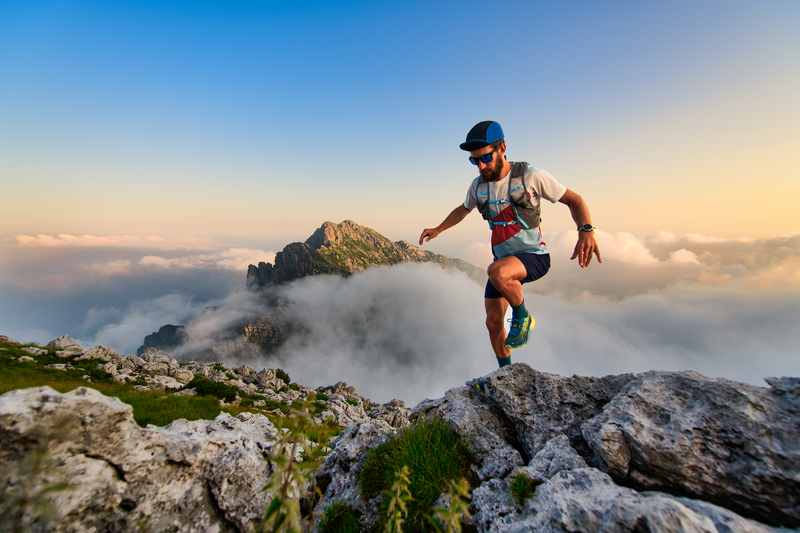
95% of researchers rate our articles as excellent or good
Learn more about the work of our research integrity team to safeguard the quality of each article we publish.
Find out more
ORIGINAL RESEARCH article
Front. Bioeng. Biotechnol. , 31 January 2024
Sec. Biomaterials
Volume 12 - 2024 | https://doi.org/10.3389/fbioe.2024.1355019
This article is part of the Research Topic Pharmaceutical Biomaterials View all 31 articles
Patients with osteoporosis often encounter clinical challenges of poor healing after bone transplantation due to their diminished bone formation capacity. The use of bone substitutes containing bioactive factors that increase the number and differentiation of osteoblasts is a strategy to improve poor bone healing. In this study, we developed an in situ dual-drug delivery system containing the bone growth factors PTH1-34 and simvastatin to increase the number and differentiation of osteoblasts for osteoporotic bone regeneration. Our system exhibited ideal physical properties similar to those of natural bone and allowed for customizations in shape through a 3D-printed scaffold and GelMA. The composite system regulated the sustained release of PTH1-34 and simvastatin, and exhibited good biocompatibility. Cell studies revealed that the composite system reduced osteoblast death, and promoted expression of osteoblast differentiation markers. Additionally, by radiographic analysis and histological observation, the dual-drug composite system demonstrated promising bone regeneration outcomes in an osteoporotic skull defect model. In summary, this composite delivery system, comprising dual-drug administration, holds considerable potential for bone repair and may serve as a safe and efficacious therapeutic approach for addressing bone defects in patients with osteoporosis.
Osteoporosis is one of the most widespread metabolic bone diseases worldwide. It has been reported that the number of individuals with osteoporosis exceeds 200 million, and this number is still rising in recent decades (Langdahl, 2021; Khandelwal and Lane, 2023). Osteoporosis is characterized by declined bone density, sparse bone trabeculae, and degenerative bone microarchitecture, which often lead to bone fractures and defects (Zhang H. et al., 2022). Bone substitutes or bone implants are often used for bone defects, but patients with osteoporosis face challenges in osseointegration due to reduced osteoblastic activity (Chandran and John, 2019). It is therefore crucial to consider these limitations when treating patients to achieve optimal outcomes.
In response to the difficulties posed by osteoporotic bone defects, a “Three in One” strategy has been proposed by researchers. The strategy includes proper bone grafting, anti-osteoporosis treatment, and subsequent bone regeneration promotion (Chen et al., 2022). Traditional bone grafts include autogenous bone, allogeneic bone, and xenografts, but supply constraints and potential immunological reactions remain substantial obstacles (Jin et al., 2021; Zhao et al., 2021). Advances in tissue engineering via biomaterial carriers have led to an opportunity to achieve tissue restoration and to deliver biological factors locally (Martin and Bettencourt, 2018; Wani et al., 2021). Methacrylate gelatin (GelMA), a hydrophilic polymeric network hydrogel, is a popular drug delivery vector and cell culture matrix due to its excellent physiological function, high water content, and controlled physicochemical properties (Sun et al., 2018; Wang F. et al., 2021). However, the mechanical characteristics of the hydrogels are significantly inferior to those exhibited by bone tissue, and thus, these hydrogels may provide inadequate support for subsequent bone tissue growth. Polylactic acid (PLA) is another degradable polymer which has received Food and Drug Administration (FDA) approval for osteoconductive scaffolds, and it is characterized by the exceptional mechanical strength similar to that of bone tissue (Barbeck et al., 2017; Grémare et al., 2018; Tilkin et al., 2020; Anita Lett et al., 2021; Xu et al., 2022). In addition, the 3D-printing properties of PLA make it particularly suitable for creating custom-fit scaffolds that match irregularly-shaped bone defects (Han et al., 2020).
Parathyroid hormone (PTH)1-34, a growth factor approved by the FDA as an anti-osteoporotic drug, is used to promote bone anabolism for the clinical treatment of osteoporosis (Hauser et al., 2021). Previous studies have suggested that PTH increases the number of osteoblasts by reducing apoptosis of these cells and exerts positive effects on bone (Silva and Bilezikian, 2015; Xie et al., 2017; Jiang et al., 2018; Chen et al., 2021; Rocha et al., 2021). Bone formation is the result of a rise in the quantity and differentiation of osteoblasts. As such, the administration of multiple bioactive factors may achieve the desired results within the skeletal environment (Wani et al., 2021). Simvastatin (SV) has demonstrated the ability to improve osteogenic differentiation by improving alveolar bone recovery, implant osseointegration, and bone tissue regeneration in various studies (Wang et al., 2018; Xu et al., 2018; Delan et al., 2020; Dang et al., 2021; Xu et al., 2021; Zhu et al., 2021). The systemic administration of PTH and SV produces a more significant osteogenic effect than either treatment alone (Jeon and Puleo, 2008; Tao et al., 2015; Tao et al., 2016). However, it is important to consider that systemic administration can result in high doses and low bioavailability, which may lead to potential toxicity and increased costs (Meng et al., 2022). Alternative approaches to drug administration may be needed to achieve more favorable outcomes.
The background provided indicates that a personalized dual delivery system consisting of bioactive PTH1-34 and SV should be considered when addressing osteoporotic bone defects. Therefore, we hypothesized that a composite PLA and GelMA scaffold loaded with PTH1-34 and SV to promote higher osteoblast numbers and differentiation could be employed as a bioactive bone scaffold to address osteoporotic bone defects (see Figure 1). In this study, a composite system composed of a 3D bioprinted PLA scaffold and GelMA was utilized to replicate the physical properties and personalized morphology of bone defects while concurrently controlling the release of osteoinductive factors. PTH1-34 was packed in poly (lactic-co-glycolic acid) (PLGA) microspheres to prevent early exposure to body fluids and to mitigate its short half-life. We assessed the osteogenic potential of the engineered delivery system in MC3T3-E1 cells in vitro as well as in osteoporotic rat cranial defects in vivo. Our results indicate that the dual release of SV and PTH1-34 from the delivery system upregulates osteogenic genes and proteins, thereby accelerating the healing process of osteoporotic bone defects. Overall, these findings highlight the potential effectiveness of the personalized biomimetic dual delivery system for osteoporotic bone repair.
