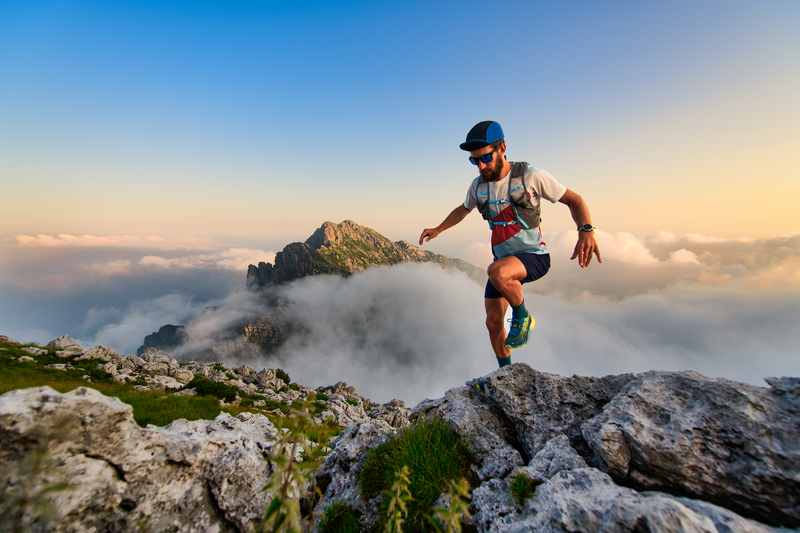
95% of researchers rate our articles as excellent or good
Learn more about the work of our research integrity team to safeguard the quality of each article we publish.
Find out more
REVIEW article
Front. Bioeng. Biotechnol. , 25 January 2024
Sec. Bioprocess Engineering
Volume 12 - 2024 | https://doi.org/10.3389/fbioe.2024.1352014
Bispecific antibodies (bsAbs) have attracted significant attention due to their dual binding activity, which permits simultaneous targeting of antigens and synergistic binding effects beyond what can be obtained even with combinations of conventional monospecific antibodies. Despite the tremendous therapeutic potential, the design and construction of bsAbs are often hampered by practical issues arising from the increased structural complexity as compared to conventional monospecific antibodies. The issues are diverse in nature, spanning from decreased biophysical stability from fusion of exogenous antigen-binding domains to antibody chain mispairing leading to formation of antibody-related impurities that are very difficult to remove. The added complexity requires judicious design considerations as well as extensive molecular engineering to ensure formation of high quality bsAbs with the intended mode of action and favorable drug-like qualities. In this review, we highlight and summarize some of the key considerations in design of bsAbs as well as state-of-the-art engineering principles that can be applied in efficient construction of bsAbs with diverse molecular formats.
In recent years, bispecific antibodies (bsAbs) have emerged as a powerful class of therapeutic molecules, opening new avenues for targeted therapy. BsAbs possess the unique ability to simultaneously engage two different targets, enabling synergistic targeting of disease pathways that cannot be obtained even with combinations of conventional immunoglobulins. While therapeutic bsAbs are engineered molecules, bsAbs can also be found in rare cases in nature where they can be formed through Fab-arm exchange of IgG4 half molecules (Rispens et al., 2011). The binding versatility of engineered bsAbs has sparked significant interest in their potential applications across a wide range of diseases, including cancer, autoimmune disorders, and infectious diseases (Labrijn et al., 2019). In a recent publication, bi- and multispecific drugs were even classified as a new wave of transformative therapeutics (Deshaies, 2020). The therapeutic potential of bsAbs has made the bsAb space very crowded and extremely competitive but it has also fostered truly ingenious feats of engineering as illustrated by the diversity of the clinical-stage bsAb landscape (Labrijn et al., 2019; Wei et al., 2022).
The dual binding activity of bsAbs enables synergistic antigen targeting with more complex mechanisms of action (MOA) than what can be obtained for conventional monoclonal antibodies. For many bsAbs the desired MOA is dependent on a certain spatiotemporal connection between the two binding events. This means that the two binding specificities must be engaged in a specific physical arrangement (e.g., positioning targets near each other to induce downstream signaling (Sampei et al., 2013)) and/or with a specific timing (e.g., simultaneously linking cells (Li et al., 2021) or sequential targeting for translocating across barriers (Wec et al., 2016; Do et al., 2020)). Such bsAbs where the MOA cannot be obtained through combination of the two separate parental antibodies are known as obligate bsAbs (Spiess et al., 2015; Labrijn et al., 2019). However, even bsAbs without obligate MOA often corroborate to be more than simply the sum of their parts, which is illustrated by non-obligate bsAbs still showing superior potency relative to combination of the parental antibodies (Kast et al., 2021). The advantage of such combinatorial MOA has been speculated to stem from avidity effects (De Gasparo et al., 2021). Dual targeting might also help minimize side effects by improving target selectivity and localization (Mazor et al., 2015a; Mazor et al., 2017), which is especially relevant to limit “on-target, off-tumor” effects (Ishiguro et al., 2017). Even in cases where the bsAb activity is comparable to that of the parental antibody combination, the bsAb may offer an advantage regarding manufacturing since only a single molecule needs to be produced (Hmila et al., 2010).
