- 1Department of Plastic Surgery, Zhejiang Rongjun Hospital, Jiaxing, Zhejiang, China
- 2General Surgery Department, Jiaxing No.1 Hospital, Jiaxing, Zhejiang, China
With the rapid development of synthetic biology, recombinant human collagen has emerged as a cutting-edge biological material globally. Its innovative applications in the fields of material science and medicine have opened new horizons in biomedical research. Recombinant human collagen stands out as a highly promising biomaterial, playing a pivotal role in crucial areas such as wound healing, stroma regeneration, and orthopedics. However, realizing its full potential by efficiently delivering it for optimal therapeutic outcomes remains a formidable challenge. This review provides a comprehensive overview of the applications of recombinant human collagen in biomedical systems, focusing on resolving this crucial issue. Additionally, it encompasses the exploration of 3D printing technologies incorporating recombinant collagen to address some urgent clinical challenges in regenerative repair in the future. The primary aim of this review also is to spotlight the advancements in the realm of biomaterials utilizing recombinant collagen, with the intention of fostering additional innovation and making significant contributions to the enhancement of regenerative biomaterials, therapeutic methodologies, and overall patient outcomes.
1 Introduction
Collagen, the most abundant protein in the extracellular matrix of animal cells, plays a pivotal role in providing structural support and regulating cellular behavior (Avila Rodríguez et al., 2018; Coppola et al., 2020). To date, 29 types of collagens have been identified, with types I, II, and III constituting over 90% of the total collagen in the human body (Meyer, 2019; Naomi et al., 2021). Collagen provides tensile strength and is the primary component of skin, bones, cartilage, and connective tissues (Law et al., 2017; Jafari et al., 2020). Due to its biocompatibility, biodegradability, and low immunogenicity, collagen has been extensively investigated and employed as a biomaterial in the field of tissue engineering and regenerative medicine (Irawan et al., 2018; Copes et al., 2019; Lin et al., 2019).
However, the majority of collagen used for biomedical applications is still derived from animal sources, such as the skin, tendons, and bones of bovines, pigs, and avian species (Avila Rodríguez et al., 2018; Felician et al., 2018). Animal-sourced collagen has inherent drawbacks. It exhibits batch-to-batch variability in quantity and quality, can potentially trigger immunogenic responses, and carries the risk of transmitting animal viruses and prions (Gauza-Włodarczyk et al., 2017a; Coppola et al., 2020). Despite the success of animal-derived collagen products like Zyderm, the pursuit of recombinant collagen aims to refine the safety and efficacy of collagen-based treatment (Shekhter et al., 2019). Produced through intricate in vitro techniques, recombinant collagens are designed to emulate the post-translational modifications seen in natural collagens, such as hydroxylation and glycosylation, thereby achieving a high degree of similarity to human collagen (Kim et al., 2017). Recombinant human collagen produced via biotechnological methods can overcome these limitations associated with xenogeneic or allogeneic collagen (Wang et al., 2022). Over the past few decades, research on recombinant collagen has made significant strides in genetic recombination, protein expression, and material preparation (Ma et al., 2022). This article provides a comprehensive review of the latest advancements in recombinant human collagen and its applications as biomaterials in tissue engineering and regenerative medicine.
Collagen possesses a characteristic triple-helical structure, composed of three polypeptide chains known as α-chains (Mi et al., 2018; Rappu et al., 2019). In vitro studies have recombinantly produced different types of collagens from isolated genes, including types I, II, III, and V collagen (Woodley et al., 2017; Doan et al., 2019; Shuai et al., 2023). Compared to natural collagen, recombinantly produced collagen achieves proper post-translational modifications, including hydroxylation and glycosylation (Shekhter et al., 2019; Deng et al., 2021). Based on the composition of α-chains, recombinant collagen can be categorized into homotrimeric (I, II, III), heterotrimeric (XI), and hybrid forms (IX) (Ferraro et al., 2017; Chen et al., 2020). Type I collagen is the most abundant type in many tissues, while type III collagen is relatively less abundant but plays a crucial role in maintaining tissue integrity and regulating scar formation (Kuivaniemi and Tromp, 2019; Di Martino et al., 2022; Harris et al., 2022). Extensive research has also been focused on type III collagen due to its therapeutic potential in promoting wound healing and tissue regeneration (Zhang Wei et al., 2018a; Xia et al., 2018; Davison-Kotler et al., 2019).
To produce recombinant collagen, expression systems including mammalian, insect, yeast, and bacterial cells have been explored (Capella-Monsonís et al., 2018; Davison-Kotler et al., 2019). Mammalian cells like CHO and HEK293 have translation mechanisms most similar to human cells and can therefore produce collagen with correct modifications (Gauza-Włodarczyk et al., 2017b; Lim et al., 2019). However, their relatively low yield and high cost hinder industrial-scale production (Felician et al., 2018; Song et al., 2018; Meng et al., 2019). Bacterial and yeast expression systems are more cost-effective but do not achieve proper post-translational processing (Amyoony et al., 2023). Therefore, strategies have been developed to enhance the quality of recombinantly produced collagen by genetically modifying host cells or supplementing post-translational enzymes. Recent research has made significant strides in optimizing expression systems and purifying large quantities of structurally native collagen (Knüppel et al., 2017; Rittié, 2017).
Following purification, recombinant collagen is fabricated into various biomaterials for biomedical applications (Zhang et al., 2019; Ghomi et al., 2021). Collagen hydrogels prepared by differential mechanisms have been extensively studied as scaffolds and drug delivery carriers (Li et al., 2020). By adjusting the hydrogel density, degree of cross-linking, and the incorporation of other biomolecules, its degradation, mechanical strength, and biological activity can be customized. The inclusion of growth factors and cells further enhances the regenerative potential of collagen hydrogels (Arakawa et al., 2017; Sarrigiannidis et al., 2021). Lyophilized collagen materials processed through freeze-drying present another format as wound dressings (Magro et al., 2017). Scaffold formats of collagen have been expanded further by various techniques including 3D printing, electrospinning, and particle sintering (Rashedi et al., 2017; Zhang et al., 2018b; Abbas et al., 2020). Functionalizing recombinant collagen biomaterials with nanoparticles, peptides, and stem cells has emerged as a promising strategy for precisely guiding tissue regeneration.
In tissue engineering applications, recombinant collagen biomaterials have been widely studied for skin regeneration due to the natural abundance of collagen in the dermis (Koons et al., 2020). Collagen hydrogels promote wound healing by stimulating cell proliferation, migration, angiogenesis, and collagen deposition (Nguyen et al., 2019; Roshanbinfar et al., 2023). When used as covers for skin grafts or wound dressings, they accelerate re-epithelialization. For load-bearing tissues, collagen scaffolds combined with stem cells hold potential in bone and cartilage regeneration. Upon implantation of collagen/stem cell constructs, substantial new bone and cartilage formation was observed in animal models (Wang et al., 2020). In vascular engineering, cell-seeded collagen tubular scaffolds have demonstrated the ability to remodel into vascular grafts (Copes et al., 2019; Minor and Kareen, 2020). New evidence has also validated the feasibility of using collagen implants to repair damaged myocardium and cornea. Looking forward, the development of optimized recombinant collagen production, functional biomaterial design, and translational research will further expand its regenerative applications (Carrabba and Madeddu, 2018; Minor and Kareen, 2020). The future of recombinant human collagen lies in overcoming the limitations of animal-sourced collagen and propelling the development in the fields of tissue engineering and regenerative medicine. Establishing stable high-yield expression systems and purification processes for industrial-scale production remains a major challenge.
This review summarizes the research on recombinant human collagen’s applications in biomedical systems, including its effects in wound treatment, stroma regeneration, and orthopedics. We explore studies on recombinant collagen-based hydrogels, scaffolds, microspheres, and dressings for healing wounds, regenerating skin, and engineering bone tissue. The review also encapsulates research on 3D printings containing recombinant collagen (Table 1). Our goal is to shed light on the advancements and inspire further innovations in recombinant collagen’s biomaterial and clinical uses, with the hope that ongoing development will improve biomaterials, therapies, and patient outcomes.
2 Hydrogel delivery of recombinant collagen for chronic wounds healing
Chronic wounds, including diabetic foot ulcers, are characterized by impaired healing and persistent inflammation (Mathew-Steiner et al., 2021). The wounds become trapped in a prolonged inflammatory stage and are unable to progress through the normal phases of healing (Las Heras et al., 2020). This results in significantly delayed closure compared to acute wounds. Chronic wounds also frequently become colonized with bacteria, leading to infection. The sustained inflammatory environment causes continuous tissue breakdown and inhibits cell proliferation and angiogenesis (Han and Roger, 2017).
Recombinant collagen scaffolds offer several advantages for chronic wound treatment (Sun et al., 2018). As the major structural component of the extracellular matrix, collagen provides an ideal environment to facilitate cell migration and enable wound closure (Koehler et al., 2018; Sun et al., 2018). Recombinant collagen allows precise control over scaffold properties like porosity and bioactivity (Catanzano et al., 2021). Despite these benefits, challenges remain in optimizing delivery of recombinant collagen to improve healing. Fast degradation rates make it difficult to achieve sustained collagen presence within dynamic wound environments. Enhancing collagen scaffold stability through chemical or physical crosslinking may help prolong bioactivity but can also negatively impact integration with native tissue (Thapa et al., 2020). Therefore, effective chronic wound therapies will likely require recombinant collagen delivery platforms that balance scaffold remodeling with regeneration of functional tissue (Ahmad et al., 2021). Further research is needed to translate the promise of recombinant collagen into effective wound treatments that overcome the barriers to healing in chronic wounds.
RhCol III, the primary collagen type in early granulation tissue, shows potential for accelerating wound closure. Hydrogels composed of rhCoI lII have been developed. These hydrogels feature porous microstructure, near-physiological swelling ratios, and significant cell adhesion. In vivo testing in diabetic mice demonstrated expedited wound closure with rhCol III treatment compared to controls. The hydrogels provide a moist environment conducive to healing and act as an in situ forming scaffold for cell migration.
In a study, Wang et al. developed a specialized recombinant human type III collagen (rhCol III) and constructed a multifunctional, microenvironment-responsive hydrogel system integrating this custom rhCol III and multifunctional antimicrobial nanoparticles (PDA@Ag NPs) (Hu et al., 2021). This advanced hydrogel showcases accelerated degradation in the setting of chronic diabetic wounds, orchestrating the regulated and demand-driven release of various therapeutic agents. Initially, the hydrogel releases PDA@Ag NPs which possess potent antimicrobial activity against Staphylococcus aureus and Escherichia coli, thereby facilitating rapid bacterial eradication. Concurrently, these nanoparticles exhibit antioxidant and anti-inflammatory properties within the wound environment. Subsequently, the release of rhCol III stimulates the proliferation and migration of murine fibroblasts and endothelial cells during the proliferative and remodeling phases of wound healing. Upon exposure to a diabetic wound site with bacterial infection, the hydrogel encounters an environment rich in reactive oxygen species and characterized by low pH, indicative of inflammation. This specific environment triggers a rapid dissolution of the boronic ester bonds within the hydrogel structure, causing it to collapse and enabling the staged release of PDA@Ag NPs and rhCol III.
As a result, the hydrogel framework collapses, facilitating the staged discharge of PDA@Ag NPs and rhCol III (Figure 1A). The Scanning Electron Microscopy (SEM) findings depicted in Figure 1B illustrate the spherical form of both PDA and PDA@Ag NPs. The payloads encapsulated within the hydrogel demonstrate a pH-sensitive release dynamic, where the rate of release notably escalates under the more acidic conditions of pH 5 (Figure 1C). The agar plate counting experiment demonstrated that the hydrogel@Ag&rhCol III group exhibited the most substantial antibacterial efficiency, as indicated by the fewest bacterial colonies (Figure 1D). The efficacy of the hydrogel in facilitating chronic wound healing was evaluated using a rat wound model infected with E. coli. Among all groups, the hydrogel@Ag&rhCol III group exhibited the most rapid wound healing, achieving a 64% wound healing rate by day 7 (Figures 1E, F). As presented in Figure 1G, the hydrogel@Ag&rhCol III group exhibited notably elevated levels of bFGF expression compared to other groups. This observation implies that the hydrogel@Ag&rhCol III has the potential to amplify the expression of bFGF, thereby fostering enhanced cell proliferation and angiogenesis.