FIGURE 1. Schematic of a composite system for in situ osteoporotic bone repair. A dual-drug delivery system was composed of PTH@PLGA microspheres and SV, which were encapsulated in a GelMA hydrogel and enhanced by a 3D-printed PLA scaffold for compressive strength. The delivery system placed in the osteoporotic cranial defect controlled the release of PTH and SV, enhanced osteoblast marker (ALP, BMP2, COL1, OCN) expression, and improved bone regeneration.
Medical-grade PLGA and PLA polymers were obtained from Daigang Bioengineering Co., Ltd. (Jinan, China). GelMA was obtained from Suzhou Yongqinquan Intelligent Equipment Co., Ltd. (Suzhou, China). SV was obtained from Macklin Biochemical Technology Co., Ltd. (Shanghai, China), and PTH1-34 was obtained from Psaitong Biotechnology Co. Ltd. (Beijing, China). The MC3T3-E1 cell line was a gift from Jilin University Stomatology Hospital. qRT-PCR related reagents were purchased from Yeasen Biotech (Shanghai, China). Antibodies were purchased from Proteintech or Servicebio Biotechnology Co., Ltd. (Wuhan, China). Other reagents were obtained from Solarbio (Beijing, China) or Beyotime (Shanghai, China).
A multiple emulsion method was used to prepare PTH1-34@PLGA microspheres as previously reported (Eswaramoorthy et al., 2012). Briefly, 500 μL of 100 μM PTH1-34 solution was emulsified in 5 mL dichloromethane PLGA solution, with a power of 15 W in an ice bath form a water-in-oil (w/o) emulsion. With vigorous stirring, the w/o emulsion was gradually added to 40 mL of 1% PVA aqueous solution to form a w/o/w solution. The solution was stirred overnight at room temperature to evaporate dichloromethane.
For the encapsulation efficiency, 10 mg PTH1-34@PLGA microspheres was added into a mixture of 0.9% NaCl and dichloromethane at room temperature to release PTH1-34 in the microspheres. The supernatant was collected by centrifugation to measure the concentration of PTH1-34. Encapsulation efficiency is determined by the following Formula (1):
A 3D bioprinter (Sunp Biomaker Pro series, Sunp Biotech, Beijing, China) was employed to produce PLA scaffolds using a fused deposition technique. Cuboid models 10 mm × 10 mm in size were used for in vitro characterization and cell culture, while cylindrical models with a diameter of φ8 mm were used for animal models. A 10% (w/v) solution of GelMA and a 0.1% (w/v) solution of photoinitiator were dissolved according to the guidelines provided by the manufacturer, and then passed through a filter membrane. 0.4 mg PTH1-34@PLGA microspheres and 0.1 mg/mL SV were added into GelMA solution at 37°C, and the prepared mixture was crosslinked under 405 nm UV light after injection into the PLA scaffold. Finally, the dual-drug delivery system was obtained and kept at 4°C before being used.
All samples were lyophilized and observed with a scanning electron microscope (JSM-IT500A) after gold spraying. The size of the microporous structure was measured using ImageJ.
Porosity was determined using the ethanol immersion method (Arumugam et al., 2020). Briefly, the initial mass of the dried samples was first measured as Wi, and then the sample was immersed in ethanol medium and vacuumed. The final weight was quickly measured and recorded as We. The porosity was performed based on the following Formula (2):
where V is the total volume of the sample, and ρ is the specific mass of ethanol at atmospheric temperature, 0.789 g/mL.
The water absorption was measured based on the ASTM standard D570 (Alam et al., 2020). The initial weight of the sample was first recorded as W0, and then the sample was submerged in neutral PBS at 37°C (Wu et al., 2020). After a 24-h immersion period, the sample was removed from the PBS, and the weight was recorded as W1. The water absorption rate of the sample was performed according to the subsequent Formula (3):
The sample was weighed as W0 and then transferred into neutral PBS at 37°C. A pH meter (INESA, China) was used to measure the pH around the samples. The remaining weight of the sample at a predetermined time was Wt. The weight loss was determined according to the subsequent Formula (4):
A universal mechanical testing machine (AG-XPLUS10KN, Shimadzu, Japan) was employed to test the mechanical properties. Compression properties were examined with a speed of 1 mm/min. Stress‒strain curves corresponding to strains of 0%–10% were used to calculate the compression modulus.
The samples were immersed in neutral PBS and underwent incubation at a temperature of 37°C. The solution was obtained at the predetermined time, and the absorbance values at 238 nm (SV) and 280 nm (PTH1-34) were measured by a spectrophotometer (UV-2100, Unico, China) (Yuan et al., 2022). The cumulative drug release was plotted in a line graph.
Blood hemocompatibility was assessed using methods described in previous studies (Ma et al., 2018). Samples incubated in PBS at 37°C, diluted with 1:1.25 fresh rat blood and re-incubated for an hour before centrifuged. Supernatant absorbance at 545 nm was measured. PBS and distilled water served as negative and positive controls, respectively. The hemolysis rate (HR) was determined according to the subsequent Formula (5):
where At, Anc, and Apc denote the absorbances of test, negative, and positive controls.
Cranial-derived preosteoblastic MC3T3-E1 cells were cultivated in an incubator consisting of a 37°C environment supplemented with 5% CO2. α-MEM containing 10% fetal bovine serum and 1% penicillin‒streptomycin was used. The cells were seeded in 96-well plates and cultured with PTH1-34 or SV containing culture medium. After incubation for 1, 3, and 5 days, 100 μL diluted CCK-8 solution was added, and OD at 450 nm was measured.
MC3T3-E1 cells were cultivated in 24-well plates for 48 h, and the cells were visualized by calcein-AM (1:1000) and PI (1:500) according to the assay instructions. Images were obtained using fluorescence microscopy (Olympus, Japan). The percentage of live/dead cells was counted using ImageJ.
MC3T3-E1 cells were cultured in osteogenic medium containing 10 mM disodium β-glycerophosphate, 50 μg/ml L-ascorbic acid, and 10−7 M dexamethasone. Cells were fixed in 4% paraformaldehyde before ALP and AR staining performed. Calcium nodules were lysed in 10% (w/v) cetylpyridinium chloride for quantification, and the OD values were measured at 562 nm (Fang et al., 2016).
Sterilized samples were wetted with culture medium overnight and then seeded with a density of 1500 cells/mm3 (Han et al., 2020; Wu et al., 2020). After coincubation for 48 h, the cells were fixed and lysed by 0.1% Triton-X. Cells were detected using fluorescence microscopy after incubation with phalloidin and DAPI in the dark.
Total cellular RNA was obtained using TRIzol, and RNA purity was determined using a Nanodrop 2000C. RNA was reverse transcribed, and cDNA amplification was performed using a CFX Connect Real Time PCR Detection System (Bio-Rad, Singapore). Supplementary Table S1 showed the primer sequences. The 2−ΔΔCt method was utilized to analyze gene expression levels.
Cells were lysed in RIPA buffer on ice and quantified by BCA method. The protein samples with 5× SDS loading buffer were loaded into the wells of the prepared SDS‒PAGE gel. Electrophoresis was performed at 80 V (for the concentrated gel) and 120 V (for the separated gel) until bromophenol blue reached the bottom of the gel. Proteins were transferred to a PVDF membrane, and then the membranes were blocked and incubated with primary and secondary antibodies. The ECL method was used for protein detection. ImageJ software was used to quantify the grayscale values.
