- 1Biological Systems and Engineering Division, Lawrence Berkeley National Laboratory, Berkeley, CA, United States
- 2Joint BioEnergy Institute, Emeryville, CA, United States
Methanotrophic bacteria are promising hosts for methane bioconversion to biochemicals or bioproducts. However, due to limitations associated with long genetic manipulation timelines and, lack of choice in genetic tools required for strain engineering, methanotrophs are currently not employed for bioconversion technologies. In this study, a rapid and reproducible electroporation protocol is developed for type 1 methanotroph, Methylotuvimicrobium alcaliphilum using common laboratory solutions, analyzing optimal electroshock voltages and post-shock cell recovery time. Successful reproducibility of the developed method was achieved when different replicative plasmids were assessed on lab adapted vs. wild-type M. alcaliphilum strains (DASS vs. DSM19304). Overall, a ∼ 3-fold decrease in time is reported with use of electroporation protocol developed here, compared to conjugation, which is the traditionally employed approach. Additionally, an inducible (3-methyl benzoate) and a constitutive (sucrose phosphate synthase) promoter is characterized for their strength in driving gene expression.
Introduction
Methane (CH4) is one of the most potent greenhouse gases (GHGs), as 1 mol of CH4 can absorb about 24 times more radiations than 1 mol of carbon dioxide (Wuebbles and Hayhoe, 2002). In the past decades, rising anthropogenic activities like biomass burning, biological waste, landfills (renewables) and extraction of natural gas, coal and petroleum (non-renewables) have contributed more to global atmospheric methane emissions than natural sources (Wuebbles and Hayhoe, 2002; Strong et al., 2015). Methane also adversely impacts climate by the slow but steady oxidation of CH4 to CO2 in the atmosphere and the rapid conversion to CO2 due to burning of natural gas as a fuel source (Boucher et al., 2009). The cost of natural gas, which is ∼90% methane, has been consistently lower than sugar prices, making it a low-cost feedstock for bioconversion to biochemicals, biofuels and bioproducts. Biological conversion of methane to other value-added materials has the potential to provide a sustainable, eco-friendly route to chemical production (Hwang et al., 2018). Moreover, bioconversion of methane has a higher carbon conversion efficiency compared to its chemical conversion, where oxidation of methane to methanol using methanotrophs has 75% conversion efficiency versus 20%–50% using chemical processes (In Yeub et al., 2014; In Yeub et al., 2015). With recent advancements in synthetic biology, methanotrophs have been used for successful production of variety of biochemicals and demonstration of proof-of-concept studies with highly selective methane bioconversion routes to many specialty chemicals as well. A recent review has discussed a list of biochemicals that can be produced such as polyhydroxyalkanoates, methanol, single cell protein, ectoine, fatty acids, lipids and organic acids (Gęsicka et al., 2021), and 90% utilization of gaseous substrate including methane for isobutanol production (Liang et al., 2022) using methanotrophs as host organisms.
Methanotrophs, or microbes that consume CH4, provide a biological and natural methane sink either via aerobic or anaerobic routes of CH4 consumption (Rhee et al., 2019). However, among the methanotrophs, aerobic methanotrophic bacteria have been mostly isolated in pure cultures and studied (Guerrero-Cruz et al., 2021). There are two types of aerobic methanotrophs, type-I methanotrophs (gammaproteobacterial) and type-II methanotrophs (alphaproteobacterial) (Hanson and Hanson, 1996). Amongst the two, type-I methanotrophs employ a more energy efficient pathway for formaldehyde assimilation into cellular biomass, using the ribulose monophosphate pathway coupled with the Entner-Doudoroff or Emden Myerhoff Parnas pathways. Therefore, type-I methanotrophs could be attractive candidates to develop as a microbial platform for biochemical/fuel production (Hanson and Hanson, 1996). Consequently, type-I methanotrophs belonging to the Gram-negative genus Methylotuvimicrobium, such as M. buryatense and M. alcaliphilum, have been previously engineered to produce fatty acid (Demidenko et al., 2017), 2,3-butanediol (Nguyen et al., 2018), lactic acid (Henard et al., 2016), and rhamnolipids (Awasthi et al., 2022). However, unlike sugar based model industrial microbial hosts such as, Escherichia coli and Bacillus subtilis, methanotrophic hosts have very limited tools for genetic manipulation (Henard and Guarnieri, 2018), hindering efforts at metabolic engineering. Therefore, advancement of genetic tools, such as faster transformation methods and characterized promoters for gene expression, is imperative to expand the utility of Methylomicrobium sp. as future microbial cell factories.
Conjugation-based transformation methods have been used conventionally for plasmid transfer in methanotrophs (Ojala et al., 2011; Puri et al., 2015). However, the challenges of conjugation for cloning or expression purposes include the involvement of multiple, time-consuming steps and that the recipient strain is susceptible to genetic changes that may occur in the plasmid during conjugation (Puri et al., 2015). Replacing conjugation with electroporation provides advantages by shortening the cloning process and providing a direct mode of DNA transfer. Recently, electroporation transformation was reported in type-I methanotrophs M. buryatense 5G; however, transformed colonies via electroporation were only obtained when plasmids were first conjugated into M. buryatense 5G, isolated from this strain, and then re-transformed to M. buryatense, as no colonies were obtained after electroporation when plasmids were directly isolated from E. coli strain TOP10 (Yan et al., 2016). Thus, this method also was indirect and required additional steps. Hence, in this study, a robust electroporation protocol was developed after evaluation and optimization of four parameters effecting electroporation efficiency. In our understanding, this is the first study to show comprehensive analysis of electroporation parameter evaluation for a methanotrophic bacteria and present a working/reproducible electroporation protocol.
