their characteristics, including mechanical properties, swelling capacity, hydrophilicity, and permeability to bioactive molecules, in response to environmental stimuli such as temperature, pH, and biological agents (Mantha et al., 2019). Their water-absorbing and swelling characteristics mimic the natural extracellular matrix (ECM), fostering an optimal environment for cellular growth and tissue engineering (Ma and Huang, 2020). Furthermore, they excel in encapsulating a range of therapeutic agents, including neuroprotective agents, chemotherapeutic drugs and even cells, and releasing them in a controlled manner, positioning them as exceptional candidates for drug delivery systems (Ghosh et al., 2024). Stimuli-responsive hydrogels have demonstrated encouraging potential in treating brain diseases over the past few decades (Peressotti et al., 2021; Grimaudo et al., 2022). However, further understanding of cell-biomaterial interaction and developing safer, more effective hydrogel systems are crucial for their translation into human therapy.
This review covers the last 5 years of fundamental research on stimuli-responsive hydrogels, discussing their characteristics, design, and preclinical applications in drug delivery and tissue engineering for brain disease treatment. It also highlights challenges and opportunities for future research in this field.
Based on polymer source, hydrogels are classified as natural, synthetic, or semi-synthetic. Natural hydrogels have biocompatibility and biodegradability but limited stability and mechanical strength (Alawami and Tannous, 2021; Janas-Naze and Zhang, 2022). Synthetic hydrogels provide mechanical strength but lack biological activity (Zhang and Huang, 2020). Semi-synthetic hydrogels combine the best of both, improving bioactivity and tunable properties (Dienes et al., 2021).
Hydrogel fabrication involves polymerization and crosslinking to create networks with varying mechanical and swelling properties (Grimaudo et al., 2022; Mashabela et al., 2022). Hydrogels can be administered intravenously, intracerebrally, intratumorally, or intranasally to treat brain diseases (Mashabela et al., 2022). Intravenous injection faces BBB penetration challenges, while intracerebral/intratumoral delivery demands hydrogels with high biocompatibility and safety. Intranasal administration offers direct access to the brain, bypassing the BBB, but is limited by nasal cavity size, mucociliary clearance, enzymatic degradation, and potential drug-induced irritation/neurotoxicity (Cassano et al., 2021).
Stimuli-responsive hydrogels are categorized as physical, chemical, or biological-responsive, depending on their triggering factors. Here we summarize the characteristics of various stimuli-responsive hydrogels suitable for brain disease treatment.
Physical-responsive hydrogels can be classified into thermo-, photo-, electro-, magnetic-, ultrasound-responsive types, each sensitive to temperature, light, electrical stimulation, magnetism and ultrasound respectively. Among these, thermo-, photo-, and electro-responsive hydrogels are the most widely used due to their practicality and effectiveness.
Thermo-responsive hydrogels shrink or expand with temperature changes, featuring hydrophobic (such as methyl, ethyl, and propyl) and hydrophilic groups (like amide and carboxyl) (Tang et al., 2021). Poloxamers (e.g., P407 and P188), also called Pluronics®, are commonly used for intranasal drug delivery due to their mucoadhesive and sol-gel transition properties (Ahmed et al., 2019; Abdeltawab et al., 2020). Hydrogels made from these poloxamers effectively deliver drugs like tetrandrine and rotigotine to the brain, but they lack mechanical strength and viscosity in physiological conditions (Wang et al., 2020; Zhang et al., 2020). To overcome this, they are often combined with other polymers like Carbopol, chitosan, and cellulose derivatives (Luo et al., 2023). Other agents, such as gellan and xanthan gums, are also used for brain drug delivery but may be costly (Rajput et al., 2018).
Photo-responsive hydrogels have photoreceptive moieties that can capture and convert light signal to chemical signals via photoreactions like isomerization, cleavage, and dimerization, thereby changing hydrogel’s physical and chemical properties (Li et al., 2019). However, the primary use of ultraviolet-reactive groups in these systems restricts their biomedical applications.
Electro-responsive hydrogels, containing conducting materials, reversibly absorb and expel water upon electrical stimulation. Their hydration, flexibility, biocompatibility, and electrochemical properties make them suitable for brain implantation, enhancing neural signal transmission and ion transport (Khan et al., 2022). Integrating electroconductive materials into hydrogels reduces inflammation and material loss risks after brain implantation (Guo and Ma, 2018).
Magnetic-responsive hydrogels use magnetic particles, including γ-Fe2O3, Fe3O4 and CoFe2O4, to deliver drugs to specific sites in response to magnetic field (Zhang et al., 2023a). Currently, these hydrogels are limited to in vitro use due to toxicity and reproducibility concerns.
Ultrasound-responsive hydrogels, with imaging-compatible polymers or contrast agents, enhance ultrasound diagnostic accuracy. These hydrogels respond to ultrasound through three mechanisms: crosslinking disruption, hyperthermia, and acoustic streaming (Zhou et al., 2022). However, their use is currently limited to in vitro neural tissue engineering for structural guidance (Cheng et al., 2020).
Ion strength-responsive hydrogels alter in response to solution ion changes, resulting in protonation or deprotonation. Controlling ionic strength can regulate hydrogel reversibility for drug release. Deacylated gellan gum has been effectively utilized in the preparation process of this hydrogel (Chen et al., 2020).
Chemical-responsive hydrogels are classified into pH-, ROS-, hypoxia-responsive types, each sensitive to pH, reactive oxygen species (ROS), and hypoxia respectively.
pH-responsive hydrogels are composed of polymers with hydrophobic moieties that can swell in water depending on pH (Tulain et al., 2018). Recently, hydrogels based on Schiff base chemistry have received attention due to their quick formation via aldehyde-amine bonds and reversible pH-responsive properties (Zhang et al., 2023b).
ROS-responsive hydrogels interact with ROS using oxygen-sensitive groups, which alter the hydrogel network (Gao and Xiong, 2021). Boronic acid crosslinking is used to create various ROS-responsive hydrogels from polymers like sodium alginate, hyaluronic acid, cellulose, chitosan, gelatin, etc.
Hypoxia-responsive hydrogels can be synthesized through incorporation of hypoxia-sensitive moieties. These moieties, including 2-nitro imidazole, azobenzene derivatives, and nitro benzyl derivatives, enable the hydrogels to specifically release drugs within hypoxic environment (Peng et al., 2021).
Biological-responsive hydrogels are classified into enzyme- and glucose-responsive types, each sensitive to enzymes or glucose.