All animals in this experiment were purchased from Liaoning Changsheng Experimental Animal Company, and all animal work was approved by the Institutional Animal Care and Use Committee of Jilin University School of Pharmaceutical Science (No. 20220034). Twenty-four 12-week-old SD rats (female, 250–280 g) were randomly divided into the sham-operated group (n = 3) and the OVX group (n = 21). All OVX rats underwent bilateral ovariectomy after anesthesia (intraperitoneal injection of pentobarbital sodium, 45 mg/kg), while a comparable mass of adipose tissue surrounding the ovary was cut off in the sham-operated group. Three months after OVX surgery, three rats in each group were selected, and the distal femurs were harvested for micro-CT examination. The remaining rats were assigned to 3 groups: (1) Con group: OVX + nondrug-loaded scaffold group (n = 6); (2) PTH group: OVX + PTH1-34 group (n = 6); and (3) PTH + SV group: OVX + PTH1-34 + SV group (n = 6). By a dental trephine, an 8 mm full-thickness circular defect was created on the midline of the rat’s parietal bone under anesthesia. After the delivery system was placed in the bony defect, rats were given penicillin postoperatively for 3 days. Rats were sacrificed by cardiac perfusion under anesthesia at 4 or 8 weeks.
To assess the extent of bone repair, X-ray (Faxitron MultiFocus, United States) and micro-CT (Scanco, Switzerland) imaging was performed. The specimens obtained were placed in 4% paraformaldehyde before X-ray and micro-CT analysis. Each planar radiography was scored according to a previous description (Spicer et al., 2012). Using micro-CT at a resolution of 34 μm, regions of interest (ROIs) were selected, and bone volume fraction (BV/TV), bone volume (BV), bone surface area (BS), trabecular number (Tb. N), trabecular thickness (Tb. Th) and trabecular separation (Tb. Sp) were determined.
Rat skull specimens were decalcified and sectioned for HE, Masson’s Trichrome, and immunohistochemical (IHC) staining. Primary antibodies against ALP (1:200, 13365-1-AP, ProteinTech, Wuhan, China), BMP2 (1:200, 18933-1-AP, ProteinTech, Wuhan, China), COL1 (1:800, GB11022-3, Servicebio, Wuhan, China), OCN (1:200, 23418-1-AP, ProteinTech, Wuhan, China), and HRP-labeled secondary antibody (1:200, 5220-0336, SeraCare, Beijing, China) were used. Viscera were harvested to observe structural changes by HE staining. The sections were observed using a photomicroscope (OLYMPUS, BX53).
All data are expressed as the mean ± standard. A minimum of 3 parallel samples were tested at each time point. Statistical analysis was performed using GraphPad Prism 8.0 software, utilizing Student’s t-test or analysis of variance (ANOVA) as appropriate. For data with unequal variances or nonnormal distributions, Welch’s t-test or the Kruskal‒Wallis nonparametric test was employed. Statistical significance was assigned as * (p < 0.05), ** (p < 0.01) or *** (p < 0.001), respectively.
We followed the procedure illustrated in Figure 2A to prepare the delivery systems. The photo of PLGA microspheres is shown in Supplementary Figure S1, and the encapsulation efficiency is 72.07% ± 1.89%. Different printing line distances on pore size and fiber size of PLA scaffolds were investigated, as shown in Supplementary Figure S2, and a printing line distance of 1.0 mm was finally selected for subsequent experiments. The gelation process of GelMA is shown in Supplementary Figure S3. The macroscopic and microscopic images of the PLA scaffold, GelMA delivery system, and PLA-GelMA system are shown in Figure 2B. All samples maintained their preset sizes. SEM revealed that the pore size in the 3D-printed PLA scaffold was approximately 500 μm, which was similar to that of natural cancellous bone (300–600 μm) (Anita Lett et al., 2021). The GelMA delivery system demonstrated microporous pores. For the PLA—GelMA composite delivery system, the microporous pores of the GelMA delivery system filled the macroporous pores of the PLA scaffold, which resulted in a close embedding relationship.
FIGURE 2. Synthesis and characterization of dual drug delivery systems. (A) Schematic diagram of the preparation process of the dual-drug delivery system. (B) Macroscopic digital photographs and microscopic morphology of the PLA, GelMA, and PLA-GelMA delivery systems under scanning electron microscopy. The (C) porosity rate, (D) water absorption rate, (E) compression modulus, (F) stress‒strain curve, (G) degradation weight loss, and (H) PTH1-34/SV cumulative release curves are shown. * and *** representing p < 0.05 and p < 0.001 respectively.
In our experiments, the porosity rates of the PLA scaffolds, the GelMA delivery system, and the PLA-GelMA system were 51.74% ± 1.74%, 59.05% ± 3.41%, and 52.82% ± 3.77%, respectively, and no notable disparities were identified among the groups (Figure 2C). The average water absorption rate of the GelMA delivery system was 1242.18% ± 85.65%, while that of PLA was 36.27% ± 4.77%. When the hydrogel was combined with the PLA scaffold, the water absorption rate decreased to 255.88% ± 24.67% (Figure 2D). The loading‒displacement curve and the stress‒strain curve showed that the stress of GelMA was always maintained at a low level, whereas the stress of PLA and PLA-GelMA increased with compression (Figure 2F;Supplementary Figure S4). The compressive modulus data of the GelMA hydrogel, the PLA scaffold, and the PLA-GelMA composite system were 17.377 ± 1.953 kPa, 3.032 ± 0.751 MPa, and 39.318 ± 6.549 MPa, respectively (Figure 2E). This result showed that the incorporation of PLA enhanced the ability of the delivery system to withstand mechanical.
We investigated the degradation characteristics of the three systems in PBS (Figure 2G). Our results showed that all three systems exhibited continuous degradation over time, with the GelMA system showing the fastest degradation rate and reaching complete degradation within 3 weeks. The PLA scaffold had a slower degradation rate and reached a weight loss rate of 34.94% ± 6.04% over 8 weeks. The degradation rate was found to be slow in the initial 3 weeks, but increased in the later stages. The PLA-GelMA system degraded quickly in the first 2 weeks, which was followed by a slower degradation rate in the remaining weeks. In addition, the pH values of all three delivery systems were maintained at physiological levels between 6.8 and 7.3 during degradation (Supplementary Figure S5).
100 ng/mL PTH and 0.2 μg/mL SV were selected for our experiments as a result of previous tests (Supplementary Figure S6). The cumulative release amount of SV and PTH1-34 in the composite system is displayed in Figure 2H. The initial release rate of SV was 6.29% ± 2.88%, followed by a gradual decline until day 19. The cumulative release of SV barely increased after 19 days, which was most likely due to complete degradation of the hydrogel. On the contrary, the initial release of PTH1-34 was not obvious (3.46% ± 0.08%), which may be because PTH1-34 was pre-encapsulated in PLGA microspheres. In addition, the cumulative release of PTH1-34 achieved a zenith around day 40. The release results showed that the composite system could allow a prolonged release of the drugs and could successfully deliver both PTH1-34 and SV in vitro, which was favorable for bone healing in a pathological environment.
To investigate the effectiveness of composite delivery systems containing PTH1-34 and/or SV, studies on the survival and differentiation status of MC3T3-E1 osteoblasts were conducted. Live/dead staining data indicated that the SV group and the control group had similar levels of dead cells (red fluorescence), as shown in Figures 3A, B. However, the PTH and PTH + SV groups showed a lower rate of cell death. Overall, all the mortality rates were far less than 30%, which indicated no cytotoxicity according to GB/T 16886.5-2017. To further investigate the combined effect of PTH1-34 and SV on osteogenic differentiation, we performed ALP staining and mineralized nodule staining (Figures 3C, D). Seven and 14 days of osteogenic induction in both the PTH group and the SV group resulted in darker ALP staining, while the darkest staining was found in the PTH + SV group. AR staining revealed enhanced mineralization in all three experimental groups, and the PTH + SV group showed the most obvious mineralization.