Further on, metabolic engineering often requires heterologous gene expression, and so a variety of promoters are needed to enable robust strain engineering. A few constitutive and inducible promoters have been previously used by different groups in genus Methylotuvimicrobium (Puri et al., 2015; Henard and Guarnieri, 2018). Therefore, in addition to improved transformation methods, more characterized promoters would be beneficial for the advancement of genetic tools. In this study, an inducible (3-methyl benzoate) and a constitutive (sucrose phosphate synthase) promoter were screened with green-fluorescent protein (GFP) reporter tag to monitor their gene expression strengths in M. alcaliphilum strain DASS, previously developed by our group as a high fatty acid producer (Awasthi et al., 2022). Robust Protocols developed for strain M. alcaliphilum strain DASS are expected to enhance the application of this strain as a potential industrial microbe for bioconverting CH4 to targeted bioproducts, with implications for tool development in other type-I and/or type-II methanotrophic bacteria.
Materials and methods
Bacterial growth and culture conditions
All bacterial strains and plasmids used in this study are listed in Table 1. E. coli was used as an intermediate host for plasmid cloning and transformation. E. coli strains were grown in Luria-Bertani (LB) media and 50 μg/mL kanamycin was added to the culture when required. E. coli was cultivated at 37°C, shaking at 200 RPM. M. alcaliphilum strains were cultured as described previously by Awasthi et al. (2022). In brief, M. alcaliphilum was grown in sealed serum bottles at 30°C at 200 RPM in Pi (π)/P3 media with 30% (w/v) NaCl, 100 μg/mL Kanamycin (kan) was added to the growth medium when required. Cells were incubated under CH4 (Ultra-pure 99.9%, Airgas) and air at ratio 1:1. M. alcaliphilum cell growth (OD600) was measured by spectrophotometer SpectraMax M2 microplate reader (Molecular devices, San Jose, CA, United States). Colony selection after transformation was performed in P3 media-agar plates kept in the anaerobic jar (Oxoid, Remel) under CH4- air (1:1) atmosphere for 5–6 days.
Electroporation optimization
For plasmid electroporation, a single colony of strain DASS was inoculated in 10 mL P3 media in 50 mL serum bottle incubated at 30°C with 200 RPM shaking. The culture was grown until an OD600 of 1.4 was reached. This culture was used as the primary inoculum, and 500 µL was subsequently added to final growth culture, 50 mL P0.75 low salt (7.5% (wt/vol) NaCl) media in a 180 mL serum bottle followed by incubation at 30°C with shaking at 200 RPM until OD600 0.8. A liquid: gas ratio of 1:5 was used for the seed culture as has been used previously (Awasthi et al., 2022), which is in the commonly used liquid: gas ratio for aerobic methanotrophs (Henard et al., 2019). For the secondary culture an alternate liquid: gas ratio of 1:4 was used (50 mL Pi media in 180 mL serum bottle) to obtain enough biomass at OD600 0.8. Cells were harvested at 5,000 RPM at 4°C for 15 min by centrifugation (Avanti J-15R, Beckman Coulter, IN, United States). Cell pellet obtained was then resuspended in 50 mL of electroporation wash buffer (as mentioned). All the wash buffers were kept at 4°C during experimental procedures. Washing was repeated three times at 5,000 RPM, 4°C for 15 min with the wash buffer. After the third wash, final cell pellet was resuspended in 150 μL of wash buffer. 60 μL of cells were transferred to electroporation cuvette (1 mm gap). Gene Pulser XCell™ (BioRad Labs GmbH, Munich, Germany) was used for electroporation. Plasmid concentration was kept at 1 μg for each electroporation transformation based on a previous report in related studies (Yan et al., 2016). Additionally, in this work a lower concentration (around 500–600 ng) of pDNA was also tested that resulted in very low/no transformation efficiency. Hence, for all electroporation experiments a standard pDNA concentration of 1 µg was used. Various electroporation voltages (as mentioned) ranging from 1.4 to 2.2 kV were screened to identify the optimum voltage. Immediately after electroporation, cells from cuvette were transferred to 10 mL of P3 media in 50 mL serum bottle, followed by recovery at 30°C under methane: air atmosphere for the duration as mentioned. After recovery the growing cells were harvested at 5,000 RPM at room temperature for 15 min, followed by plating on P3 (kanamycin) selection plates and incubated at 30°C in anaerobic jar under CH4: air (1:1) atmosphere. Colonies were observed and tabulated in 5–6 days after incubation.
Plasmid construction
All plasmid construction was performed using NEB HiFi assembly conceptually based on Gibson assembly (Gibson et al., 2009) using E. coli TOP10F as the initial plasmid propagating host. The primers used in this study are listed in Table 2. Inducible promoter, Pm (3-methyl benzoate) was cloned from plasmid 138475 (pORTMAGE-Pa1) purchased form Addgene. The Pm promoter (1.946 kb) fragment was PCR amplified using primers Pm fragment forward/reverse (Table 2) and the vector pCAH01_emGFP was linearized by PCR amplification using primers pCAH01_emGFP vector forward/reverse (Table 2), the PCR amplicons of promoter and linearized vector were assembled using NEB HiFi assembly kit, resulting in pSGDA1. Similarly, sucrose phosphate synthase promoter (Psps; 792 bp) was PCR amplified using primers Psps fragment forward/reverse (Table 2) with template pDA21 (Table 1), vector pCAH01_emGFP was linearized using primers Psps vector forward/reverse (Table 2). Vector and insert were assembled using HiFi assembly (NEB) to construct pSGDA2.