Enzyme-responsive hydrogels incorporate biomolecules that can be cleaved by specific enzymes such as matrix metalloproteinases (MMPs), proteinase K, hyaluronidase and esterase, resulting in altered swelling properties of the gel (Sobczak, 2022). Hyaluronic acid hydrogels can be degraded by hyaluronidase and esterase, while in some cases, by adding MMP-cleavable (PLGL, GCDSGGRMSMPVSDGG) or inactive peptides (GCRDFGAIGQDGDRGG), hydrogels can be made responsive to MMPs (Jian et al., 2018; Adak et al., 2020).
Glucose-responsive hydrogels change their sol-gel behavior based on glucose levels, of which common types include concanavalin A, glucose oxidase, and phenylboronic acid (PBA) hydrogels (Morariu, 2023).
To broaden the capabilities and uses of hydrogels, there’s been a surge of interest in creating dual or even multiple-stimuli-responsive hydrogels. A straightforward method for creating these hydrogels is by incorporating multiple stimuli-responsive materials into existing composite hydrogel systems. Dual ROS/enzyme-, ROS/glucose-, pH/thermo-, ion strength/thermo-, and even triple ROS/pH/thermo-responsive hydrogels have been utilized in brain disease treatment (Table 1).
Table 1. Summary of preclinical in vivo study evaluating the therapeutic efficacy of stimuli-responsive hydrogels in brain disease treatment.
Given the intricate composition of brain tissue, the restoration of functional connectivity between axons, neural circuits, and non-neuronal cells poses a significant challenge for stimuli-responsive hydrogels in treating brain diseases (Halim et al., 2021). These hydrogels must exhibit excellent biocompatibility and biodegradability to minimize immune activation during treatment (Lee et al., 2021; Zamproni et al., 2021). Additionally, the mechanical properties of the hydrogels must closely mimic the softness of brain tissue, typically ranging from 0.1 to 0.3 kPa, to favor neural growth, migration, and neurite extension (Xia et al., 2017; Yang et al., 2017). Substrate topography also plays a pivotal role, providing nano- or micro-structured environments that can guide cell growth and regulate neural cell differentiation (Seo et al., 2018; Wang et al., 2019). Moreover, porosity is a critical factor that influences nutrient diffusion, waste removal, and cell seeding, penetration, and growth within the hydrogel matrix (Bružauskaitė et al., 2016; Shi et al., 2022). Typically, pore sizes in the range of 95–150 µm are considered optimal for neural tissue culture (Shi et al., 2022). To support cell attachment and enhance cellular interactions, immobilization of substances such as poly-L-lysin, fibronectin, gelatin, laminin, collagen, and peptides (RGD, IKVAV, GRGDS, mi-GDPGYIGSR, and mi-GQASSIKVA) is often required (Balion et al., 2020; Long et al., 2020; El-Husseiny et al., 2022). Last, conductive materials show promise in electrical stimulation treatment and recording, but their safety and biocompatibility must be rigorously examined due to concerns regarding cytotoxicity and chronic inflammation (Khan et al., 2022).
Therapeutic agents, including small compounds, peptides, proteins, nucleic acids, cells, and extracellular vesicles, are the key component of stimuli-responsive hydrogels, playing a pivotal role in brain disease treatment by reducing ROS damage and inflammation, promoting neural regeneration, and inducing tumor cell death (Ghosh et al., 2024). To achieve precise and controlled targeted delivery of therapeutic agents, these hydrogels capitalize on the patient’s physiological and pathological environments. Thermo-, ion strength-, and pH-responsive hydrogels exploit temperature variations, ion particles, and pH levels in nasal cavities or brain tissues to form in situ gels. Elevated ROS levels and enzyme expression in diseased brain tissues enable ROS- or enzyme-responsive hydrogels to release therapeutic agents with precision. In diabetics, high glucose triggers glucose-responsive hydrogels to release therapeutic agents. These hydrogels revolutionize brain disease treatment, providing controlled and targeted drug delivery.
Recently, hydrogels with self-assembly, self-healing, nanocomposite hybrid, and nano-size properties show promise for brain disease treatment (Jooken et al., 2023). Peptide-based self-assembling hydrogels exhibit high water content, tunable properties, and injectability, of which self-assembly process is governed by precise hydrophobic/hydrophilic interactions and hydrogen bond formation (Oliveira et al., 2022). Self-healing hydrogels overcome strength limitations, with reversible polymer chains enabling spontaneous repair and enhanced durability, in which chitosan and alginate are commonly used in their production (Zhu et al., 2023). Nanocomposite hydrogels hold superior physical, electrical, and biological properties, particularly for neural regeneration (Wang et al., 2022). Nano-sized hydrogels, or nanogels, are 20–200 nm in size, offering superior targeting and tissue access (Hajebi et al., 2019; Peng et al., 2021).
Brain diseases typically exhibit pathological features such as neural loss or death, vascular dysfunction, inflammation, oxidative stress, and increased expression of MMPs in affected tissues (El-Husseiny et al., 2022; Ghosh et al., 2024). These environmental cues can be exploited by hydrogels that respond to various stimuli, enabling targeted therapeutic delivery. Additionally, therapeutic agents like neuroprotective drugs, peptides, antioxidants, and growth factors can be released by hydrogels to alleviate these pathological conditions. Exceptionally, hypoxia within GBM tissues can be targeted by hypoxia-responsive hydrogels loaded with antitumor drugs, ranging from small compounds to nucleic acids. Notably, nanocomposite hybrid hydrogels and nanogels demonstrate remarkable performance in drug delivery, effectively traversing BBB. Recent preclinical in vivo studies evaluating the therapeutic efficacy of stimuli-responsive hydrogels in brain disease treatment are listed in Table 1.
Encapsulating mesenchymal stromal cells (MSCs) or growth factors into hydrogels can enhance anti-inflammatory effects and promote neural regeneration. Yao et al. developed an injectable thermo-responsive hydrogel using chitosan, hydroxyethyl cellulose, hyaluronic acid, and beta-glycerophosphate (Yao et al., 2019). This hydrogel mimics brain tissue’s rheological behavior, liquefying at <25°C and solidifying at body temperature. When loaded with human umbilical cord-derived MSCs (hUC-MSCs) and injected into TBI rat brains, it enhanced MSC survival and retention, resulting in elevated brain-derived neurotrophic factor, neuron survival, and improved learning and memory, outperforming that of MSC-alone treatment. Separately, Zheng et al. created a blue light crosslinked hydrogel with imidazole-modified gelatin methacrylate and polydopamine/stromal-cell derived factor-1 (PDA@SDF-1α) nanoparticles loaded with human amniotic MSCs (hAMSCs). This hydrogel promoted hAMSC migration to injury sites and neuronal differentiation, repairing cryogenic brain injury in rats (Zheng et al., 2021).