FIGURE 3. Effect of dual administration of PTH1-34 and SV on the survival and differentiation of MC3T3-E1 cells. (A) Live/dead staining images. The scale bar is 200 μm. (B) Quantitative analysis of the cell death ratio. (C) ALP and AR staining images. The scale bar is 500 μm. (D) Quantitative analysis of ALP activity and calcium nodules. *, **, and *** representing p < 0.05, p < 0.01, and p < 0.001, respectively.
The adhesive behaviors of the MC3T3-E1 cells cocultured on different composite systems were observed by examining fibrillar actin and nuclei. The results of rhodamine-phalloidin and DAPI staining showed that MC3T3-E1 cells were able to grow on all composite delivery systems (Figure 4A). The cells had a shuttle shape, with cell protrusions interconnected with each other in a reticular pattern. The counts of red-stained actin and blue-stained nuclei were observed to be elevated in the PTH-containing groups. The qRT-PCR analysis indicated that Alp, Bmp2, Col1, and Ocn genes were upregulated in both the PTH group and the SV group, while these genes were expressed at the highest levels in the PTH + SV group (Figure 4B). Consistent with the trend of the PCR assay results, the expression of related osteogenic proteins was also significantly upregulated after PTH and SV co-loading (Figures 4C, D). The above results indicated that the dual-drug strategy of PTH1-34 and SV from the composite delivery system could exert osteogenic effects in vitro and that the comedication therapeutic effect was better than that of single-drug treatment.
FIGURE 4. Effect of different delivery systems on adhesion and osteogenic marker expression in MC3T3-E1 cells. (A) Fluorescence images of MC3T3-E1 cells cultured on different systems (red: F-actin; blue: nucleus). The scale bar indicates 500 μm. (B) Osteogenic gene expression levels in MC3T3-E1 cells. (C) Osteogenic protein expression levels and (D) quantitative analysis. ** and *** representing p < 0.01 and p < 0.001 respectively.
Osteoporotic bone defects are usually difficult to repair, therefore, we established an osteoporotic model to evaluate the ability of the dual-drug composite delivery system to repair osteoporotic bone defects. The hemolysis rate was below 2% (Supplementary Figure S7), which is considered biosafety for implantation in vivo according to ASTM standards (Anita Lett et al., 2021). The entire animal experimental schedule is summarized in Figure 5A, and the surgical procedure is shown in Supplementary Figures S8, S11. In OVX rats, the volume and density of bone trabeculae in the distal femur were decreased, and the bone marrow cavity was expanded (Figure 5B). Quantitative analysis revealed that BV, BV/TV, and Tb. N values demonstrated reductions compared to the sham group, whereas Tb. Sp displayed an elevation in the epiphysis (Figure 5C). Furthermore, the body weight was increased (Supplementary Figure S9), and the uterus was atrophied (Supplementary Figure S10) in OVX rats. The above results demonstrated the successful establishment of the osteoporosis model.
FIGURE 5. The osteogenic capacity of the dual-drug delivery systems in vivo. (A) Illustration of the schedule of the animal experimental procedure. (B) Micro-CT snapshots of the distal femur of sham and OVX rats and (C) analysis results. (D) Radiographs and (E) micro-CT 3D-reconstructed images of the skull defect after 4 and 8 weeks. (F) Planar radiographic score of the skull and (G) quantitative micro-CT analysis. The scale bars are 5 mm *, **, and *** representing p < 0.05, p < 0.01, and p < 0.001, respectively.
4 and 8 weeks after the delivery system was implanted, all the scaffolds remained at the defect without detachment. X-ray images revealed varying degrees of novel bone in the rat skull in both the PTH group and the PTH + SV group, whereas minimal novel bone was found in the control group (Figure 5D). Both the PTH groups exhibited improved planar radiography scores after 4 and 8 weeks, although the difference was not statistically significant (Figure 5F). As shown in the micro-CT 3D-reconstructed images, almost no increase was observed in the defects in the control group, while island-like and irregular new bone were seen in the other two groups (Figure 5E). BV/TV, BV, BS, and Tb. N values increased in the PTH + SV group, and Tb. Sp values decreased at 4 and 8 weeks. However, no differences were observed in Tb. Th among the three groups (Figure 5G).
In the histological observation by HE staining (Figures 6A, C), the defect was replenished with a fibrous layer of sparse reticular fibrous tissue in the control group. Conversely, several islands of bone-like structures were visible in the reticular fibrous tissue in the PTH group, and osteoblasts and osteocytes could be seen. More novel bone was observed to connect to the host bone or through the material and connective tissue in the PTH + SV group, which resulted in a significantly larger area of new bone and more osteoblasts. By 8 weeks, all groups showed similar growth trends but exhibited greater bone mass and less residual material compared with groups at 4 weeks. Masson’s trichrome staining (Figures 6B, D) demonstrated that the defects consisted of sparse and disordered collagen in the control group, whereas the collagen was denser and more orderly in the PTH and PTH + SV groups. IHC staining (Figure 7) showed that a greater extent of positive staining for ALP, BMP2, COL1, and OCN in the PTH group, and this increase in positive staining was particularly noticeable in the PTH + SV group.
FIGURE 6. Histological photographs of the skull defect at 4 and 8 weeks. (A) HE staining. The black arrow indicates osteoblasts, and the blue arrow indicates osteocytes. (B) Masson’s trichrome staining. The red arrow indicates collagen fibers. (C) New bone area fraction and (D) blue staining area fraction. The scale bars at low magnification (×1) and high magnification (×40) are 1 mm and 50 μm, respectively. HB: host bone, NB: new bone, RM: remaining materials. * and ** representing p < 0.05 and p < 0.01 respectively.
FIGURE 7. IHC staining at 4 and 8 weeks. (A) Expression of ALP, BMP2, COL1, and OCN proteins in rat cranial bone. The scale bar is 50 μm. (B) Quantitative analysis of IHC staining. NB: new bone, RM: remaining materials. *, **, and *** representing p < 0.05, p < 0.01, and p < 0.001, respectively.
Finally, we conducted a toxicological evaluation of the dual-drug composite delivery system (Supplementary Figure S12). The sections of the viscera from rats who received the delivery system revealed no significant abnormalities, which indicated that the dual-drug composite delivery system does not induce any toxic reactions.
Osteoporosis is a prevalent metabolic bone disorders that affects a significant number of individuals worldwide, resulting in pain, fractures, and substantial annual costs. Worse still, impaired bone regeneration also hinders the repair process after trauma or infection. Therefore, a functional composite system carrying bioactive factors or drugs would provide a good repair strategy for difficult-to-heal bone defects.
