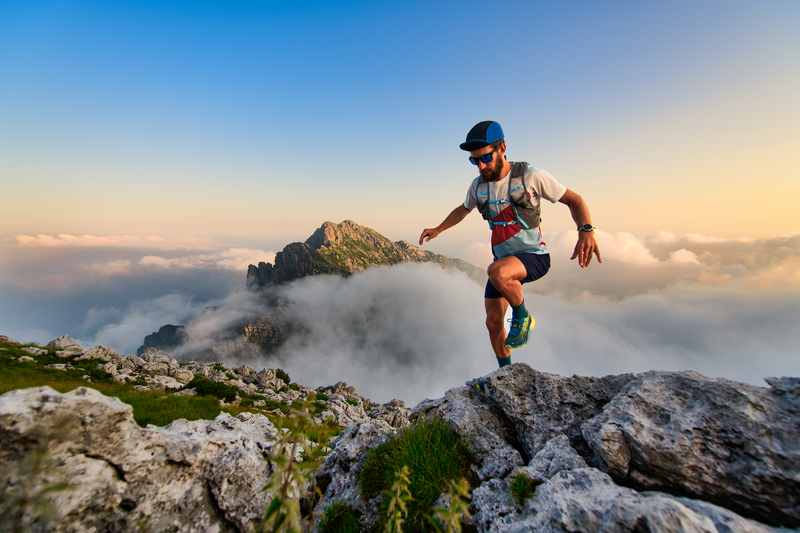
95% of researchers rate our articles as excellent or good
Learn more about the work of our research integrity team to safeguard the quality of each article we publish.
Find out more
BRIEF RESEARCH REPORT article
Front. Bioeng. Biotechnol. , 15 May 2024
Sec. Synthetic Biology
Volume 12 - 2024 | https://doi.org/10.3389/fbioe.2024.1398467
This article is part of the Research Topic Microbial Synthetic Biology Drives the Carbon Source Revolution View all 5 articles
Acetogens are among the key microorganisms involved in the bioproduction of commodity chemicals from diverse carbon resources, such as biomass and waste gas. Thermophilic acetogens are particularly attractive because fermentation at higher temperatures offers multiple advantages. However, the main target product is acetic acid. Therefore, it is necessary to reshape metabolism using genetic engineering to produce the desired chemicals with varied carbon lengths. Although such metabolic engineering has been hampered by the difficulty involved in genetic modification, a model thermophilic acetogen, M. thermoacetica ATCC 39073, is the case with a few successful cases of C2 and C3 compound production, other than acetate. This brief report attempts to expand the product spectrum to include C4 compounds by using strain Y72 of Moorella thermoacetica. Strain Y72 is a strain related to the type strain ATCC 39073 and has been reported to have a less stringent restriction-modification system, which could alleviate the cumbersome transformation process. A simplified procedure successfully introduced a key enzyme for acetoin (a C4 chemical) production, and the resulting strains produced acetoin from sugars and gaseous substrates. The culture profile revealed varied acetoin yields depending on the type of substrate and culture conditions, implying the need for further engineering in the future. Thus, the use of a user-friendly chassis could benefit the genetic engineering of M. thermoacetica.
One of the global challenges in this modern era is establishing a sustainable society free from fossil resource-dependent industries. A key factor is the utilization of sustainable resources, such as biomass, and recycling of used materials. In this context, one of the promising approaches will be the biological conversion of these resources to conventional chemicals.
Acetogens are a group of microorganisms with unique and versatile metabolic pathways that catabolize both biomass-derived sugars and gaseous substrates to produce mainly acetic acid. Near stoichiometric conversion of hexose sugar to acetic acid (one hexose molecule is converted to three acetic acid molecules) is possible, inferring great metabolic potential (Drake and Daniel, 2004). Moreover, the gaseous substrates include hydrogen (H2), carbon monoxide (CO), and carbon dioxides (CO2), which can be derived not only from waste gases but also from the gasification of organic materials, including recalcitrant resources, such as lignin from biomass. Therefore, acetogens are promising candidates for biological catalysts that produce conventional chemicals by utilizing sustainable resources. In particular, thermophilic acetogens have multiple advantages over others, such as low risk of contamination, lower cooling cost, faster reaction speed, and simplified product recovery (Taylor et al., 2009; Abdel-Banat et al., 2010; Redl et al., 2017; Kato et al., 2021).