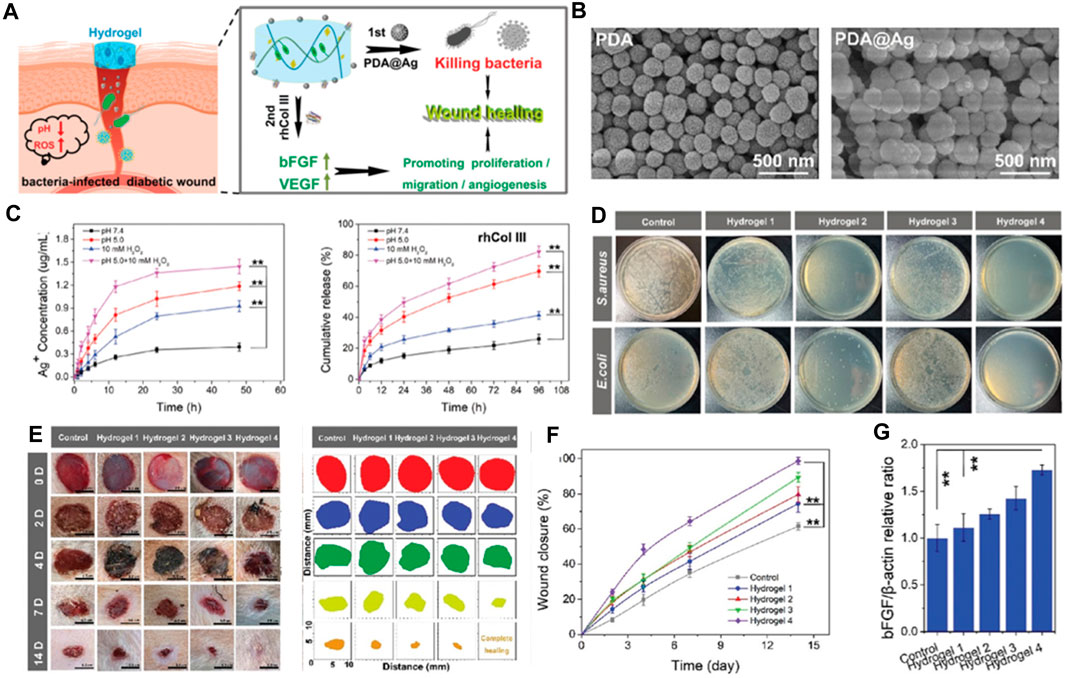
FIGURE 1. (A) The microenvironment-responsive hydrogel and its therapeutic mechanism contributing to the promotion of chronic wound healing. (B) The SEM images of for PDA and PDA@Ag NPs. (C) The release profiles of payloads from hydrogel. (D) Representative images of S. aureus and E. coli following a 12-h treatment with various hydrogel formulations. (E) Illustrative examples of the progression of wound closure on days 0, 2, 4, 7, and 14 (right) after being subjected to different treatments at predetermined time points (left, n = 8). (F) Over the course of 14 days, the rate of wound contraction was also tracked and quantified. (G) The proportional protein expression ratio of bFGF to b-actin. Note the following hydrogel types: (1) Hydrogel 1: Control hydrogel. (2) Hydrogel 2: Hydrogel encapsulating PDA@Ag nanoparticles. (3) Hydrogel 3: Hydrogel encapsulating rhCol III. (4) Hydrogel 4: Hydrogel encapsulating both PDA@Ag and rhCol III. Reproduced with permission from ref Hu et al. (2021).
To summarize, the hydrogel responsive to microenvironmental changes has shown exceptional capabilities in combating bacteria and promoting cell growth and movement, successfully speeding up the healing process of chronic diabetic wounds in both laboratory and real-world scenarios. This research confirms the significant potential of newly designed rhCol III for use in mending and regenerating long-term wounds. As the authers look ahead, they foresee the creation and implementation of further bespoke products based on recombinant human collagen, contributing to advancements in human health and wellbeing.
Wang et al. centers on a pivotal study that delves into the application of recombinant human collagen III protein hydrogels and extracellular vesicles (EVs) in skin wound healing (Figure 2A) (Xu et al., 2022). The research team conducted a series of experiments to assess the efficacy of these hydrogels and EVs in promoting wound healing. The findings suggest that the hydrogels capably released the EVs, thereby stimulating cell proliferation, migration, and angiogenesis (Figures 2B–D). Initially, the study underscores the crucial role of skin as a protective barrier for the human body and tackles the challenges associated with the wound healing process, such as diabetes, vascular insufficiency, and local pressure alterations. The authors elucidate that collagen III, a vital component of the extracellular matrix, plays a significant role in wound healing (Figures 2E, F). They delineate the preparation process of recombinant human collagen III protein hydrogels and discuss the role of EVs in sustained therapeutic agent release for wound healing promotion.
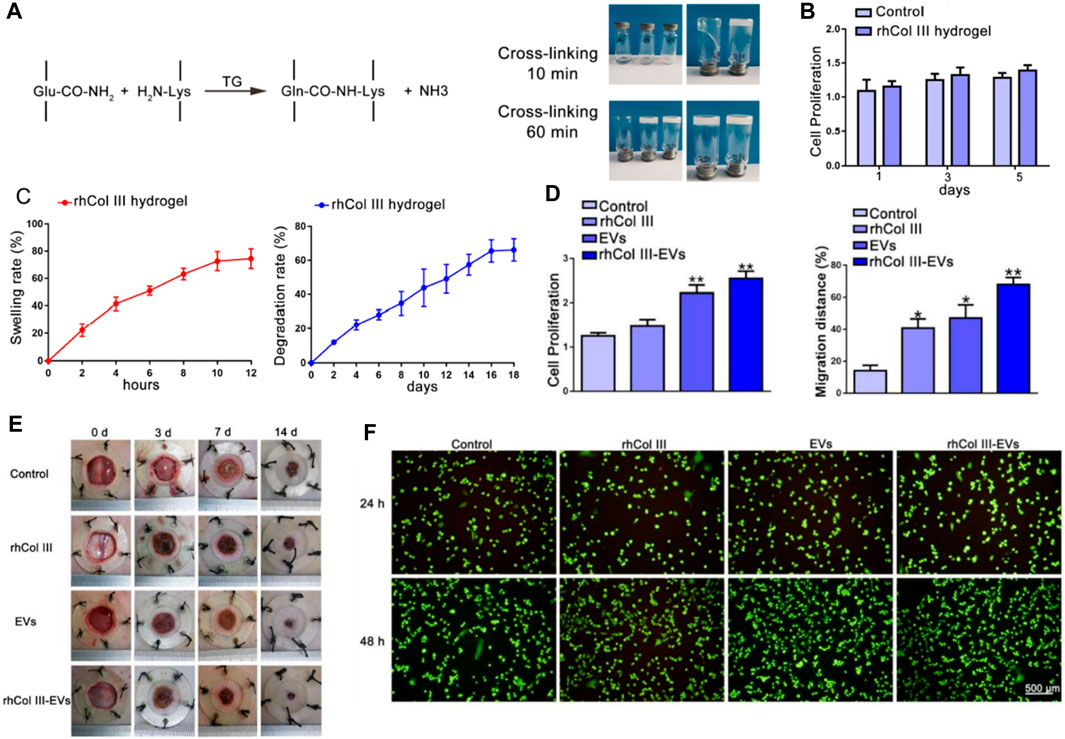
FIGURE 2. (A) Illustrative representation of the procedure for preparing the recombinant human collagen III (rhCol III) hydrogel. (B) Impact of the rhCol III hydrogel extract on cellular proliferation. (C) Swelling ratio observed in the rhCol III hydrogel and the rate of degradation identified in the rhCol III hydrogel. (D) Proliferations and migration distance of L292 cells in rhCol III hydrogel. (E) images of wounds from each group, taken at baseline (0 days) and subsequent timepoints (3, 7, and 14 days). (F) Comparison of angiogenesis in human umbilical vein endothelial cells (HUVECs) in various groups. Reproduced with permission from ref Xu et al. (2022).
The researchers conducted several experiments to determine the efficacy of these hydrogels and EVs in wound healing. The hydrogels successfully released the EVs, enhancing cell proliferation, migration, and angiogenesis. They also suppressed the inflammatory response and promoted wound healing in a diabetic rat skin injury model. The study concludes that these hydrogels and EVs hold significant potential in skin wound healing, presenting a novel approach for chronic wound treatment. In summary, the authors offer an intricate discourse on the employment of hydrogels as a delivery system for recombinant collagen, and the utilization of EVs for sustained therapeutic agent release. They effectively illustrate that the hydrogels proficiently discharge the EVs, thereby facilitating wound healing in a diabetic rat skin injury model. This insight contributes a novel and promising stratagem to the therapeutic repertoire for chronic wound management.
Presently, hydrogels stand as an encouraging scaffold material for tissue engineering and regenerative strategies, largely due to their high-water content, tissue-like mechanical properties, and adjustable physical features (Kathawala et al., 2019; Su et al., 2021). Collagen-based hydrogels, in particular, are appealing for their ability to mimic the extracellular matrix of connective tissues. Recombinant collagen boasts several advantages over tissue-extracted collagen, such as enhanced standardization and tunability, and it circumvents issues of immunogenicity or pathogen transmission (Muhonen et al., 2017). Nevertheless, striking the right balance between factors like swelling, degradation, pore size, and mechanics remains a challenge in optimizing hydrogel design and collagen incorporation. Moreover, the production of most recombinant collagen relies on mammalian cell culture systems, adding a considerable cost (Chen et al., 2020). There is a call for further research to boost recombinant collagen yields and devise efficient purification strategies to curtail expenses (Chen et al., 2022). In summary, while recombinant collagen-loaded hydrogels offer a promising path in tissue repair, further optimization and cost-cutting measures are essential to usher these technologies from the laboratory to clinical practice. Future research should focus on scalable recombinant collagen production, the incorporation of cell instructive signals, and in vivo assessment of performance and host response.
3 The broad application potential of recombinant collagen in corneal stroma regeneration
Recombinant collagen has emerged as a promising biomaterial for various regenerative medicine applications owing to its versatility, biocompatibility, and improved safety compared to animal-derived collagens (Strauss and Chmielewski, 2017). As the most abundant protein in the human body and a major component of connective tissue, collagen plays a critical role in supporting cell growth, adhesion, and organization during tissue regeneration (Sheehy et al., 2018). Recombinant collagen can be biosynthesized using genetic engineering approaches, allowing precise control over collagen type, structure, degradation kinetics, and functionalization with biological signals (Felician et al., 2018). This advanced engineering of molecular and material properties makes recombinant collagen highly adaptable for developing scaffolds, hydrogel, coatings, and delivery systems tailored to promote regeneration across diverse tissues including skin, bone, cartilage, vasculature, and others (Addi et al., 2017; Quinlan et al., 2017; Yang et al., 2021a). The modular and customizable nature of recombinant collagen, along with its inherent bioactivity and biodegradability, enables the design of therapeutic platforms that synergize with endogenous regenerative processes (McPhail et al., 2020). Further research and clinical translation of recombinant collagen-based therapies holds promise for enabling more effective and safer regenerative medicine solutions. In 2021, Sun et al. developed recombinant human collagen hydrogels with hierarchically ordered microstructures to regenerate corneal stroma (Kong et al., 2022). The RHC are modified with methacrylate anhydride (MA) to mimic native corneal properties. The collagen hydrogels have aligned microgrooves and inverse opal nanopores (MI-RHCMA). In vitro experiments show MI-RHCMA hydrogels guided organized growth and differentiation of limbal stromal stem cells into keratocytes compared to random collagen gels. In vivo rat studies demonstrated MI-RHCMA implants integrate with host tissue and regenerate damaged corneal stroma better than controls.