Ideal osteogenic biomaterials must possess several characteristics, such as biocompatibility, suitable shape and porosity, matched mechanical force, osteoconductivity, osteoinductivity, and degradability (Wang S. et al., 2021; Sang et al., 2022; Xue et al., 2022). Additionally, a delivery system that serves as a local platform to sustainably release active substances should be considered. GelMA is an ideal platform for controlled drug release and cell adhesion (Sun et al., 2018; Collins et al., 2021; Kimicata et al., 2021). The hydrogels establish a biomimetic microenvironment for bone tissue engineering (Meng et al., 2022; Xue et al., 2022), but their biomechanical properties are slightly inadequate compared with those of hard bone tissue. To reinforce the mechanical strength of GelMA, secondary structural scaffolds are typically incorporated into the hydrogel matrix (Boere et al., 2014). Here, we employed PLA as the secondary scaffold due to its strength properties and 3D printable properties, and the combined design of polymer and hydrogel provides a biomimetic organoid for bone regeneration (Xue et al., 2022). Our composite drug delivery system achieved satisfactory results in terms of physical properties. First, personalized irregular and regular tissue defects can be perfectly replicated using a 3D-printed scaffold and a light-curing hydrogel, both of which can combine efficiently. Second, the physical parameters of the delivery system, such as porosity and degradability, are comparable to those of natural bone, and similar physical properties will be conducive to subsequent cell behavior and nutrient exchange in the environment. The porosity of the delivery systems ranged from 51.74% to 59.05%, which is consistent with the porosity of cancellous bone reported in the literature (Collins et al., 2021). We also found a high absorption rate in GelMA and a low absorption rate in the PLA scaffold, while a moderate water absorption rate was found in the PLA- GelMA system. We speculated that this result was related to the incorporation of the internal hydrophobic PLA scaffold, which acted as a “cage” to confine the swelling of the GelMA hydrogel. Therefore, the composite system could absorb exudate and nutrients while maintaining its macroscopic morphology and porosity. The appropriate degradation time of scaffolds contributes to the stability of the bone healing space in the early stage as well as the replacement of bone tissue in the later stage. We observed accelerated degradation during the later stages of GelMA and PLA, which could be because the eroded material created larger pores and increased the contact area over time. GelMA degrades rapidly within 1 month (Wang F. et al., 2021), whereas PLA degrades in 70 days- 5.5 years (Saini et al., 2016; Gasparotto et al., 2022). Therefore, the PLA-GelMA system demonstrated a marked initial degradation but subsequently decelerated in the later stage of degradation due to GelMA depletion. The advantage of this is that even if GelMA degrades rapidly in the first stage, the presence of PLA in the composite system can still provide ongoing support for new bone growth. Third, the mechanical characters of hydrogels (∼5–110 kPa) are generally inferior to those exhibited by natural bone (∼0.2–80 MPa) (Zhao et al., 2016; Zhou et al., 2021), thereby augmenting a risk of material collapse during subsequent tissue regeneration. A 3D-printed PLA scaffold structure can improve the support of biological cue-rich hydrogels (Han et al., 2020; Wang et al., 2022). That is, the PLA-GelMA composite delivery system in this study integrated the characteristics of PLA and GelMA and met the physicochemical requirements of bone scaffolds. In addition, drugs encapsulated in the GelMA hydrogel could achieve sustained release, which can provide continuous stimulation for subsequent treatment.
During bone formation, osteoblasts undergo four stages: proliferation, extracellular matrix maturation, mineralization, and osteoblast apoptosis. The increased number of osteoblasts and their degree of differentiation contribute to the final structure and quality of bone tissue. PTH1-34 has been approved by the FDA as a clinical drug for the treatment of osteoporosis. PTH1-34 interacts with PTH1R on the surface of osteoblasts and osteocytes and contributes to expanding the number of osteoblasts by reducing apoptosis and exerting an anabolic effect on bone (Silva and Bilezikian, 2015; Chen et al., 2021). Previous studies have used PTH in combination with bisphosphonates to achieve better osteogenesis and reduce the amount of PTH in the treatment of osteoporosis (Zhang and Song, 2020; Zhou et al., 2022). However, one risk of bisphosphonates is medication-related osteonecrosis of the jaw (Pires et al., 2005). Statins have a similar effect to bisphosphonates in inhibiting the mevalonate pathway, but carry no risk of osteonecrosis and promote osteogenesis through multiple signaling pathways (Xia et al., 2018; Jin et al., 2021; Guo et al., 2022). Previous studies reported that the combined use of two drugs resulted in a greater osteogenic effect, but the drugs in these studies were administered systemically (Jeon and Puleo, 2008; Tao et al., 2015; Tao et al., 2016). In this investigation, we examined the combined influences of PTH and SV on osteoblasts and osteoporotic bone defects in situ via the PLA-GelMA composite delivery system. According to the cell viability assay and differentiation experiments, 100 ng/mL PTH1-34 and 0.2 μg/mL SV were demonstrated to be safe for osteoblasts and exerted superior osteogenic effects, as such, these concentrations were used in subsequent experiments. We further observed the biological synergistic effect of PTH1-34 and SV on osteoblasts using the composite delivery system. Our results suggested that the cell death rates in the PTH and PTH + SV groups were slightly reduced, while osteogenic differentiation was significantly improved in all experimental groups. Bone formation is highly correlated with the number of osteoblasts and their level of differentiation. The number of osteoblasts was expanded by PTH administration, which led to increased ALP expression and mineralization. In addition, SV promoted osteogenic differentiation, which further improved bone formation. For this reason, the PTH + SV group showed the most significant enhancement in ALP activity and calcium nodule staining.
Immunofluorescence staining showed that the cocultured MC3T3-E1 cells were able to adhere to all composite scaffolds, which is beneficial for subsequent bone formation. The PTH group and the PTH + SV group displayed higher cell counts, possibly related to the reduction in apoptosis induced by PTH administration (Silva and Bilezikian, 2015; Chen et al., 2021; Jin et al., 2021). We also conducted a study to examine the expression of osteogenesis-related genes and proteins, and the results were consistent with the ALP and AR staining mentioned above. Alp is a representative early osteogenic marker, while Ocn serves as a representative late osteogenic marker. Bmp2 induces bone formation and regeneration during early embryonic development, and Col1 is the predominant collagenous fiber in the bone matrix (Zhang Y. et al., 2022). According to our experimental data, the combination of PTH1-34 and SV upregulated osteogenic genes and proteins, which indicates that they contribute to early and late osteogenesis. Notably, a substantial rise in Bmp2 gene expression was observed in the SV group, while PTH expression was not significantly different. This phenomenon might be credited to the functional role of SV in eliciting osteogenic differentiation via enhanced BMP2 expression (Mundy et al., 1999; Kodach et al., 2011).
Using in vivo experiments, we first established an osteoporosis model in rats by performing bilateral ovariectomy. The decreased volume of femoral trabecular bone, along with the increased weight and atrophic uterus, confirmed the successful establishment of the model. An 8 mm rat cranial defect is defined as a typical critical bone defect (Spicer et al., 2012). Indeed, the control group in this study showed minimal new bone formation. Although the delivery system is theoretically osteoconductive, the impaired osteogenic capacity in osteoporotic conditions still prevents bone formation. When the anti-osteoporotic factors were loaded, a greater increase in new bone production was noted. Histological staining at 4 and 8 weeks revealed that the PTH + SV group exhibited the most extensive new bone area as well as the greatest collagen content. Additionally, this group also showcased the highest extent of immunopositive IHC staining for ALP, BMP2, COL1, and OCN, which concurred with the findings obtained from in vitro experimentation. Furthermore, the hemolysis test and HE staining of various organs revealed no obvious hemolysis reaction or tissue toxicity as a result of any of the composite systems, which demonstrates the biosafety of in vivo implantation.
