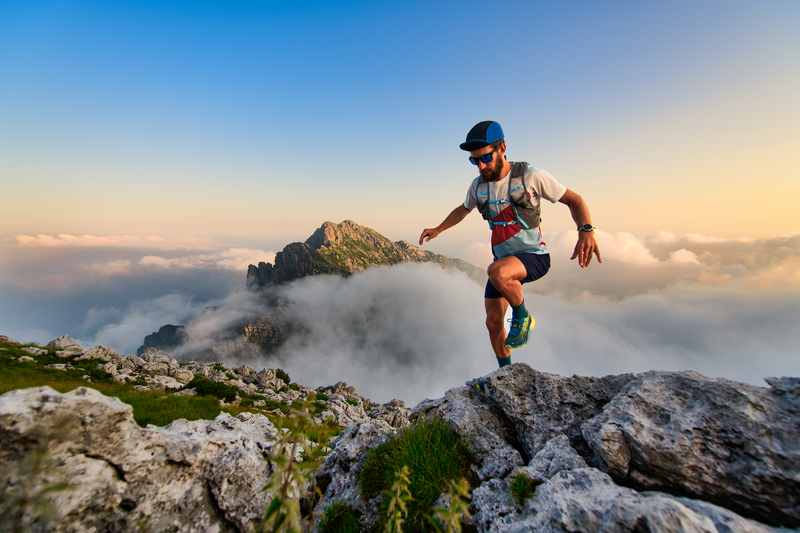
95% of researchers rate our articles as excellent or good
Learn more about the work of our research integrity team to safeguard the quality of each article we publish.
Find out more
REVIEW article
Front. Bioeng. Biotechnol. , 08 August 2024
Sec. Tissue Engineering and Regenerative Medicine
Volume 12 - 2024 | https://doi.org/10.3389/fbioe.2024.1357696
This article is part of the Research Topic Tendons and Ligaments: Development, Pathogenesis, Tissue Engineering, and Regenerative Medicine View all 11 articles
This review offered a comprehensive analysis of tendon and ligament injuries, emphasizing the crucial role of tendon-derived stem cells (TDSCs) in tissue engineering as a potential solution for these challenging medical conditions. Tendon and ligament injuries, prevalent among athletes, the elderly, and laborers, often result in long-term disability and reduced quality of life due to the poor intrinsic healing capacity of these avascular structures. The formation of biomechanically inferior scar tissue and a high rate of reinjury underscore the need for innovative approaches to enhance and guide the regenerative process. This review delved into the complexities of tendon and ligament structure and function, types of injuries and their impacts, and the limitations of the natural repair process. It particularly focused on the role of TDSCs within the context of tissue engineering. TDSCs, with their ability to differentiate into tenocytes, are explored in various applications, including biocompatible scaffolds for cell tracking, co-culture systems to optimize tendon-bone healing, and graft healing techniques. The review also addressed the challenges of immunoreactivity post-transplantation, the importance of pre-treating TDSCs, and the potential of hydrogels and decellularized matrices in supporting tendon regeneration. It concluded by highlighting the essential roles of mechanical and molecular stimuli in TDSC differentiation and the current challenges in the field, paving the way for future research directions.
Tendon and ligament injuries are common among athletes, the elderly, and individuals engaged in physical labor, comprising a significant portion of musculoskeletal disorders (Leong et al., 2020). These injuries not only cause pain and dysfunction but can also lead to long-term disability and a substantial decrease in quality of life. The complex structure and biomechanical properties of tendons and ligaments make them particularly challenging to heal, with current treatments often failing to fully restore the original strength and functionality (Cottrell et al., 2016). This highlights the clinical importance of developing more effective repair strategies.
The intrinsic healing capacity of tendons and ligaments is notably poor due to their avascular nature, which restricts the influx of cells and nutrients to the injury site (Andarawis-Puri et al., 2015). Consequently, the repair process is slow and often leads to the formation of scar tissue, lacking the original tissue’s biomechanical properties. As a result, there is a high rate of reinjury and an extended recovery period. These challenges necessitate innovative approaches that can enhance and direct the regenerative process, ensuring the restoration of the tendon or ligament to its native state (Nichols et al., 2019).
Tissue engineering has emerged as a promising field offering potential therapeutic strategies for the repair and regeneration of damaged tendons and ligaments (Lim et al., 2019). By incorporating principles from cell biology, material science, and engineering, tissue engineering aims to develop biological substitutes that can restore, maintain, or enhance tissue function (Ruiz-Alonso et al., 2021). This multidisciplinary approach often involves the utilization of stem cells (Tevlin et al., 2016), biocompatible scaffolds (Stace et al., 2018), and bioactive molecules (Atienza-Roca et al., 2018) to create an environment conducive to healing. With the capability to differentiate into tenocytes, the cells responsible for maintaining tendon structure and function, TDSCs are at the forefront of research as a cellular source for tissue-engineered constructs (Zhang et al., 2023). This literature review will explore the role of TDSCs within the context of tissue engineering and investigate how this synergy might pave the way for effective tendon and ligament repair.
Tendons and ligaments are vital components of the musculoskeletal system, serving as crucial connectors within the body. Tendons attach muscle to bone, enabling movement by transmitting forces generated by muscle contractions to the skeleton. Ligaments connect bones to each other, offering joint stability and guiding motion. Both structures consist of dense fibrous connective tissue, primarily composed of collagen fibers, which provide tensile strength and elasticity (Thorpe and Screen, 2016). The main structural component of tendons is type I collagen (Col I), a protein arranged in a highly ordered manner that forms the tendon’s primary load-bearing structure. Collagen molecules themselves have an elongated triple helix structure, and these molecules are further packed tightly together to form microfibers. Microfibers aggregate into thicker fiber bundles, and these fiber bundles are then aggregated to form large fiber bundles visible in tendons. These thicker fiber bundles can be called tendon clusters. Tendon clusters are composed of tendon inner chambers and tendon outer chambers. The inner compartment is composed of tenocytes and multiscale assembled collagen clusters, and the outer compartment is synovium-like tissue that connects blood vessels and the nervous system. Each level of structure increases the overall strength and elasticity of the tendon (Franchi et al., 2007; Thorpe and Screen, 2016). As show in Figures 1A, 2. The main cell types in tendons are tenocytes and tendon-specific stem cells which we evaluate in this review as TDSCs. These cells are distributed between collagen fibers and are responsible for synthesizing and breaking down collagen, thus participating in the maintenance, repair and regeneration of tendons. Tenocytes are flat and aligned parallel to the direction of collagen fibers, which helps them transmit mechanical and chemical signals efficiently throughout the tendon. In addition to collagen fibers and tenocytes, tendons also contain a certain amount of matrix and interstitium, which are filled between cells and fibers (Chen et al., 2022). The matrix is primarily composed of proteoglycans and glycoproteins, which are highly absorbent and help maintain tendon lubrication and serve as a medium for nutrient transport (Subramanian and Schilling, 2015). The presence of interstitium is critical to the overall health and function of the tendon, providing a supportive environment that helps maintain the tendon’s structural stability and transmit force. The microstructure of tendons shows a hierarchical tissue structure, from single collagen molecules to microfibers, to fiber bundles, and finally to the entire tendon. This hierarchical structure enables tendons to have extremely high mechanical properties, which can not only withstand high-intensity tensile forces, but also have a certain degree of elasticity, ensuring effective force transmission between muscles and bones (Zabrzyński et al., 2018). This distinctive composition and alignment enable tendons and ligaments to endure substantial mechanical stress during daily activities and athletic pursuits (Zitnay and Weiss, 2018).
Figure 1. Morphology and structure of tendons and TDSCs. (A) Morphological picture of tendon tissue; (B) Morphological picture of TDSCs. (A,B) are pictures obtained by the author in previous research.
From a developmental perspective, tendon tissue originates from the mesoderm in the embryo. Some mesoderm cells separate and concentrate in the ectoderm. These cells differentiate into bones, cartilage, tendons, and other connective tissues. Cells characterized by Sox9 positive expression in tendon tissue are considered to be the source of TDSCs. One of the defining characteristics of TDSCs lies in their capacity for self-renewal, which denotes their ability to replicate while retaining an undifferentiated state—an essential aspect for continual tissue maintenance. These cells exhibit multipotency, suggesting their potential to differentiate into various cell types, primarily tenocytes, but also potentially including adipocytes, chondrocytes, and osteocytes under specific conditions (Bi et al., 2007; Rui et al., 2010; Liu Q. et al., 2018). The morphological characteristics of TDSC mainly show spindle or fibroblast morphology (Figure 1B). The initial growth rate is slow, and it generally reaches 40%–50% confluence about 10 days after separation (Zhang Z. et al., 2021). Identification of TDSCs involves specific surface markers, such as Scleraxis (Scx), a critical transcription factor in tendon development, and Mohawk (Mkx), another tendon-specific transcription factor. Additional markers include tenomodulin (Tnmd), a type II transmembrane glycoprotein, and CD44, a cell surface glycoprotein involved in cell-cell and cell-matrix interactions. These markers are used to isolate and identify TDSCs from tendon tissues. Moreover, markers such as CD146, CD105, CD90, Oct4, SSEA-4, and Nucleostemin are also employed for identifying TDSCs (Yang et al., 2016; Lee et al., 2018; Jo et al., 2019; Li et al., 2021; Wei and Lu, 2021). At the cellular marker level, the molecular markers of TDSCs closely resemble those of bone marrow mesenchymal stem cells (BM-MSCs). However, TDSCs specifically express tendon markers such as Scx, Mkx, Tnmd, and Col I (Zhang et al., 2018). Similar to BM-MSCs, TDSCs possess differentiation potential toward osteoblasts, chondrocytes, tendons, and adipocytes. Notably, TDSCs exhibit enhanced chondrogenic and tenogenic differentiation capabilities (Jiang et al., 2023). Following tendon injury, the altered local microenvironment may induce aberrant differentiation of TDSCs, leading to impaired tendon repair (Zhang and Wang, 2014). TDSCs play a pivotal role in maintaining tendon homeostasis by contributing to the continuous turnover of the extracellular matrix within tendons, thereby ensuring structural integrity and functionality. Furthermore, they exhibit responsiveness to mechanical stimuli, a crucial aspect given the primary function of tendons in force transmission (Wang HN. et al., 2020). Changes in mechanical loading can influence TDSC behavior, impacting both their proliferation and differentiation.