In their research, RHCMA was engineered by integrating MA onto the collagen macromolecular chain via a condensation reaction between amino and carboxyl groups, as depicted in Figure 3A. This method effectively maintained the inherent superior biocompatibility of collagen hydrogel. The distinctive structure of the MI-RHCMA hydrogel patch is clearly illustrated in Figure 3B. The cross-sectional view showcases the convexity and indentation of the microgrooves, which further reveal the existence of inverse opal pores within the microgroove. Figures 3C, D provide a graphical representation of the compressive strain-stress relationship, along with the maximum compressive stress experienced by the RHCMA hydrogel when submerged in PBS. When it comes to cell behavior, LSSCs displayed a tendency to form an organized and elongated structure on MI-RHCMA hydrogel patches, contrasting with their random distribution on unpatterned RHCMA hydrogel surfaces, as shown in Figure 3E. The surgical and post-surgical observations are depicted in Figure 3F. MI-RHCMA hydrogel patches were grafted onto the left eyes of rats, with the right eyes serving as controls. These assessments were performed immediately post-surgery and at 1, 2, and 4 weeks following the operation. Figure 3G presents the results of a histological analysis for measuring corneal stromal and epithelial thickness. Interestingly, no significant statistical variation was observed in the thickness of the corneal epithelium across the allograft, MI-RHCMA, RHCMA, and native corneas.
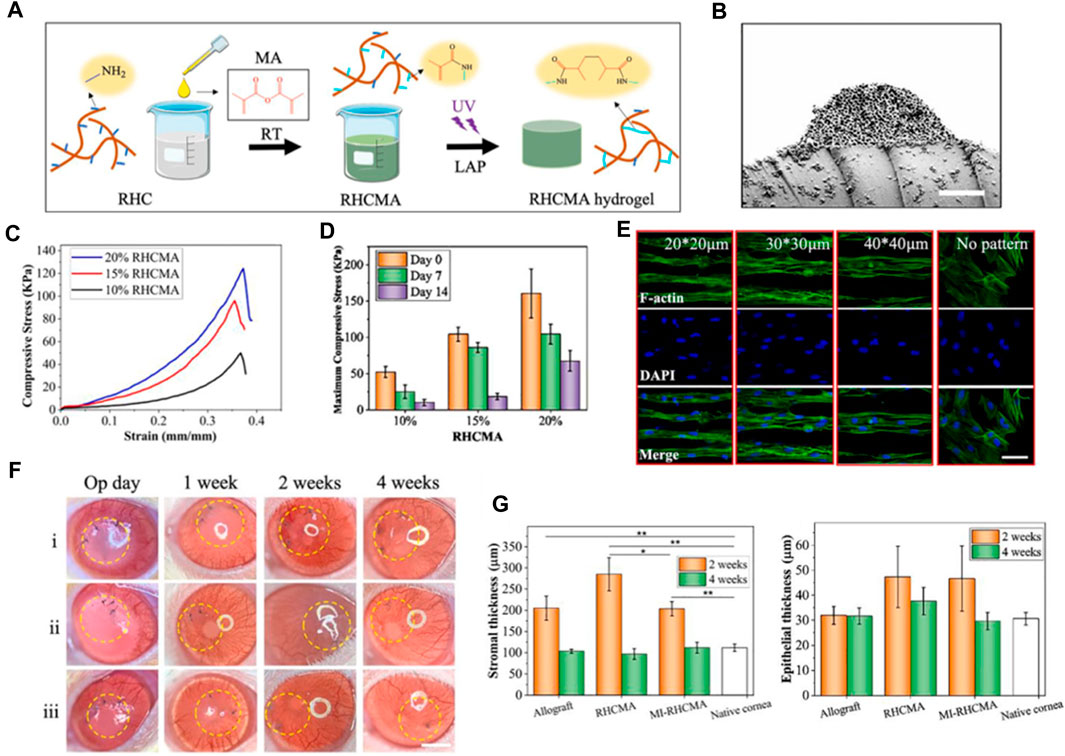
FIGURE 3. (A) Graphic representation of rhcma hydrogel synthesis process. (B) Cross-sectional SEM visuals of the mi-RHCMA hydrogel patch. Scale bar, 5 μm. (C) Compressive strain-stress relationships of various RHCMA hydrogels post 60-min PBS soak. (D) Histograms displaying the peak compressive stress for different RHCMA hydrogels following immersed in PBS. (E) Diagram exhibiting the cytoskeletal and nuclear staining in LSSCs hosted on constructs. Scale bar, 5 μm. (F) Representative photographs illustrating the post-surgical ocular conditions of corneas that treated with i) allograft, ii) RHCMA, and iii) MI-RHCMA hydrogel patches, captured immediately. Scale bar, 1.5 mm. (G) Measurements of the thickness of the stroma (B) and the epithelium (C) were taken at day 14 and day 28 post-operation for corneas that had undergone transplantation with allograft, RHCMA, and MI-RHCMA hydrogel patches. Reproduced with permission from ref Kong et al. (2022).
To summarize, researchers fabricated a novel recombinant human collagen hydrogel designed for corneal tissue restoration. The final product was a hierarchically structured hydrogel, crafted through the amalgamation of RHCMA hydrogel, lithography, and photonic crystal techniques. This material, featuring inverse opal nanopores and aligned microgrooves, alongside ordered topological indications, promoted the aligned growth and differentiation of LSSCs into keratocytes in vitro. Moreover, RHCMA hydrogels with these organized microstructures were found to boost tissue repair processes and foster the regeneration of damaged stromal tissue in vivo. These attributes underscore their promising potential in the domains of tissue repair and stroma regeneration.
Recombinant human collagen (RHC) polypeptide holds a significant edge over natural collagen sources in the realm of tissue engineering applications (Yang et al., 2021b). This is particularly advantageous when compared to animal-derived collagens, as it considerably reduces the risk of immune rejection upon implantation (Parmar et al., 2017). One of the key advantages of RHC is its capacity for precise and customizable biosynthesis. This allows for the engineering of specific peptide sequences, integrin binding sites, growth factors, and cross-linking into the polypeptide chain (Haagdorens et al., 2019; Wang, 2021). Such level of control paves the way for tuning the properties of RHC to achieve optimal performance in specific applications. In addition, the production of RHC yields a highly consistent and reproducible biomaterial, thereby ensuring uniformity in its quality (Wang, 2021). This process also eradicates risks associated with pathogen transmission from animal sources and negates the need for reliance on animal harvesting, thus providing an abundant, sustainable supply of human collagen (Rico-Llanos et al., 2021). The degradation rate of RHC is tunable, and it boasts processing versatility, and overall customizability, which further enhances its suitability as a biomaterial. These properties make RHC an ideal biomaterial for the development of engineered tissues and scaffolds for various applications. These include, but are not limited to, skin grafts, tendon/ligament repair, wound healing, and other regenerative medicine applications.
The potential for employing scaffolds as vehicles for recombinant collagen in the fields of tissue engineering and regenerative medicine is considerable. These scaffolds deliver a three-dimensional construct reminiscent of the natural extracellular matrix, fostering cellular attachment, proliferation, and differentiation. Scaffolds comprising recombinant collagen present numerous benefits compared to conventional scaffolds manufactured from animal-derived collagen. The former can be produced on a large scale, with meticulous regulation of composition and purity (He et al., 2018). Additionally, recombinant collagen scaffolds can be functionalized with elements such as cell-binding motifs, growth factors, and other biologically active molecules to enhance their efficacy.
Nevertheless, significant obstacles remain. Emulating the intricate architecture and diverse protein composition of native ECM continues to be a challenging task. Matching the degradation rate of scaffolds with the pace of cell/tissue growth persistently proves difficult (Jeon et al., 2017). Engineering tissues over 1 mm in thickness necessitates innovative strategies for vascularization. Moreover, understanding how the physicochemical properties of scaffolds impact cell behavior is still lacking. Current research pursuits are focused on deepening our understanding of cell-matrix interactions, designing innovative biomaterials and processing methodologies, and augmenting the functional characteristics of engineered tissues (Huang et al., 2018). In conclusion, while recombinant collagen scaffolds represent a promising avenue for regenerative medicine, additional research is required to enhance scaffold bioactivity, degradation, and integration within host tissues.
4 Utilization of recombinant human collagen in bone tissue repair
Recombinant human collagen has emerged as a significant asset in the field of bone regenerative engineering (Andrews et al., 2019; Fushimi et al., 2020). RhCOL retains the biological attributes of natural collagen while circumventing the issues associated with immunogenicity and pathogen transmission. RhCOL scaffolds facilitate the adhesion, proliferation, and differentiation of osteoblasts in vitro. In vivo studies illustrate enhanced bone regeneration when rhCOL is used in conjunction with bone marrow-derived mesenchymal stem cells (BMSCs) and/or osteogenic growth factors (Chan et al., 2017).
The composition and structure of rhCOL scaffolds can be precisely tailored to mimic the native bone extracellular matrix. This is achieved through manipulation of collagen crosslinking, mineral content, and the incorporation of bioactive motifs, enabling a controlled degradation rate that synchronizes with new bone deposition (Bien et al., 2020). Moreover, rhCOL scaffolds surpass the limitations of traditional bone graft materials by supporting cellular growth and providing precise control over structural and functional properties (Muhonen et al., 2017; Bien et al., 2020). Ongoing research endeavors aim to optimize integration and healing outcomes as rhCOL transitions from laboratory research to clinical implementation for bone engineering applications.
Sandri et al. delves into the creation of a synthetic bone substitute that emulates the biochemical and biophysical cues intrinsic to the native bone extracellular matrix (Ramírez-Rodríguez et al., 2017). The investigative team employed a recombinant collagen-based scaffold, which was enriched with the tri-amino acid sequence arginine-glycine-aspartate (RGD), aiming to bolster the interaction and differentiation of mesenchymal stem cells. The study witnessed promising progress in the generation of superior quality bone grafts, a feat achieved through biomimetic mineralization of synthetic engineering peptides under the influence of magnesium ions. The novelty of this study hinges on the application of a synthetic bone substitute that, across all scales from macro to nano, replicates the biochemical and biophysical cues of the bone extracellular matrix.
Three distinct scaffold compositions were characterized by SEM analysis, non-mineralized (RCP), mineralized (Ap/RCP), and mineralized alongside magnesium (MgAp/RCP) possess a highly porous structure with interconnected pores. The mineralized scaffolds exhibit a more compact structure compared to their non-mineralized counterpart, and the incorporation of magnesium leads to a more uniform and homogeneous structure (Figure 4A). Encapsulated Ca2+ and Rcp display a consistent and prolonged release pattern (Figures 4B, C). The scaffold demonstrates superior cytocompatibility, exhibiting no adverse or toxic effects on cells (Figure 4D). Detailed examination revealed that the MgAp/RCP scaffolds exhibited the most pronounced MSC proliferation within 28 days (Figure 4E). Through the use of qPCR, they analyzed the messenger RNA (mRNA) levels of ALP, RUNX2, OPN, and COL1, aiming to discern the influence that the three types of scaffolds exerted on the expression of osteogenic markers. Scaffolds exert a significant influence on the expression levels of these mRNAs (Figure 4F).