In summary, this study aimed to design a personalized biomimetic local drug delivery system to repair bone defects in osteoporosis. For the first time, this novel approach applied PTH and SV in combination for in situ bone defects. The dual-drug delivery system can be summarized by the following characteristics. First, 3D-printed PLA scaffolds and injectable light-curing hydrogels could replicate irregular shapes to better conform to the unique profile of bone defects. Second, the composite delivery system has physical properties that match those of natural bone tissue and successfully achieved dual-controlled release of PTH1-34 and SV. Third, the cytological, radiological, and histological analyses of the drug delivery system demonstrated its safety and efficacy. The dual release of the active molecules PTH1-34 and SV synergistically enhanced the repair of osteoporotic defects, possibly through an increased cell counts and an enhanced differentiation of osteoblasts.
However, despite our promising results, we also acknowledge that our work has some limitations. The cranial bone defect did not fully recover within the 8-week observation period. Therefore, future investigations should consider additional factors, longer observation times, and additional underlying mechanisms. In addition, the concentrations of PTH1-34 and SV were screened separately through in vitro experiments, and an appropriate combination of the two drug doses might yield more optimized results. Nevertheless, these limitations do not undermine the potential of the dual-drug delivery system to promote bone regeneration through the release of PTH1-34 and SV. Consequently, this localized personalized biomimetic dual-drug composite delivery system presents an encouraging and safe approach for the medical therapeutics of bone defects in individuals with osteoporosis.
The original contributions presented in the study are included in the article/Supplementary Material, further inquiries can be directed to the corresponding authors.
The animal study was approved by the Institutional Animal Care and Use Committee of Jilin University School of Pharmaceutical Science. The study was conducted in accordance with the local legislation and institutional requirements.
TX: Data curation, Formal Analysis, Investigation, Writing–original draft. SG: Visualization, Writing–review and editing. NY: Methodology, Writing–review and editing. QZ: Writing–review and editing. YZ: Investigation, Writing–review and editing. TL: Methodology, Writing–review and editing. ZL: Funding acquisition, Project administration, Writing–review and editing. BH: Project administration, Resources, Writing–review and editing.
The author(s) declare financial support was received for the research, authorship, and/or publication of this article. This work was supported by the Jilin Province Development and Reform Commission (Grant No. 2023C043-3); Science and Technology Department of Jilin Province (Grant No. 20230204076YY and 20220401102YY); Changchun Science and Technology Bureau (Grant No. 21ZGY01).
Figure 1; Figure 2A; Figure 5A were partly generated from Figdraw (ID: WIRIW84164) or Servier Medical Art (http://smart.servier.com/), licensed under a Creative Common Attribution 3.0 Generic License. (https://creativecommons.org/licenses/by/3.0/).
The authors declare that the research was conducted in the absence of any commercial or financial relationships that could be construed as a potential conflict of interest.
All claims expressed in this article are solely those of the authors and do not necessarily represent those of their affiliated organizations, or those of the publisher, the editors and the reviewers. Any product that may be evaluated in this article, or claim that may be made by its manufacturer, is not guaranteed or endorsed by the publisher.
The Supplementary Material for this article can be found online at: https://www.frontiersin.org/articles/10.3389/fbioe.2024.1355019/full#supplementary-material
Alam, F., Shukla, V., Varadarajan, K., and Kumar, S. (2020). Microarchitected 3D printed polylactic acid (PLA) nanocomposite scaffolds for biomedical applications. J. Mech. Behav. Biomed. 103, 103576. doi:10.1016/j.jmbbm.2019.103576
Anita Lett, J., Sagadevan, S., Léonard, E., Fatimah, I., Hossain, M., Mohammad, F., et al. (2021). Bone tissue engineering potentials of 3D printed magnesium-hydroxyapatite in polylactic acid composite scaffolds. Artif. Organs 45 (12), 1501–1512. doi:10.1111/aor.14045
Arumugam, S., Kandasamy, J., Shah, A., Sultan, M., Safri, S., Majid, M., et al. (2020). Investigations on the mechanical properties of glass fiber/sisal fiber/chitosan reinforced hybrid polymer sandwich composite scaffolds for bone fracture fixation applications. Polymers 12 (7), 1501. doi:10.3390/polym12071501
Barbeck, M., Serra, T., Booms, P., Stojanovic, S., Najman, S., Engel, E., et al. (2017). Analysis of the in vitro degradation and the in vivo tissue response to Bi-layered 3D-printed scaffolds combining PLA and biphasic PLA/bioglass components - guidance of the inflammatory response as basis for osteochondral regeneration. Bioact. Mat. 2 (4), 208–223. doi:10.1016/j.bioactmat.2017.06.001
Boere, K., Visser, J., Seyednejad, H., Rahimian, S., Gawlitta, D., Steenbergen, M., et al. (2014). Covalent attachment of a three-dimensionally printed thermoplast to a gelatin hydrogel for mechanically enhanced cartilage constructs. Acta Biomater. 10 (6), 2602–2611. doi:10.1016/j.actbio.2014.02.041
Chandran, S., and John, A. (2019). Osseointegration of osteoporotic bone implants: role of stem cells, silica and strontium - a concise review. J. Clin. Orthop. Trauma 10 (1), S32–S36. doi:10.1016/j.jcot.2018.08.003
Chen, T., Wang, Y., Hao, Z., Hu, Y., and Li, J. (2021). Parathyroid hormone and its related peptides in bone metabolism. Biochem. Pharmacol. 192, 114669. doi:10.1016/j.bcp.2021.114669
Chen, X., Hu, Y., Geng, Z., and Su, J. (2022). The ‘three in one’ bone repair strategy for osteoporotic fractures. Front. Endocrinol. 13, 910602. doi:10.3389/fendo.2022.910602
Collins, M., Ren, G., Young, K., Pina, S., Reis, R., and Oliveira, J. (2021). Scaffold fabrication technologies and structure/function properties in bone tissue engineering. Adv. Funct. Mat. 31 (21), 2010609. doi:10.1002/adfm.202010609
Dang, L., Zhu, J., and Song, C. (2021). The effect of topical administration of simvastatin on entochondrostosis and intramembranous ossification: an animal experiment. J. Orthop. Transl. 28, 1–9. doi:10.1016/j.jot.2020.11.009
Delan, W., Zakaria, M., Elsaadany, B., ElMeshad, A., Mamdouh, W., and Fares, A. (2020). Formulation of simvastatin chitosan nanoparticles for controlled delivery in bone regeneration: optimization using box-behnken design, stability and in vivo study. Int. J. Pharm. 577, 119038. doi:10.1016/j.ijpharm.2020.119038