Results
Optimization of electroporation for M. alcaliphilum
Conjugation is the traditional mode of plasmid (pDNA) transformation for methanotrophs which takes about 4–5 weeks. In this work, electroporation-based transformation method was evaluated and optimized to accelerate the pDNA transfer procedure. Two plasmids, pCAH01 (7.6 kb size; expression vector; Table1) and pCM433 (5.3 kb size; cloning vector; Table 1) were initially evaluated to identify the optimal conditions of a) wash buffers, b) voltage, and c) cell recovery time. Both plasmids were first isolated from E. coli TOP10F and subsequently electroporated to M. alcaliphilum DASS. Four wash buffers (autoclaved Milli Q water, 10% (w/v) sucrose, 10% (v/v) glycerol, 30% (v/v) polyethylene glycol (PEG) that have been previously shown to work in either M. buryatense, E. coli, Bacillus or Staphylococcus carnosus (Okamoto et al., 1997; Löfblom et al., 2007; Wu et al., 2010; Yan et al., 2016) were evaluated to enhance electroporation efficiency for M. alcaliphilum. A standard voltage of 1.8 kV was used for this experiment, as has been reported earlier (Elmore et al., 2023). Transformation efficiency was calculated based on the number of colony forming units (CFUs)/µg of plasmid DNA. As shown in Figure 1A, no CFUs were observed on plates with cells washed with 10% sucrose and 30% PEG, indicating that these solutions were not effective under the conditions tested in this transformation process. Plasmids pCAH01 and pCM433 yielded 3 and 4 CFU/μg DNA, respectively, when 10% glycerol was used as wash buffer. The highest CFUs were reported with the use of autoclaved milli Q water, resulting in ∼25 CFU/μg of plasmid for pCM433 (Figure 1A).
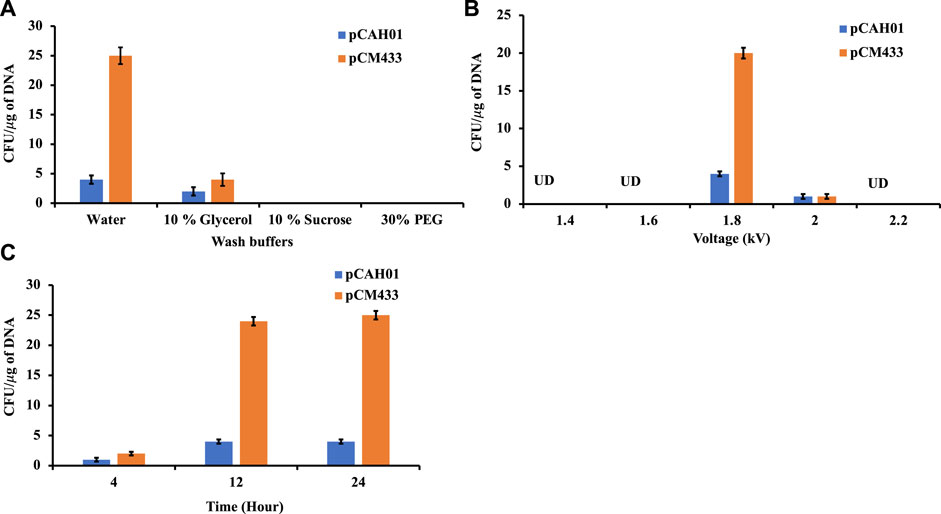
Figure 1. Evaluation of different electroporation conditions for plasmid transformation in Methylotuvimicrobium alcaliphilum strain DASS. (A) Effect of wash buffers on electroporation efficiency; (B) Impact of voltage range; (C) Post electroporation cell recovery time. UD, undetected.
Next, the effect of different electroporation voltages (1.4, 1.6, 1.8, 2.0, 2.2 kV) was tested. CFUs were observed only on 1.8 and 2.0 kV treated cells, with ∼20 fold for pCM433 and ∼4-fold for pCAH01 more CFUs at 1.8 kV than 2.0 kV (Figure 1B). Post shock cell recovery time is a vital factor for viability of cells exposed to extreme voltage shocks. Thus, the duration of cell recovery time can impact the transformation efficiency as observed in the experiments. To evaluate this for M. alcaliphilum DASS, cells were cultivated for 4, 12, and 24 h post electroporation in P3 medium under methane: air (1:1) atmosphere (Figure 1C). Both 12 and 24 h recovery times had comparable CFUs/µg DNA, which were 3- fold higher than CFUs observed for the 4 h recovery, indicating that the post-shock cell recovery time impacts transformation efficiency, and 12 h incubation is optimal. Overall, the optimized electroporation conditions established by these experiments include three critical parameters-cell wash by autoclaved milliQ water, electroporation at 1.8 kV and 12 h post shock cell recovery time for strain M. alcaliphilum DASS.
The optimized condition was then assessed on other replicative plasmids pAWP78 and pCM184 (plasmids of varying sizes; Table 1) for M. alcaliphilum (Figure 2A). The pDNA transformation was successful in each trial (n = 4), confirming the replication of protocol is irrespective of sizes and, cloning or expression plasmid (Table 1). M. alcaliphilum DASS is an evolved strain (Awasthi et al., 2022), and resulting mutations could have impacted the transformation efficiency relative to the parent strain. Therefore, the optimized electroporation protocol was evaluated for the wild type (WT) parent strain DSM19304 to establish the reproducibility of the developed method. Data (Figure 2B) shows the transformation procedure was reproducible in WT.