For the practical application of thermophilic acetogens, it is essential to produce chemicals that are more diverse than acetate. One method is to screen for new strains with the metabolic capacity to produce these chemicals. Another method is genetic engineering of thermophilic acetogens to introduce new metabolic pathways and tune innate pathways. However, acetogens are known for their inaccessibility in genetic modification (Bourgade et al., 2021). To date, the most successful cases of metabolic engineering to produce other chemicals have been achieved using the model thermophilic acetogen Moorella thermoacetica ATCC 39073. These cases are limited to the production of C2 and C3 compounds, such as ethanol (C2), lactate, acetone, and isopropanol (C3) (Iwasaki et al., 2017; Rahayu et al., 2017; Kato et al., 2021; Kato et al., 2024). Metabolic engineering has been accomplished by overcoming genetic barriers via DNA premethylation (Kita et al., 2013a). However, the method is cumbersome, enabling only a small set of engineered strains obtained.
Previously, another strain of M. thermoacetica, Y72, was reported to be genetically more accessible than ATCC 39073, because of its less strict restriction-modification system (Kita et al., 2013b). The genome is closely related to ATCC 39073, according to the draft genome sequence, with a similar size and the same GC content (56%) (Tsukahara et al., 2014). Phylogenetic analysis revealed that Y72 belongs to the same clade as ATCC 39073, and an average nucleotide analysis further supported the similarity of the two genomes (Redl et al., 2019). Despite this similarity, strain Y72 possesses fewer genes for the restriction-modification system than ATCC 39073, which is assumed to be the reason why Y72 shows high transformation efficiency (Tsukahara et al., 2014). Therefore, Y72 may be useful as an alternative strain for the model ATCC 39073 for metabolic engineering in introducing new pathways.
Acetoin (3-hydroxy-2-butanone) is an important C4 chemical in the food, medical, and chemical industries and was selected by the U.S. Department of Energy (DOE) as one of the 30 compounds that require prioritized production from biomass sugars and syngas (Werpy et al., 2004). However, genetic engineering of thermophilic acetogens for acetoin production has not yet been reported. Moreover, no studies have reported the production of C4 chemicals using thermophilic acetogens.
In this study, metabolic engineering for the C4 chemical production by a thermophilic acetogen was attempted by taking advantage of the strain M. thermoacetica Y72, with acetoin as the target product. Furthermore, engineered strains were evaluated as the place to study for chassis development.
A pyrF knockout strain of M. thermoacetica Y72 was used in this study for genetic transformation (Kita et al., 2013b). The pyrF knockout strain and the derivative strains were cultured in serum bottles under anaerobic conditions at 55°C unless otherwise noted.
The culture medium was prepared by mixing 1.0 g of NH4Cl, 0.1 g of KCl, 0.2 g of MgSO4·7H2O, 0.8 g of NaCl, 0.1 g of KH2PO4, 0.02 g of CaCl2·2H2O, 2.0 g of NaHCO3, 10 mL of trace elements, 10 mL of Wolfe’s vitamin solution, and 1.0 mg of resazurin per liter of water (Tanner, 1989; Tanner et al., 1993). After adjusting the pH to 6.9 with 6N HCl, the medium was prepared anaerobically by boiling and cooling under an N2/CO2 (80/20 ratio) atmosphere. After cooling, the medium was dispensed into 125-mL serum bottles under N2/CO2 atmosphere. Serum bottles were crimp-sealed and autoclaved. Yeast extract and l-cysteine·H2O stock solutions were prepared separately and added to the medium before inoculation to reach a final concentration of 1.0 and 1.2 g/L, respectively. The total medium volume was adjusted to 50 mL. When sugars were supplied as substrates, stock solutions were prepared separately and added to the medium at a final concentration of 10–11 mM. Gaseous substrates were added to the headspace of the bottles by adjusting the partial pressure during injection. When syngas was used, CO and H2 were injected at 0.04 MPa, respectively. When CO2+H2 gas was used, the headspace was replaced with a mixed gas (CO2:H2 = 8:2) and adjusted to 0.2 MPa. The seed culture was grown on fructose, and used for sugar-based cultures and CO2+H2 gas-based culture (5% inoculum). In the case of syngas-based culture, the sugar-based seed culture was subsequently inoculated to a syngas-containing medium, which was used as the seed culture. All the inoculum was from the exponential phase.