Utilizing enzymes in the injured brain tissues, Adak et al. designed MMP9-responsive peptide-based hydrogels (SFNV) for TBI treatment. These hydrogels released neuroprotective peptides (NAVSIQ) by MMP9-mediated cleavage of PLGL tetrapeptide linker, and promoted neurogenesis in hippocampal regions of cryogenic injury mice (Adak et al., 2020). In another case, Jeong et al. conjugated dexamethasone with hyaluronic acid in a poly (ethylene) glycol-bis-(acryloyloxy acetate) hydrogel (PEG-bis-AA), This formulation sustained dexamethasone release, reducing inflammatory cytokines and enhancing motor recovery in TBI rats 7 days post-injury (Jeong et al., 2021).
Iron overload worsens neurodegeneration by promoting ROS production (Tang et al., 2020). Qiu et al. incorporated desferoxamine mesylate, an iron chelator, into a boron ester-bonded hydrogel composed of 3-aminophenylboronic acid-grafted hyaluronic acid and polyvinyl alcohol (PVA). This hydrogel self-healed and responded to ROS due to the boron ester bond, alleviating iron overload and oxidative stress in brain-injured rats, improving motor, learning, and memory functions (Qiu et al., 2024). For better drug delivery and release, Qian et al. created an injectable dual ROS/enzyme-responsive hydrogel composed of poly (propylene sulfide)120 (PPS120) and curcumin within a triglycerol monostearate (TM) hydrogel. Injection into TBI mice brains caused MMPs to cleave the TM coat, PPS120 to react with ROS, and curcumin to scavenge ROS. This reduced reactive glia cells, inflammation, brain edema, and improved BBB integrity, enhancing nerve regeneration and behavioral recovery in TBI mice (Qian et al., 2021).
Antioxidants and growth factors are embedded in hydrogels to promote neurogenesis and angiogenesis. In a case, a chitosan micellar hydrogel encapsulated hydrophilic minocycline and hydrophobic edaravone drugs for stroke treatment. Injection in rats promptly released the hydrophilic drug and sustains release of the hydrophobic drug, leading to significant behavioral recovery (∼84%) due to sequential anti-inflammatory and neurogenesis effects (Lin et al., 2023). Another study reported the functional repair of the hippocampus post-ischemia using a pluronic-chitosan/aniline-pentamer hydrogel loaded with vascular endothelial growth factor (VEGF). This hydrogel mimics brain tissue conductivity (10–4 S/cm), enabling sustained VEGF release upon intracerebral administration. This approach significantly reduced infarct size by >70% and improved hippocampal-dependent learning and memory, outperforming VEGF delivery alone (Nourbakhsh et al., 2020). A third study used polyelectrolyte complex nanoparticles loaded with SDF-1α and basic fibroblast growth factor, modified with MMP-cleavable peptides, and combined with hyaluronic acid to form enzyme-responsive hydrogels. These hydrogels exhibited superior neurological recovery compared to free growth factors or bare hydrogels in an ischemic stroke model through intracerebral administration, enhancing neurogenesis and angiogenesis (Jian et al., 2018).
ROS and high blood glucose hamper stroke recovery. Jiang et al. designed a dual glucose/ROS-responsive hydrogel loaded with neural stem cell-derived extracellular vesicles (NSC-EVs) for diabetic stroke treatment (Jiang et al., 2022). The hydrogel, made from crosslinking PBA-modified hyaluronic acid with PVA, prolonged EV retention and activity in the brain. NSC-EVs released miRNAs vital for angiogenesis, reducing brain atrophy and enhancing neurobehavioral recovery in diabetic stroke mice.
The use of intranasal administration has demonstrated significantly improved drug delivery for the treatment of Alzheimer’s disease. For instance, Curcumin-loaded mesoporous silica nanoparticles in chitosan and P407 hydrogel improved permeation of drugs and cognitive function in an Alzheimer’s mouse model (Ribeiro et al., 2022). In another study, Chen et al. developed dual pH/thermo-responsive hydrogels with neuroprotective timosaponin BII for Alzheimer’s disease (Chen et al., 2020). This hydrogel integrated ion-sensitive deacetylated gellan gum, thermo-sensitive Poloxamer 407, and sodium alginate, harnessing rapid sol-gel transition triggered by heat and Ca2+ in the nasal cavity. Studies on mice showed improved memory, language, reduced cognitive decline and neuroinflammation.
In recent 5 years, there has been a lack of preclinical animal studies for the treatment of Parkinson’s disease.
Intranasal administration of thermo-responsive gels can effectively deliver drugs to brain tissue. An antidepressant drug, genipin, was combined with hydroxypropyl-β-cyclodextrin (HP-β-CD) and mixed with P407/P188/PEG8000 to create a thermo-responsive hydrogel for intranasal delivery. This hydrogel effectively and sustainably released genipin in mouse brains, improving antidepressant effects (Qi et al., 2021). In another case, icariin was encapsulated in alginate nanogels and integrated into a P407/P188 hydrogel for sustained release after intranasal administration, leading to rapid antidepressant effects in mice and rat models (Xu et al., 2020). Depression is linked to oxidative stress, making antioxidant therapy promising (Bhatt et al., 2020). Recently, a combination of nanoparticles and hydrogels demonstrated impressive drug delivery capabilities (Liu et al., 2023). In this system, amphiphilic polymers (DEX-g-PBAP) made of phenylboronic acid pinacol ester (PBAP) and dextran (DEX) with ROS-sensitive borate ester bonds were used to load antidepressant olanzapine (Olz) to form nanoparticles (Olz/DP NPs). Olz/DP NPs were then modified with amino borane to get Olz/DPA NPs, and further conjugated with hexa-arginine (R6) to create Olz/RDPA NPs. Olz/RDPA NPs were then encapsulated in a poloxamer (P407/P188) hydrogel. Upon intranasal administration in a rat model, the NPs were released from the hydrogel and transported to the brain via the nasal-brain pathway effectively. High ROS levels in the brain triggered NP breakdown, releasing Olz. Simultaneously, H2 released from amino borane scavenged •OH, reversing oxidative stress in the brain and alleviating depressive-like behaviors.