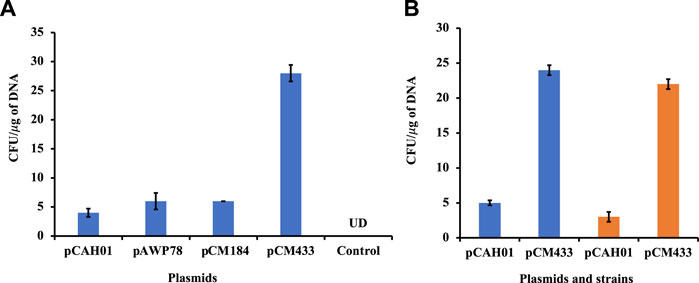
Figure 2. Evaluation of electroporation protocol reproducibility in M. alcaliphilum. (A) Using different replicative vectors; (B) In different variants of M. alcaliphilum, strains DASS (blue) and wild-type DSM19304 (orange). UD, undetected.
In the scope of improving transformation efficiency of plasmids, another strategy that employs specific methylation-based plasmid propagation was also evaluated. Bacterial endonucleases differentiate native DNA from foreign DNA due to differential DNA methylation at specific, short (∼4–8 nucleotides) sequences with using a DNA methyl transferase to protect the host chromosome at sites that are cleaved by a corresponding restriction enzyme. Thus, unmethylated or improperly methylated foreign DNA will be degraded upon entering the cell (Vasu and Nagaraja, 2013). In E. coli, two type of and site-specific DNA methyl transferases- Dam and Dcm have been reported, encoded by dam (DNA adenine methyltransferase) and dcm (DNA cytosine methyltransferase), respectively (Marinus and Løbner-Olesen, 2014). Notably, it has been mentioned that Dam and Dcm methylation pattern might decrease the transformation efficiency in alternate hosts (Marinus and Løbner-Olesen, 2014). Therefore, to further optimize the pDNA transformation efficiency, prior to electroporation in M. alcaliphilum, pCAH01 was propagated in various E. coli strains (Table 1): TOP10F (dam+; DNA adenine methyltransferase, dcm+; DNA cytosine methyltransferase), C2523 (Δdcm), GM272 (Δdam Δdcm). No colonies were obtained on plates with transformed plasmids isolated from E. coli strains C2523, GM272 (Supplementary Figure S1). Similar results after the repeated trials (n = 3) depicts that dam and dcm methylation of E. coli enables transformation of plasmid in M. alcaliphilum, and absence of any methylation pattern were deleterious. In future studies, Methylomicrobium specific methylation should be evaluated if it enhances plasmid transformation efficiency as has been reported in E. coli strain AG5645 (Riley et al., 2023).
Promoter evaluation for heterologous gene expression
Promoters driving gene expression are critical for rational genetic engineering. However, the availability of well characterized promoter systems for inducible and/or constitutive gene expression in non-model hosts like M. alcaliphilum is limited compared to model organisms like E. coli. In this work, to create another inducible system, the 3-Methylbenzoate (MB) inducible promoter (Pm) of Pseudomonas putida (Marqués et al., 1999) was evaluated in M. alcaliphilum using green florescent protein as a reporter on plasmid pSGDA1 (Table 1; Figure 3A). M. alcaliphilum (pSGDA1) cultures were induced by addition of 3MB in increasing concentrations up to 48 h, and 3MB dose dependent response on growth and fluorescence was monitored. Growth of host, measured as optical density (OD600nm), was mostly unaffected by increasing concentration of 3MB till 400 μM, monitored until 48 h (Supplementary Figure S2A). The normalized GFP fluorescence intensity was observed to have increased almost 2-fold from 100 to 400 μM of 3MB, followed by intensity saturation by further increase in 3MB concentrations, indicating optimal activity of Pm promoter in presence of 400 μM 3MB (Figure 3A).
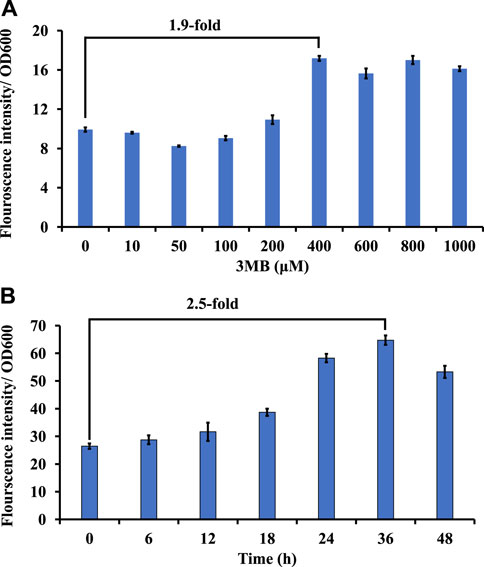
Figure 3. Screening of inducible and constitutive promoters using GFP as a reporter in strain DASS. (A) Growth-normalized fluorescence intensity (GFP409/OD600) for Pm promoter in response to 3MB concentration. (B) Growth-normalized fluorescence intensity (GFP409/OD600) for Psps promoter in P3 media.