Upon tendon injury, TDSCs become activated, migrating to the site of damage. Subsequently, they proliferate and differentiate to replace lost or damaged cells, playing a fundamental role in the initial stages of tendon repair. However, their response may occasionally lead to aberrant healing, characterized by scar tissue formation, highlighting the necessity for precise modulation of TDSC activity to optimize tendon repair (Lui and Chan, 2011; Huang et al., 2021).
TDSCs exhibit substantial promise in the realms of regenerative medicine and tissue engineering. These cells can be harvested, cultured, and potentially subjected to genetic modification to augment their regenerative potential. They are explored in various therapeutic approaches, including stem cell injections (Yin et al., 2018; Chen et al., 2021), tissue-engineered constructs (Zhang and Cheng, 2013), and gene therapy (Lai et al., 2022), to improve tendon repair and function. TDSCs play an integral role in tendon biology, contributing significantly to both the maintenance and repair of tendon tissues. Their capacity for self-renewal and differentiation, combined with their responsiveness to mechanical stimuli, positions them as pivotal targets in strategies designed to improve tendon regeneration and repair (Harvey et al., 2019). Understanding the detailed characteristics and behaviors of TDSCs is imperative for advancing therapies related to tendons and ameliorating outcomes in tendon injuries and disorders.
The isolation of TDSCs entails enzymatic digestion of tendon tissue to release the cells, followed by employing cell sorting techniques like flow cytometry to isolate stem cell markers. The digestive juice currently used to digest tendon tissue is mainly type I collagenase (Randelli et al., 2016a) or type II collagenase (Brown et al., 2014). Upon isolation, TDSCs are cultured in specialized media that sustains their growth and stemness. These conditions often incorporate a three-dimensional scaffold mirroring the native tendon environment, thereby fostering natural behavior and differentiation potential in the cells. However, reported culture conditions exhibit variance across studies. While the most commonly used medium is low-glucose. Dulbecco’s modified Eagle medium (DMEM) medium (Chen et al., 2012; Qiu et al., 2019; Ni et al., 2021), others include high-glucose DMEM medium (Brown et al., 2014; Rajpar and Barrett, 2020), α-MEM medium (Feng et al., 2020), or DMEM/F12 medium (Song et al., 2017). Moreover, experiments commonly supplement with 10%–20% fetal bovine serum (Randelli et al., 2016b; Yin et al., 2019; Lai et al., 2022; Ni et al., 2023). In addition to culture media and serum, researchers often add certain small molecule substances to the culture system to promote cell growth. The most common ones are ascorbic acid (Popov et al., 2015a) and L-glutamine (Ni et al., 2012). A few researchers also add Growth factor fibroblast growth factor-2 (FGF2) (Di Meglio et al., 2020) or connective tissue growth factor (CTGF) (Lui et al., 2016). However, there is currently no absolutely unified standard for the training system, which may be related to the habits of researchers and the specific purpose of the experiment.
Progress in culture techniques, exemplified by dynamic bioreactors applying mechanical stimulation, has notably amplified both the proliferation and tenogenic differentiation of TDSCs, yielding a more physiologically relevant model for investigating tendon biology and regenerative therapies. Table 1 illustrates 10 representative cell isolation and culture conditions. Recent research has indicated that 3D culture systems favor the growth and differentiation of TDSCs, establishing them as a pivotal entry point for tissue engineering (Hsieh et al., 2018; Yin et al., 2020).
TDSCs represent a distinct subset of stem cells located within tendons, characterized by their unique ability to differentiate into tenocytes, the fundamental cells responsible for maintaining tendon structure and function. The domain of tendon tissue engineering endeavors to harness the regenerative potential of these cells to mend or substitute damaged tendon tissue, posing a considerable challenge owing to the restricted healing capabilities of tendons. Tables 2–4 provides an overview of ongoing research concerning TDSCs in tendon tissue engineering.
Pre-treatment of TDSCs involves subjecting these cells to specific growth factors or environmental cues before transplantation. This process aims to augment the cells’ inherent healing capabilities, rendering them more effective once introduced into the injury site. Furthermore, this approach can be customized to promote specific cellular behaviors, such as increased proliferation or directed differentiation, which are indispensable for facilitating effective tendon repair (Wang et al., 2023).
In the research on TDSCs treatment of tendon-related diseases, the study of molecular mechanisms has always been a hot topic. A clear molecular mechanism can provide a reference for pretreatment of seed cells for tissue engineering. Cheng et al. (2014) intervened the expression of TNF alpha-stimulated gene/protein 6 (TSG-6) in TDSC and found in the experiment that TDSCs can promote the repair of rotator cuff injury. Therefore, TSG-6 plays an important role in TDSCs repairing rotator cuff injuries. In a human TDSC study, TDSCs from fetal Achilles tendons were isolated and cultured to overexpress FGF-2. The researchers found that after overexpression of FGF-2, the expression of tendon-related factors Scx and type III collagen increased. Transplanting FGF-2 overexpressing TDSC into the Achilles tendon notch in rats can promote Achilles tendon healing (Guo et al., 2020). In the process of tendinopathy, inflammation and programmed death are pathological processes that inhibit tendon self-repair. Tripartite Motif Containing 54 (TRIM54) inhibits inflammation and apoptosis in rat TDSCs by stabilizing YOD1 deubiquitinase (YOD1) (Chen et al., 2024).
Promoting tendon directional differentiation of TDSCs is an important research topic in this field. The study found that STIP1 homology and U-box containing protein 1 (STUB1) (Han W. et al., 2017), transforming growth factor-beta 1 (TGF-β1) (Han P. et al., 2017), and focal adhesion kinase- Extracellular signal-regulated kinase 1/2 (FAK-ERK1/2) signaling pathways (Liu C. et al., 2018) are factors that promote the differentiation of TDSC into tendon, while tumor necrosis factor α (TNF-α) (Han P. et al., 2017), interleukin 10 (IL -10) (Deng et al., 2018) and the janus kinase/signal transducer and activator of transcription 3 (JAK/Stat3) signaling pathways (Deng et al., 2018) are inhibitors of this process. In tendon diseases, in addition to the repair of tendon tissue, the repair of the tendon-bone connection is a more important issue. Because in most tendon diseases, such as rotator cuff injuries, cruciate ligament reconstruction, etc., it is necessary to restore the connection and normal physiological gradient between the tendon and bone. TDSCs are often used to study the role of tendon-bone junction repair. The normal tendon-bone insertion gradient is a bone-mineralized fibrocartilage-nonmineralized fibrocartilage-tendon structure. Researchers study the gradient of tendon-bone insertion point repair by TDSCs from different angles and mechanisms. Studies have found that bone morphogenetic protein 2 (BMP-2) and TGF-β1 can promote osteogenic and tenogenic differentiation of mouse TDSC in vivo and in vitro (Wei et al., 2023). As an upstream signal of TGF-β1, transforming growth interacting factor 1 (TGIF1) plays an inhibitory role in the chondrogenic differentiation process of TDSCs. Manipulating the expression of TGIF1 can promote the repair of tendon-bone junctions (Chen et al., 2015). The aging of stem cells is manifested by the decrease in differentiation and proliferation capabilities, apoptosis or fibrosis, resulting in a decrease in the tendon’s ability to self-repair. Targeting the aging mechanism of TDSCs can provide important targets for the treatment of tendon-related diseases. A study on human-derived TDSCs found that the expression of CBP/p300-interacting transactivator with Glu/Asp-rich C-terminal domain, 2 (CITED2) decreased in aging tendon, and TGFβ2 regulated the aging of TDSC through CITED2 (Hu et al., 2017). Inflammation can induce premature senescence of TDSCs, and the NF-κB signaling pathway plays an important regulatory role in this process (Xu K. et al., 2021; Wang et al., 2022). During tendon repair, excessive osteogenic differentiation can cause complications of heterotopic ossification. In rat TDSCs, ERK1/2 is involved in the osteogenic differentiation of TDSC (Li et al., 2016).
Co-culture systems, involving the simultaneous cultivation of diverse cell types like BM-MSCs and TDSCs, play a pivotal role in enhancing tendon-bone healing in tissue engineering. The study by Liu et al. (2019) demonstrated that optimal co-culture ratios, particularly a 1:1 ratio of BM-MSCs and TDSCs, significantly boost cell proliferation, differentiation, and tenogenic activities. These interactions are further influenced by factors such as Tenascin C, capable of impacting cellular behaviors like osteogenesis and chondrogenesis. Additionally, molecular pathways involving Rho-associated kinase (ROCK), insulin-like growth factor 1 receptor (IGF-1R), and methyl ethyl ketone (MEK) are implicated in these processes, highlighting a complex regulatory network. Another study suggests that a balanced co-culture of BM-MSCs and TDSCs not only enhances proliferation and collagen production but also elevates tenogenic marker gene expression and collagen matrix production, particularly in equal proportion co-cultures (Wu et al., 2016). This approach has been proven to effectively promote tendon healing in a rat model, underscoring its potential as an enhanced cell source for tendon tissue engineering (Figure 3).
Moreover, interactions between human adipose-derived stem cells and tendon cells have shown temporal regulation of tenogenesis, emphasizing the significance of extracellular matrix (ECM) synthesis and remodeling in co-cultured systems. These interactions lead to controlled cell proliferation and elongation, along with an improved ratio of collagen type I to III, crucial for generating high-quality tendon tissue. Co-culture systems in tendon tissue engineering are indispensable for optimizing tendon-bone healing (Costa-Almeida et al., 2018). The synergy between different cell types in co-cultures, coupled with the regulation of specific molecular pathways and ECM remodeling, plays a vital role in enhancing tendon repair and regeneration.