PAOPA, a D2 allosteric modulator, significantly reduced schizophrenia-like symptoms in rats using hydrogels composed of oxidized starch nanoparticles and carboxymethyl chitosan. Its potency and bioavailability are emphasized by achieving relief with half the intraperitoneal dosage through intranasal delivery (Majcher et al., 2021).
Chitosan nanoparticles were employed to enhance the delivery of the antiepileptic drug Oxcarbazepine in Pluronic F127 (also known as poloxamer P407) and sodium carboxymethyl cellulose hydrogel (Abou-Taleb and El-Ganainy, 2023). This formulation exhibited improved antiepileptic activity and anti-inflammatory effects upon intranasal administration.
Direct intratumoral injection of stimuli-responsive hydrogels that leverage tumor microenvironment factors, such as low pH and high enzyme expression, is an efficient strategy to overcome the BBB impediment for drug delivery. Recently, injectable thermo-responsive hydrogels encapsulating salinomycin using copolymers Pluronic F127 and poly (dl-lactide-co-glycolide-b–ethylene glycol-b-dl-lactide-co-glycolide) (PLGA–PEG-PLGA) improved drug release and cytotoxicity, significantly reducing GBM tumor growth, surpassing the effect of free salinomycin alone (4-fold) (Norouzi et al., 2021). In another case, a dual pH/thermo-responsive hydrogel, made from carboxylic acid-terminated oligosulfamethazine and gelatin, transforms rapidly sol-to-gel in response to body temperature and low pH. This hydrogel can sustainably release paclitaxel intratumorally thanks to gelatin’s MMP-cleavage site for antitumor effects in GBM mice (Kang et al., 2021). In a third study, TM-based MMP9-responsive hydrogels loaded with Temozolomide and O6-benzylamine prevented recurrence in post-operative glioma models (Zhao et al., 2020). Encapsulation of therapeutic nucleic acids is also a promising method for treating GBM. Recently, an injectable drug/gene delivery system using a thermosensitive chitosan-based polymer solution to entrap stomatin-like protein 2 (SLP2) shRNA and irinotecan (CPT-11)-loaded cetuximab (CET)-conjugated graphene oxide (GO-CET/CPT11) has been created (CPN@GO-CET/CPT11@shRNA). This formed a hydrogel depot for localized, sustained delivery of therapeutics. Efficient transfection of U87 cancer cells with SLP2 shRNA was achieved using this hydrogel, demonstrated in vivo using tumor-bearing mice. This hydrogel offers extended drug release and shRNA delivery advantages, broadening GBM treatment modalities (Lu et al., 2020).
Nanocomposite hybrids and nanosized gels, as emerging nanotechnologies, have demonstrated significant potential in enhancing intravenous drug delivery for the treatment of GBM. Plk1 upregulation in GBM can be suppressed by Plk1 inhibitor Volasertib. Loaded into angiopep-2-decorated chimeric polypeptide polymersome, Volasertib forms a nanogel with a size of approximately 76 nm from poly(ethylene glycol)-b-poly(L-tyrosine)-b-poly(Laspartic acid). This nanogel traversed BBB and GBM membranes via lipoprotein receptor-related protein 1 (LRP-1)-mediated transcytosis and endocytosis respectively, rapid releasing of Volasertib by proteinase K, suppressing GBM growth and enhancing survival in mice (Fan et al., 2021). In another case, hypoxia-degradable zwitterionic phosphorylcholine nanogel, HPMPC, penetrated the BBB by mimicking cell membrane structure after intravenous administration, releasing doxorubicin by azobenzene moiety degradation in tumor tissues of GBM mouse models (Peng et al., 2021).
In this review, we examined the distinguishing features of a range of stimuli-responsive hydrogels, particularly those that respond to thermal, photonic, magnetic, electrical, and biological cues. Our emphasis lies in highlighting how these hydrogels can capitalize on the pathophysiological features of diseased brains by developing responsive biomaterials. Additionally, we outlined their varied applications and underlying therapeutic mechanisms, including neurogenesis promotion, anti-inflammatory, anti-apoptotic, anti-oxidative, and angiogenic effects, all contributing to the enhancement of therapeutic outcomes (summarized in Figure 1). These hydrogels offer numerous benefits, including precise targeting, controlled release, mechanical support, and biocompatibility, rendering them promising candidates for the treatment of brain diseases in the future.
Figure 1. Schematic diagram of stimuli-responsive hydrogels for brain disease treatment in recent preclinical in vivo study. BBB: blood-brain barrier; bFGF: basic fibroblast growth factor; hAMSCs: human amniotic mesenchymal stromal cells; hUC-MSCs: human umbilical cord mesenchymal stem cells; NSC-EVs: neural stem cell-derived extracellular vesicles; ROS: reactive oxygen species; SDF-1α: stromal-cell-derived factor-1α; SLP2 shRNA: stomatin-like protein 2 short hairpin RNA; VEGF: vascular endothelial growth factor.
These advanced systems, despite their potential, exhibit several limitations that necessitate careful consideration during their application based on the specific pathophysiological characteristics of the target disease. For instance, stimuli-responsive systems relying on alterations in pH, ROS, or enzymes may exhibit suboptimal sensitivity to nuanced microenvironmental changes, potentially resulting in underperformance. Additionally, the in vivo microenvironment poses challenges, such as the formation of a protein corona around nanoparticles, which can significantly reduce or even eliminate the therapeutic efficacy of these systems. Other inherent limitations include thermal denaturation, UV-induced carcinogenesis, insufficient mechanical strength, and material toxicity. Furthermore, the hydrogels’ propensity for high swelling can result in elevated local tissue pressure, which may compromise their mechanical integrity and exacerbate secondary brain injury. Therefore, a thorough understanding of these limitations and careful evaluation of their applicability in specific cases are essential for the optimal utilization of these systems in therapeutic applications.
The integration of nanotechnology, artificial intelligence, and three-dimensional printing into the development of hydrogels presents a compelling opportunity for the creation of innovative therapeutic strategies in brain diseases (Gao et al., 2023). Yet, a rigorous preclinical evaluation encompassing safety, efficacy, and stability is paramount before clinical translation. Key challenges include elucidating hydrogel-neural microenvironment interactions, minimizing immunogenicity, and optimizing targeted delivery. While animal models offer insights, they may not fully recapitulate human disease complexities. Therefore, bridging the gap between in vitro/in vivo models and human diseases remains a significant challenge (Shabani et al., 2023). Future research must prioritize enhancing hydrogel biocompatibility, especially by exploring natural materials that facilitate cellular recognition and integration. Identifying the optimal therapeutic window in various brain diseases, considering disease progression, is crucial for maximizing therapeutic potential. Additionally, scalable manufacturing, regulatory adherence, and economic viability are essential for successful commercialization.