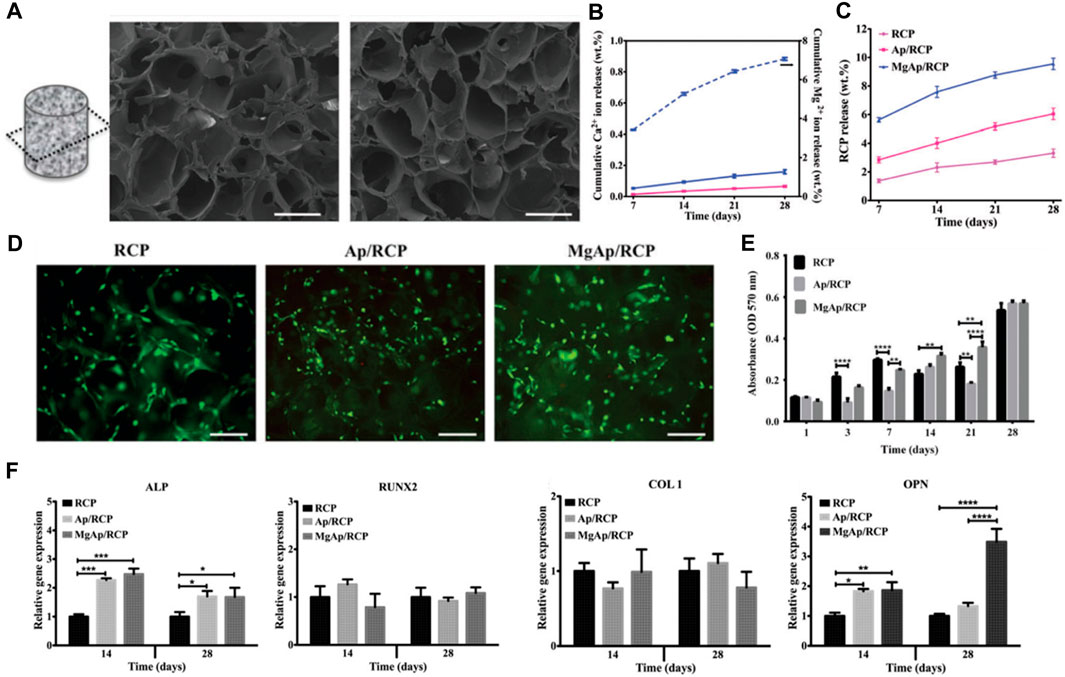
FIGURE 4. (A) SEM analysis of MgAp/RCP scaffolds. Scale bar, 250 mm. Release profiles of (B) Ca2+ and (C) RCP from MgAp/RCP scaffolds. (D) Staining of live and dead cells was performed for the investigation of cytocompatibility. (E) The MTT assay was employed to evaluate cell viability at multiple time points: after 1, 3, 7, 14, 21, and 28 days. (F) mRNA expression investigation of alkaline phosphatase (ALP), collagen I (COL1), osteopontin (OPN) and runtrelated transcription factor 2 (RUNX2). Reproduced with permission from ref Ramírez-Rodríguez et al. (2017).
In 2018, Farrell et al. presented a research investigation focused on developing a novel in situ gelling hydrogel, embedded with recombinant collagen peptide microspheres (Fahmy-Garcia et al., 2018). This unique slow-release system is designed to stimulate ectopic bone formation. The study’s objective was to introduce a promising solution for extensive bone defect repair, employing natural biomaterials which are biodegradable, biocompatible, and can actively interact with the extracellular matrix and cells. The injectable formulation simplifies application and can potentially expedite patient recovery time.
The research process comprised the production of the hydrogel and microspheres, succeeded by in vitro and in vivo examinations to assess their properties and effectiveness. The hydrogel was synthesized using a blend of gelatin, hyaluronic acid, and β-glycerophosphate (Figure 5A), while the microspheres were fashioned using recombinant collagen peptide and poly (lactic-co-glycolic acid) (PLGA). Various techniques, including scanning electron microscopy (Figure 5B), Fourier-transform infrared spectroscopy, and rheological analysis, were employed to characterize the hydrogel and microspheres. Alginate hydrogels containing RCP-MS demonstrated a slower release rate, indicating the synergistic effect of microspheres and hydrogels in controlling the release. During the in vitro experiments, the hydrogel and microspheres’ biocompatibility and osteogenic potential were evaluated. The findings indicated that the hydrogel and microspheres promoted cells proliferation and differentiation into osteoblasts, suggesting their potential for bone tissue engineering applications. In vivo experiments evaluated the hydrogel and microspheres’ efficacy in stimulating ectopic bone formation in a rat model. The results revealed significant enhancement in bone formation in comparison to the control group, as substantiated by micro-computed tomography and histological analysis. The hydrogel and microspheres also facilitated the infiltration of immune cells, including macrophages and M2-like macrophages, which play an essential role in bone regeneration.
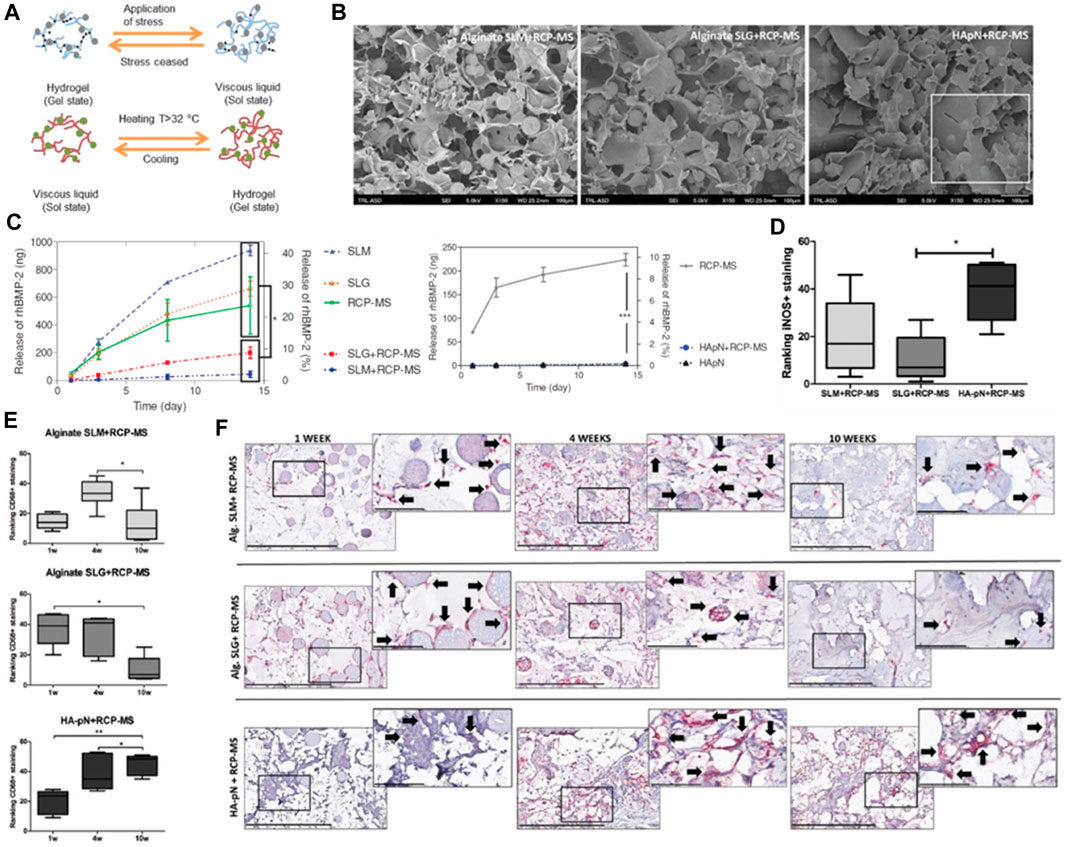
FIGURE 5. (A) The diagram depicts the structural network of a hydrogel infused with microspheres. (B) SEM visuals of the SLM+RCP-MS, SLG+RCP-MS, and HApN+RCP-MS formulations are presented. Scale bar, 100 μm. (C) The dispersion of BMP-2 is contrasted among various precursors. (D) Positive iNOS staining observed in the formulations after a period of 10 weeks. (E) Two impartial reviewers classified the implants based on the occurrence of CD68 positive staining observed in the formulations at the intervals of 1, 4, and 10 weeks. (F) Representative sample from each implant formulation at 1 and 10 weeks, with a scale bar indicating 400 μm. Reproduced with permission from ref Fahmy-Garcia et al. (2018).
In summary, the research demonstrated the potential of the novel in situ gelling hydrogel loaded with recombinant collagen peptide microspheres as a slow-release system to induce ectopic bone formation. Both the hydrogel and microspheres displayed excellent biocompatibility and osteogenic potential in vitro and significantly augmented bone formation in vivo. The findings indicate that this injectable formulation could serve as a promising solution for extensive bone defect repair by leveraging natural, biodegradable, and biocompatible biomaterials that interact with the extracellular matrix and cells.
5 Application of recombinant human collagen in 3D bioprinting
Recombinant human collagen has attracted growing interest in 3D bioprinting due to its biocompatibility, low immunogenicity, and customizable biochemical and mechanical properties (Lee et al., 2019; Osidak et al., 2020; Muthusamy et al., 2021). Studies have engineered recombinant human collagen with tailored supramolecular assemblies, crosslinking densities, and matrix stiffnesses to resemble native extracellular matrices (Włodarczyk-Biegun and Del Campo, 2017; Hong et al., 2018). This permits precise control over microenvironments for directing cell fate processes (Tytgat et al., 2020). Moreover, recombinant collagen allows incorporation of cell-adhesive peptides, growth factors, and cytokines to modulate cell behaviors. Currently, recombinant human collagen-based bioinks have been utilized to bioprint tissue constructs such as skin, cartilage, bone, blood vessels, and liver (Zhang et al., 2021). Looking ahead, recombinant human collagen bioinks hold great promise for fabricating complex heterogeneous tissues with biomimetic architectures, compositions, and functions (Gungor-Ozkerim et al., 2018; Isaacson et al., 2018; Gudapati et al., 2020). However, challenges remain in scalable recombinant collagen production and developing universal crosslinking strategies to enhance print fidelity (Martyniak et al., 2022). Further interdisciplinary research on optimizing recombinant human collagen designs, crosslinking mechanisms, and printing processes is critical to enable wide clinical translations of 3D bioprinted tissues and organs (Stepanovska et al., 2021).
In 2022, Jin et al. presents the formulation of photo-responsive bioinks based on chitosan and recombinant human collagen for 3D bioprinting (Yang et al., 2022a). The authors delve into the merits of employing these materials, including their biocompatibility, biodegradability, and their proficiency to foster cell proliferation and differentiation. They underscore the cruciality of managing shear stress during the printing operation to preserve the integrity of stem cells (Figure 6).