The application of TDSC sheets has significantly advanced graft healing techniques in tendon repair, particularly in anterior cruciate ligament (ACL) reconstruction. These sheets, often combined with growth factors, serve as a direct source of reparative cells and bioactive molecules at the injury site, thereby fostering improved graft integration and healing outcomes. In a rat model, TDSC sheets were employed to encase ACL grafts. Treated with connective tissue growth factor and ascorbic acid, the sheets augmented graft healing by elevating tunnel bone mineral density and bone volume, enhancing osteointegration, and preserving greater intra-articular graft integrity. These findings were supported by the presence of GFP-positive cells, signifying the successful integration of the TDSC sheets into the healing process (Lui et al., 2014). Zhang Y. et al. (2021) implemented a stepwise culture strategy for TDSCs, resulting in a significant upregulation of tendon-related genes and proteins. By integrating this culture system with 3D printing technology, researchers embedded chemically empowered TSPCs within a biocompatible hydrogel to engineer tendon grafts, displaying an enhanced capacity to promote functional tendon repair and regeneration both in vivo and in situ. Using genetically modified TDSCs to improve cell sheet properties. Wei et al. (2023) found that BMP-2 can promote osteogenic differentiation of TDSC, while TGF-β1 can promote tenogenic differentiation of TDSC. Simultaneous transfection of BMP-2 and TGF-β1 into TDSCs can significantly improve the maturation of tendon-bone junctions. If the Cell sheet is made with allogeneic TDSC, it may cause rejection (Dang et al., 2021). Therefore, decellularization and leaving the extracellular matrix will greatly reduce the possibility of rejection (Inci et al., 2020). Yao et al. (2023) investigated the use of decellularized TDSC (dTDSC) sheets, which retained the bioactive factors of the natural extracellular matrix and fostered graft healing after ACLR. These sheets exhibited heightened bone formation, improved graft osteointegration, and enhanced midsubstance graft integrity. This method also influenced macrophage polarization and MMP/TIMP expression, contributing to better healing outcomes. These advancements underscore the potential of TDSC sheets in enhancing graft healing in tendon repair, particularly in ACL reconstruction. Through leveraging the unique properties of TDSCs and innovative techniques such as 3D printing and decellularization, these approaches offer promising strategies to improve graft integration, facilitate tissue regeneration, and ultimately enhance the outcomes of tendon repair surgeries.
Mechanical and molecular stimuli play pivotal roles in influencing the behavior and differentiation of TDSCs. Mechanical stimuli, such as stretch or compression, mimic the natural mechanical environment of tendons, while molecular stimuli encompass signaling molecules that can guide cell behavior. Understanding the impact of these stimuli on TSPC differentiation is crucial for devising effective strategies for tendon repair. Several research endeavors elucidate these mechanisms. In their study, Liu et al. (2017) delved into the influence of mechanical stretching on TDSC differentiation, revealing the crucial role of the cystic fibrosis transmembrane conductance regulator (CFTR) in tenogenic differentiation. Their findings indicated irregularities, diminished mechanical properties, and reduced matrix formation in tendon tissues of CFTR-dysfunctional mice. Furthermore, RNA sequencing analysis unveiled abnormal activation of the Wnt/β-catenin signaling pathway in TDSCs from these mice. Notably, inhibiting the pERK1/2 signaling pathway fostered tenogenic differentiation and tendon regeneration, suggesting that CFTR regulates tendon differentiation through the β-catenin/pERK1/2 pathway.
Zhang and Wang (2010) investigated the impact of mechanical stretching on TDSC behavior, observing that low mechanical stretching facilitated TDSC differentiation into tenocytes, potentially maintaining tendon homeostasis. However, excessive stretching led to differentiation into non-tenocyte lineages, contributing to tendinopathy features such as lipid accumulation and tissue calcification. Additionally, Chen et al. (2012) explored the effect of autologous platelet-rich clot releasate (PRCR) on TDSCs subjected to mechanical stretching, finding that PRCR increased TDSC numbers and the production of collagen types I and III and TGF-β1, favoring tenocyte differentiation while suppressing adipocyte, chondrocyte, and osteocyte lineages.
Researchers have also discovered that mechanical stress impacts the tendon-bone healing process. Continuous passive motion (CPM) therapy has been shown to enhance healing by promoting fibrocartilage formation, increasing load-bearing capacity, and up-regulating key genes at the tendon-bone interface. Moreover, mechanical stretch has been found to improve cell proliferation and matrix synthesis in a co-culture of BM-MSCs and TCs, underscoring the regenerative potential of local precursor cells in tendon-bone healing (Song et al., 2017). The interplay between mechanical and molecular stimuli in TDSC differentiation and tendon healing offers valuable insights for future tendon injury management strategies.
For TDSCs, the matrix morphology and surface morphology in the cell culture environment have an important impact on the differentiation of TDSCs. Culturing normal TDSCs on a stiff matrix can promote TDSCs maturation and tendon differentiation (Kim et al., 2018). Preserving the stemness of TDSCs in vitro culture is a very important issue (Zhang and Wang, 2013; Chen et al., 2016; Yu et al., 2017). Compared with ordinary plastic culture plates, culturing TDSC on the surface of decellularized tendons can preserve the stemness of TDSC (Zhang et al., 2011). The use of a specially designed parallel microgrooved polydimethylsiloxane (PDMS) membrane to culture TDSCs can guide the elongation of TDSCs. Under such culture conditions, the ability of TDSCs to differentiate into adipogenesis, chondrogenesis, and osteogenesis is significantly inhibited. The ability of tendon differentiation is enhanced (Shi et al., 2017). Therefore, the application of hard, regular, and parallel culture matrix morphology in vitro culture systems may be meaningful for subsequent stem cell treatments.
Scaffold-based regeneration involves the use of engineered structures specifically designed to facilitate cell attachment, growth, and differentiation. Collagen fiber scaffold used in research on tendon-related diseases (Fan et al., 2008; Sun et al., 2014; Tabesh et al., 2022). Ouyang’s team used homemade collagen fiber scaffolds (Chen et al., 2008) as carriers for TDSCs. In vivo experiments observed that the combination of collagen fiber scaffolds and TDSCs resulted in significant collagen production and reduced immune rejection (Shen et al., 2012). Compared with randomly arranged collagen fiber scaffolds, parallel-arranged collagen fiber scaffolds can achieve better mechanical properties as carriers of TDSCs (Zheng et al., 2017). Decellularized matrices represent scaffolds derived from natural tissues, wherein cellular components are removed to minimize immunogenicity while retaining the structural and functional cues of the extracellular matrix. Within tendon tissue engineering, these matrices serve as a foundational support guiding TDSC differentiation and promoting tissue regeneration, thus playing a pivotal role in the development of tissue-engineered tendon constructs. Investigative studies have delved into their influence on TDSCs and tendon repair. Collagen matrices extracted from various tissues (tendon, bone, and dermis) facilitated TSPC adhesion and proliferation. Notably, tendon-derived matrix encouraged a tendinous phenotype and suppressed osteogenesis in hTDSCs (Yin et al., 2013). Moreover, engineered tendon matrix (ETM) from decellularized tendon tissues stimulated TSPC proliferation and preserved stemness in vitro, subsequently enhancing the formation of tendon-like tissue in vivo, underscoring the potential of ETM in tendon healing (Zhang et al., 2011). When combined with TSPCs, decellularized extracellular matrix from porcine tendons demonstrated superior results in promoting tendon regeneration compared to mesenchymal stromal cells (Song et al., 2018). Additionally, decellularized tendon hydrogel from Macaca mulatta supported mTDSC proliferation, migration, and tenogenic differentiation, with the native ECM components of T-gel augmenting these behaviors (Ning et al., 2021). The application of decellularized matrices presents promising prospects in tendon tissue engineering, effectively steering TSPC behavior and fostering tendon repair.
Hydrogels utilized in tendon repair constitute water-swollen, cross-linked polymeric networks simulating the extracellular matrix, thus establishing an environment conducive to cell growth and tissue development. Numerous studies have investigated the potential of hydrogel systems combined with TSPCs to enhance tendon healing and regeneration. In a particular study, a sequential culture strategy using small molecules was developed to potentiate TSPCs for enhanced therapeutic applications. Through high-throughput screening, specific small molecules were identified, leading to heightened TSPC proliferation, initiation of tenogenesis, and subsequent maturation. When these chemically empowered TSPCs were embedded within a biocompatible hydrogel using 3D printing technology, they demonstrated improved functional tendon repair and regeneration in vivo (Zhang Y. et al., 2021). Another investigation assessed the use of chitosan/β-glycerophosphate/collagen (C/GP/Co) hydrogel in combination with TDSCs for Achilles tendon healing in a rat model. The research illustrated that local application of TDSCs with C/GP/Co hydrogel significantly improved tendon healing compared to control groups, as evidenced by histological, immunohistochemical, and biomechanical assessments (Yang et al., 2017).