The DNA sequence of the bsALDC from Bacillus subtilis IPE5-4 was codon-optimized for M. thermoacetica and synthesized (Genewiz). The PCR primers used for the following procedure are listed in Supplementary Table S1. Plasmid pHM23 for the introduction of bsALDC into pduL2 region was constructed as follows. Primers JK109 and JK110 were used for PCR amplification of the bsALDC sequence. The amplified fragment was cloned using the In-Fusion HD Cloning kit (Takara) into the PCR-amplified plasmid using primer sets JK52 and JK71 with pK18-ΔpduL2::ldh as the template (Iwasaki et al., 2017). The plasmid pK18-ΔpyrF::bsALDC, which was used to introduce bsALDC into pyrF region, was constructed in the same manner. Primers, bsALDC_FW bsALDC_RV, and a template, pHM23, were used for the insert. Primers, Vec_FW and Vec_RV, and a template, pK18-kan2 (Iwasaki et al., 2013), were used for the vector. The constructed plasmids were introduced into M. thermoacetica Y72 following an electroporation protocol described previously, except for omitting the DNA premethylation procedure (Kita et al., 2013b; Kato et al., 2021). Homologous recombination was confirmed via PCR amplification using the primer sets pduL2_check_FW and pduL2_check_Rv for the pduL2 locus, and pyrF72_upF and pyrF_downR for the pyrF locus.
To analyze cell growth and products, 1 mL of the culture was sampled. Cell growth was determined by measuring the optical density (OD) at 600 nm. The products in the supernatant were analyzed via HPLC (LC-2000 Plus HPLC; Jasco, Tokyo, Japan) using a refractive index detector (RI-2031 Plus; Jasco). Shodex RSpak KC-811 column (Showa Denko, Kanagawa, Japan) and Shodex RSpak KC-G guard column (Showa Denko) were used. The column temperature was maintained at 60°C, and the isocratic method was used in 0.1% (v/v) phosphoric acid at a flow rate of 0.7 mL/min.
One route for the biological production of acetoin is decarboxylation of acetolactate (Maina et al., 2022). Acetolactate is a primary metabolic intermediate in the synthesis of branched-chain amino acids, such as valine, leucine, and isoleucine. Because M. thermoacetica Y72 can grow in the basal medium which does not contain these amino acids derived from acetolactate, the strain Y72 should be able to synthesize acetolactate by itself (Kita et al., 2013b). Furthermore, acetolactate is synthesized from pyruvate by acetolactate synthase, which is involved in both heterotrophic and autotrophic growth of acetogens (Schuchmann and Muller, 2014). The genome contains genes encoding acetolactate synthase, whose amino acid sequence is highly similar to those in the ATCC 39073 (similarity >99%). Therefore, the introduction of a decarboxylase specific to acetolactate could enable acetoin production in the homoacetogen M. thermoacetica Y72.
As shown in Figure 1A, one hexose sugar molecule can be converted into one acetoin molecule by the introduction of acetolactate decarboxylase. In this metabolic pathway, two adenosine triphosphate (ATP) molecules are involved in glycolysis and support cellular energy production. Notably, the two NADH molecules were produced by reducing NAD+ and were not oxidized or recycled. Such a redox imbalance causes metabolic arrest, and it is important to maintain acetate production to some extent for NAD+/NADH maintenance.
Figure 1. Design of metabolic pathways for acetoin production in Moorella thermoacetica. Pathways for acetate and acetoin production from sugars (A) and gaseous substrates (B). The substrates are colored orange, and the key enzymatic reaction of bsALDC for acetoin production is shown in red.
In contrast, on gaseous substrates, two molecules of CO2 or CO were converted into one acetyl-CoA molecule. Acetyl-CoA is converted to acetate, coupled with substrate-level phosphorylation (SLP), for ATP formation in acetogenesis, which would cover a large portion of the cellular energy supply for autotrophic growth. When acetoin is produced, two acetyl-CoA molecules are used for one acetoin molecule (Figure 1B). As a result, the acetyl-CoA molecules that are supposed to be used for SLP are lost, resulting in an insufficient ATP supply. It is important to maintain a certain level of acetate production to achieve sufficient SLP. Thus, these strains were designed to preserve acetate production.