Constitutive promoters are known for their constant expression of genes in a host cell, across different set of culturing conditions (Li et al., 2015). This host constitutively produces considerate amount of sucrose to maintain osmotic balance in its native halo-alkaline environment (But et al., 2015). Thus, recently, the constitutive sucrose phosphate synthase promoter (Psps) was shown to drive expression of a recombinant rhamnolipid pathway in strain DASS (Awasthi et al., 2022); however, the strength of this promoter remained uncharacterized. To study the strength and duration of expression, GFP was expressed under the Psps (pSGDA2; Figure 3B). Strain DASS (pSGDA2) cultures were grown till 48 h (Supplementary Figure S2B). Gene expression was observed to be coupled to cell growth, and it peaked at 24–36 h, at the early to mid-stationary phase of growth (Figure 3B) with 2.5-fold increase compared to 0 h.
Discussion
Conjugation is the traditional mode of plasmid (pDNA) transformation for methanotrophs, which requires multiple steps and a long time (∼4 weeks) (Puri et al., 2015), a process limitation that unfortunately holds back the host from fast genetic manipulation time-line, a trait sought for microbial biocatalysts. Thus, in the efforts to make M. alcaliphilum a robust host for strain engineering, it is imperative to address the limitations of established pDNA transformation methods. Electroporation is a one-step, clean pDNA transfer procedure, which also prevents mutations that may occur during plasmid transfer to multiple hosts for mating or conjugating (Huang and Wilks, 2017). Thus, direct plasmid electroporation is time saving, also when compared to other methods demonstrated in methanotrophs, where the re-transformation of plasmids is required from methanotrophs strain back into cloning strain (Yan et al., 2016). In this work, the highest CFUs were reported with the use of autoclaved milli Q water, resulting in ∼25 CFU/μg of plasmid for pCM433. This result affirms the use of autoclaved milli Q water as has been previously shown to work for electroporation in M. buryatense 5G (Yan et al., 2016). The efficiency of transformation represented by CFU/μg DNA, was consistently higher for pCM433 in all trials. pCM433 plasmid is 2.3 kb lesser in size than pCHA01 (Table 1). It was reported previously that transformation efficiency decreases linearly with increase in plasmid size (Hanahan, 1983). This may explain the lesser obtained CFU/μg DNA in pCHA01 compared to pCM433, in this study. Finally, the resulting workflow (Figure 4) shows the overall impact of improving this method, resulting in ∼3 times reduction in the duration and effort of implementing electroporation for heterologous DNA transfer in M. alcaliphilum for expression and cloning vectors. Currently, industrial organisms such as Escherichia coli, Saccharomyces cerevisiae, Pichia pastoris take around 12 h, 2–6 days and 4–6 days respectively to show colonies in selection plates after plasmids transformation. Here in M. alcaliphilum, with the presented approach, colony appearance occurs at ∼5–6 days after transformation in antibiotic selections plates. Therefore, electroporation may help bring the methanotrophs closer to other commonly used industrial hosts in DNA transfer and colony selection timeline.
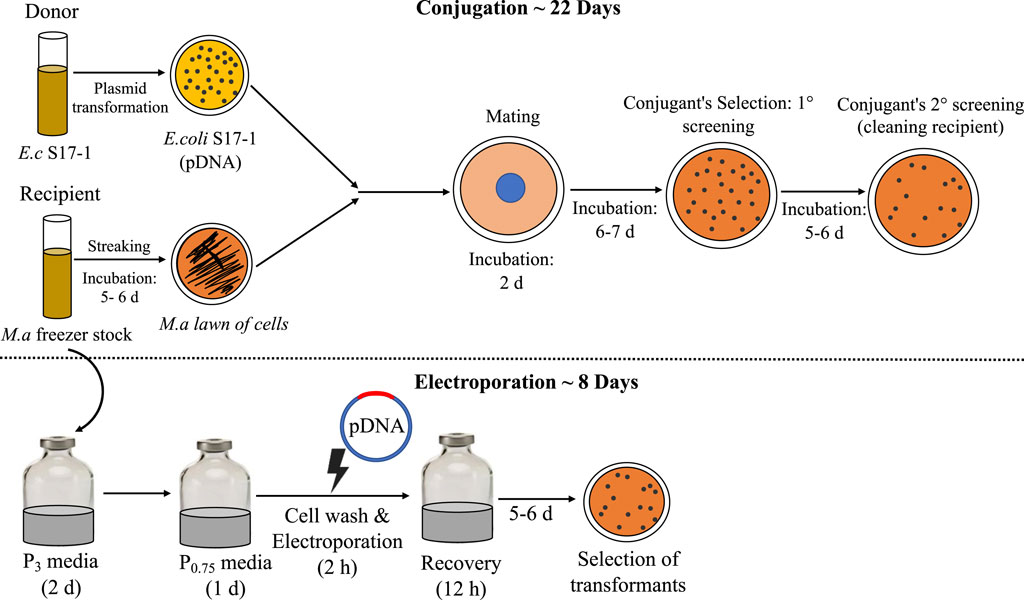
Figure 4. Comparison of conjugation and electroporation: the steps involved and timeline. E.c, E. coli; M.a, M. alcaliphilum.
Regulated promoter-gene systems are vital for gene expression during strain engineering to control/tune recombinant product formation. Thus, to add to the promoter library in M. alcaliphilum, strength of a constitutive promoter Psps (sucrose phosphate synthase) and an inducible promoter, Pm (3-methyl benzoate) were evaluated. In this work, Pm promoter showed a 2-fold increase in presence of 400 µM 3MB. Very recently, the Pm promoter was shown to be 5.7-fold in presence of 1 mM benzoate in Methylococcus capsulatus (Jeong et al., 2023). Though, concentration of inducer above ≥0.4 mM were found to be adversely impacting the growth of the host (Supplementary Figure S2A), the results of this study establish the robustness of the Pm promoter across the genus. A small decrease in OD of Pm promoter by 0.2–0.3 in magnitude, at 36–48 h was observed only at higher concentrations (800–1,000 μM) of 3MB (Supplementary Figure S2B) indicating a stress response by cell at high dose of inducer concentrations (Shis and Bennett, 2013). As an obligate methanotroph, M. alcaliphilum is incapable to metabolize aromatic substrates as a growth or energy source therefore, aromatic substrate-based inducers are a potential range of promoters that could be further evaluated for this host.