Furthermore, Ying et al. (2020) explored the rejuvenation of aged/degenerative human TDSCs using a self-assembling nanofiber matrix composed of RADA peptide hydrogel. This nanocomposite hydrogel supported the survival, proliferation, and rejuvenation of TDSCs, potentially overcoming challenges associated with cell aging and degeneration in tendon repair. Thermosensitive hydrogels, including a butane diisocyanate (BDI)-collagen hydrogel and methylcellulose/polyvinyl alcohol/polyvinylpyrrolidone-based hydrogel, were examined as injectable cell delivery carriers for TDSCs. These hydrogels exhibited biocompatibility, supported TDSC behavior, and induced differentiation toward tenogenic and osteogenic lineages, making them valuable candidates for tendon tissue engineering (Yin et al., 2018). A magnetically-responsive nanocomposite hydrogel composed of collagen type I and aligned iron oxide nanoparticles showed potential for promoting the alignment and tenogenesis of TDSCs, addressing the issue of uniform cell arrangement in tendon tissue engineering (Xu Y. et al., 2021). Mao et al. (2022) utilized TDSCs combined with small intestinal submucosa (SIS) scaffolds to improve Achilles tendon repair. In vitro, SIS facilitated TDSC adhesion and tenogenic differentiation. In vivo, TDSCs-SIS scaffolds augmented tendon regeneration and reduced adhesion formation through M2 macrophage polarization. An injectable thermosensitive hydrogel containing kartogenin-loaded bioactive glass nanoparticles displayed promise in addressing rotator cuff injuries. It induced chondrogenesis and osteogenesis in TDSCs, promoting fibrocartilage and bone layer regeneration in a rabbit model of chronic cuff tears (Huang et al., 2022). Zhang et al. (2023) employed a RADA peptide hydrogel with human TSPCs for patellar tendon repair. Results demonstrated improved function recovery, enhanced matrix organization, reduced complications, and decreased heterotopic ossification, suggesting a promising approach for clinical tendon repair. The utilization of hydrogel-based approaches in conjunction with TSPCs presents compelling prospects for enhancing tendon repair and regeneration. Collectively, these studies underscored the potential of hydrogel systems to furnish a conducive microenvironment for TSPCs, foster tenogenesis, and ameliorate functional outcomes in tendon tissue engineering and clinical applications.
Allogeneic TSPCs, exhibiting typical stem cell characteristics, were employed in a study focusing on shoulder repair. These cells were seeded onto knitted silk-collagen sponge scaffolds, demonstrating clonogenicity, high proliferation, multidifferentiation potential, non-immunogenicity, and immunosuppressive properties. In a rabbit model, these TSPCs facilitated tendon regeneration by differentiating into tenocytes, diminishing immunological rejection, and augmenting collagen deposition and biomechanical properties (Chen et al., 2012). Silk-Based Materials in Tendon Engineering: Silk fibroin (SF) films, both with bionic microstructures and smooth surfaces, have exhibited promise in tendon tissue engineering. The 10 μm bionic SF film showcased mechanical properties comparable to native tendons. When TSPCs were seeded on these films, they underwent significant changes in cell morphology, prompted the upregulation of tenogenic genes (COL1A1, TNC, TNMD, SCX), and facilitated TSPC adherence and differentiation (Lu et al., 2020a). Furthermore, SF films with bionic microstructures activated focal adhesion kinase (FAK), playing a pivotal role in enhancing TSPC differentiation. In brief, silk-based materials, particularly bionic SF films, present potential for tendon repair by guiding TSPC morphology and promoting tenogenic differentiation through FAK activation (Lu et al., 2020b). These materials, especially SF films with bionic microstructures, demonstrate substantial promise in tendon tissue engineering. They not only guide TSPC morphology but also enhance tenogenic differentiation through FAK activation, making them customizable biomaterials for tendon repair applications.
The domain of TDSCs and tissue engineering is evolving towards a more comprehensive approach integrating advanced biomaterials, cellular therapies, and an understanding of the biomechanical environment. This multifaceted strategy holds significant promise for surmounting traditional challenges in tendon repair and regeneration, offering new pathways for effective treatments of tendon injuries and disorders.
Although there are currently no clinical studies specifically on tendon stem cells, several clinical studies on stem cells in tendon-related diseases have been initiated. Some of these studies have already reached conclusions. The stem cells used in these clinical studies are primarily derived from bone marrow and adipose tissue. Stem cell therapy has shown good safety in the treatment of tendon diseases. In the treatment of partial tears in the supraspinatus tendon, although stem cell injections did not show significant effects compared to placebo, all participants only reported transient pain at the injection site with no persistent adverse events (Chun et al., 2022). Additionally, the use of adipose-derived stem cells in treating lateral epicondylosis demonstrated no significant adverse effects, confirming their safety (Lee et al., 2015). Preoperative bone channelling combined with stem cell treatment in rotator cuff repair did not result in any adverse events or significant differences in healing rates compared to sham procedures (Lapner et al., 2021). Stem cell therapy has shown potential efficacy in the repair of tendon ruptures. Adipose-derived stem cells significantly improved structural outcomes in rotator cuff repair, although there were no clinical differences during a 28-month follow-up period; MRI data indicated a significantly lower retear rate in the treatment group compared to the control group (Kim et al., 2017). Moreover, bone marrow-derived stem cells used in the acute repair of achilles tendons showed no reruptures and allowed earlier resumption of walking and sporting activities (Stein et al., 2015). In another study, bone marrow-derived MSCs used in rotator cuff repair enhanced healing rates and prevented reruptures over a 10-year period, showing substantial improvements in tendon integrity (Hernigou et al., 2014). In the treatment of patellar tendinopathy, bone marrow-derived stem cells showed significant improvements in tendon structure at 6 months compared to leukocyte-poor platelet-rich plasma (Rodas et al., 2021). In chronic tendinopathy, adipose-derived stem cells provided faster recovery in pain relief and functional improvement compared to SVF treatment (Lee et al., 2015). For non-insertional Achilles tendinopathy, both PRP and SVF treatments significantly improved clinical scores, but SVF-treated patients achieved faster improvements (Gomes et al., 2012). Stem cell therapy has demonstrated good safety and potential efficacy in tendon-related diseases. Although in some cases stem cell treatments did not show significant improvements over conventional treatments, they have shown considerable advantages in improving tendon structural integrity. We believe that in current clinical research, the means of using stem cell therapy is simply injection. No optimization methods such as improving the microenvironment or using induction factors have been considered. These are the factors that are studied more in basic medical research. Future research should continue to explore optimal strategies and long-term effects in clinical applications to fully leverage the regenerative potential of stem cells.
In conclusion, the field of tendon tissue engineering, particularly involving TDSCs, is rapidly progressing, providing novel insights and strategies for addressing tendon and ligament injuries. The utilization of TDSCs in various therapeutic approaches, such as scaffold-based regeneration, has demonstrated promising results in enhancing tendon repair and function. Nonetheless, challenges remain in optimizing cell-scaffold interactions, sustaining long-term cell viability and function, and translating research findings into clinical applications. There are currently no clinical trials on TDSCs. Therefore clinical research is also very important in this field. Future research should concentrate on integrated approaches that combine advanced biomaterials, precise cellular manipulation, and a deeper understanding of the biomechanical environment of tendons. This multifaceted strategy possesses the potential to overcome the traditional challenges in tendon repair and regeneration, furnishing effective treatments for tendon injuries and disorders. As the discipline advances, it holds great promise for improving the outcomes of tendon repair, potentially revolutionizing the management of tendon and ligament injuries in clinical practice.
WH: Writing–original draft, Writing–review and editing. CJ: Conceptualization, Writing–review and editing. PZ: Writing–review and editing. XH: Writing–review and editing. XG: Writing–review and editing. SZ: Conceptualization, Writing–original draft, Writing–review and editing.
The author(s) declare that financial support was received for the research, authorship, and/or publication of this article. This study was supported by Shaoxing Science and Technology Plan Project (2023A14009) and the Shaoxing Health Science and Technology Plan (2023SK013).
The authors declare that the research was conducted in the absence of any commercial or financial relationships that could be construed as a potential conflict of interest.
All claims expressed in this article are solely those of the authors and do not necessarily represent those of their affiliated organizations, or those of the publisher, the editors and the reviewers. Any product that may be evaluated in this article, or claim that may be made by its manufacturer, is not guaranteed or endorsed by the publisher.