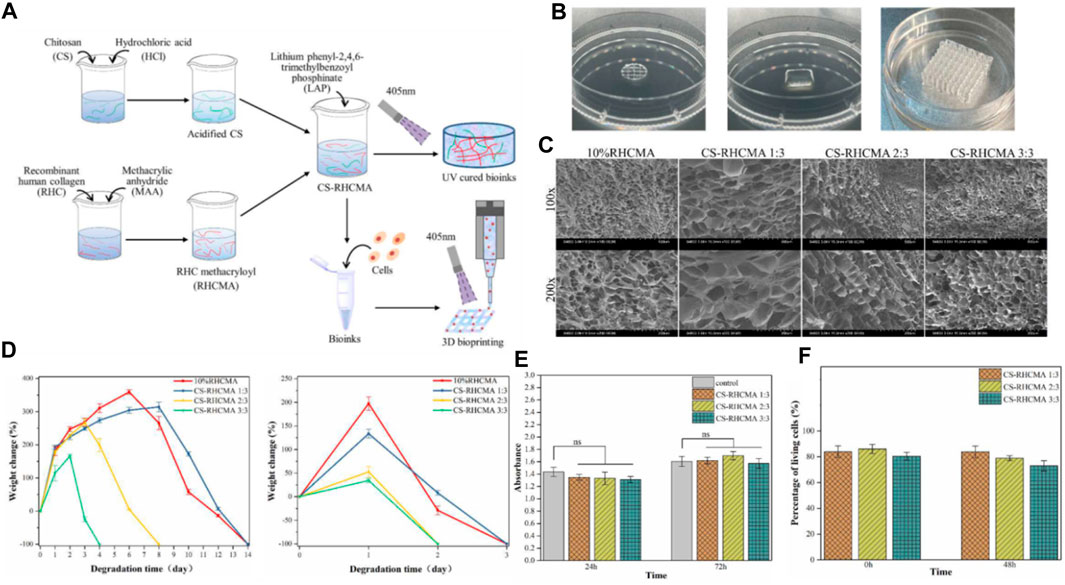
FIGURE 6. (A) Diagrammatic representation of the CS-RHCMA bioinks preparation process. (B) Different structures created by 3D printed constructs. (C) SEM images of the internal morphology of freeze-dried RHCMA and CS-RHCMA samples. Scale bar, 200 µm. (D) Degradation of RHCMA and CS-RHCMA samples when incubated in PBS solution (pH 7.2) and lysozyme solution (pH 6.5), respectively. (E) Assessment of HUVECs viability cultured in sample extracts at timeframes of 24 and 72 h. None significant (ns) indicates p > 0.05. (F) Evaluation of in vitro biocompatibility for HUVECs-laden CS-RHCMA bioinks post-printing. Reproduced with permission from ref Yang et al. (2022a).
Type-III recombinant human collagen methacryloyl/acidified chitosan (CS-RHCMA) bioinks were synthesized by incorporating acidified chitosan into a RHCMA solution. The RHCMA was derived by altering recombinant human collagen with methacrylic anhydride. The CS-RHCMA composites were created by amalgamating the acidified chitosan with the RHCMA solution in varying proportions, facilitating the adjustment of the bioinks’ mechanical resilience and internal pore dimensions. The integration of chitosan into RHCMA enhanced the printability of the bioinks, yielding well-structured 3D constructs via extrusion-based 3D printing (Figure 6A). Figure 6B shows different structures created by 3D printed constructs using the CS-RHCMA bioinks. The authors demonstrate the versatility of the bioinks by creating various structures, including a honeycomb structure, a spiral structure, and a grid structure. The printed HUVECs are well sustained within the lattices prepared from the CS-RHCMA samples, with nearly 80% of the cells being alive after the extrusion-based printing. This suggests that the CS-RHCMA bioinks are suitable for 3D bioprinting and can support the growth and viability of HUVECs. SEM images present the internal structure of freeze-dried RHCMA and CS-RHCMA samples. Both samples show a uniformly distributed, interconnected pore structure (Figure 6C). Introducing chitosan to RHCMA increased pore size: average pore size in CS-RHCMA 1:3 is 128 μm, larger than 66 μm in 10% RHCMA. Further CS content increases lead to smaller pores and denser pore walls, with CS-RHCMA 3:3 having the smallest size of 58 μm. These findings indicate that adding chitosan to RHCMA allows control over the bioinks’ internal structure, influencing the mechanical properties and cell behavior in printed constructs. Figure 6D depict the degradation of RHCMA and CS-RHCMA in PBS (pH 7.2) and lysozyme solution (pH 6.5), measured by weight loss over time. Lysozyme-incubated samples degraded completely in 4 days, while those in PBS took 14 days. RHC and CS-RHCMA 1:3 degraded slower but increasing CS ratio sped up degradation. This is likely due to changes in mechanical properties from added acidified CS disrupting gelation networks. Thus, higher strength UV-cured bioinks resist fast breakdown, and degradation rate can be adjusted by varying bioink composition, influencing the stability and longevity of printed constructs. Figure 6E demonstrates that all the UV-cured CS-RHCMA bioinks were cytocompatible and suitable for 3D bioprinting in vitro.
The findings affirm the cytocompatibility of UV-cured CS-RHCMA bioinks, rendering them suitable for in vitro 3D bioprinting. The viability of cells within the bioprinted lattice approximated 80%, underscoring the bioinks’ ability to foster HUVECs growth and survival (Figure 6F). These insights are pivotal in advancing bioinks for 3D bioprinted vascularized tissues and organ constructs.
The novelty of this research rests on the generation of a photo-responsive bioink capable of fabricating intricate 3D constructs with superior resolution and cell viability. The authors illustrate the promise of this bioink for tissue engineering applications, encompassing the production of skin and cartilage tissues.
Nonetheless, this work is not without its challenges. These include the necessity for further refinement of the printing protocol and the imperative to scale up manufacturing for clinical use. Moreover, the authors acknowledge that additional research is requisite to fully comprehend the enduring impacts of employing these materials in a living organism.
Recombinant human collagen has garnered interest in 3D bioprinting due to its biocompatibility, low immunogenicity, and adaptable biochemical and mechanical traits (Elalouf, 2021). Research has tailored RHC to mimic native extracellular matrices with custom supramolecular assemblies, crosslinking densities, and matrix firmness, allowing precise control over cellular microenvironments for directing cell fate (Cui et al., 2017). Furthermore, RHC allows the integration of cell-adhesive peptides, growth factors, and cytokines to influence cell behaviors (Dai et al., 2021). At present, RHC-based bioinks are used to bioprint various tissue constructs, including skin, cartilage, bone, blood vessels, and liver (Tang et al., 2021). Moving forward, RHC bioinks possess significant potential for crafting complex, heterogeneous tissues with biomimetic structures, compositions, and functionalities (Zhang et al., 2017; Matai et al., 2020). Nevertheless, hurdles persist in scalable RHC production and devising universal crosslinking strategies for improved print fidelity. Continued interdisciplinary research on refining RHC designs, crosslinking mechanisms, and printing methodologies is crucial for broad clinical translation of 3D bioprinted tissues and organs.
6 Conclusion and discussion
The production of recombinant human collagen (RHC) is a complex biotechnological process that encompasses the utilization of specific host cells modified to express human collagen genes. The process begins with the isolation of the relevant human genes encoding collagen, which are then cloned into vectors–DNA molecules capable of carrying foreign DNA into a host cell. These vectors are subsequently introduced into host cells such as Escherichia coli, yeast, or mammalian cells, which have been chosen based on their ability to produce collagen in a form that retains its native structure and function (He et al., 2018; Sheehy et al., 2018).
Following transformation, the host cells are cultured in a controlled environment that is optimized for the expression of the collagen gene. The production involves the scaling up of cell cultures in bioreactors, where conditions such as temperature, pH, and nutrient supply are meticulously managed to maximize yield and product quality (Włodarczyk-Biegun and Del Campo, 2017; Lee et al., 2019). Post-translational modifications crucial for collagen stability and function, such as hydroxylation and glycosylation, are carefully orchestrated within the production system.
The benefits of using RHC instead of animal-derived collagen are multifaceted (Radke et al., 2018; Lagali, 2020; Elalouf, 2021): (1) safety: RHC reduces the potential for zoonotic disease transmission and immunogenic reactions as it is produced in a controlled environment without sourcing from animal tissues; (2) Consistency: the production of RHC can be tightly regulated to ensure batch-to-batch consistency, which is a significant challenge with animal-derived collagen due to natural biological variability; (3) Customization: RHC can be modified at the genetic level to include specific amino acid sequences or to introduce particular post-translational modifications, which is not feasible with animal-derived collagen. This allows for the creation of collagen with precise characteristics required for specific applications. (4) Ethical Considerations: RHC production avoids the ethical concerns associated with the use of animal products.
It is important to highlight that customizing animal-derived collagen is inherently challenging. The extraction process from animal tissues can lead to batch variability, and the complexity of the native collagen structure makes it difficult to modify post-translationally. This results in a product that may not be consistently reliable for precise biomedical applications, where uniformity in structure and function is paramount (Osidak et al., 2020).
RHC has emerged as a biomaterial with extraordinary versatility and promise in the realms of tissue engineering and regenerative medicine, presenting a multitude of benefits over traditional animal-derived collagens. Its uniform composition, markedly reduced immunogenicity, and the amenability to molecular engineering for bespoke applications highlight its broad potential in diverse biomedical applications (Nocera et al., 2018). Research has underscored the adaptability of RHC in various formulations such as hydrogels, scaffolds, and lyophilized substances, which have been successfully applied in healing wounds, regenerating skin, and reconstructing osseous and cartilaginous tissues.
The ability to enhance these RHC-based materials with growth factors, cellular elements, or nanoparticles opens up avenues for precision customization, aligning material properties with the nuanced demands of specific therapeutic contexts. The efficacy of RHC is evident in its application to skin grafts, weight-bearing tissue repair, and the engineering of vascular grafts—areas where there is substantial documentation of its success, particularly in preclinical animal studies (Curtin et al., 2018; Elalouf, 2021; Yang et al., 2022a).
To expand upon this, the future of RHC research is poised to delve into next-level innovations that may redefine therapeutic approaches. For instance, the integration of RHC with cutting-edge bio-fabrication technologies, such as 3D bioprinting, has the potential to construct tissues and organs with unprecedented complexity and functionality (Liverani et al., 2017; Rico-Llanos et al., 2021). This would not only revolutionize how we approach complex tissue reconstruction but also hold implications for personalized medicine, where RHC-based tissues are tailored to individual patient’s biological profiles.
Moreover, there exists a burgeoning interest in exploring the synergistic combinations of RHC with synthetic polymers, which may yield composite materials with enhanced mechanical properties and biological functionalities. Such composites could offer new solutions for the regeneration of tissues that require a high degree of biomechanical resilience, such as in the case of intervertebral disc repair or the reconstruction of load-bearing joints (He et al., 2018; Rico-Llanos et al., 2021; Binlateh et al., 2022).
Another prospective area of RHC application lies in the realm of controlled drug delivery systems (Lagali, 2020; Yang et al., 2022b). By embedding therapeutic agents within RHC matrices, it may be possible to achieve localized, sustained release of drugs at injury sites, thereby enhancing the healing process while minimizing systemic side effects.
The ongoing research and future explorations are expected to not only address the current limitations surrounding RHC production and application but also to unlock novel therapeutic paradigms. As we stand on the cusp of these scientific advancements, RHC research is truly at an inflection point, with the anticipation that future studies will bring forth groundbreaking applications that will cement RHC’s status as an invaluable asset in biomedical engineering and beyond. (Liverani et al., 2017).
RHC is an area of intense research interest due to its potential applications in biomedicine, particularly in tissue engineering and regenerative medicine (Parmar et al., 2017; Yang et al., 2021b; Gajbhiye and Wairkar, 2022). The future direction of RHC-related research is shaped by the need for safer, more effective, and customizable biomaterials. Here are several promising avenues for future research:
6.1 Enhanced biomimicry
Future research will likely focus on improving the biochemical and biomechanical properties of RHC to more closely mimic the native characteristics of human collagen. This includes fine-tuning the amino acid composition, crosslinking patterns, and molecular alignment to replicate the mechanical strength and biological signaling present in the human body.
6.2 Genetic engineering advances
Advancements in genetic engineering techniques can be applied to modify the genes used to produce RHC, leading to collagens with specific properties or functions that are difficult to obtain from natural collagens. This can enable the custom design of collagen molecules for specific medical applications.
6.3 3D Bioprinting integration
The integration of RHC with advanced 3D bioprinting techniques is an exciting frontier. Research will likely explore the development of specialized bioinks that can be used to print complex, multicellular tissues and organs with high precision.
6.4 Smart biomaterials
The development of “smart” RHC-based materials that can respond to physiological stimuli, such as changes in pH or temperature, could revolutionize drug delivery systems and dynamic tissue scaffolds that adapt to the healing process.
6.5 Personalized medicine applications
With the advent of personalized medicine, RHC could be tailored to individual patients based on their genetic makeup, potentially improving the outcomes of treatments and reducing the risk of adverse reactions.
By addressing these future directions, RHC research can contribute to creating more effective therapeutic strategies and innovative solutions for complex medical challenges.