Acetolactate decarboxylase was selected for heterologous expression analysis. Because the enzyme must be tolerant to the elevated temperatures in thermophiles, bsALDC from B. subtilis IPE5-4 was selected (Jia et al., 2017). Enzymatic activity was maintained at high levels at the optimum growth temperature of M. thermoacetica (Drake and Daniel, 2004).
To heterologously express bsALDC in M. thermoacetica Y72, the coding nucleotide sequence was codon-optimized and placed under the constitutive glycerol-3-phosphate dehydrogenase (G3PD) promoter. The plasmid DNA construct was designed to introduce the promoter-gene set (PG3PD-bsALDC), either at the pyrF or pduL2 locus of the chromosome, by homologous recombination. The PG3PD-bsALDC was placed in homologous regions upstream and downstream of pyrF and pduL2 (Figure 2A). pyrF gene was used as a selection marker to transform the pyrF-knockout strain, which is uracil auxotroph (Kita et al., 2013b). When the PG3PD-bsALDC was introduced into the pyrF locus, the acetate production pathway remained intact. When the PG3PD-bsALDC was introduced into the pduL2 locus, acetate production was lowered due to the disruption of one of the two genes encoding phosphoacetyl transferase.
Figure 2. DNA construction for introducing bsALDC into the chromosome of Moorella thermoacetica Y72 by homologous recombination. (A) shows the schematic representation of the constructed plasmids and the homologous recombination events in the pyrF or pduL2 region. (B) shows the agarose gel electrophoresis following PCR amplification of the pyrF or pduL2 region. The size shifts of the amplified DNA confirmed the successful introduction of the bsALDC constructs in the pyrF or pduL2 region. 1.9 kb of the pyrF region was shifted to 2.7, and 0.9 kb of the pduL2 region was shifted to 2.3 kb. M, DNA size marker; lane 1, the Y72-Km strain; lane 2, the Y72-pyrF::bsALDC strain; lane 3, the Y72-pduL2::bsALDC strain.
The resulting plasmids were introduced into M. thermoacetica Y72 without any customized DNA modifications. Despite skipping the modification step, the target strains were successfully obtained (Figure 2B).
Two engineered strains containing bsALDC were tested for acetoin production from sugars. A hexose sugar, fructose, was used for the fermentation. First, a strain with an intact acetate production pathway, Y72-pyrF::bsALDC, was cultured (Figure 3A). The strain grew with two end-products in the culture supernatant. The major product was acetate, accompanied by much less acetoin. After consuming all the supplemented fructose (11.1 mM), the acetate concentration reached 21.5 mM and the acetoin concentration reached 2.3 mM. As a result, 0.21 mol-acetoin/mol-fructose was obtained.
Figure 3. Acetoin production from sugars. The cell growth (OD), substrate consumption, acetate and acetoin productions were monitored over time in the fructose-supplemented culture. The Y72-pyrF::bsALDC (A) and the Y72-pduL2::bsALDC (B) strains are shown, respectively. (C) The acetoin and acetate production yields by the Y72-pduL2::bsALDC strain from fructose were compared between 55°C and 60°C. (D) The acetoin and acetate production yields by the Y72-pduL2::bsALDC strain were compared between hexose (fructose) and pentose (xylose) sugars. Data are presented as the mean with the SDs of three biological replicates. Some error bars are smaller than the symbols of data plots.
Next, the Y72-pduL2::bsALDC strain, with a deficient acetate production pathway (pduL2 knockout), was cultured under the same conditions (Figure 3B). The strain grew with two end-products of different profiles. The amount of acetoin was much higher, reaching 7.7 mM, whereas the acetate production decerased to 9.1 mM. The tuning of carbon flow by pduL2 knockout was effective in enhancing the target product, acetoin. The maximum cell growth was lower than that of the Y72-pyrF::bsALDC strain, most likely because of lowered ATP production coupled with acetate production. It is known that the cellular biomass amount is correlated with the energy production (Bauchop and Elsden, 1960).