Alberton, P., Dex, S., Popov, C., Shukunami, C., Schieker, M., and Docheva, D. (2015). Loss of tenomodulin results in reduced self-renewal and augmented senescence of tendon stem/progenitor cells. Stem Cells Dev. 24 (5), 597–609. doi:10.1089/scd.2014.0314
Andarawis-Puri, N., Flatow, E. L., and Soslowsky, L. J. (2015). Tendon basic science: development, repair, regeneration, and healing. J. Orthop. Res. 33 (6), 780–784. doi:10.1002/jor.22869
Atienza-Roca, P., Cui, X., Hooper, G. J., Woodfield, T. B. F., and Lim, K. S. (2018). Growth factor delivery systems for tissue engineering and regenerative medicine. Adv. Exp. Med. Biol. 1078, 245–269. doi:10.1007/978-981-13-0950-2_13
Bi, Y., Ehirchiou, D., Kilts, T. M., Inkson, C. A., Embree, M. C., Sonoyama, W., et al. (2007). Identification of tendon stem/progenitor cells and the role of the extracellular matrix in their niche. Nat. Med. 13 (10), 1219–1227. doi:10.1038/nm1630
Brown, J. P., Finley, V. G., and Kuo, C. K. (2014). Embryonic mechanical and soluble cues regulate tendon progenitor cell gene expression as a function of developmental stage and anatomical origin. J. Biomech. 47 (1), 214–222. doi:10.1016/j.jbiomech.2013.09.018
Brown, J. P., Galassi, T. V., Stoppato, M., Schiele, N. R., and Kuo, C. K. (2015). Comparative analysis of mesenchymal stem cell and embryonic tendon progenitor cell response to embryonic tendon biochemical and mechanical factors. Stem Cell Res. Ther. 6 (1), 89. doi:10.1186/s13287-015-0043-z
Chen, B., Liang, Y., Zhang, J., Bai, L., Xu, M., Han, Q., et al. (2021). Synergistic enhancement of tendon-to-bone healing via anti-inflammatory and pro-differentiation effects caused by sustained release of Mg(2+)/curcumin from injectable self-healing hydrogels. Theranostics 11 (12), 5911–5925. doi:10.7150/thno.56266
Chen, E., Yang, L., Ye, C., Zhang, W., Ran, J., Xue, D., et al. (2018). An asymmetric chitosan scaffold for tendon tissue engineering: in vitro and in vivo evaluation with rat tendon stem/progenitor cells. Acta Biomater. 73, 377–387. doi:10.1016/j.actbio.2018.04.027
Chen, H., Chen, X., Yang, L., Sheng, S., Yang, J., Lu, Y., et al. (2024). TRIM54 alleviates inflammation and apoptosis by stabilizing YOD1 in rat tendon-derived stem cells. J. Biol. Chem. 300 (1), 105510. doi:10.1016/j.jbc.2023.105510
Chen, H., Ge, H. A., Wu, G. B., Cheng, B., Lu, Y., and Jiang, C. (2016). Autophagy prevents oxidative stress-induced loss of self-renewal capacity and stemness in human tendon stem cells by reducing ROS accumulation. Cell Physiol. Biochem. 39 (6), 2227–2238. doi:10.1159/000447916
Chen, L., Dong, S. W., Tao, X., Liu, J. P., Tang, K. L., and Xu, J. Z. (2012). Autologous platelet-rich clot releasate stimulates proliferation and inhibits differentiation of adult rat tendon stem cells towards nontenocyte lineages. J. Int. Med. Res. 40 (4), 1399–1409. doi:10.1177/147323001204000418
Chen, L., Jiang, C., Tiwari, S. R., Shrestha, A., Xu, P., Liang, W., et al. (2015). TGIF1 gene silencing in tendon-derived stem cells improves the tendon-to-bone insertion site regeneration. Cell Physiol. Biochem. 37 (6), 2101–2114. doi:10.1159/000438568
Chen, X., Qi, Y. Y., Wang, L. L., Yin, Z., Yin, G. L., Zou, X. H., et al. (2008). Ligament regeneration using a knitted silk scaffold combined with collagen matrix. Biomaterials 29 (27), 3683–3692. doi:10.1016/j.biomaterials.2008.05.017
Chen, Z., Chen, P., Ruan, R., Chen, L., Yuan, J., Wood, D., et al. (2020). Applying a three-dimensional uniaxial mechanical stimulation bioreactor system to induce tenogenic differentiation of tendon-derived stem cells. J. Vis. Exp. 162. doi:10.3791/61278
Chen, Z., Chen, P., Zheng, M., Gao, J., Liu, D., Wang, A., et al. (2022). Challenges and perspectives of tendon-derived cell therapy for tendinopathy: from bench to bedside. Stem Cell Res. Ther. 13 (1), 444. doi:10.1186/s13287-022-03113-6
Cheng, B., Ge, H., Zhou, J., and Zhang, Q. (2014). TSG-6 mediates the effect of tendon derived stem cells for rotator cuff healing. Eur. Rev. Med. Pharmacol. Sci. 18 (2), 247–251.
Cheung, T. S., Lau, P. M., Lu, H., Ho, H. P., Lui, P. P. Y., and Kong, S. K. (2016). Cytotoxic and sublethal effects of silver nanoparticles on tendon-derived stem cells - implications for tendon engineering. Toxicol. Res. (Camb). 5 (1), 318–330. doi:10.1039/c5tx00349k
Chun, S. W., Kim, W., Lee, S. Y., Lim, C. Y., Kim, K., Kim, J. G., et al. (2022). A randomized controlled trial of stem cell injection for tendon tear. Sci. Rep. 12 (1), 818. doi:10.1038/s41598-021-04656-z
Costa-Almeida, R., Calejo, I., Reis, R. L., and Gomes, M. E. (2018). Crosstalk between adipose stem cells and tendon cells reveals a temporal regulation of tenogenesis by matrix deposition and remodeling. J. Cell Physiol. 233 (7), 5383–5395. doi:10.1002/jcp.26363
Cottrell, J. A., Turner, J. C., Arinzeh, T. L., and O'Connor, J. P. (2016). The biology of bone and ligament healing. Foot Ankle Clin. 21 (4), 739–761. doi:10.1016/j.fcl.2016.07.017
Dang, L. H., Hung, S. H., Tseng, Y., Quang, L. X., Le, N. T. N., Fang, C. L., et al. (2021). Partial decellularized scaffold combined with autologous nasal epithelial cell sheet for tracheal tissue engineering. Int. J. Mol. Sci. 22 (19), 10322. doi:10.3390/ijms221910322
Deng, G., Li, K., Chen, S., Chen, P., Zheng, H., Yu, B., et al. (2018). Interleukin-10 promotes proliferation and migration, and inhibits tendon differentiation via the JAK/Stat3 pathway in tendon-derived stem cells in vitro. Mol. Med. Rep. 18 (6), 5044–5052. doi:10.3892/mmr.2018.9547
Di Meglio, F., Sacco, A., Belviso, I., Romano, V., Sirico, F., Loiacono, C., et al. (2020). Influence of supplements and drugs used for the treatment of musculoskeletal disorders on adult human tendon-derived stem cells. Muscles, Ligaments and Tendons J. (MLTJ). 10 (3), 376. doi:10.32098/mltj.03.2020.04
Fan, H., Liu, H., Wang, Y., Toh, S. L., and Goh, J. C. (2008). Development of a silk cable-reinforced gelatin/silk fibroin hybrid scaffold for ligament tissue engineering. Cell Transpl. 17 (12), 1389–1401. doi:10.3727/096368908787648047
Feng, H., Xing, W., Han, Y., Sun, J., Kong, M., Gao, B., et al. (2020). Tendon-derived cathepsin K-expressing progenitor cells activate Hedgehog signaling to drive heterotopic ossification. J. Clin. Invest. 130 (12), 6354–6365. doi:10.1172/jci132518
Franchi, M., Trirè, A., Quaranta, M., Orsini, E., and Ottani, V. (2007). Collagen structure of tendon relates to function. ScientificWorldJournal 7, 404–420. doi:10.1100/tsw.2007.92
Gomes, J. L. E., da Silva, R. C., Silla, L. M., Abreu, M. R., and Pellanda, R. (2012). Conventional rotator cuff repair complemented by the aid of mononuclear autologous stem cells. Knee Surg. Sports Traumatol. Arthrosc. 20 (2), 373–377. doi:10.1007/s00167-011-1607-9
Guo, D., Li, H., Liu, Y., Yu, X., Zhang, X., Chu, W., et al. (2020). Fibroblast growth factor-2 promotes the function of tendon-derived stem cells in Achilles tendon restoration in an Achilles tendon injury rat model. Biochem. Biophys. Res. Commun. 521 (1), 91–97. doi:10.1016/j.bbrc.2019.10.082
Han, P., Cui, Q., Lu, W., Yang, S., Shi, M., Li, Z., et al. (2019). Hepatocyte growth factor plays a dual role in tendon-derived stem cell proliferation, migration, and differentiation. J. Cell Physiol. 234 (10), 17382–17391. doi:10.1002/jcp.28360
Han, P., Cui, Q., Yang, S., Wang, H., Gao, P., and Li, Z. (2017b). Tumor necrosis factor-α and transforming growth factor-β1 facilitate differentiation and proliferation of tendon-derived stem cells in vitro. Biotechnol. Lett. 39 (5), 711–719. doi:10.1007/s10529-017-2296-3
Han, W., Chen, L., Liu, J., and Guo, A. (2017a). Enhanced tenogenic differentiation and tendon-like tissue formation by CHIP overexpression in tendon-derived stem cells. Acta Biochim. Biophys. Sin. (Shanghai) 49 (4), 311–317. doi:10.1093/abbs/gmx005
Harvey, T., Flamenco, S., and Fan, C. M. (2019). A Tppp3(+)Pdgfra(+) tendon stem cell population contributes to regeneration and reveals a shared role for PDGF signalling in regeneration and fibrosis. Nat. Cell Biol. 21 (12), 1490–1503. doi:10.1038/s41556-019-0417-z
Hernigou, P., Flouzat Lachaniette, C. H., Delambre, J., Zilber, S., Duffiet, P., Chevallier, N., et al. (2014). Biologic augmentation of rotator cuff repair with mesenchymal stem cells during arthroscopy improves healing and prevents further tears: a case-controlled study. Int. Orthop. 38 (9), 1811–1818. doi:10.1007/s00264-014-2391-1
Hsieh, C. F., Yan, Z., Schumann, R. G., Milz, S., Pfeifer, C. G., Schieker, M., et al. (2018). In vitro comparison of 2D-cell culture and 3D-cell sheets of scleraxis-programmed bone marrow derived mesenchymal stem cells to primary tendon stem/progenitor cells for tendon repair. Int. J. Mol. Sci. 19 (8), 2272. doi:10.3390/ijms19082272