However, challenges persist. To fully realize the potential of RHC, high-yield, cost-effective production systems need to be established (Fu et al., 2019; Groetsch et al., 2019). Systems based on mammalian cells are often associated with low yields and high costs, while bacterial and yeast systems, although more cost-effective, may not achieve the necessary post-translational modifications. Innovations in genetic manipulation and enzyme supplementation may offer potential solutions to these problems (Radke et al., 2018). The use of RHC in 3D bioprinting also invites further exploration. As technologies evolve, RHC’s potential to be used in the fabrication of complex, heterogeneous tissues with biomimetic architectures, compositions, and functionalities could be transformative.
In summary, the research summarized in this review underscores the significant potential of RHC in tissue engineering and regenerative medicine. Despite remaining challenges, the progress made so far in the development and application of RHC is encouraging, and the future of RHC looks promising. As the field continues to advance, RHC is likely to play an increasingly important role in the development of improved biomaterials, therapies, and patient outcomes.
Author contributions
LC: Conceptualization, Supervision, Writing–original draft, Writing–review and editing. ZZ: Writing–review and editing. DY: Writing–review and editing. MY: Writing–review and editing. JM: Writing–review and editing.
Funding
The author(s) declare that no financial support was received for the research, authorship, and/or publication of this article.
Conflict of interest
The authors declare that the research was conducted in the absence of any commercial or financial relationships that could be construed as a potential conflict of interest.
Publisher’s note
All claims expressed in this article are solely those of the authors and do not necessarily represent those of their affiliated organizations, or those of the publisher, the editors and the reviewers. Any product that may be evaluated in this article, or claim that may be made by its manufacturer, is not guaranteed or endorsed by the publisher.
References
Abbas, Y., Brunel, L. G., Hollinshead, M. S., Fernando, R. C., Gardner, L., Duncan, I., et al. (2020). Generation of a three-dimensional collagen scaffold-based model of the human endometrium. Interface Focus 10 (2), 20190079. doi:10.1098/rsfs.2019.0079
Addi, C., Murschel, F., and De Crescenzo, G. (2017). Design and use of chimeric proteins containing a collagen-binding domain for wound healing and bone regeneration. Tissue Eng Part B Rev. 23, no. 2 : 163–182. doi:10.1089/ten.teb.2016.0280
Ahmad, T., McGrath, S., Sirafim, C., Amaral, R. J. F. C. do, Soong, S.-L., Sitram, R., et al. (2021). Development of wound healing scaffolds with precisely-triggered sequential release of therapeutic nanoparticles. Biomater. Sci. 9 (12), 4278–4288. doi:10.1039/d0bm01277g
Amyoony, J., Gorman, M., Dabas, T., Moss, R., and McSweeney, M. B. (2023). Consumer perception of collagen from different sources: an investigation using hedonic scale and check all that apply.
Andrews, S., Cheng, A., Stevens, H., Logun, M. T., Webb, R., Jordan, E., et al. (2019). Chondroitin sulfate glycosaminoglycan scaffolds for cell and recombinant protein-based bone regeneration. Stem Cells Transl. Med. 8 (6), 575–585. doi:10.1002/sctm.18-0141
Arakawa, C., Ng, R., Tan, S., Kim, S., Wu, B., and Lee, M. (2017). Photopolymerizable chitosan-collagen hydrogels for bone tissue engineering: photopolymerizable chitosan-collagen hydrogels. Photopolymerizable Chitosan–Collagen Hydrogels Bone Tissue Eng. 11 (1), 164–174. doi:10.1002/term.1896
Avila Rodríguez, M. I., Rodríguez Barroso, L. G., and Sánchez, M. L. (2018). Collagen: a review on its sources and potential cosmetic applications. J. Cosmet. Dermatol 17 (1), 20–26. doi:10.1111/jocd.12450
Bien, N. D., Miura, K.-ichiro, Sumita, Y., Nakatani, Y., Shido, R., Kajii, F., et al. (2020). Bone regeneration by low-dose recombinant human bone morphogenetic protein-2 carried on octacalcium phosphate collagen composite. J. Hard Tissue Biol. 29 (2), 123–130. doi:10.2485/jhtb.29.123
Binlateh, T., Thammanichanon, P., Rittipakorn, P., Thinsathid, N., and Jitprasertwong, P. (2022). Collagen-based biomaterials in periodontal regeneration: current applications and future perspectives of plant-based collagen. Biomimetics 7 (2), 34. doi:10.3390/biomimetics7020034
Capella-Monsonís, H., Coentro, J. Q., Graceffa, V., Wu, Z., and Dimitrios, I. (2018). An experimental toolbox for characterization of mammalian collagen type I in biological specimens. Nat. Protoc. 13 (3), 507–529. doi:10.1038/nprot.2017.117
Carrabba, M., and Madeddu, P. (2018). Current strategies for the manufacture of small size tissue engineering vascular grafts. Front. Bioeng. Biotechnol. 6, 41. doi:10.3389/fbioe.2018.00041
Catanzano, O., Quaglia, F., and Joshua, S. (2021). Wound dressings as growth factor delivery platforms for chronic wound healing. Expert Opin. Drug Deliv. Boateng 18 (6), 737–759. doi:10.1080/17425247.2021.1867096
Chan, Su J., Love, C., Spector, M., Cool, S. M., Nurcombe, V., and Lo, E. H. (2017). Endogenous regeneration: engineering growth factors for stroke. Neurochem. Int. 107, 57–65. doi:10.1016/j.neuint.2017.03.024
Chen, Lu, Guttieres, D., Koenigsberg, A., Barone, P. W., Sinskey, A. J., and Stacy, L. (2022). Large-scale cultured meat production: trends, challenges and promising biomanufacturing technologies. Biomaterials 280, 121274. doi:10.1016/j.biomaterials.2021.121274
Chen, Z., Fan, D., and Shang, L. (2020). Exploring the potential of the recombinant human collagens for biomedical and clinical applications: a short review. Biomed. Mater. 16 (1), 012001. doi:10.1088/1748-605x/aba6fa
Copes, F., Pien, N., Van Vlierberghe, S., Boccafoschi, F., and Mantovani, D. (2019). Collagen-based tissue engineering strategies for vascular medicine. Front. Bioeng. Biotechnol. 7, 166. doi:10.3389/fbioe.2019.00166
Coppola, D., Oliviero, M., Andrea Vitale, G., Lauritano, C., D’Ambra, I., Iannace, S., et al. (2020). Marine collagen from alternative and sustainable sources: extraction, processing and applications. Mar. Drugs 18 (4), 214. doi:10.3390/md18040214
Cui, H., Nowicki, M., Fisher, J. P., and Grace Zhang, L. (2017). 3d bioprinting for organ regeneration. Adv. Healthc. Mater. 6 (1), 1601118. doi:10.1002/adhm.201601118
Curtin, C., Nolan, J. C., Conlon, R., Deneweth, L., Gallagher, C., Tan, Y. J., et al. (2018). A physiologically relevant 3d collagen-based scaffold–neuroblastoma cell system exhibits chemosensitivity similar to orthotopic xenograft models. Acta biomater. 70, 84–97. doi:10.1016/j.actbio.2018.02.004
Dai, M., Belaïdi, J.-P., Fleury, G., Garanger, E., Rielland, M., Schultze, X., et al. (2021). Elastin-like polypeptide-based bioink: a promising alternative for 3d bioprinting. Biomacromolecules 22 (12), 4956–4966. doi:10.1021/acs.biomac.1c00861
Davison-Kotler, E., Marshall, W. S., García-Gareta, E., and García-Gareta, E. (2019). Sources of collagen for biomaterials in skin wound healing. Bioengineering 6 (3), 56. doi:10.3390/bioengineering6030056
Deng, A., Yang, Y., Du, S., Yang, X., Pang, S., Wang, X., et al. (2021). Preparation of a recombinant collagen-peptide (Rhc)-Conjugated chitosan thermosensitive hydrogel for wound healing. Mater Sci Eng C Mater Biol Appl. 119, 111555, doi:10.1016/j.msec.2020.111555
Di Martino, J. S., Nobre, A. R, Mondal, C., Taha, I., Farias, E. F., Fertig, E. J., et al. (2022). A tumor-derived type iii collagen-rich ecm niche regulates tumor cell dormancy. Nat. cancer 3 (1), 90–107. doi:10.1038/s43018-021-00291-9
Doan, N.-D., DiChiara, A. S., M Del Rosario, A., and Schiavoni, R. P. (2019) Matthew D collagen: methods shoulders, and protocols mass spectrometry-based proteomics to define intracellular collagen interactomes. Methods Mol. Biol. 95–114. doi:10.1007/978-1-4939-9095-5_7
Elalouf, A. (2021). Immune response against the biomaterials used in 3D bioprinting of organs. Transpl. Immunol. 69, 101446. doi:10.1016/j.trim.2021.101446
Felician, F. F., Xia, C., Qi, W., and Xu, H. (2018). Collagen from marine biological sources and medical applications. Chem Biodivers 15, 5, e1700557, doi:10.1002/cbdv.201700557
Ferraro, V., Gaillard-Martinie, B., Sayd, T., Chambon, C., Anton, M., and Santé-Lhoutellier, V. (2017). Collagen type I from bovine bone. Effect of animal age, bone anatomy and drying methodology on extraction yield, self-assembly, thermal behaviour and electrokinetic potential. Int. J. Biol. Macromol. 97, 55–66. doi:10.1016/j.ijbiomac.2016.12.068
Fu, Yu, Therkildsen, M., Aluko, R. E., and Lametsch, R. (2019). Exploration of collagen recovered from animal by-products as a precursor of bioactive peptides: successes and challenges. Crit. Rev. Food Sci. Nutr. 59 (13), 2011–2027. doi:10.1080/10408398.2018.1436038
Fushimi, H., Hiratsuka, T., Ai, O., Ono, Y., Ogura, I., and Nishimura, I. (2020). Recombinant collagen polypeptide as a versatile bone graft biomaterial. Commun. Mat. 1 (1), 87. doi:10.1038/s43246-020-00089-9
Gajbhiye, S., and Wairkar, S. (2022). Collagen fabricated delivery systems for wound healing: a new roadmap. Biomater. Adv. 142, 213152. doi:10.1016/j.bioadv.2022.213152
Gauza-Włodarczyk, M., Kubisz, L., and Dariusz, W. (2017a), Amino acid composition in determination of collagen origin and assessment of physical factors effects. Int J Biol Macromol 104, 987–991. doi:10.1016/j.ijbiomac.2017.07.013
Gauza-Włodarczyk, M., Kubisz, L., Mielcarek, S., and Włodarczyk, D. (2017b). Comparison of thermal properties of fish collagen and bovine collagen in the temperature range 298–670 K. Mater Sci Eng C Mater Biol Appl. 80, 468–471. doi:10.1016/j.msec.2017.06.012
Ghomi, E. R., Nourbakhsh, N., and Kenari, M. A. (2021). Mina zare, and seeram journal of biomedical materials research Part B: applied biomaterials ramakrishna. Collagen-Based Biomaterials Biomed. Appl. 109 (12), 1986–1999. doi:10.1002/jbm.b.34881