Using a constitutive promoter for a desired rate of heterologous gene expression and biochemical production is a common practice in strain engineering (Bienick et al., 2014; Yan and Fong, 2017). In this direction, constitutive gene expression has been previously studied in the Methylotuvimicrobium genus under promoters expressing housekeeping genes like, pmoC, pmoA, pmoB (particulate methane monooxygenase), mxa (methanol dehydrogenase) and hpi (hexulose phosphate isomerase) (Henard and Guarnieri, 2018). A recent report showcased the strength of constitutive promoters such as PDnaA (2.81-fold), PIntegrase (3.45-fold), PrpmB (22.09-fold), P(2Fe–2S)-binding protein (41.54-fold) using GFP as reporter protein in Methylomonas sp. DH-1 (Lee et al., 2021). Psps earlier studied by our group (Awasthi et al., 2022) is shown to efficiently perform 2.5-fold increase in gene expression, in this work. Thus, the endogenous promoter- Psps, inducible promoter- Pm, and electroporation protocol developed in this study, aids to the advancement of genetic engineering practices in M. alcaliphilum and should be evaluated and expanded to other type-1 methanotrophs.
Conclusion
The type-I methanotroph M. alcaliphilum is capable of methane bioconversion and has the potential to become a model cell factory to produce biochemicals and bioproducts from methane. Advancements in genetic tools for these microbes are needed to accelerate their use as-a model host to study methanotrophy, and/or an industrial host. This study showcased an optimized and reproducible electroporation-based plasmid transformation protocol that reduces the time of plasmid transformation from 4–5 weeks to 2 weeks as compared to conventional conjugation-based method. The materials employed for the developed electroporation method are standard materials and an economical wash solution-autoclaved milli Q water. The reproducibility of this protocol was shown with different cloning and expression vectors, as well as, in the lab adapted strain DASS and the wild type parent strain, DSM19304. Additionally, a responsive inducible promoter Pm (inducer 3MB) and a growth based constitutive promoter (Psps) were established for driving efficient gene expression. These methods and promoter systems can be further evaluated for their effectiveness and reproducibility in other type-I methanotrophs.
Data availability statement
The raw data supporting the conclusion of this article will be made available by the authors, without undue reservation.
Author contributions
SG: Data curation, Formal Analysis, Investigation, Methodology, Validation, Visualization, Writing–original draft. SS: Project administration, Supervision, Writing–review and editing. BS: Project administration, Resources, Supervision, Writing–review and editing. DA: Conceptualization, Formal Analysis, Funding acquisition, Methodology, Project administration, Resources, Supervision, Writing–review and editing.
Funding
The author(s) declare that financial support was received for the research, authorship, and/or publication of this article. This work was generously supported by the Laboratory Directed Research and Development Program of Lawrence Berkeley National Laboratory under the US Department of Energy contract no. DE-AC02-05CH11231.
Acknowledgments
We thank Michael Guarnieri of NREL for providing expression and replicative plasmid pCAH01 for Methylotuvimicrobium alcaliphilum. We thank Marina Kalyuzhnaya of SDSU for providing the cloning vectors pCM433 and pCM184. SG thanks Lawrence Berkeley National Laboratory (LBNL) for the postdoctoral scholar fellowship. We would like to thank Justin Tan from University of California, Berkeley for his support in promoter screening work.
Conflict of interest
The authors declare that the research was conducted in the absence of any commercial or financial relationships that could be construed as a potential conflict of interest.
Publisher’s note
All claims expressed in this article are solely those of the authors and do not necessarily represent those of their affiliated organizations, or those of the publisher, the editors and the reviewers. Any product that may be evaluated in this article, or claim that may be made by its manufacturer, is not guaranteed or endorsed by the publisher.