Using the Y72-pduL2::bsALDC strain, a few additional features were characterized. As the optimum temperature for the M. thermoacetica growth is between 55°C and 60°C, the effect of temperature was compared in this range (Figure 3C). The acetoin yield, 0.72 mol-acetoin/mol-fructose at 55°C, was lowered to 0.22 mol-acetoin/mol-fructose at 60°C. A different sugar, xylose (pentose), was tested instead of fructose (hexose) (Figure 3D). Acetoin was produced from xylose at the yield of 0.34 mol-acetoin/mol-xylose at 55°C, which is lower than that from fructose by half. Acetoin yield tended to decrease in both cases.
Following the demonstration of acetoin production from sugars, acetoin production from gaseous substrates was tested. We selected syngas (CO, H2 and CO2) and CO2+H2 gas, because the autotrophic growth and chemical production would be different based on the energy source (Hu et al., 2016); CO provides higher energy than H2. While syngas contains both CO and H2 as energy sources, CO2+H2 gas contains only H2.
When the Y72-pduL2::bsALDC strain was cultured in the syngas, the strain grew with abundant acetate production (Figure 4A). Acetoin production was 20-fold less than acetate production (0.5 mM vs 9.5 mM). Formate, an intermediate in the metabolism of CO2, was not observed, indicating that the sufficient ATP was provided. ATP was required to process formate to formyl-THF (Figure 1B). However, when the same strain was cultured in CO2+H2 gas, the OD value remained almost constant, indicating that the cells did not grow. As the major metabolite, a significant amount of formate was accumulated, indicating that ATP was in short supply (Figure 4B), consistent with the growth profile. Acetate was gradually accumulated (2.4 mM). Acetoin was also produced and accumulated gradually; however, the amount remained limited (0.6 mM), as in the syngas culture.
Figure 4. Acetoin production from gaseous substrates. The cell growth (OD) and the production of acetate, acetoin, and formate were monitored over time. Syngas (A) or CO2+H2 gas (B) was supplemented in the head space of culture vials. Data are presented as the mean with SDs of three (A) and two (B) biological replicates, respectively. Some error bars are smaller than the symbols of data plots. Fructose concentration was shown in (B) to indicate its absence and the residual amount from the seed culture.
This brief report describes the metabolic conversion of a thermophilic homoacetogen, M. thermoacetica Y72, using genetic engineering. The strain originally produced only acetate (Kita et al., 2013b), and the successful introduction of a key gene conferred the acetoin production. This was the first reported case of C4 chemical production by a thermophilic acetogen. Strain Y72 has been reported to be less stringent in the restriction-modification system, providing high transformation efficiency. A simplified procedure without customized DNA premodification may be helpful in accelerating the metabolic engineering of thermophilic acetogens. There are a few reports concerning acetoin production by thermophiles, but not by thermophilic acetogens that utilize gaseous substrates (Xiao et al., 2012; Sheng et al., 2023).
Two strains for acetoin production were constructed by tuning carbon flux. Based on the design of the metabolic pathways (Figure 1), certain levels of acetate production are needed to maintain metabolism in terms of the metabolic redox state and energy supply. The Y72-pduL2::bsALDC strain showed higher acetoin production than the Y72-pyrF::bsALDC strain, demonstrating that knockout of a gene in the acetate pathway was effective in improving acetoin production.
Varying the fermentation temperature and sugar substrate (hexose to pentose) maintained acetoin production itself; however, these changes significantly affected the yield. The differences could be derived from the responsible enzymatic activities, levels of gene expression, or metabolic redox state, in addition to the original metabolic features of M. thermoacetica Y72. For example, both key enzymatic activities, PduL1 for acetate and bsALDC for acetoin, were higher at 60°C than at 55°C in vitro (Breitkopf et al., 2016; Jia et al., 2017). Furthermore, PduL1 activity was more enhanced to a greater extent by a temperature shift (Breitkopf et al., 2016). These differences may have contributed to different culture profiles at different temperatures. In addition, it has been reported that the NAD(P)/NAD(P)H ratio affects distribution of the metabolic flux during acetoin and byproducts (Bao et al., 2015). The difference due to sugar type could be derived from this redox state (Bao et al., 2015). Some of these factors may affect the consequences of competing chemical productions. In future studies, the involvement of these multiple factors should be investigated to optimize the acetoin production from sugars.