Collectively, future trends in stimuli-responsive hydrogel development emphasize: multifunctional biomaterials integrating therapy, imaging, tissue regeneration, stem cell support, immune modulation, and antibacterial properties; exploration of novel stimuli like inflammatory enzymes for enhanced targeting; optimization of biomaterials for biosafety, efficacy, and cost-effectiveness; addressing patient compliance, convenience, and cost issues; and advancing our pathological understanding of brain diseases to design effective therapies. Collaborations across disciplines are key to translating hydrogel technologies to clinical applications.
In conclusion, stimuli-responsive hydrogels hold significant potential for revolutionizing the treatment of brain diseases. A multidisciplinary approach that integrates expertise from various fields is crucial for advancing this research and ultimately bringing new therapeutic options to patients.
BX: Writing–original draft, Writing–review and editing. HX: Writing–original draft, Writing–review and editing.
The author(s) declare that financial support was received for the research, authorship, and/or publication of this article. The authors thank the financial support from the National Natural Science Foundation of China (32300675), Sichuan Science and Technology Program (2022YFS0615), Luzhou Science and Technology Program of China (2023SYF136, 2023JYJ019), Doctoral Research Initiation Fund of Affiliated Hospital of Southwest Medical University (22069, 22070).
The authors declare that the research was conducted in the absence of any commercial or financial relationships that could be construed as a potential conflict of interest.
All claims expressed in this article are solely those of the authors and do not necessarily represent those of their affiliated organizations, or those of the publisher, the editors and the reviewers. Any product that may be evaluated in this article, or claim that may be made by its manufacturer, is not guaranteed or endorsed by the publisher.
Abdeltawab, H., Svirskis, D., and Sharma, M. (2020). Formulation strategies to modulate drug release from poloxamer based in situ gelling systems. Expert Opin. Drug Deliv. 17, 495–509. doi:10.1080/17425247.2020.1731469
Abou-Taleb, B. A., and EL-Ganainy, S. O. (2023). Thermoresponsive gel-loaded oxcarbazepine nanosystems for nose- to-brain delivery: enhanced antiepileptic activity in rats. Pharm. Res. 40, 1835–1852. doi:10.1007/s11095-023-03552-7
Adak, A., Das, G., Khan, J., Mukherjee, N., Gupta, V., Mallesh, R., et al. (2020). Extracellular matrix (ECM)-Mimicking neuroprotective injectable sulfo-functionalized peptide hydrogel for repairing brain injury. ACS Biomater. Sci. Eng. 6, 2287–2296. doi:10.1021/acsbiomaterials.9b01829
Ahmed, S., Gull, A., Aqil, M., Danish Ansari, M., and Sultana, Y. (2019). Poloxamer-407 thickened lipid colloidal system of agomelatine for brain targeting: characterization, brain pharmacokinetic study and behavioral study on Wistar rats. Colloids Surf. B Biointerfaces 181, 426–436. doi:10.1016/j.colsurfb.2019.05.016
Alawami, A. Z., and Tannous, Z. (2021). Late onset hypersensitivity reaction to hyaluronic acid dermal fillers manifesting as cutaneous and visceral angioedema. J. Cosmet. Dermatol 20, 1483–1485. doi:10.1111/jocd.13894
Balion, Z., Cėpla, V., Svirskiene, N., Svirskis, G., Druceikaitė, K., Inokaitis, H., et al. (2020). Cerebellar cells self-assemble into functional organoids on synthetic, chemically crosslinked ECM-mimicking peptide hydrogels. Biomolecules 10, 754. doi:10.3390/biom10050754
Bhatt, S., Nagappa, A. N., and Patil, C. R. (2020). Role of oxidative stress in depression. Drug Discov. Today 25, 1270–1276. doi:10.1016/j.drudis.2020.05.001
Bružauskaitė, I., Bironaitė, D., Bagdonas, E., and Bernotienė, E. (2016). Scaffolds and cells for tissue regeneration: different scaffold pore sizes-different cell effects. Cytotechnology 68, 355–369. doi:10.1007/s10616-015-9895-4
Cassano, R., Servidio, C., and Trombino, S. (2021). Biomaterials for drugs nose-brain transport: a new therapeutic approach for neurological diseases. Mater. (Basel) 14, 1802. doi:10.3390/ma14071802
Cheng, K. W., Alhasan, L., Rezk, A. R., AL-Abboodi, A., Doran, P. M., Yeo, L. Y., et al. (2020). Fast three-dimensional micropatterning of PC12 cells in rapidly crosslinked hydrogel scaffolds using ultrasonic standing waves. Biofabrication 12, 015013. doi:10.1088/1758-5090/ab4cca
Chen, W., Li, R., Zhu, S., Ma, J., Pang, L., Ma, B., et al. (2020). Nasal timosaponin BII dually sensitive in situ hydrogels for the prevention of Alzheimer’s disease induced by lipopolysaccharides. Int. J. Pharm. 578, 119115. doi:10.1016/j.ijpharm.2020.119115
Dienes, J., Browne, S., Farjun, B., Amaral Passipieri, J., Mintz, E. L., Killian, G., et al. (2021). Semisynthetic hyaluronic acid-based hydrogel promotes recovery of the injured tibialis anterior skeletal muscle form and function. ACS Biomater. Sci. Eng. 7, 1587–1599. doi:10.1021/acsbiomaterials.0c01751
EL-Husseiny, H. M., Mady, E. A., Hamabe, L., Abugomaa, A., Shimada, K., Yoshida, T., et al. (2022). Smart/stimuli-responsive hydrogels: cutting-edge platforms for tissue engineering and other biomedical applications. Mater Today Bio 13, 100186. doi:10.1016/j.mtbio.2021.100186