Supplementary material
The Supplementary Material for this article can be found online at: https://www.frontiersin.org/articles/10.3389/fbioe.2024.1412410/full#supplementary-material
References
Awasthi, D., Tang, Y.-H., Amer, B., Baidoo, E. E. K., Gin, J., Chen, Y., et al. (2022). Adaptive evolution of Methylotuvimicrobium alcaliphilum to grow in the presence of rhamnolipids improves fatty acid and rhamnolipid production from CH4. J. Industrial Microbiol. Biotechnol. 49, kuac002. doi:10.1093/jimb/kuac002
Bienick, M. S., Young, K. W., Klesmith, J. R., Detwiler, E. E., Tomek, K. J., and Whitehead, T. A. (2014). The interrelationship between promoter strength, gene expression, and growth rate. PLOS ONE 9, e109105. doi:10.1371/journal.pone.0109105
Boucher, O., Friedlingstein, P., Collins, B., and Shine, K. P. (2009). The indirect global warming potential and global temperature change potential due to methane oxidation. Environ. Res. Lett. 4, 044007. doi:10.1088/1748-9326/4/4/044007
But, S. Y., Khmelenina, V. N., Reshetnikov, A. S., Mustakhimov, I. I., Kalyuzhnaya, M. G., and Trotsenko, Y. A. (2015). Sucrose metabolism in halotolerant methanotroph Methylomicrobium alcaliphilum 20Z. Arch. Microbiol. 197, 471–480. doi:10.1007/s00203-015-1080-9
Demidenko, A., Akberdin, I. R., Allemann, M., Allen, E. E., and Kalyuzhnaya, M. G. (2017). Fatty acid biosynthesis pathways in Methylomicrobium buryatense 5G(B1). Front. Microbiol. 7, 2167. doi:10.3389/fmicb.2016.02167
Elmore, J. R., Dexter, G. N., Baldino, H., Huenemann, J. D., Francis, R., Peabody, G. L., et al. (2023). High-throughput genetic engineering of nonmodel and undomesticated bacteria via iterative site-specific genome integration. Sci. Adv. 9, eade1285. doi:10.1126/sciadv.ade1285
Gęsicka, A., Oleskowicz-Popiel, P., and Łężyk, M. (2021). Recent trends in methane to bioproduct conversion by methanotrophs. Biotechnol. Adv. 53, 107861. doi:10.1016/j.biotechadv.2021.107861
Gibson, D. G., Young, L., Chuang, R. Y., Venter, J. C., Hutchison, C. A., and Smith, H. O. (2009). Enzymatic assembly of DNA molecules up to several hundred kilobases. Nat. Methods 6, 343–345. doi:10.1038/nmeth.1318
Guerrero-Cruz, S., Vaksmaa, A., Horn, M. A., Niemann, H., Pijuan, M., and Ho, A. (2021). Methanotrophs: discoveries, environmental relevance, and a perspective on current and future applications. Front. Microbiol. 12, 678057. doi:10.3389/fmicb.2021.678057
Hanahan, D. (1983). Studies on transformation of Escherichia coli with plasmids. J. Mol. Biol. 166, 557–580. doi:10.1016/s0022-2836(83)80284-8
Hanson, R. S., and Hanson, T. E. (1996). Methanotrophic bacteria. Microbiol. Rev. 60, 439–471. doi:10.1128/mmbr.60.2.439-471.1996
Henard, C. A., Akberdin, I. R., Kalyuzhnaya, M. G., and Guarnieri, M. T. (2019). Muconic acid production from methane using rationally-engineered methanotrophic biocatalysts. Green Chem. 21, 6731–6737. doi:10.1039/c9gc03722e
Henard, C. A., and Guarnieri, M. T. (2018). “Metabolic engineering of methanotrophic bacteria for industrial biomanufacturing,” in Methane biocatalysis: paving the way to sustainability. Editors M. G. KALYUZHNAYA,, and X.-H. XING (Cham: Springer International Publishing).
Henard, C. A., Smith, H., Dowe, N., Kalyuzhnaya, M. G., Pienkos, P. T., and Guarnieri, M. T. (2016). Bioconversion of methane to lactate by an obligate methanotrophic bacterium. Sci. Rep. 6, 21585. doi:10.1038/srep21585
Huang, W., and Wilks, A. (2017). A rapid seamless method for gene knockout in Pseudomonas aeruginosa. BMC Microbiol. 17, 199. doi:10.1186/s12866-017-1112-5
Hwang, I. Y., Nguyen, A. D., Nguyen, T. T., Nguyen, L. T., Lee, O. K., and Lee, E. Y. (2018). Biological conversion of methane to chemicals and fuels: technical challenges and issues. Appl. Microbiol. Biotechnol. 102, 3071–3080. doi:10.1007/s00253-018-8842-7
In Yeub, H., Dong Hoon, H., Jae Hoon, L., Chang-Ho, P., In Seop, C., Jin Won, L., et al. (2015). Batch conversion of methane to methanol using methylosinus trichosporium OB3b as biocatalyst. J. Microbiol. Biotechnol. 25, 375–380. doi:10.4014/jmb.1412.12007
In Yeub, H., Seung Hwan, L., Yoo Seong, C., Si Jae, P., Jeong Geol, N., In Seop, C., et al. (2014). Biocatalytic conversion of methane to methanol as a key step for development of methane-based biorefineries. J. Microbiol. Biotechnol. 24, 1597–1605. doi:10.4014/jmb.1407.07070
Jeong, J., Kim, T. H., Jang, N., Ko, M., Kim, S. K., Baek, J. I., et al. (2023). A highly efficient and versatile genetic engineering toolkit for a methanotroph-based biorefinery. Chem. Eng. J. 453, 139911. doi:10.1016/j.cej.2022.139911
Lee, H.-M., Ren, J., Yu, M.-S., Kim, H., Kim, W. Y., Shen, J., et al. (2021). Construction of a tunable promoter library to optimize gene expression in Methylomonas sp. DH-1, a methanotroph, and its application to cadaverine production. Biotechnol. Biofuels 14, 228. doi:10.1186/s13068-021-02077-8