Eswaramoorthy, R., Chang, C., Wu, S., Wang, G., Chang, J., and Ho, M. (2012). Sustained release of PTH(1–34) from PLGA microspheres suppresses osteoarthritis progression in rats. Acta Biomater. 8 (6), 2254–2262. doi:10.1016/j.actbio.2012.03.015
Fang, X., Xie, J., Zhong, L., Li, J., Rong, D., Li, X., et al. (2016). Biomimetic gelatin methacrylamide hydrogel scaffolds for bone tissue engineering. J. Mat. Chem. B 4 (6), 1070–1080. doi:10.1039/c5tb02251g
Gasparotto, M., Bellet, P., Scapin, G., Busetto, R., Rampazzo, C., Vitiello, L., et al. (2022). 3D printed graphene-PLA scaffolds promote cell alignment and differentiation. Int. J. Mol. Sci. 23 (3), 1736. doi:10.3390/ijms23031736
Grémare, A., Guduric, V., Bareille, R., Heroguez, V., Latour, S., L’heureux, N., et al. (2018). Characterization of printed PLA scaffolds for bone tissue engineering. J. Biomed. Mat. Res. A 106 (4), 887–894. doi:10.1002/jbm.a.36289
Guo, C., Wan, R., He, Y., Lin, S., Cao, J., Qiu, Y., et al. (2022). Therapeutic targeting of the mevalonate–geranylgeranyl diphosphate pathway with statins overcomes chemotherapy resistance in small cell lung cancer. Nat. Cancer 3 (5), 614–628. doi:10.1038/s43018-022-00358-1
Han, S., Cha, M., Jin, Y., Lee, K., and Lee, J. (2020). BMP-2 and hMSC dual delivery onto 3D printed PLA-biogel scaffold for critical-size bone defect regeneration in rabbit tibia. Biomed. Mat. 16 (1), 015019. doi:10.1088/1748-605X/aba879
Hauser, B., Alonso, N., and Riches, P. (2021). Review of current real-world experience with teriparatide as treatment of osteoporosis in different patient groups. J. Clin. Med. 10 (7), 1403. doi:10.3390/jcm10071403
Jeon, J., and Puleo, D. (2008). Alternating release of different bioactive molecules from a complexation polymer system. Biomaterials 29 (26), 3591–3598. doi:10.1016/j.biomaterials.2008.05.011
Jiang, L., Zhang, W., Wei, L., Zhou, Q., Yang, G., Qian, N., et al. (2018). Early effects of parathyroid hormone on vascularized bone regeneration and implant osseointegration in aged rats. Biomaterials 179, 15–28. doi:10.1016/j.biomaterials.2018.06.035
Jin, H., Ji, Y., Cui, Y., Xu, L., Liu, H., and Wang, J. (2021). Simvastatin-incorporated drug delivery systems for bone regeneration. ACS Biomater. Sci. Eng. 7 (6), 2177–2191. doi:10.1021/acsbiomaterials.1c00462
Khandelwal, S., and Lane, N. (2023). Osteoporosis: review of etiology, mechanisms, and approach to management in the aging population. Endocrin. Metab. Clin. 52 (2), 259–275. doi:10.1016/j.ecl.2022.10.009
Kimicata, M., Mahadik, B., and Fisher, J. (2021). Long-term sustained drug delivery via 3D printed masks for the development of a heparin-loaded interlayer in vascular tissue engineering applications. ACS Appl. Mat. Interfaces 13 (43), 50812–50822. doi:10.1021/acsami.1c16938
Kodach, L., Jacobs, R., Voorneveld, P., Wildenberg, M., Verspaget, H., Wezel, T., et al. (2011). Statins augment the chemosensitivity of colorectal cancer cells inducing epigenetic reprogramming and reducing colorectal cancer cell ‘stemness’ via the bone morphogenetic protein pathway. Gut 60 (11), 1544–1553. doi:10.1136/gut.2011.237495
Langdahl, B. (2021). Overview of treatment approaches to osteoporosis. Brit. J. Pharmacol. 178 (9), 1891–1906. doi:10.1111/bph.15024
Ma, L., Wang, X., Zhao, N., Zhu, Y., Qiu, Z., Li, Q., et al. (2018). Integrating 3D printing and biomimetic mineralization for personalized enhanced osteogenesis, angiogenesis, and osteointegration. ACS Appl. Mat. Interfaces 10 (49), 42146–42154. doi:10.1021/acsami.8b17495
Martin, V., and Bettencourt, A. (2018). Bone regeneration: biomaterials as local delivery systems with improved osteoinductive properties. Mat. Sci. Eng. C. Mat. 82, 363–371. doi:10.1016/j.msec.2017.04.038
Meng, F., Yin, Z., Ren, X., Geng, Z., and Su, J. (2022). Construction of local drug delivery system on titanium-based implants to improve osseointegration. Pharmaceutics 14 (5), 1069. doi:10.3390/pharmaceutics14051069
Mundy, G., Garrett, R., Harris, S., Chan, J., Chen, D., Rossini, G., et al. (1999). Stimulation of bone formation in vitro and in rodents by statins. Science 286 (5446), 1946–1949. doi:10.1126/science.286.5446.1946
Pires, F., Miranda, A., Cardoso, E., Cardoso, A., Fregnani, E., Pereira, C., et al. (2005). Oral avascular bone necrosis associated with chemotherapy and biphosphonate therapy. Oral Dis. 11 (6), 365–369. doi:10.1111/j.1601-0825.2005.01130.x
Rocha, T., Cavalcanti, A., Leal, A., Dias, R., Costa, R., Ribeiro, G., et al. (2021). PTH1-34 improves devitalized allogenic bone graft healing in a murine femoral critical size defect. Injury 52 (3), S3–S12. doi:10.1016/j.injury.2021.03.063
Saini, P., Arora, M., and Kumar, M. (2016). Poly(Lactic acid) blends in biomedical applications. Adv. Drug Deliv. Rev. 107, 47–59. doi:10.1016/j.addr.2016.06.014
Sang, S., Wang, S., Yang, C., Geng, Z., and Zhang, X. (2022). Sponge-inspired sulfonated polyetheretherketone loaded with polydopamine-protected osthole nanoparticles and berberine enhances osteogenic activity and prevents implant-related infections. Chem. Eng. J. 437, 135255. doi:10.1016/j.cej.2022.135255
Silva, B., and Bilezikian, J. (2015). Parathyroid hormone: anabolic and catabolic actions on the skeleton. Curr. Opin. Pharm. 22, 41–50. doi:10.1016/j.coph.2015.03.005
Spicer, P., Kretlow, J., Young, S., Jansen, J., Kasper, F., and Mikos, A. (2012). Evaluation of bone regeneration using the rat critical size calvarial defect. Nat. Protoc. 7 (10), 1918–1929. doi:10.1038/nprot.2012.113
Sun, M., Sun, Xi., Wang, Z., Guo, S., Yu, G., and Yang, H. (2018). Synthesis and properties of gelatin methacryloyl (GelMA) hydrogels and their recent applications in load-bearing tissue. Polymers 10 (11), E1290. doi:10.3390/polym10111290
Tao, Z., Zhou, W., Bai, B., Cui, W., Lv, Y., Yu, X., et al. (2016). The effects of combined human parathyroid hormone (1–34) and simvastatin treatment on the interface of hydroxyapatite-coated titanium rods implanted into osteopenic rats femurs. J. Mat. Sci. Mat. M. 27 (3), 43. doi:10.1007/s10856-015-5650-9
Tao, Z., Zhou, W., Tu, K., Huang, Z., Zhou, Q., Sun, T., et al. (2015). The effects of combined human parathyroid hormone (1-34) and simvastatin treatment on osseous integration of hydroxyapatite-coated titanium implants in the femur of ovariectomized rats. Injury 46 (11), 2164–2169. doi:10.1016/j.injury.2015.08.034
Tilkin, R., Régibeau, N., Lambert, S., and Grandfils, C. (2020). Correlation between surface properties of polystyrene and polylactide materials and fibroblast and osteoblast cell line behavior: a critical overview of the literature. Biomacromolecules 21 (6), 1995–2013. doi:10.1021/acs.biomac.0c00214