Fan, Q., Liu, Y., Cui, G., Zhong, Z., and Deng, C. (2021). Brain delivery of Plk1 inhibitor via chimaeric polypeptide polymersomes for safe and superb treatment of orthotopic glioblastoma. J. Control Release 329, 1139–1149. doi:10.1016/j.jconrel.2020.10.043
Feigin, V. L., Vos, T., Nichols, E., Owolabi, M. O., Carroll, W. M., Dichgans, M., et al. (2020). The global burden of neurological disorders: translating evidence into policy. Lancet Neurol. 19, 255–265. doi:10.1016/s1474-4422(19)30411-9
Gao, F., and Xiong, Z. (2021). Reactive oxygen species responsive polymers for drug delivery systems. Front. Chem. 9, 649048. doi:10.3389/fchem.2021.649048
Gao, Q., Lee, J. S., Kim, B. S., and Gao, G. (2023). Three-dimensional printing of smart constructs using stimuli-responsive biomaterials: a future direction of precision medicine. Int. J. Bioprint 9, 638. doi:10.18063/ijb.v9i1.638
Ghosh, S., Ghosh, S., Sharma, H., Bhaskar, R., Han, S. S., and Sinha, J. K. (2024). Harnessing the power of biological macromolecules in hydrogels for controlled drug release in the central nervous system: a review. Int. J. Biol. Macromol. 254, 127708. doi:10.1016/j.ijbiomac.2023.127708
Grimaudo, M. A., Krishnakumar, G. S., Giusto, E., Furlani, F., Bassi, G., Rossi, A., et al. (2022). Bioactive injectable hydrogels for on demand molecule/cell delivery and for tissue regeneration in the central nervous system. Acta Biomater. 140, 88–101. doi:10.1016/j.actbio.2021.11.038
Guo, B., and Ma, P. X. (2018). Conducting polymers for tissue engineering. Biomacromolecules 19, 1764–1782. doi:10.1021/acs.biomac.8b00276
Hajebi, S., Rabiee, N., Bagherzadeh, M., Ahmadi, S., Rabiee, M., Roghani-Mamaqani, H., et al. (2019). Stimulus-responsive polymeric nanogels as smart drug delivery systems. Acta Biomater. 92, 1–18. doi:10.1016/j.actbio.2019.05.018
Halim, A., Qu, K. Y., Zhang, X. F., and Huang, N. P. (2021). Recent advances in the application of two-dimensional nanomaterials for neural tissue engineering and regeneration. ACS Biomater. Sci. Eng. 7, 3503–3529. doi:10.1021/acsbiomaterials.1c00490
Huang, X., Ye, Y., Zhang, J., Zhang, X., Ma, H., Zhang, Y., et al. (2022). Reactive oxygen species scavenging functional hydrogel delivers procyanidins for the treatment of traumatic brain injury in mice. ACS Appl. Mater Interfaces 14, 33756–33767. doi:10.1021/acsami.2c04930
Janas-Naze, A., and Zhang, W. (2022). Perioperative anaphylaxis to fibrin sealants in children with Noonan Syndrome: a retrospective study. Ann. Allergy Asthma Immunol. 129, 95–100. doi:10.1016/j.anai.2022.03.014
Jeong, D. U., Bae, S., Macks, C., Whitaker, J., Lynn, M., Webb, K., et al. (2021). Hydrogel-mediated local delivery of dexamethasone reduces neuroinflammation after traumatic brain injury. Biomed. Mater. 16, 035002. doi:10.1088/1748-605x/abc7f1
Jian, W. H., Wang, H. C., Kuan, C. H., Chen, M. H., Wu, H. C., Sun, J. S., et al. (2018). Glycosaminoglycan-based hybrid hydrogel encapsulated with polyelectrolyte complex nanoparticles for endogenous stem cell regulation in central nervous system regeneration. Biomaterials 174, 17–30. doi:10.1016/j.biomaterials.2018.05.009
Jiang, Y., Wang, R., Wang, C., Guo, Y., Xu, T., Zhang, Z., et al. (2022). Brain microenvironment responsive and pro-angiogenic extracellular vesicle-hydrogel for promoting neurobehavioral recovery in type 2 diabetic mice after stroke. Adv. Healthc. Mater 11, e2201150. doi:10.1002/adhm.202201150
Jooken, S., Deschaume, O., and Bartic, C. (2023). Nanocomposite hydrogels as functional extracellular matrices. Gels 9, 153. doi:10.3390/gels9020153
Kang, J. H., Turabee, M. H., Lee, D. S., Kwon, Y. J., and Ko, Y. T. (2021). Temperature and pH-responsive in situ hydrogels of gelatin derivatives to prevent the reoccurrence of brain tumor. Biomed. Pharmacother. 143, 112144. doi:10.1016/j.biopha.2021.112144
Khan, Z. M., Wilts, E., Vlaisavljevich, E., Long, T. E., and Verbridge, S. S. (2022). Electroresponsive hydrogels for therapeutic applications in the brain. Macromol. Biosci. 22, e2100355. doi:10.1002/mabi.202100355
Lee, W. H., Cha, G. D., and Kim, D. H. (2021). Flexible and biodegradable electronic implants for diagnosis and treatment of brain diseases. Curr. Opin. Biotechnol. 72, 13–21. doi:10.1016/j.copbio.2021.07.027
Li, L., Scheiger, J. M., and Levkin, P. A. (2019). Design and applications of photoresponsive hydrogels. Adv. Mater 31, e1807333. doi:10.1002/adma.201807333
Lin, S.-H., Huang, A. P.-H., and Hsu, S.-H. (2023). Injectable, micellar chitosan self-healing hydrogel for asynchronous dual-drug delivery to treat stroke rats. Adv. Funct. Mater. 33, 2303853. doi:10.1002/adfm.202303853
Liu, L., Liu, M., Xiu, J., Zhang, B., Hu, H., Qiao, M., et al. (2023). Stimuli-responsive nanoparticles delivered by a nasal-brain pathway alleviate depression-like behavior through extensively scavenging ROS. Acta Biomater. 171, 451–465. doi:10.1016/j.actbio.2023.09.038
Long, Y., Yan, L., Dai, H., Yang, D., Wu, X., Dong, X., et al. (2020). Enhanced proliferation and differentiation of neural stem cells by peptide-containing temperature-sensitive hydrogel scaffold. Mater Sci. Eng. C Mater Biol. Appl. 116, 111258. doi:10.1016/j.msec.2020.111258
Lu, Y. J., Lan, Y. H., Chuang, C. C., Lu, W. T., Chan, L. Y., Hsu, P. W., et al. (2020). Injectable thermo-sensitive chitosan hydrogel containing CPT-11-loaded EGFR-targeted graphene oxide and SLP2 shRNA for localized drug/gene delivery in glioblastoma therapy. Int. J. Mol. Sci. 21, 7111. doi:10.3390/ijms21197111