Groetsch, A., Gourrier, A., Schwiedrzik, J., Sztucki, M., Beck, R. J., Shephard, J. D., et al. (2019). Compressive behaviour of uniaxially aligned individual mineralised collagen fibres at the micro-and nanoscale. Acta biomater. 89, 313–329. doi:10.1016/j.actbio.2019.02.053
Gudapati, H., Parisi, D., Colby, R. H., and Matter Ozbolat, I. T. S. (2020). Rheological investigation of collagen, fibrinogen, and thrombin solutions for drop-on-demand 3d bioprinting. Soft Matter 16 (46), 10506–10517. doi:10.1039/d0sm01455a
Gungor-Ozkerim, P. S., Inci, I., Zhang, Yu S., Ali, K., and Remzi, M. (2018). Bioinks for 3D bioprinting: an overview. Biomater. Sci. 6 (5), 915–946. doi:10.1039/c7bm00765e
Haagdorens, M., Cėpla, V., Melsbach, E., Koivusalo, L., Skottman, H., Griffith, M., et al. (2019). Vitro cultivation of limbal epithelial stem cells on surface-modified crosslinked collagen scaffolds. Stem Cells Int. 2019, 7867613. doi:10.1155/2019/7867613
Han, G., and Roger, C. (2017). Chronic wound healing: a review of current management and treatments. Adv. Ther. 34, 599–610. doi:10.1007/s12325-017-0478-y
Harris, R. B., Fonseca, F. L. A., Sharp, M. H., and Charlie, R. (2022). Functional characterization of undenatured type ii collagen supplements: are they interchangeable? J. Diet. Suppl. Ottinger 19 (6), 717–732. doi:10.1080/19390211.2021.1931621
He, B., Zhao, J., Ou, Y., and Jiang, D. (2018). Biofunctionalized peptide nanofiber-based composite scaffolds for bone regeneration. Mater Sci Eng C Mater Biol. 90, 728–738. doi:10.1016/j.msec.2018.04.063
Hong, N., Yang, G.-H., Lee, J., and Kim, G. (2018). 3d bioprinting and its in vivo applications. J. Biomed. Mat. Res. 106 (1), 444–459. doi:10.1002/jbm.b.33826
Hu, C., Liu, W., Long, L., Wang, Z., Yuan, Y., Zhang, W., et al. (2021). Microenvironment-responsive multifunctional hydrogels with spatiotemporal sequential release of tailored recombinant human collagen type iii for the rapid repair of infected chronic diabetic wounds. J. Mater. Chem. B Lu 9 (47), 9684–9699. doi:10.1039/d1tb02170b
Huang, B., Wu, Z., Ding, S., Yuan, Y., and Changsheng, L. (2018). Localization and promotion of recombinant human bone morphogenetic protein-2 bioactivity on extracellular matrix mimetic chondroitin sulfate-functionalized calcium phosphate cement scaffolds. Acta Biomater. 71, 184–199. doi:10.1016/j.actbio.2018.01.004
Irawan, V., Sung, T.-C., Higuchi, A., and Ikoma, T. (2018). Collagen scaffolds in cartilage tissue engineering and relevant approaches for future development. Tissue Eng. Regen. Med. 15, 673–697. doi:10.1007/s13770-018-0135-9
Isaacson, A., Stephen, S., and Che, J. (2018). 3D bioprinting of a corneal stroma equivalent. Exp. Eye Res. 173, 188–193. doi:10.1016/j.exer.2018.05.010
Jafari, H., Lista, A., Mafosso Siekapen, M., Ghaffari-Bohlouli, P., Nie, L., Alimoradi, H., et al. (2020). Fish collagen: extraction, characterization, and applications for biomaterials engineering. Polym. (Basel). 12 (10), 2230. doi:10.3390/polym12102230
Jeon, E. Y., Choi, B. H., Jung, D., Hwang, B. H., and Cha, H. J. (2017), Natural healing-inspired collagen-targeting surgical protein glue for accelerated scarless skin regeneration, Biomaterials 134, 154–165. doi:10.1016/j.biomaterials.2017.04.041
Kathawala, M. H., Ng, W. L., Liu, D., Naing, M. W., Yeong, W. Y., Spiller, K. L., et al. (2019). Healing of chronic wounds: an update of recent developments and future possibilities. Tissue Eng Part B Rev. 25, 5, 429–444. doi:10.1089/ten.teb.2019.0019
Kim, C.-H., Ju, M.-Ha, and Kim, B. J. (2017). Comparison of recombinant human bone morphogenetic protein-2-infused absorbable collagen sponge, recombinant human bone morphogenetic protein-2-coated tricalcium phosphate, and platelet-rich fibrin-mixed tricalcium phosphate for sinus augmentation in rabbits. J. Dent. Sci. 12 (3), 205–212. doi:10.1016/j.jds.2017.01.003
Knüppel, L., Ishikawa, Y., Aichler, M., Heinzelmann, K., Hatz, R., Behr, J., et al. (2017). A novel antifibrotic mechanism of nintedanib and pirfenidone. Inhibition of collagen fibril assembly. Am. J. Respir. Cell Mol. Biol. 57 (1), 77–90. doi:10.1165/rcmb.2016-0217oc
Koehler, J., Brandl, F. P., and Goepferich, A. M. (2018). Hydrogel wound dressings for bioactive treatment of acute and chronic wounds. Eur. Polym. J. 100, 1–11. doi:10.1016/j.eurpolymj.2017.12.046
Kong, B., Sun, L., Liu, R., Chen, Y., Shang, Y., Tan, H., et al. (2022). Recombinant human collagen hydrogels with hierarchically ordered microstructures for corneal stroma regeneration. Chem. Eng. J. 428, 131012. doi:10.1016/j.cej.2021.131012
Koons, G. L., Diba, M., and Antonios, G. (2020). Materials design for bone-tissue engineering. Nat. Rev. Mater. Mikos 5 (8), 584–603. doi:10.1038/s41578-020-0204-2
Kuivaniemi, H., and Tromp, G. (2019). Type iii collagen (Col3a1): gene and protein structure, tissue distribution, and associated diseases. Gene 707, 151–171. doi:10.1016/j.gene.2019.05.003
Lagali, N. (2020). Corneal stromal regeneration: current status and future therapeutic potential. Curr. Eye Res. 45 (3), 278–290. doi:10.1080/02713683.2019.1663874
Las Heras, K., Igartua, M., Santos-Vizcaino, E., and Hernandez, R. M. (2020). Chronic wounds: current status, available strategies and emerging therapeutic solutions. J. Control. Release 328, 532–550. doi:10.1016/j.jconrel.2020.09.039
Law, J. X., Liau, L. L., Saim, A., Yang, Y., and Idrus, R. (2017). Electrospun collagen nanofibers and their applications in skin tissue engineering. Electrospun Collagen Nanofibers Their Appl. Skin Tissue Eng. 14, 699–718. doi:10.1007/s13770-017-0075-9
Lee, ARHA, Hudson, A. R., Shiwarski, D. J., Tashman, J. W., Hinton, T. J., Yerneni, S., et al. (2019). 3d bioprinting of collagen to rebuild components of the human heart. Science 365, 6452, 482–487. doi:10.1126/science.aav9051
Li, J., Zhang, Y.-J., Lv, Z.-Y., Liu, K., Meng, C.-X., Zou, Bo, et al. (2020). The observed difference of macrophage phenotype on different surface roughness of mineralized collagen. Regen. Biomater. 7 (2), 203–211. doi:10.1093/rb/rbz053
Lim, Y.-S., Ye-Jin, Ok, Hwang, S.-Y., Kwak, J.-Y., and Yoon, S. (2019). Marine collagen as a promising biomaterial for biomedical applications. Mar. drugs 17 (8), 467. doi:10.3390/md17080467
Lin, K., Zhang, D., Helena Macedo, M., Cui, W., Bruno, S., and Guofang, S. (2019). Advanced collagen-based biomaterials for regenerative biomedicine. Adv. Funct. Mater. 29 (3), 1804943. doi:10.1002/adfm.201804943
Liverani, C., Mercatali, L., Cristofolini, L., Giordano, E., Minardi, S., Della Porta, G., et al. (2017). Investigating the mechanobiology of cancer cell–ECM interaction through collagen-based 3D scaffolds. Cell. Mol. Bioeng. 10, 223–234. doi:10.1007/s12195-017-0483-x
Ma, L., Liang, X., Yu, S., and Zhou, J. (2022). Expression, characterization, and application potentiality evaluation of recombinant human-like collagen in pichia pastoris. Bioresour. Bioprocess. 9 (1), 119–215. doi:10.1186/s40643-022-00606-3
Magro, M. G., Carlos Kuga, M., Adad Ricci, W., Cristina Keine, K., Rodrigues Tonetto, M., Linares Lima, S., et al. (2017). Endodontic management of open apex teeth using lyophilized collagen sponge and mta cement: report of Two cases. Iran. Endod. J. 12 (2), 248–252. doi:10.22037/iej.2017.48
Martyniak, K., Lokshina, A., Cruz, M. A., Karimzadeh, M., Kemp, R., and Thomas, J. (2022). Biomaterial composition and stiffness as decisive properties of 3D bioprinted constructs for type II collagen stimulation. Acta Biomater. 152, 221–234. doi:10.1016/j.actbio.2022.08.058
Matai, I., Kaur, G., Amir, S., McClinton, A., and Laurencin, C. T. B. (2020). Progress in 3d bioprinting technology for tissue/organ regenerative engineering. Biomaterials 226: 119536. doi:10.1016/j.biomaterials.2019.119536
Mathew-Steiner, S. S., Roy, S., and Chandan, K. S. (2021). Collagen in wound healing. Bioeng. (Basel). 8 (5), 63. doi:10.3390/bioengineering8050063
McPhail, M. J., Janus, J. R., and Lott, D. G. (2020). Advances in regenerative medicine for otolaryngology/head and neck surgery. BMJ 29, 369. doi:10.1136/bmj.m718
Meng, D., Tanaka, H., Kobayashi, T., Hatayama, H., Zhang, Xi, Ura, K., et al. (2019). The effect of alkaline pretreatment on the biochemical characteristics and fibril-forming abilities of types I and II collagen extracted from bester sturgeon by-products. Int. J. Biol. Macromol. 131, 572–580. doi:10.1016/j.ijbiomac.2019.03.091
Meyer, M. (2019), Processing of collagen based biomaterials and the resulting materials properties. Biomed Eng Online 18, 1: 1–74. doi:10.1186/s12938-019-0647-0
Mi, Yu, Gao, Y., Fan, D., Duan, Z., Fu, R., Liang, L., et al. (2018). Stability improvement of human collagen α1(I) chain using insulin as a fusion partner. Chin. J. Chem. Eng. 26 (12), 2607–2614. doi:10.1016/j.cjche.2018.04.008
Minor, A. J., and Kareen, L. K. (2020). Engineering a collagen matrix for cell-instructive regenerative angiogenesis. J. Biomed. Mater Res. B Appl. Biomater. 108 (6), 2407–2416. doi:10.1002/jbm.b.34573
Muhonen, V., Narcisi, R., Nystedt, J., Korhonen, M., van Osch, G. J. V. M., and Kiviranta, I. (2017). Recombinant human type ii collagen hydrogel provides a xeno-free 3d micro-environment for chondrogenesis of human bone marrow-derived mesenchymal stromal cells. J. Tissue Eng. Regen. Med. 11 (3), 843–854. doi:10.1002/term.1983
Muthusamy, S., Kannan, S., Lee, M., Sanjairaj, V., Lu, W. F., Fuh, J. Y. H., et al. (2021). 3D bioprinting and microscale organization of vascularized tissue constructs using collagen-based bioink. Biotechnol. Bioeng. 118 (8), 3150–3163. doi:10.1002/bit.27838
Naomi, R., Ridzuan, P. M., and Polymers Bahari, H. (2021). Current insights into collagen type I. Polym. (Basel). 13 (16), 2642. doi:10.3390/polym13162642
Nguyen, T.-U., Watkins, K. E., and Kishore, V. (2019). Photochemically crosslinked cell-laden methacrylated collagen hydrogels with high cell viability and functionality. J. Biomed. Mat. Res. A 107 (7), 1541–1550. doi:10.1002/jbm.a.36668
Nocera, A. D., Comín, R., Salvatierra, N. A., and Paula Cid, M. (2018). Development of 3d printed fibrillar collagen scaffold for tissue engineering. Biomed. Microdevices 20, 26–13. doi:10.1007/s10544-018-0270-z