Liang, B., Fu, R., Ma, Y., Hu, L., Fei, Q., and Xing, X.-H. (2022). Turning C1-gases to isobutanol towards great environmental and economic sustainability via innovative biological routes: two birds with one stone. Biotechnol. Biofuels Bioprod. 15, 107. doi:10.1186/s13068-022-02202-1
Li, S., Wang, J., Li, X., Yin, S., Wang, W., and Yang, K. (2015). Genome-wide identification and evaluation of constitutive promoters in streptomycetes. Microb. Cell Factories 14, 172. doi:10.1186/s12934-015-0351-0
Löfblom, J., Kronqvist, N., Uhlén, M., Ståhl, S., and Wernérus, H. (2007). Optimization of electroporation-mediated transformation: Staphylococcus carnosus as model organism. J. Appl. Microbiol. 102, 736–747. doi:10.1111/j.1365-2672.2006.03127.x
Marinus, M. G., and Løbner-Olesen, A. (2014). DNA methylation. EcoSal Plus 6. doi:10.1128/ecosalplus.esp-0003-2013
Marqués, S., Manzanera, M., González-Pérez, M.-M., Gallegos, M.-T., and Ramos, J. L. (1999). The XylS-dependent Pm promoter is transcribed in vivo by RNA polymerase with σ32 or σ38 depending on the growth phase. Mol. Microbiol. 31, 1105–1113. doi:10.1046/j.1365-2958.1999.01249.x
Marx, C. J., and Lidstrom, M. E. (2002). Broad-host-range cre-lox system for antibiotic marker recycling in gram-negative bacteria. BioTechniques 33, 1062–1067. doi:10.2144/02335rr01
Nguyen, A. D., Hwang, I. Y., Lee, O. K., Kim, D., Kalyuzhnaya, M. G., Mariyana, R., et al. (2018). Systematic metabolic engineering of Methylomicrobium alcaliphilum 20Z for 2,3-butanediol production from methane. Metab. Eng. 47, 323–333. doi:10.1016/j.ymben.2018.04.010
Ojala, D. S., Beck, D. A., and Kalyuzhnaya, M. G. (2011). Genetic systems for moderately halo(alkali)philic bacteria of the genus Methylomicrobium. Methods Enzymol. 495, 99–118. doi:10.1016/b978-0-12-386905-0.00007-3
Okamoto, A., Kosugi, A., Koizumi, Y., Yanagida, F., and Udaka, S. (1997). High efficiency transformation of Bacillus brevis by electroporation. Biosci. Biotechnol. Biochem. 61, 202–203. doi:10.1271/bbb.61.202
Puri, A. W., Owen, S., Chu, F., Chavkin, T., Beck, D. A., Kalyuzhnaya, M. G., et al. (2015). Genetic tools for the industrially promising methanotroph Methylomicrobium buryatense. Appl. Environ. Microbiol. 81, 1775–1781. doi:10.1128/aem.03795-14
Rhee, S.-K., Awala, S. I., and Nguyen, N.-L. (2019). “Enrichment and isolation of aerobic and anaerobic methanotrophs,” in Methanotrophs: Microbiology fundamentals and biotechnological applications. Editor E. Y. LEE (Cham: Springer International Publishing).
Riley, L. A., Payne, I. C., Tumen-Velasquez, M., and Guss, A. M. (2023). Simple and rapid site-specific integration of multiple heterologous DNAs into the Escherichia coli chromosome. J. Bacteriol. 205, e0033822. doi:10.1128/jb.00338-22
Shis, D. L., and Bennett, M. R. (2013). Library of synthetic transcriptional AND gates built with split T7 RNA polymerase mutants. Proc. Natl. Acad. Sci. 110, 5028–5033. doi:10.1073/pnas.1220157110
Strong, P. J., Xie, S., and Clarke, W. P. (2015). Methane as a resource: can the methanotrophs add value? Environ. Sci. Technol. 49, 4001–4018. doi:10.1021/es504242n
Vasu, K., and Nagaraja, V. (2013). Diverse functions of restriction-modification systems in addition to cellular defense. Microbiol. Mol. Biol. Rev. 77, 53–72. doi:10.1128/mmbr.00044-12
Wuebbles, D. J., and Hayhoe, K. (2002). Atmospheric methane and global change. Earth-Science Rev. 57, 177–210. doi:10.1016/s0012-8252(01)00062-9
Wu, N., Matand, K., Kebede, B., Acquaah, G., and Williams, S. (2010). Enhancing DNA electrotransformation efficiency in Escherichia coli DH10B electrocompetent cells. Electron. J. Biotechnol. 13, 0–22. doi:10.2225/vol13-issue5-fulltext-11
Yan, Q., and Fong, S. S. (2017). Challenges and advances for genetic engineering of non-model bacteria and uses in consolidated bioprocessing. Front. Microbiol. 8, 2060. doi:10.3389/fmicb.2017.02060
Keywords: electroporation, Methylomicrobium alcaliphilum, constitutive promoter, inducible promoter, methanotrophs
Citation: Goswami S, Singer SW, Simmons BA and Awasthi D (2024) Optimization of electroporation method and promoter evaluation for type-1 methanotroph, Methylotuvimicrobium alcaliphilum. Front. Bioeng. Biotechnol. 12:1412410. doi: 10.3389/fbioe.2024.1412410
Received: 04 April 2024; Accepted: 26 April 2024;
Published: 15 May 2024.
Edited by:
Sujit Jagtap, University of Illinois at Urbana-Champaign, United StatesReviewed by:
Ashwini Bedekar, University of Illinois at Urbana-Champaign, United StatesPrimata Mardina, Lambung Mangkurat University, Indonesia
Akash Patil, Braskem America Inc., United States
Copyright © 2024 Goswami, Singer, Simmons and Awasthi. This is an open-access article distributed under the terms of the Creative Commons Attribution License (CC BY). The use, distribution or reproduction in other forums is permitted, provided the original author(s) and the copyright owner(s) are credited and that the original publication in this journal is cited, in accordance with accepted academic practice. No use, distribution or reproduction is permitted which does not comply with these terms.
*Correspondence: Deepika Awasthi, ZGF3YXN0aGlAbGJsLmdvdg==