Luo, J., Zhao, X., Guo, B., and Han, Y. (2023). Preparation, thermal response mechanisms and biomedical applications of thermosensitive hydrogels for drug delivery. Expert Opin. Drug Deliv. 20, 641–672. doi:10.1080/17425247.2023.2217377
Ma, J., and Huang, C. (2020). Composition and mechanism of three-dimensional hydrogel system in regulating stem cell fate. Tissue Eng. Part B Rev. 26, 498–518. doi:10.1089/ten.teb.2020.0021
Majcher, M. J., Babar, A., Lofts, A., Leung, A., Li, X., Abu-Hijleh, F., et al. (2021). In situ-gelling starch nanoparticle (SNP)/O-carboxymethyl chitosan (CMCh) nanoparticle network hydrogels for the intranasal delivery of an antipsychotic peptide. J. Control Release 330, 738–752. doi:10.1016/j.jconrel.2020.12.050
Mantha, S., Pillai, S., Khayambashi, P., Upadhyay, A., Zhang, Y., Tao, O., et al. (2019). Smart hydrogels in tissue engineering and regenerative medicine. Mater. (Basel) 12, 3323. doi:10.3390/ma12203323
Mashabela, L. T., Maboa, M. M., Miya, N. F., Ajayi, T. O., Chasara, R. S., Milne, M., et al. (2022). A comprehensive review of cross-linked gels as vehicles for drug delivery to treat central nervous system disorders. Gels 8, 563. doi:10.3390/gels8090563
Morariu, S. (2023). Advances in the design of phenylboronic acid-based glucose-sensitive hydrogels. Polym. (Basel) 15, 582. doi:10.3390/polym15030582
Norouzi, M., Firouzi, J., Sodeifi, N., Ebrahimi, M., and Miller, D. W. (2021). Salinomycin-loaded injectable thermosensitive hydrogels for glioblastoma therapy. Int. J. Pharm. 598, 120316. doi:10.1016/j.ijpharm.2021.120316
Nourbakhsh, M., Zarrintaj, P., Jafari, S. H., Hosseini, S. M., Aliakbari, S., Pourbadie, H. G., et al. (2020). Fabricating an electroactive injectable hydrogel based on pluronic-chitosan/aniline-pentamer containing angiogenic factor for functional repair of the hippocampus ischemia rat model. Mater Sci. Eng. C Mater Biol. Appl. 117, 111328. doi:10.1016/j.msec.2020.111328
Oliveira, C. B. P., Gomes, V., Ferreira, P. M. T., Martins, J. A., and Jervis, P. J. (2022). Peptide-based supramolecular hydrogels as drug delivery agents: recent advances. Gels 8, 706. doi:10.3390/gels8110706
Peng, S., Ouyang, B., Xin, Y., Zhao, W., Shen, S., Zhan, M., et al. (2021). Hypoxia-degradable and long-circulating zwitterionic phosphorylcholine-based nanogel for enhanced tumor drug delivery. Acta Pharm. Sin. B 11, 560–571. doi:10.1016/j.apsb.2020.08.012
Peressotti, S., Koehl, G. E., Goding, J. A., and Green, R. A. (2021). Self-assembling hydrogel structures for neural tissue repair. ACS Biomater. Sci. Eng. 7, 4136–4163. doi:10.1021/acsbiomaterials.1c00030
Qi, X.-J., Xu, D., Tian, M.-L., Zhou, J.-F., Wang, Q.-S., and Cui, Y.-L. (2021). Thermosensitive hydrogel designed for improving the antidepressant activities of genipin via intranasal delivery. Mater. Des. 206, 109816. doi:10.1016/j.matdes.2021.109816
Qian, F., Han, Y., Han, Z., Zhang, D., Zhang, L., Zhao, G., et al. (2021). In situ implantable, post-trauma microenvironment-responsive, ROS Depletion Hydrogels for the treatment of Traumatic brain injury. Biomaterials 270, 120675. doi:10.1016/j.biomaterials.2021.120675
Qiu, Y., Zeng, Y., Zhang, C., Lv, X., Ling, Y., Si, Y., et al. (2024). A ROS-responsive loaded desferoxamine (DFO) hydrogel system for traumatic brain injury therapy. Biomed. Mater 19, 025016. doi:10.1088/1748-605x/ad1dfd
Rajput, A., Bariya, A., Allam, A., Othman, S., and Butani, S. B. (2018). In situ nanostructured hydrogel of resveratrol for brain targeting: in vitro-in vivo characterization. Drug Deliv. Transl. Res. 8, 1460–1470. doi:10.1007/s13346-018-0540-6
Ribeiro, T. D. C., SáBIO, R. M., Luiz, M. T., DE Souza, L. C., Fonseca-Santos, B., Cides Da Silva, L. C., et al. (2022). Curcumin-loaded mesoporous silica nanoparticles dispersed in thermo-responsive hydrogel as potential alzheimer disease therapy. Pharmaceutics 14, 1976. doi:10.3390/pharmaceutics14091976
Seo, J., Kim, J., Joo, S., Choi, J. Y., Kang, K., Cho, W. K., et al. (2018). Nanotopography-promoted formation of axon collateral branches of hippocampal neurons. Small 14, e1801763. doi:10.1002/smll.201801763
Shabani, L., Abbasi, M., Azarnew, Z., Amani, A. M., and Vaez, A. (2023). Neuro-nanotechnology: diagnostic and therapeutic nano-based strategies in applied neuroscience. Biomed. Eng. Online 22, 1. doi:10.1186/s12938-022-01062-y
Shi, M., Xu, Q., Ding, L., Xia, Y., Zhang, C., Lai, H., et al. (2022). Cell infiltrative inner connected porous hydrogel improves neural stem cell migration and differentiation for functional repair of spinal cord injury. ACS Biomater. Sci. Eng. 8, 5307–5318. doi:10.1021/acsbiomaterials.2c01127
Sobczak, M. (2022). Enzyme-responsive hydrogels as potential drug delivery systems-state of knowledge and future prospects. Int. J. Mol. Sci. 23, 4421. doi:10.3390/ijms23084421
Tang, L., Wang, L., Yang, X., Feng, Y., Li, Y., and Feng, W. (2021). Poly(N-isopropylacrylamide)-based smart hydrogels: design, properties and applications. Prog. Mater. Sci. 115, 100702. doi:10.1016/j.pmatsci.2020.100702
Tang, S., Gao, P., Chen, H., Zhou, X., Ou, Y., and He, Y. (2020). The role of iron, its metabolism and ferroptosis in traumatic brain injury. Front. Cell Neurosci. 14, 590789. doi:10.3389/fncel.2020.590789