Hu, C., Zhang, Y., Tang, K., Luo, Y., Liu, Y., and Chen, W. (2017). Downregulation of CITED2 contributes to TGFβ-mediated senescence of tendon-derived stem cells. Cell Tissue Res. 368 (1), 93–104. doi:10.1007/s00441-016-2552-1
Huang, K., Du, J., Xu, J., Wu, C., Chen, C., Chen, S., et al. (2022). Tendon-bone junction healing by injectable bioactive thermo-sensitive hydrogel based on inspiration of tendon-derived stem cells. Mater. Today Chem. 23, 100720. doi:10.1016/j.mtchem.2021.100720
Huang, Z., Yin, Z., Xu, J., Fei, Y., Heng, B. C., Jiang, X., et al. (2021). Tendon stem/progenitor cell subpopulations and their implications in tendon biology. Front. Cell Dev. Biol. 9, 631272. doi:10.3389/fcell.2021.631272
Inci, I., Norouz Dizaji, A., Ozel, C., Morali, U., Dogan Guzel, F., and Avci, H. (2020). Decellularized inner body membranes for tissue engineering: a review. J. Biomater. Sci. Polym. Ed. 31 (10), 1287–1368. doi:10.1080/09205063.2020.1751523
Jiang, D., Xu, B., and Gao, P. (2018). Effects of young extracellular matrix on the biological characteristics of aged tendon stem cells. Adv. Clin. Exp. Med. 27 (12), 1625–1630. doi:10.17219/acem/75503
Jiang, L., Lu, J., Chen, Y., Lyu, K., Long, L., Wang, X., et al. (2023). Mesenchymal stem cells: an efficient cell therapy for tendon repair (Review). Int. J. Mol. Med. 52 (2), 70. doi:10.3892/ijmm.2023.5273
Jo, C. H., Lim, H. J., and Yoon, K. S. (2019). Characterization of tendon-specific markers in various human tissues, tenocytes and mesenchymal stem cells. Tissue Eng. Regen. Med. 16 (2), 151–159. doi:10.1007/s13770-019-00182-2
Kim, S. J., Tatman, P. D., Song, D. H., Gee, A. O., Kim, D. H., and Kim, S. J. (2018). Nanotopographic cues and stiffness control of tendon-derived stem cells from diverse conditions. Int. J. Nanomedicine 13, 7217–7227. doi:10.2147/ijn.s181743
Kim, Y. S., Sung, C. H., Chung, S. H., Kwak, S. J., and Koh, Y. G. (2017). Does an injection of adipose-derived mesenchymal stem cells loaded in fibrin glue influence rotator cuff repair outcomes? A clinical and magnetic resonance imaging study. Am. J. Sports Med. 45 (9), 2010–2018. doi:10.1177/0363546517702863
Lai, F., Wang, J., Tang, H., Huang, P., Liu, J., He, G., et al. (2022). VEGF promotes tendon regeneration of aged rats by inhibiting adipogenic differentiation of tendon stem/progenitor cells and promoting vascularization. Faseb J. 36 (8), e22433. doi:10.1096/fj.202200213r
Lapner, P., Pollock, J. W., Laneuville, O., Uhthoff, H. K., Zhang, T., Sheikh, A., et al. (2021). Preoperative bone marrow stimulation does not improve functional outcomes in arthroscopic cuff repair: a prospective randomized controlled trial. Bone Jt. J. 103-b (1), 123–130. doi:10.1302/0301-620x.103b1.bjj-2020-0011.r2
Laranjeira, M., Domingues, R. M. A., Costa-Almeida, R., Reis, R. L., and Gomes, M. E. (2017). 3D mimicry of native-tissue-fiber architecture guides tendon-derived cells and adipose stem cells into artificial tendon constructs. Small 13 (31). doi:10.1002/smll.201700689
Lee, K. J., Clegg, P. D., Comerford, E. J., and Canty-Laird, E. G. (2018). A comparison of the stem cell characteristics of murine tenocytes and tendon-derived stem cells. BMC Musculoskelet. Disord. 19 (1), 116. doi:10.1186/s12891-018-2038-2
Lee, S. Y., Kim, W., Lim, C., and Chung, S. G. (2015). Treatment of lateral epicondylosis by using allogeneic adipose-derived mesenchymal stem cells: a pilot study. Stem Cells 33 (10), 2995–3005. doi:10.1002/stem.2110
Leong, N. L., Kator, J. L., Clemens, T. L., James, A., Enamoto-Iwamoto, M., and Jiang, J. (2020). Tendon and ligament healing and current approaches to tendon and ligament regeneration. J. Orthop. Res. 38 (1), 7–12. doi:10.1002/jor.24475
Li, K., Deng, G., Deng, Y., Chen, S., Wu, H., Cheng, C., et al. (2019). High cholesterol inhibits tendon-related gene expressions in tendon-derived stem cells through reactive oxygen species-activated nuclear factor-κB signaling. J. Cell Physiol. 234 (10), 18017–18028. doi:10.1002/jcp.28433
Li, P., Xu, Y., Gan, Y., Song, L., Zhang, C., Wang, L., et al. (2016). Role of the ERK1/2 signaling pathway in osteogenesis of rat tendon-derived stem cells in normoxic and hypoxic cultures. Int. J. Med. Sci. 13 (8), 629–637. doi:10.7150/ijms.16045
Li, S., Sun, Y., Chen, Y., Lu, J., Jiang, G., Yu, K., et al. (2023). Sandwich biomimetic scaffold based tendon stem/progenitor cell alignment in a 3D microenvironment for functional tendon regeneration. ACS Appl. Mater Interfaces 15 (3), 4652–4667. doi:10.1021/acsami.2c16584
Li, Y., Wu, T., and Liu, S. (2021). Identification and distinction of tenocytes and tendon-derived stem cells. Front. Cell Dev. Biol. 9, 629515. doi:10.3389/fcell.2021.629515
Lim, W. L., Liau, L. L., Ng, M. H., Chowdhury, S. R., and Law, J. X. (2019). Current progress in tendon and ligament tissue engineering. Tissue Eng. Regen. Med. 16 (6), 549–571. doi:10.1007/s13770-019-00196-w
Lin, Y., Zhang, L., Liu, N. Q., Yao, Q., Van Handel, B., Xu, Y., et al. (2019). In vitro behavior of tendon stem/progenitor cells on bioactive electrospun nanofiber membranes for tendon-bone tissue engineering applications. Int. J. Nanomedicine 14, 5831–5848. doi:10.2147/ijn.s210509
Liu, C., Luo, J. W., Liang, T., Lin, L. X., Luo, Z. P., Zhuang, Y. Q., et al. (2018b). Matrix stiffness regulates the differentiation of tendon-derived stem cells through FAK-ERK1/2 activation. Exp. Cell Res. 373 (1-2), 62–70. doi:10.1016/j.yexcr.2018.08.023
Liu, Q., Zhu, Y., Amadio, P. C., Moran, S. L., Gingery, A., and Zhao, C. (2018a). Isolation and characterization of multipotent Turkey tendon-derived stem cells. Stem Cells Int. 2018, 3697971. doi:10.1155/2018/3697971
Liu, X., Chen, W., Zhou, Y., Tang, K., and Zhang, J. (2015). Mechanical tension promotes the osteogenic differentiation of rat tendon-derived stem cells through the wnt5a/wnt5b/JNK signaling pathway. Cell Physiol. Biochem. 36 (2), 517–530. doi:10.1159/000430117
Liu, Y., Xu, J., Xu, L., Wu, T., Sun, Y., Lee, Y. W., et al. (2017). Cystic fibrosis transmembrane conductance regulator mediates tenogenic differentiation of tendon-derived stem cells and tendon repair: accelerating tendon injury healing by intervening in its downstream signaling. Faseb J. 31 (9), 3800–3815. doi:10.1096/fj.201601181r
Liu, Y., Yuan, C., Zhou, M., and Tang, K. (2019). Co-Cultured bone-marrow derived and tendon stem cells: novel seed cells for bone regeneration. Open Life Sci. 14, 568–575. doi:10.1515/biol-2019-0063
Lu, K., Chen, X., Tang, H., Zhou, M., He, G., Liu, J., et al. (2020a). Bionic silk fibroin film induces morphological changes and differentiation of tendon stem/progenitor cells. Appl. Bionics Biomech. 2020, 8865841. doi:10.1155/2020/8865841
Lu, K., Chen, X., Tang, H., Zhou, M., He, G., Lu, Z., et al. (2020b). Bionic silk fibroin film promotes tenogenic differentiation of tendon stem/progenitor cells by activating focal adhesion kinase. Stem Cells Int. 2020, 1–10. doi:10.1155/2020/8857380
Lui, P. P., and Chan, K. M. (2011). Tendon-derived stem cells (TDSCs): from basic science to potential roles in tendon pathology and tissue engineering applications. Stem Cell Rev. Rep. 7 (4), 883–897. doi:10.1007/s12015-011-9276-0
Lui, P. P., Wong, O. T., and Lee, Y. W. (2014). Application of tendon-derived stem cell sheet for the promotion of graft healing in anterior cruciate ligament reconstruction. Am. J. Sports Med. 42 (3), 681–689. doi:10.1177/0363546513517539
Lui, P. P., Wong, O. T., and Lee, Y. W. (2016). Transplantation of tendon-derived stem cells pre-treated with connective tissue growth factor and ascorbic acid in vitro promoted better tendon repair in a patellar tendon window injury rat model. Cytotherapy 18 (1), 99–112. doi:10.1016/j.jcyt.2015.10.005
Mao, X., Yao, L., Li, M., Zhang, X., Weng, B., Zhu, W., et al. (2022). Enhancement of tendon repair using tendon-derived stem cells in small intestinal submucosa via M2 macrophage polarization. Cells 11 (17), 2770. doi:10.3390/cells11172770
Ni, M., Lui, P. P., Rui, Y. F., Lee, Y. W., Lee, Y. W., Tan, Q., et al. (2012). Tendon-derived stem cells (TDSCs) promote tendon repair in a rat patellar tendon window defect model. J. Orthop. Res. 30 (4), 613–619. doi:10.1002/jor.21559
Ni, M., Sun, W., Li, Y., Ding, L., Lin, W., Peng, H., et al. (2021). Sox11 modified tendon-derived stem cells promote the repair of osteonecrosis of femoral head. Cell Transpl. 30, 096368972110538. doi:10.1177/09636897211053870