Acetoin production from gaseous substrates has been demonstrated using syngas and CO2+H2 gas. In both cases, small amounts of acetoin were produced. One reason for this low production may be the utilization of pyruvate as a key intermediate. Whereas the conversion of acetyl-CoA to acetate provides ATP, the conversion of acetyl-CoA to pyruvate requires an energy source (Figure 1B). Because the energy provision from gaseous substrates is low (Schuchmann and Muller, 2014; Debabov, 2021), cellular growth or anabolic metabolism in acetogens is limited; hence, pyruvate production is limited. Energy shortage was evident in the culture profile, especially when H2 was solely provided as the energy source, with inactive cell growth and excess formate accumulation. A drastic strategy is necessary for higher acetoin production through enhanced pyruvate production accompanied by sufficient energy in gaseous substrates. However, the detectable level of acetoin indicated that the redox state of metabolism was not as heavily impaired as that of the ethanol-producing Mt-ΔpduL2::aldh strain (Takemura et al., 2021). The Mt-ΔpduL2::aldh strain had a pduL2 knockout and enhanced the expression of aldehyde dehydrogenase for ethanol production. However, it only produced formate and acetate in CO2+H2 gas.
Acetoin production by a thermophilic acetogen was demonstrated by successful genetic engineering of M. thermoacetica Y72 using a simplified procedure. The engineered strains produced acetoin from diverse carbon sources, and the culture profiles indicated metabolic bottlenecks that warrant further investigation. Notably, the acetoin productivity needs to be far more enhanced for economically viable production. Nevertheless, this study is valued in a concrete progress for the development of a microbial chassis of thermophilic acetogens for the carbon conversion, such as sugars and carbon-rich gases.
The original contributions presented in the study are included in the article/Supplementary Material, further inquiries can be directed to the corresponding author.
JK: Conceptualization, Data curation, Investigation, Methodology, Visualization, Writing–original draft, Writing–review and editing. TF: Conceptualization, Data curation, Investigation, Methodology, Writing–review and editing. SK: Writing–review and editing. KW: Writing–review and editing. MW: Writing–review and editing. YusN: Writing–review and editing. YA: Writing–review and editing. TM: Writing–review and editing. KM: Writing–review and editing. YutN: Conceptualization, Funding acquisition, Project administration, Writing–review and editing.
The author(s) declare that financial support was received for the research, authorship, and/or publication of this article. This study was supported by the JST-Mirai Program (Grant Number JPMJMI18E5, Japan).
We thank Hikaru Miyaoka for providing technical assistance.
This work has been included in a patent application by Hiroshima University and AIST.
All claims expressed in this article are solely those of the authors and do not necessarily represent those of their affiliated organizations, or those of the publisher, the editors and the reviewers. Any product that may be evaluated in this article, or claim that may be made by its manufacturer, is not guaranteed or endorsed by the publisher.
The Supplementary Material for this article can be found online at: https://www.frontiersin.org/articles/10.3389/fbioe.2024.1398467/full#supplementary-material
Abdel-Banat, B. M., Hoshida, H., Ano, A., Nonklang, S., and Akada, R. (2010). High-temperature fermentation: how can processes for ethanol production at high temperatures become superior to the traditional process using mesophilic yeast? Appl. Microbiol. Biotechnol. 85, 861–867. doi:10.1007/s00253-009-2248-5
Bao, T., Zhang, X., Zhao, X., Rao, Z., Yang, T., and Yang, S. (2015). Regulation of the NADH pool and NADH/NADPH ratio redistributes acetoin and 2,3-butanediol proportion in Bacillus subtilis. Biotechnol. J. 10, 1298–1306. doi:10.1002/biot.201400577