Terstappen, G. C., Meyer, A. H., Bell, R. D., and Zhang, W. (2021). Strategies for delivering therapeutics across the blood-brain barrier. Nat. Rev. Drug Discov. 20, 362–383. doi:10.1038/s41573-021-00139-y
Tulain, U. R., Ahmad, M., Rashid, A., Malik, M. Z., and Iqbal, F. M. (2018). Fabrication of pH-responsive hydrogel and its in vitro and in vivo evaluation. Adv. Polym. Technol. 37, 290–304. doi:10.1002/adv.21668
Wang, F., Yang, Z., Liu, M., Tao, Y., Li, Z., Wu, Z., et al. (2020). Facile nose-to-brain delivery of rotigotine-loaded polymer micelles thermosensitive hydrogels: in vitro characterization and in vivo behavior study. Int. J. Pharm. 577, 119046. doi:10.1016/j.ijpharm.2020.119046
Wang, L., Wu, Y., Hu, T., Ma, P. X., and Guo, B. (2019). Aligned conductive core-shell biomimetic scaffolds based on nanofiber yarns/hydrogel for enhanced 3D neurite outgrowth alignment and elongation. Acta Biomater. 96, 175–187. doi:10.1016/j.actbio.2019.06.035
Wang, X., Ye, L., He, W., Teng, C., Sun, S., Lu, H., et al. (2022). In situ targeting nanoparticles-hydrogel hybrid system for combined chemo-immunotherapy of glioma. J. Control. Release 345, 786–797. doi:10.1016/j.jconrel.2022.03.050
Xia, T., Liu, W., and Yang, L. (2017). A review of gradient stiffness hydrogels used in tissue engineering and regenerative medicine. J. Biomed. Mater Res. A 105, 1799–1812. doi:10.1002/jbm.a.36034
Xu, D., Lu, Y.-R., Kou, N., Hu, M.-J., Wang, Q.-S., and Cui, Y.-L. (2020). Intranasal delivery of icariin via a nanogel-thermoresponsive hydrogel compound system to improve its antidepressant-like activity. Int. J. Pharm. 586, 119550. doi:10.1016/j.ijpharm.2020.119550
Yang, Y., Wang, K., Gu, X., and Leong, K. W. (2017). Biophysical regulation of cell behavior-cross talk between substrate stiffness and nanotopography. Eng. (Beijing) 3, 36–54. doi:10.1016/j.eng.2017.01.014
Yao, M., Chen, Y., Zhang, J., Gao, F., Ma, S., and Guan, F. (2019). Chitosan-based thermosensitive composite hydrogel enhances the therapeutic efficacy of human umbilical cord MSC in TBI rat model. Mater. Today Chem. 14, 100192. doi:10.1016/j.mtchem.2019.08.011
Zamproni, L. N., Mundim, M., and Porcionatto, M. A. (2021). Neurorepair and regeneration of the brain: a decade of bioscaffolds and engineered microtissue. Front. Cell Dev. Biol. 9, 649891. doi:10.3389/fcell.2021.649891
Zhang, J., Wang, Y., Shu, X., Deng, H., Wu, F., and He, J. (2023a). Magnetic chitosan hydrogel induces neuronal differentiation of neural stem cells by activating RAS-dependent signal cascade. Carbohydr. Polym. 314, 120918. doi:10.1016/j.carbpol.2023.120918
Zhang, L., Pang, L., Zhu, S., Ma, J., Li, R., Liu, Y., et al. (2020). Intranasal tetrandrine temperature-sensitive in situ hydrogels for the treatment of microwave-induced brain injury. Int. J. Pharm. 583, 119384. doi:10.1016/j.ijpharm.2020.119384
Zhang, W., Liu, W., Long, L., He, S., Wang, Z., Liu, Y., et al. (2023b). Responsive multifunctional hydrogels emulating the chronic wounds healing cascade for skin repair. J. Control Release 354, 821–834. doi:10.1016/j.jconrel.2023.01.049
Zhang, Y., and Huang, Y. (2020). Rational design of smart hydrogels for biomedical applications. Front. Chem. 8, 615665. doi:10.3389/fchem.2020.615665
Zhao, Z., Shen, J., Zhang, L., Wang, L., Xu, H., Han, Y., et al. (2020). Injectable postoperative enzyme-responsive hydrogels for reversing temozolomide resistance and reducing local recurrence after glioma operation. Biomater. Sci. 8, 5306–5316. doi:10.1039/d0bm00338g
Zheng, Y., Wu, G., Chen, L., Zhang, Y., Luo, Y., Zheng, Y., et al. (2021). Neuro-regenerative imidazole-functionalized GelMA hydrogel loaded with hAMSC and SDF-1α promote stem cell differentiation and repair focal brain injury. Bioact. Mater. 6, 627–637. doi:10.1016/j.bioactmat.2020.08.026
Zhou, Y., Liu, G., and Guo, S. (2022). Advances in ultrasound-responsive hydrogels for biomedical applications. J. Mater. Chem. B 10, 3947–3958. doi:10.1039/d2tb00541g
Keywords: stimuli-responsive, hydrogels, brain disease, drug delivery, tissue engineering
Citation: Xie B and Xie H (2024) Application of stimuli-responsive hydrogel in brain disease treatment. Front. Bioeng. Biotechnol. 12:1450267. doi: 10.3389/fbioe.2024.1450267
Received: 17 June 2024; Accepted: 26 June 2024;
Published: 18 July 2024.
Edited by:
Yao Luo, Sichuan University, ChinaCopyright © 2024 Xie and Xie. This is an open-access article distributed under the terms of the Creative Commons Attribution License (CC BY). The use, distribution or reproduction in other forums is permitted, provided the original author(s) and the copyright owner(s) are credited and that the original publication in this journal is cited, in accordance with accepted academic practice. No use, distribution or reproduction is permitted which does not comply with these terms.
*Correspondence: Huangfan Xie, eGllMTk5MUBzd211LmVkdS5jbg==
Disclaimer: All claims expressed in this article are solely those of the authors and do not necessarily represent those of their affiliated organizations, or those of the publisher, the editors and the reviewers. Any product that may be evaluated in this article or claim that may be made by its manufacturer is not guaranteed or endorsed by the publisher.
Research integrity at Frontiers
Learn more about the work of our research integrity team to safeguard the quality of each article we publish.