Wang, C., Wang, Y., Lin, C., Lee, T., Fu, Y., Ho, M., et al. (2018). Combination of a bioceramic scaffold and simvastatin nanoparticles as a synthetic alternative to autologous bone grafting. Int. J. Mol. Sci. 19 (12), E4099. doi:10.3390/ijms19124099
Wang, F., Xia, D., Wang, S., Gu, R., Yang, F., Zhao, X., et al. (2021). Photocrosslinkable Col/PCL/Mg composite membrane providing spatiotemporal maintenance and positive osteogenetic effects during guided bone regeneration. Bioact. Mater 13, 53–63. doi:10.1016/j.bioactmat.2021.10.019
Wang, S., Shi, K., Lu, J., Sun, W., Han, Q., Che, L., et al. (2021). Microsphere-embedded hydrogel sustained-release system to inhibit postoperative epidural fibrosis. ACS Appl. Bio Mat. 4 (6), 5122–5131. doi:10.1021/acsabm.1c00347
Wang, Y., Wang, J., Gao, R., Liu, X., Feng, Z., Zhang, C., et al. (2022). Biomimetic glycopeptide hydrogel coated PCL/nHA scaffold for enhanced cranial bone regeneration via macrophage M2 polarization-induced osteo-immunomodulation. Biomaterials 285, 121538. doi:10.1016/j.biomaterials.2022.121538
Wani, T., Khan, R., Rather, A., Beigh, M., and Sheikh, F. (2021). Local dual delivery therapeutic strategies: using biomaterials for advanced bone tissue regeneration. J. Control. Release 339, 143–155. doi:10.1016/j.jconrel.2021.09.029
Wu, Z., Meng, Z., Wu, Q., Zeng, D., Guo, Z., Yao, J., et al. (2020). Biomimetic and osteogenic 3D silk fibroin composite scaffolds with nano MgO and mineralized hydroxyapatite for bone regeneration. J. Tissue Eng. 11, 204173142096779. doi:10.1177/2041731420967791
Xia, Y., Xie, Y., Yu, Z., Xiao, H., Jiang, G., Zhou, X., et al. (2018). The mevalonate pathway is a druggable target for vaccine adjuvant discovery. Cell. 175 (4), 1059–1073. doi:10.1016/j.cell.2018.08.070
Xie, Z., Weng, S., Li, H., Yu, X., Lu, S., Huang, K., et al. (2017). Teriparatide promotes healing of critical size femur defect through accelerating angiogenesis and degradation of β-TCP in OVX osteoporotic rat model. Biomed. Pharmacother. 96, 960–967. doi:10.1016/j.biopha.2017.11.141
Xu, D., Chen, S., Xie, C., Liang, Q., and Xiao, X. (2022). Cryogenic 3D printing of modified polylactic acid scaffolds with biomimetic nanofibrous architecture for bone tissue engineering. J. Biomat. Sci. Polym. E. 33 (4), 532–549. doi:10.1080/09205063.2021.1997210
Xu, L., Sun, X., Zhu, G., Mao, J., Baban, B., and Qin, X. (2021). Local delivery of simvastatin maintains tooth anchorage during mechanical tooth moving via anti-inflammation property and AMPK/MAPK/NF-kB inhibition. J. Cell. Mol. Med. 25 (1), 333–344. doi:10.1111/jcmm.16058
Xu, R., Shi, G., Xu, L., Gu, Q., Fu, Y., Zhang, P., et al. (2018). Simvastatin improves oral implant osseointegration via enhanced autophagy and osteogenesis of BMSCs and inhibited osteoclast activity. J. Tissue Eng. Regen. Med. 12 (5), 1209–1219. doi:10.1002/term.2652
Xue, X., Zhang, H., Liu, H., Wang, S., Li, J., Zhou, Q., et al. (2022). Rational design of multifunctional CuS nanoparticle-PEG composite soft hydrogel-coated 3D hard polycaprolactone scaffolds for efficient bone regeneration. Adv. Funct. Mater 32 (33), 2202470. doi:10.1002/adfm.202202470
Yuan, X., Yuan, Z., Wang, Y., Wan, Z., Wang, X., Yu, S., et al. (2022). Vascularized pulp regeneration via injecting simvastatin functionalized GelMA cryogel microspheres loaded with stem cells from human exfoliated deciduous teeth. Mater Today Bio 13, 100209. doi:10.1016/j.mtbio.2022.100209
Zhang, C., and Song, C. (2020). Combination therapy of PTH and antiresorptive drugs on osteoporosis: a review of treatment alternatives. Front. Pharmacol. 11, 607017. doi:10.3389/fphar.2020.607017
Zhang, H., Hu, Y., Chen, X., Wang, S., Cao, L., Dong, S., et al. (2022). Expert consensus on the bone repair strategy for osteoporotic fractures in China. Front. Endocrinol. 13, 989648. doi:10.3389/fendo.2022.989648
Zhang, Y., Wang, H., Huangfu, H., Zhang, X., Zhang, H., Qin, Q., et al. (2022). 3D printing of bone scaffolds for treating infected mandible bone defects through adjustable dual-release of chlorhexidine and osteogenic peptide. Mat. Des. 224, 111288. doi:10.1016/j.matdes.2022.111288
Zhao, X., Lang, Q., Yildirimer, L., Lin, Z., Cui, W., Annabi, N., et al. (2016). Photocrosslinkable gelatin hydrogel for epidermal tissue engineering. Adv. Healthc. Mat. 5 (1), 108–118. doi:10.1002/adhm.201500005
Zhao, Z., Li, G., Ruan, H., Chen, K., Cai, Z., Lu, G., et al. (2021). Capturing magnesium ions via microfluidic hydrogel microspheres for promoting cancellous bone regeneration. ACS Nano 15 (8), 13041–13054. doi:10.1021/acsnano.1c02147
Zhou, C., Wang, Y., Meng, J., Yao, M., Xu, H., Wang, C., et al. (2022). Additive effect of parathyroid hormone and zoledronate acid on prevention particle wears-induced implant loosening by promoting periprosthetic bone architecture and strength in an ovariectomized rat model. Front. Endocrinol. 13, 871380. doi:10.3389/fendo.2022.871380
Zhou, X., Zhou, G., Junka, R., Chang, N., Anwar, A., Wang, H., et al. (2021). Fabrication of polylactic acid (PLA)-Based porous scaffold through the combination of traditional bio-fabrication and 3D printing Technology for bone regeneration. Colloid. Surf. B 197, 111420. doi:10.1016/j.colsurfb.2020.111420
Keywords: bone regeneration, osteoporosis, drug delivery system, PTH1-34, simvastatin
Citation: Xu T, Gao S, Yang N, Zhao Q, Zhang Y, Li T, Liu Z and Han B (2024) A personalized biomimetic dual-drug delivery system via controlled release of PTH1-34 and simvastatin for in situ osteoporotic bone regeneration. Front. Bioeng. Biotechnol. 12:1355019. doi: 10.3389/fbioe.2024.1355019
Received: 13 December 2023; Accepted: 17 January 2024;
Published: 31 January 2024.
Edited by:
Jianxun Ding, Chinese Academy of Sciences (CAS), ChinaReviewed by:
Zhen Geng, Shanghai University, ChinaCopyright © 2024 Xu, Gao, Yang, Zhao, Zhang, Li, Liu and Han. This is an open-access article distributed under the terms of the Creative Commons Attribution License (CC BY). The use, distribution or reproduction in other forums is permitted, provided the original author(s) and the copyright owner(s) are credited and that the original publication in this journal is cited, in accordance with accepted academic practice. No use, distribution or reproduction is permitted which does not comply with these terms.
*Correspondence: Zhihui Liu, bGl1X3poQGpsdS5lZHUuY24=; Bing Han, aGFuYkBqbHUuZWR1LmNu
Disclaimer: All claims expressed in this article are solely those of the authors and do not necessarily represent those of their affiliated organizations, or those of the publisher, the editors and the reviewers. Any product that may be evaluated in this article or claim that may be made by its manufacturer is not guaranteed or endorsed by the publisher.
Research integrity at Frontiers
Learn more about the work of our research integrity team to safeguard the quality of each article we publish.