Bauchop, T., and Elsden, S. R. (1960). The growth of micro-organisms in relation to their energy supply. J. Gen. Microbiol. 23, 457–469. doi:10.1099/00221287-23-3-457
Bourgade, B., Minton, N. P., and Islam, M. A. (2021). Genetic and metabolic engineering challenges of C1-gas fermenting acetogenic chassis organisms. FEMS Microbiol. Rev. 45, fuab008. doi:10.1093/femsre/fuab008
Breitkopf, R., Uhlig, R., Drenckhan, T., and Fischer, R. J. (2016). Two propanediol utilization-like proteins of Moorella thermoacetica with phosphotransacetylase activity. Extremophiles 20, 653–661. doi:10.1007/s00792-016-0854-6
Debabov, V. G. (2021). Acetogens: biochemistry, bioenergetics, genetics, and biotechnological potential. Microbiology 90, 273–297. doi:10.1134/s0026261721030024
Drake, H. L., and Daniel, S. L. (2004). Physiology of the thermophilic acetogen Moorella thermoacetica. Res. Microbiol. 155, 869–883. doi:10.1016/j.resmic.2004.10.002
Hu, P., Chakraborty, S., Kumar, A., Woolston, B., Liu, H., Emerson, D., et al. (2016). Integrated bioprocess for conversion of gaseous substrates to liquids. Proc. Natl. Acad. Sci. U. S. A. 113, 3773–3778. doi:10.1073/pnas.1516867113
Iwasaki, Y., Kita, A., Sakai, S., Takaoka, K., Yano, S., Tajima, T., et al. (2013). Engineering of a functional thermostable kanamycin resistance marker for use in Moorella thermoacetica ATCC39073. FEMS Microbiol. Lett. 343, 8–12. doi:10.1111/1574-6968.12113
Iwasaki, Y., Kita, A., Yoshida, K., Tajima, T., Yano, S., Shou, T., et al. (2017). Homolactic acid fermentation by the genetically engineered thermophilic homoacetogen Moorella thermoacetica ATCC 39073. Appl. Environ. Microbiol. 83, e00247. doi:10.1128/aem.00247-17
Jia, X., Liu, Y., and Han, Y. (2017). A thermophilic cell-free cascade enzymatic reaction for acetoin synthesis from pyruvate. Sci. Rep. 7, 4333. doi:10.1038/s41598-017-04684-8
Kato, J., Matsuo, T., Takemura, K., Kato, S., Fujii, T., Wada, K., et al. (2024). Isopropanol production via the thermophilic bioconversion of sugars and syngas using metabolically engineered Moorella thermoacetica. Biotechnol. Biofuels Bioprod. 17, 13. doi:10.1186/s13068-024-02460-1
Kato, J., Takemura, K., Kato, S., Fujii, T., Wada, K., Iwasaki, Y., et al. (2021). Metabolic engineering of Moorella thermoacetica for thermophilic bioconversion of gaseous substrates to a volatile chemical. Amb. Express 11, 59. doi:10.1186/s13568-021-01220-w
Kita, A., Iwasaki, Y., Sakai, S., Okuto, S., Takaoka, K., Suzuki, T., et al. (2013a). Development of genetic transformation and heterologous expression system in carboxydotrophic thermophilic acetogen Moorella thermoacetica. J. Biosci. Bioeng. 115, 347–352. doi:10.1016/j.jbiosc.2012.10.013
Kita, A., Iwasaki, Y., Yano, S., Nakashimada, Y., Hoshino, T., and Murakami, K. (2013b). Isolation of thermophilic acetogens and transformation of them with the pyrF and kanr genes. Biosci. Biotechnol. Biochem. 77, 301–306. doi:10.1271/bbb.120720
Maina, S., Prabhu, A. A., Vivek, N., Vlysidis, A., Koutinas, A., and Kumar, V. (2022). Prospects on bio-based 2,3-butanediol and acetoin production: recent progress and advances. Biotechnol. Adv. 54, 107783. doi:10.1016/j.biotechadv.2021.107783
Rahayu, F., Kawai, Y., Iwasaki, Y., Yoshida, K., Kita, A., Tajima, T., et al. (2017). Thermophilic ethanol fermentation from lignocellulose hydrolysate by genetically engineered Moorella thermoacetica. Bioresour. Technol. 245, 1393–1399. doi:10.1016/j.biortech.2017.05.146
Redl, S., Poehlein, A., Esser, C., Bengelsdorf, F. R., Jensen, T. O., Jendresen, C. B., et al. (2019). Genome-based comparison of all species of the genus Moorella, and status of the species Moorella thermoacetica and Moorella thermoautotrophica. Front. Microbiol. 10, 3070. doi:10.3389/fmicb.2019.03070