Ni, Q., Zhu, J., Li, Z., Li, B., Wang, H., and Chen, L. (2023). Simvastatin promotes rat Achilles tendon-bone interface healing by promoting osteogenesis and chondrogenic differentiation of stem cells. Cell Tissue Res. 391 (2), 339–355. doi:10.1007/s00441-022-03714-w
Nichols, A. E. C., Best, K. T., and Loiselle, A. E. (2019). The cellular basis of fibrotic tendon healing: challenges and opportunities. Transl. Res. 209, 156–168. doi:10.1016/j.trsl.2019.02.002
Nie, D., Zhou, Y., Wang, W., Zhang, J., and Wang, J. H. (2021). Mechanical overloading induced-activation of mTOR signaling in tendon stem/progenitor cells contributes to tendinopathy development. Front. Cell Dev. Biol. 9, 687856. doi:10.3389/fcell.2021.687856
Ning, C., Gao, C., Li, P., Fu, L., Chen, W., Liao, Z., et al. (2022). Dual-phase aligned composite scaffolds loaded with tendon-derived stem cells for achilles tendon repair. Adv. Ther. 5. doi:10.1002/adtp.202200081
Ning, L. J., Zhang, Y. J., Zhang, Y. J., Zhu, M., Ding, W., Jiang, Y. L., et al. (2021). Enhancement of migration and tenogenic differentiation of Macaca mulatta tendon-derived stem cells by decellularized tendon hydrogel. Front. Cell Dev. Biol. 9, 651583. doi:10.3389/fcell.2021.651583
Popov, C., Burggraf, M., Kreja, L., Ignatius, A., Schieker, M., and Docheva, D. (2015b). Mechanical stimulation of human tendon stem/progenitor cells results in upregulation of matrix proteins, integrins and MMPs, and activation of p38 and ERK1/2 kinases. BMC Mol. Biol. 16, 6. doi:10.1186/s12867-015-0036-6
Popov, C., Kohler, J., and Docheva, D. (2015a). Activation of EphA4 and EphB2 reverse signaling restores the age-associated reduction of self-renewal, migration, and actin turnover in human tendon stem/progenitor cells. Front. Aging Neurosci. 7, 246. doi:10.3389/fnagi.2015.00246
Qiu, S., Sun, Y., Xu, J., Wen, G., Yu, Y., Wu, T., et al. (2019). Ferulic acid improves self-renewal and differentiation of human tendon-derived stem cells by upregulating early growth response 1 through hypoxia. Genesis 57 (9), e23291. doi:10.1002/dvg.23291
Rajpar, I., and Barrett, J. G. (2020). Multi-differentiation potential is necessary for optimal tenogenesis of tendon stem cells. Stem Cell Res. Ther. 11 (1), 152. doi:10.1186/s13287-020-01640-8
Randelli, P., Menon, A., Ragone, V., Creo, P., Alfieri Montrasio, U., Perucca, O. C., et al. (2016a). Effects of the pulsed electromagnetic field PST® on human tendon stem cells: a controlled laboratory study. BMC Complement. Altern. Med. 16, 293. doi:10.1186/s12906-016-1261-3
Randelli, P., Menon, A., Ragone, V., Creo, P., Bergante, S., Randelli, F., et al. (2016b). Limoges product treatment increases the proliferation rate of human tendon stem cells without affecting their stemness and differentiation capability. Stem Cells Int. 2016, 1–11. doi:10.1155/2016/4373410
Rodas, G., Soler-Rich, R., Rius-Tarruella, J., Alomar, X., Balius, R., Orozco, L., et al. (2021). Effect of autologous expanded bone marrow mesenchymal stem cells or leukocyte-poor platelet-rich plasma in chronic patellar tendinopathy (with gap >3 mm): preliminary outcomes after 6 Months of a double-blind, randomized, prospective study. Am. J. Sports Med. 49 (6), 1492–1504. doi:10.1177/0363546521998725
Rui, Y. F., Lui, P. P., Li, G., Fu, S. C., Lee, Y. W., and Chan, K. M. (2010). Isolation and characterization of multipotent rat tendon-derived stem cells. Tissue Eng. Part A 16 (5), 1549–1558. doi:10.1089/ten.tea.2009.0529
Rui, Y. F., Lui, P. P., Ni, M., Chan, L. S., Lee, Y. W., and Chan, K. M. (2011). Mechanical loading increased BMP-2 expression which promoted osteogenic differentiation of tendon-derived stem cells. J. Orthop. Res. 29 (3), 390–396. doi:10.1002/jor.21218
Ruiz-Alonso, S., Lafuente-Merchan, M., Ciriza, J., Saenz-Del-Burgo, L., and Pedraz, J. L. (2021). Tendon tissue engineering: cells, growth factors, scaffolds and production techniques. J. Control Release 333, 448–486. doi:10.1016/j.jconrel.2021.03.040
Sevivas, N., Teixeira, F. G., Portugal, R., Direito-Santos, B., Espregueira-Mendes, J., Oliveira, F. J., et al. (2018). Mesenchymal stem cell secretome improves tendon cell viability in vitro and tendon-bone healing in vivo when a tissue engineering strategy is used in a rat model of chronic massive rotator cuff tear. Am. J. Sports Med. 46 (2), 449–459. doi:10.1177/0363546517735850
Shen, W., Chen, J., Yin, Z., Chen, X., Liu, H., Heng, B. C., et al. (2012). Allogenous tendon stem/progenitor cells in silk scaffold for functional shoulder repair. Cell Transpl. 21 (5), 943–958. doi:10.3727/096368911x627453
Shi, Y., Fu, Y., Tong, W., Geng, Y., Lui, P. P., Tang, T., et al. (2012). Uniaxial mechanical tension promoted osteogenic differentiation of rat tendon-derived stem cells (rTDSCs) via the Wnt5a-RhoA pathway. J. Cell Biochem. 113 (10), 3133–3142. doi:10.1002/jcb.24190
Shi, Y., Zhou, K., Zhang, W., Zhang, Z., Zhou, G., Cao, Y., et al. (2017). Microgrooved topographical surface directs tenogenic lineage specific differentiation of mouse tendon derived stem cells. Biomed. Mater 12 (1), 015013. doi:10.1088/1748-605x/12/1/015013
Song, F., Jiang, D., Wang, T., Wang, Y., Chen, F., Xu, G., et al. (2017). Mechanical loading improves tendon-bone healing in a rabbit anterior cruciate ligament reconstruction model by promoting proliferation and matrix formation of mesenchymal stem cells and tendon cells. Cell Physiol. Biochem. 41 (3), 875–889. doi:10.1159/000460005
Song, H., Yin, Z., Wu, T., Li, Y., Luo, X., Xu, M., et al. (2018). Enhanced effect of tendon stem/progenitor cells combined with tendon-derived decellularized extracellular matrix on tendon regeneration. Cell Transpl. 27 (11), 1634–1643. doi:10.1177/0963689718805383
Stace, E. T., Nagra, N. S., Tiberwel, S., Khan, W., and Carr, A. J. (2018). The use of electrospun scaffolds in musculoskeletal tissue engineering: a focus on tendon and the rotator cuff. Curr. Stem Cell Res. Ther. 13 (8), 619–631. doi:10.2174/1574888x13666180129105707
Stein, B. E., Stroh, D. A., and Schon, L. C. (2015). Outcomes of acute Achilles tendon rupture repair with bone marrow aspirate concentrate augmentation. Int. Orthop. 39 (5), 901–905. doi:10.1007/s00264-015-2725-7
Subramanian, A., and Schilling, T. F. (2015). Tendon development and musculoskeletal assembly: emerging roles for the extracellular matrix. Development 142 (24), 4191–4204. doi:10.1242/dev.114777
Sun, L., Li, H., Qu, L., Zhu, R., Fan, X., Xue, Y., et al. (2014). Immobilized lentivirus vector on chondroitin sulfate-hyaluronate acid-silk fibroin hybrid scaffold for tissue-engineered ligament-bone junction. Biomed. Res. Int. 2014, 1–10. doi:10.1155/2014/816979
Tabesh, H., Elahi, Z., Amoabediny, Z., and Rafiei, F. (2022). Elimination of induced hypoxic regions in depth of 3D porous silk scaffolds by the introduction of channel configuration. Biomed. Res. Int. 2022, 1–12. doi:10.1155/2022/9767687
Tarafder, S., Brito, J. A., Minhas, S., Effiong, L., Thomopoulos, S., and Lee, C. H. (2019). In situ tissue engineering of the tendon-to-bone interface by endogenous stem/progenitor cells. Biofabrication 12 (1), 015008. doi:10.1088/1758-5090/ab48ca
Tevlin, R., Walmsley, G. G., Marecic, O., Hu, M. S., Wan, D. C., and Longaker, M. T. (2016). Stem and progenitor cells: advancing bone tissue engineering. Drug Deliv. Transl. Res. 6 (2), 159–173. doi:10.1007/s13346-015-0235-1
Thorpe, C. T., and Screen, H. R. (2016). Tendon structure and composition. Adv. Exp. Med. Biol. 920, 3–10. doi:10.1007/978-3-319-33943-6_1
Wang, C., Zhou, Z., Song, W., Cai, Z., Ding, Z., Chen, D., et al. (2022). Inhibition of IKKβ/NF-κB signaling facilitates tendinopathy healing by rejuvenating inflamm-aging induced tendon-derived stem/progenitor cell senescence. Mol. Ther. Nucleic Acids 27, 562–576. doi:10.1016/j.omtn.2021.12.026
Wang, H. N., Huang, Y. C., and Ni, G. X. (2020a). Mechanotransduction of stem cells for tendon repair. World J. Stem Cells 12 (9), 952–965. doi:10.4252/wjsc.v12.i9.952
Wang, T., Thien, C., Wang, C., Ni, M., Gao, J., Wang, A., et al. (2018). 3D uniaxial mechanical stimulation induces tenogenic differentiation of tendon-derived stem cells through a PI3K/AKT signaling pathway. Faseb J. 32 (9), 4804–4814. doi:10.1096/fj.201701384r
Wang, Y., He, G., Tang, H., Shi, Y., Zhu, M., Kang, X., et al. (2020b). Aspirin promotes tenogenic differentiation of tendon stem cells and facilitates tendinopathy healing through regulating the GDF7/Smad1/5 signaling pathway. J. Cell Physiol. 235 (5), 4778–4789. doi:10.1002/jcp.29355
Wang, Y., Li, M., Lin, Y., Yin, F., Shan, H., and Wu, T. (2023). Dimethyl oxalylglycine activates tendon-derived stem cells to promote regeneration of achilles tendon rupture in rats via HIF-1α. Adv. Ther. 6 (3), 2200164. doi:10.1002/adtp.202200164