Osidak, E. O., Vadim Igorevich, K., Mariya Sergeevna, O., and Sergey Petrovich, D. (2020). Collagen as bioink for bioprinting: a comprehensive review. Int. J. Bioprint. 6 (3), 270. doi:10.18063/ijb.v6i3.270
Parmar, P. A., Jean-Philippe St-Pierre, L. W. C., Spicer, C. D., Stoichevska, V., Peng, Y. Y., Werkmeister, J. A., et al. (2017). Enhanced articular cartilage by human mesenchymal stem cells in enzymatically mediated transiently RGDS-functionalized collagen-mimetic hydrogels. Acta Biomater. 51, 75–88. doi:10.1016/j.actbio.2017.01.028
Quinlan, E., López-Noriega, A., Thompson, E. M., Hibbitts, A., Cryan, S. A., and O'Brien, F. J. (2017). Controlled release of vascular endothelial growth factor from spray-dried alginate microparticles in collagen-hydroxyapatite scaffolds for promoting vascularization and bone repair: functionalized CHA scaffolds for controlled release of VEGF to enhance bone repair. J. Tissue Eng. Regen. Med. 11 (4), 1097–1109. doi:10.1002/term.2013
Radke, D., Jia, W., Sharma, D., Fena, K., Wang, G., Goldman, J., et al. (2018). Tissue engineering at the blood-contacting surface: a review of challenges and strategies in vascular graft development. Adv. Healthc. Mater. 7 (15), 1701461. doi:10.1002/adhm.201701461
Ramírez-Rodríguez, G. B., Montesi, M., Panseri, S., Sprio, S., and Sandri, M. (2017). Biomineralized recombinant collagen-based scaffold mimicking native bone enhances mesenchymal stem cell interaction and differentiation. Tissue Eng. Part A 23 (23-24), 1423–1435. doi:10.1089/ten.tea.2017.0028
Rappu, P., M Salo, A., Myllyharju, J., and Heino, J. (2019). Role of prolyl hydroxylation in the molecular interactions of collagens. Essays Biochem. 63 (3), 325–335. doi:10.1042/ebc20180053
Rashedi, I., Talele, N., Wang, X.-H., Hinz, B., Radisic, M., and Keating, A. (2017). Collagen scaffold enhances the regenerative properties of mesenchymal stromal cells. PLoS ONE 12 (10), e0187348. doi:10.1371/journal.pone.0187348
Rico-Llanos, G. A., Borrego-González, S., Moncayo-Donoso, M., Becerra, J., and Visser, R. (2021). Collagen type I biomaterials as scaffolds for bone tissue engineering. Polym. (Basel). 13 (4), 599. doi:10.3390/polym13040599
Rittié, L. (2017), Type I collagen purification from rat tail tendons. Methods Mol Biol 1627, 287–308. doi:10.1007/978-1-4939-7113-8_19
Roshanbinfar, K., Kolesnik-Gray, M., Angeloni, M., Schruefer, S., Fiedler, M., Schubert, D. W., et al. (2023). Collagen hydrogel containing polyethylenimine-gold nanoparticles for drug release and enhanced beating properties of engineered cardiac tissues. Adv. Healthc. Mater. 12, 2202408. doi:10.1002/adhm.202202408
Sarrigiannidis, S. O., Rey, J. M., Dobre, O., González-García, C., J Dalby, M., and Salmeron-Sanchez, M. (2021). A tough act to follow: collagen hydrogel modifications to improve mechanical and growth factor loading capabilities. Mat. Today Bio 10, 100098. doi:10.1016/j.mtbio.2021.100098
Sheehy, E. J., Cunniffe, G. M., and O'Brien, F. J. (2018). “Collagen-based biomaterials for tissue regeneration and repair,” in Peptides and proteins as biomaterials for tissue regeneration and repair (Elsevier), 127-50.
Shekhter, A. B., Fayzullin, A. L., Vukolova, M. N., Rudenko, T. G., Osipycheva, V. D., and Litvitsky, P. F. (2019). Medical applications of collagen and collagen-based materials. Curr. Med. Chem. 26 (3), 506–516. doi:10.2174/0929867325666171205170339
Shuai, X., Kang, N., Li, Y., Bai, M., Zhou, X., Zhang, Y., et al. (2023). Recombination humanized type iii collagen promotes oral ulcer healing. Oral Dis. doi:10.1111/odi.14540
Song, K.-Mo, Jung, S. K., Kim, Y.Ho, Kim, Y. E., and Hyouck Lee, N., (2018). Development of industrial ultrasound system for mass production of collagen and biochemical characteristics of extracted collagen. Food Bioprod. Process., 110, 96–103. doi:10.1016/j.fbp.2018.04.001
Stepanovska, J., Supova, M., Hanzalek, K., Broz, A., and Matejka, R. (2021). Collagen bioinks for bioprinting: a systematic review of hydrogel properties, bioprinting parameters, protocols, and bioprinted structure characteristics. Biomedicines 9 (9), 1137. doi:10.3390/biomedicines9091137
Strauss, K., and Chmielewski, J. (2017). Advances in the design and higher-order assembly of collagen mimetic peptides for regenerative medicine. Curr. Opin. Biotechnol. 46, 34–41. doi:10.1016/j.copbio.2016.10.013
Su, J., Li, J., Liang, J., Zhang, K., and Li, J. (2021). Hydrogel preparation methods and biomaterials for wound dressing. Life 11 (10), 1016. doi:10.3390/life11101016
Sun, L., Gao, W., Fu, X., Shi, M., Xie, W., Zhang, W., et al. (2018). Enhanced wound healing in diabetic rats by nanofibrous scaffolds mimicking the basketweave pattern of collagen fibrils in native skin. Biomater. Sci. 6 (2), 340–349. doi:10.1039/c7bm00545h
Tang, M., Rich, J. N., and Chen, S. (2021). Biomaterials and 3d bioprinting strategies to model glioblastoma and the blood–brain barrier. Adv. Mater. 33 (5), 2004776. doi:10.1002/adma.202004776
Thapa, R. K., Margolis, D. J., Kiick, K. L., and Millicent, O. (2020). Enhanced wound healing via collagen-turnover-driven transfer of PDGF-BB gene in a murine wound model. ACS Appl. Bio Mat. 3 (6), 3500–3517. doi:10.1021/acsabm.9b01147
Tytgat, L., Agnes Dobos, A, Markovic, M., Lana Van Damme, , Van Hoorick, J., Bray, F., et al. (2020). High-resolution 3d bioprinting of photo-cross-linkable recombinant collagen to serve tissue engineering applications. Biomacromolecules 21 (10), 3997–4007. doi:10.1021/acs.biomac.0c00386
Wang, C., Brisson, B. K., Terajima, M., Li, Q., Hoxha, K., Han, B., et al. (2020). Type III collagen is a key regulator of the collagen fibrillar structure and biomechanics of articular cartilage and meniscus. Matrix Biol. 85, 47–67. doi:10.1016/j.matbio.2019.10.001
Wang, H. (2021). A review of the effects of collagen treatment in clinical studies. Polym. (Basel). 13 (22), 3868. doi:10.3390/polym13223868
Wang, J., Hu, H., Wang, J., He, Q., Gao, Y., Xu, Y., et al. (2022). Characterization of recombinant humanized collagen type iii and its influence on cell behavior and phenotype. John J. Leather Sci. Ramshaw, Eng. 4 (1), 33. doi:10.1186/s42825-022-00103-5
Włodarczyk-Biegun, M. K., and Del Campo, A. (2017). 3D bioprinting of structural proteins. Biomaterials 134, 180–201. doi:10.1016/j.biomaterials.2017.04.019
Woodley, D. T., Cogan, J., Hou, Y., Lyu, C., Marinkovich, M. P., Keene, D., et al. (2017). Gentamicin induces functional type VII collagen in recessive dystrophic epidermolysis bullosa patients. J. Clin. Invest. 127 (8), 3028–3038. doi:10.1172/jci92707
Xia, H., Li, X., Gao, W., Fu, X., Fang, R. H., Zhang, L., and Zhang, K. (2018). Tissue repair and regeneration with endogenous stem cells. Nat. Rev. Mat. 3 (7), 174–193. doi:10.1038/s41578-018-0027-6
Xu, L., Liu, Y., Tang, L., Xiao, H., Yang, Z., and Wang, S. (2022). Preparation of recombinant human collagen iii protein hydrogels with sustained release of extracellular vesicles for skin wound healing. Int. J. Mol. Sci. 23 (11), 6289. doi:10.3390/ijms23116289
Yang, Li, Wu, H., Lu, Lu, He, Q., Xi, B., Yu, H., et al. (2021b). A tailored extracellular matrix (Ecm)-Mimetic coating for cardiovascular stents by stepwise assembly of hyaluronic acid and recombinant human type iii collagen, Biomaterials 276, 121055. doi:10.1016/j.biomaterials.2021.121055
Yang, Y., and Ritchie, A. C. (2021a). Recombinant human collagen/chitosan-based soft hydrogels as biomaterials for soft tissue engineering. Mater Sci Eng C Mater Biol Appl. 121, 111846. doi:10.1016/j.msec.2020.111846
Yang, Y., Wang, Z., Xu, Y., Xia, J., Xu, Z., Zhu, S., et al. (2022a). Preparation of chitosan/recombinant human collagen-based photo-responsive bioinks for 3d bioprinting. Gels 8 (5), 314. doi:10.3390/gels8050314
Yang, Y., Xu, R., Wang, C., Guo, Y., Sun, W., and Ouyang, L. (2022b). Recombinant human collagen-based bioinks for the 3D bioprinting of full-thickness human skin equivalent. Int. J. Bioprinting 4, 18.
Zhang, D., Wu, X., Chen, J., and Lin, K. (2018b). The development of collagen based composite scaffolds for bone regeneration. Bioact. Mat. 3 (1), 129–138. doi:10.1016/j.bioactmat.2017.08.004
Zhang, N., Cheng, Y., Hu, X., and Yeo, J. (2019). Toward rational algorithmic design of collagen-based biomaterials through multiscale computational modeling. Curr. Opin. Chem. Eng. 24, 79–87. doi:10.1016/j.coche.2019.02.011
Zhang, W., Bai, X., Zhao, B., Li, Y., Zhang, Y., Li, Z., et al. (2018a). Cell-free therapy based on adipose tissue stem cell-derived exosomes promotes wound healing via the PI3K/Akt signaling pathway. Exp. Cell Res. 370 (2), 333–342. doi:10.1016/j.yexcr.2018.06.035
Zhang, Yi, Kumar, P., Lv, S., Xiong, Di, Zhao, H., Cai, Z., et al. (2021). Recent advances in 3D bioprinting of vascularized tissues. Recent Adv. 3d Bioprinting Vasc. Tissues 199, 109398. doi:10.1016/j.matdes.2020.109398
Keywords: tissue engineering, regenerative, biomaterials, recombinant human collagen, biomedicine
Citation: Cao L, Zhang Z, Yuan D, Yu M and Min J (2024) Tissue engineering applications of recombinant human collagen: a review of recent progress. Front. Bioeng. Biotechnol. 12:1358246. doi: 10.3389/fbioe.2024.1358246
Received: 19 December 2023; Accepted: 31 January 2024;
Published: 14 February 2024.
Edited by:
Wenliang Wang, The University of Texas at Austin, United StatesReviewed by:
Seungil Kim, University of Pittsburgh, United StatesDana Akilbekova, Nazarbayev University, Kazakhstan
Copyright © 2024 Cao, Zhang, Yuan, Yu and Min. This is an open-access article distributed under the terms of the Creative Commons Attribution License (CC BY). The use, distribution or reproduction in other forums is permitted, provided the original author(s) and the copyright owner(s) are credited and that the original publication in this journal is cited, in accordance with accepted academic practice. No use, distribution or reproduction is permitted which does not comply with these terms.
*Correspondence: Lili Cao, caoll215@163.com