Redl, S., Sukumara, S., Ploeger, T., Wu, L., Olshoj Jensen, T., Nielsen, A. T., et al. (2017). Thermodynamics and economic feasibility of acetone production from syngas using the thermophilic production host Moorella thermoacetica. Biotechnol. Biofuels 10, 150. doi:10.1186/s13068-017-0827-8
Schuchmann, K., and Muller, V. (2014). Autotrophy at the thermodynamic limit of life: a model for energy conservation in acetogenic bacteria. Nat. Rev. Microbiol. 12, 809–821. doi:10.1038/nrmicro3365
Sheng, L., Madika, A., Lau, M. S. H., Zhang, Y., and Minton, N. P. (2023). Metabolic engineering for the production of acetoin and 2,3-butanediol at elevated temperature in Parageobacillus thermoglucosidasius NCIMB 11955. Front. Bioeng. Biotechnol. 11, 1191079. doi:10.3389/fbioe.2023.1191079
Takemura, K., Kato, J., Kato, S., Fujii, T., Wada, K., Iwasaki, Y., et al. (2021). Autotrophic growth and ethanol production enabled by diverting acetate flux in the metabolically engineered Moorella thermoacetica. J. Biosci. Bioeng. 132, 569–574. doi:10.1016/j.jbiosc.2021.08.005
Tanner, R. S. (1989). Monitoring sulfate-reducing bacteria - comparison of enumeration media. J. Microbiol. Methods 10, 83–90. doi:10.1016/0167-7012(89)90004-3
Tanner, R. S., Miller, L. M., and Yang, D. (1993). Clostridium ljungdahlii sp. nov., an acetogenic species in clostridial rRNA homology group I. Int. J. Syst. Bacteriol. 43, 232–236. doi:10.1099/00207713-43-2-232
Taylor, M. P., Eley, K. L., Martin, S., Tuffin, M. I., Burton, S. G., and Cowan, D. A. (2009). Thermophilic ethanologenesis: future prospects for second-generation bioethanol production. Trends Biotechnol. 27, 398–405. doi:10.1016/j.tibtech.2009.03.006
Tsukahara, K., Kita, A., Nakashimada, Y., Hoshino, T., and Murakami, K. (2014). Genome-guided analysis of transformation efficiency and carbon dioxide assimilation by Moorella thermoacetica Y72. Gene 535, 150–155. doi:10.1016/j.gene.2013.11.045
Werpy, T. A., Petersen, G. E., Aden, A., Bozell, J. J., Holladay, J. E., White, J. F., et al. (2004). Top value added chemicals from biomass: volume I - results of screening for potential candidates from sugars and synthesis gas. Tech. Rep. Top Value Added Chem. Biomass 1. doi:10.2172/15008859
Keywords: thermophilic acetogen, genetic engineering, C4 chemicals, acetoin, biomass, gaseous substrates
Citation: Kato J, Fujii T, Kato S, Wada K, Watanabe M, Nakamichi Y, Aoi Y, Morita T, Murakami K and Nakashimada Y (2024) Genetic engineering of a thermophilic acetogen, Moorella thermoacetica Y72, to enable acetoin production. Front. Bioeng. Biotechnol. 12:1398467. doi: 10.3389/fbioe.2024.1398467
Received: 10 March 2024; Accepted: 29 April 2024;
Published: 15 May 2024.
Edited by:
Fu-Li Li, Chinese Academy of Sciences (CAS), ChinaReviewed by:
Zijun Xiao, China University of Petroleum (East China), ChinaCopyright © 2024 Kato, Fujii, Kato, Wada, Watanabe, Nakamichi, Aoi, Morita, Murakami and Nakashimada. This is an open-access article distributed under the terms of the Creative Commons Attribution License (CC BY). The use, distribution or reproduction in other forums is permitted, provided the original author(s) and the copyright owner(s) are credited and that the original publication in this journal is cited, in accordance with accepted academic practice. No use, distribution or reproduction is permitted which does not comply with these terms.
*Correspondence: Yutaka Nakashimada, bnl1dGFrYUBoaXJvc2hpbWEtdS5hYy5qcA==
†These authors have contributed equally to this work
Disclaimer: All claims expressed in this article are solely those of the authors and do not necessarily represent those of their affiliated organizations, or those of the publisher, the editors and the reviewers. Any product that may be evaluated in this article or claim that may be made by its manufacturer is not guaranteed or endorsed by the publisher.
Research integrity at Frontiers
Learn more about the work of our research integrity team to safeguard the quality of each article we publish.