Wei, B., Li, Z., Lin, Y., Hu, X., Xu, L., Wang, S., et al. (2023). BMP-2/TGF-β1 gene insertion into ligament-derived stem cells sheet promotes tendon-bone healing in a mouse. Biotechnol. J. 18 (5), e2200470. doi:10.1002/biot.202200470
Wei, B., and Lu, J. (2021). Characterization of tendon-derived stem cells and rescue tendon injury. Stem Cell Rev. Rep. 17 (5), 1534–1551. doi:10.1007/s12015-021-10143-9
Wu, T., Liu, Y., Wang, B., Sun, Y., Xu, J., Yuk-Wai, L. W., et al. (2016). The use of cocultured mesenchymal stem cells with tendon-derived stem cells as a better cell source for tendon repair. Tissue Eng. Part A 22 (19-20), 1229–1240. doi:10.1089/ten.tea.2016.0248
Xu, K., Lin, C., Ma, D., Chen, M., Zhou, X., He, Y., et al. (2021a). Spironolactone ameliorates senescence and calcification by modulating autophagy in rat tendon-derived stem cells via the NF-κB/MAPK pathway. Oxid. Med. Cell Longev. 2021, 1–15. doi:10.1155/2021/5519587
Xu, Y., Wang, Q., Li, Y., Gan, Y., Li, P., Li, S., et al. (2015). Cyclic tensile strain induces tenogenic differentiation of tendon-derived stem cells in bioreactor culture. Biomed. Res. Int. 2015, 1–13. doi:10.1155/2015/790804
Xu, Y., Yin, H., Chu, J., Eglin, D., Serra, T., and Docheva, D. (2021b). An anisotropic nanocomposite hydrogel guides aligned orientation and enhances tenogenesis of human tendon stem/progenitor cells. Biomater. Sci. 9 (4), 1237–1245. doi:10.1039/d0bm01127d
Yang, J., Zhao, Q., Wang, K., Liu, H., Ma, C., Huang, H., et al. (2016). Isolation and biological characterization of tendon-derived stem cells from fetal bovine. Vitro Cell Dev. Biol. Anim. 52 (8), 846–856. doi:10.1007/s11626-016-0043-z
Yang, Q., Li, J., Su, W., Yu, L., Li, T., Wang, Y., et al. (2022). Electrospun aligned poly(ε-caprolactone) nanofiber yarns guiding 3D organization of tendon stem/progenitor cells in tenogenic differentiation and tendon repair. Front. Bioeng. Biotechnol. 10, 960694. doi:10.3389/fbioe.2022.960694
Yang, Z., Cao, H., Gao, S., Yang, M., Lyu, J., and Tang, K. (2017). Effect of tendon stem cells in chitosan/β-glycerophosphate/collagen hydrogel on achilles tendon healing in a rat model. Med. Sci. Monit. 23, 4633–4643. doi:10.12659/msm.906747
Yao, S., Liang, Z., Lee, Y. W., Yung, P. S. H., and Lui, P. P. Y. (2023). Bioactive decellularized tendon-derived stem cell sheet for promoting graft healing after anterior cruciate ligament reconstruction. Am. J. Sports Med. 51 (1), 66–80. doi:10.1177/03635465221135770
Yin, H., Caceres, M. D., Yan, Z., Schieker, M., Nerlich, M., and Docheva, D. (2019). Tenomodulin regulates matrix remodeling of mouse tendon stem/progenitor cells in an ex vivo collagen I gel model. Biochem. Biophys. Res. Commun. 512 (4), 691–697. doi:10.1016/j.bbrc.2019.03.063
Yin, H., Strunz, F., Yan, Z., Lu, J., Brochhausen, C., Kiderlen, S., et al. (2020). Three-dimensional self-assembling nanofiber matrix rejuvenates aged/degenerative human tendon stem/progenitor cells. Biomaterials 236, 119802. doi:10.1016/j.biomaterials.2020.119802
Yin, H., Yan, Z., Bauer, R. J., Peng, J., Schieker, M., Nerlich, M., et al. (2018). Functionalized thermosensitive hydrogel combined with tendon stem/progenitor cells as injectable cell delivery carrier for tendon tissue engineering. Biomed. Mater 13 (3), 034107. doi:10.1088/1748-605x/aaadd1
Yin, Z., Chen, X., Chen, J. L., Shen, W. L., Hieu Nguyen, T. M., Gao, L., et al. (2010). The regulation of tendon stem cell differentiation by the alignment of nanofibers. Biomaterials 31 (8), 2163–2175. doi:10.1016/j.biomaterials.2009.11.083
Yin, Z., Chen, X., Zhu, T., Hu, J. J., Song, H. X., Shen, W. L., et al. (2013). The effect of decellularized matrices on human tendon stem/progenitor cell differentiation and tendon repair. Acta Biomater. 9 (12), 9317–9329. doi:10.1016/j.actbio.2013.07.022
Yin, Z., Sun, L., Shi, L., Nie, H., Dai, J., and Zhang, C. (2022). Bioinspired bimodal micro-nanofibrous scaffolds promote the tenogenic differentiation of tendon stem/progenitor cells for achilles tendon regeneration. Biomater. Sci. 10 (3), 753–769. doi:10.1039/d1bm01287h
Yu, Y., Lin, L., Zhou, Y., Lu, X., Shao, X., Lin, C., et al. (2017). Effect of hypoxia on self-renewal capacity and differentiation in human tendon-derived stem cells. Med. Sci. Monit. 23, 1334–1339. doi:10.12659/msm.903892
Zabrzyński, J., Łapaj, Ł., Paczesny, Ł., Zabrzyńska, A., and Grzanka, D. (2018). Tendon - function-related structure, simple healing process and mysterious ageing. Folia Morphol. Warsz. 77 (3), 416–427. doi:10.5603/fm.a2018.0006
Zhang, H., Dai, Y., Long, H., Cao, R., Shi, L., Zhao, J., et al. (2023). Tendon stem/progenitor cell-laden nanofiber hydrogel enhanced functional repair of patellar tendon. Tissue Eng. Part A 29 (5-6), 150–160. doi:10.1089/ten.tea.2022.0183
Zhang, J., Li, B., and Wang, J. H. (2011). The role of engineered tendon matrix in the stemness of tendon stem cells in vitro and the promotion of tendon-like tissue formation in vivo. Biomaterials 32 (29), 6972–6981. doi:10.1016/j.biomaterials.2011.05.088
Zhang, J., Nie, D., Williamson, K., McDowell, A., Hogan, M. V., and Wang, J. H. (2020). Moderate and intensive mechanical loading differentially modulate the phenotype of tendon stem/progenitor cells in vivo. PLoS One 15 (12), e0242640. doi:10.1371/journal.pone.0242640
Zhang, J., and Wang, J. H. (2010). Mechanobiological response of tendon stem cells: implications of tendon homeostasis and pathogenesis of tendinopathy. J. Orthop. Res. 28 (5), 639–643. doi:10.1002/jor.21046
Zhang, J., and Wang, J. H. (2013). Human tendon stem cells better maintain their stemness in hypoxic culture conditions. PLoS One 8 (4), e61424. doi:10.1371/journal.pone.0061424
Zhang, J., and Wang, J. H. (2014). Prostaglandin E2 (PGE2) exerts biphasic effects on human tendon stem cells. PLoS One 9 (2), e87706. doi:10.1371/journal.pone.0087706
Zhang, Q., and Cheng, B. (2013). Tendon-derived stem cells as a new cell source for tendon tissue engineering. Front. Biosci. (Landmark Ed. 18 (2), 756–764. doi:10.2741/4138
Zhang, Y., Lei, T., Tang, C., Chen, Y., Liao, Y., Ju, W., et al. (2021b). 3D printing of chemical-empowered tendon stem/progenitor cells for functional tissue repair. Biomaterials 271, 120722. doi:10.1016/j.biomaterials.2021.120722
Zhang, Y. J., Chen, X., Li, G., Chan, K. M., Heng, B. C., Yin, Z., et al. (2018). Concise review: stem cell fate guided by bioactive molecules for tendon regeneration. Stem Cells Transl. Med. 7 (5), 404–414. doi:10.1002/sctm.17-0206
Zhang, Z., Li, Y., Zhang, T., Shi, M., Song, X., Yang, S., et al. (2021a). Hepatocyte growth factor-induced tendon stem cell conditioned medium promotes healing of injured achilles tendon. Front. Cell Dev. Biol. 9, 654084. doi:10.3389/fcell.2021.654084
Zheng, Z., Ran, J., Chen, W., Hu, Y., Zhu, T., Chen, X., et al. (2017). Alignment of collagen fiber in knitted silk scaffold for functional massive rotator cuff repair. Acta Biomater. 51, 317–329. doi:10.1016/j.actbio.2017.01.041
Keywords: tendon-derived stem cell, tendon injury, tissue engineer, nanotechnology, seed cell
Citation: He W, Jiang C, Zhou P, Hu X, Gu X and Zhang S (2024) Role of tendon-derived stem cells in tendon and ligament repair: focus on tissue engineer. Front. Bioeng. Biotechnol. 12:1357696. doi: 10.3389/fbioe.2024.1357696
Received: 18 December 2023; Accepted: 29 July 2024;
Published: 08 August 2024.
Edited by:
Dai Fei Elmer Ker, The Chinese University of Hong Kong, ChinaReviewed by:
Takashi Taguchi, Western University of Health Sciences, United StatesCopyright © 2024 He, Jiang, Zhou, Hu, Gu and Zhang. This is an open-access article distributed under the terms of the Creative Commons Attribution License (CC BY). The use, distribution or reproduction in other forums is permitted, provided the original author(s) and the copyright owner(s) are credited and that the original publication in this journal is cited, in accordance with accepted academic practice. No use, distribution or reproduction is permitted which does not comply with these terms.
*Correspondence: SongOu Zhang, enNvMDEwQDE2My5jb20=
†These authors share first authorship
Disclaimer: All claims expressed in this article are solely those of the authors and do not necessarily represent those of their affiliated organizations, or those of the publisher, the editors and the reviewers. Any product that may be evaluated in this article or claim that may be made by its manufacturer is not guaranteed or endorsed by the publisher.
Research integrity at Frontiers
Learn more about the work of our research integrity team to safeguard the quality of each article we publish.