The dual targeting activity of bsAbs can broadly be characterized as working in-trans or in-cis. The possibility of judiciously combining selected antigen-binding domains to specifically act on the same (in-cis) or different (in-trans) cellular/molecular targets in a highly tailored manner is essential in synergistic bsAb functionalities.
In-trans acting bsAbs engage distinct targets to create a physical linkage. For bsAbs engaging cellular targets in-trans this means the MOA is dependent on binding of two different cells thus permitting bridging of the cells. A common example of in-trans cellular binding are bispecific T cell engagers that bind to both a tumor associated antigen and CD3, a highly potent costimulatory receptor, thus bridging T cell and tumor cells (Löffler et al., 2000; Hoffmann et al., 2005) (Figure 1A). The bispecific T cell engagers are bypassing the natural T cell activation through clustering of low-affinity T cell receptors (Krogsgaard and Davis, 2005) and recruit T cells directly towards cells expressing the tumor-associated antigen (Löffler et al., 2000). The term “in-trans” has also been used on a molecular level to describe a biparatopic anti-HER2 bsAb that bind two distinct HER2 molecules (in-trans) rather than the same (in-cis). The cross-binding links adjacent molecules to induce distinct HER2 reorganization with complement-dependent cytotoxicity not seen for combinations of monospecific anti-HER2 antibodies (Weisser et al., 2023). Such molecular in-trans binding is primarily relevant for biparatopic bsAbs targeting different and non-overlapping epitopes on the same antigenic target.
FIGURE 1. Obligate mechanisms of action for bsAbs. (A) In-trans cell bridging established a physical link between different cells through the bsAb. This mechanism is especially relevant for T cell redirecting bsAbs where the physical connection helps target the cytotoxicity of the activated T cells. (B) In-cis bridging of receptors causes agonistic crosslinking and associated activation of receptor signaling. (C) In-cis antagonism blocks receptor association thus preventing signaling through the receptor complex. (D) Piggybacking bsAbs use one specificity for targeting a receptor that facilitates translocation across a barrier to an otherwise inaccessible compartment where the second specificity exerts its functionality. The lightning bolt indicates an activity mediated by binding of the antibody. (E) BsAbs can exert localization effects. An example is agonistic bsAbs that also contain a tumor-targeted specificity, which limits on-target, off-tumor effects by restricting the agonistic mechanism to sites where the tumor marker is present. (F) BsAbs acting as a co-factor mimetic by accurately positioning of enzyme and substrate.
In-cis acting bsAbs primarily target antigens on the same cell (Figures 1B,C). Agonistic bsAbs that activate two receptors on the surface of the same cell (cellular in-cis activation) can be used to selectively control signaling mechanisms by precisely steering bridging of receptor subunits. In one example, a bsAb targeting the interleukin (IL)-2 receptor subunits IL-2Rβ and IL-2Rγ was used to increase signaling through the intermediate affinity IL-2Rβγ while downregulating signaling through the high affinity IL-2Rαβγ (Ha et al., 2021). In another example a dual agonistic bispecific Surrobody targeting death receptor (DR) 4 and 5 showed superior potency than combinations of the parental antibodies potentially due to heterodimeric clustering of DR4 and DR5 (Milutinovic et al., 2016).
Another type of in-cis activating bsAbs is known as co-factor mimetics that essentially acts to promote optimal positioning of an enzyme and its cognate substrate (Figure 1F). Coagulation factor FVIIIa mimetics have been developed to promote complexation of FIXa and FX (Sampei et al., 2013; Østergaard et al., 2021). Assembly of the FIXa-FX enzyme-substrate complex leads to formation of activated factor Xa (FXa), which is a key mediator of the coagulation pathway (Borensztajn et al., 2008) and the bsAbs are therefore relevant for treatment of hemophilia A (Shima et al., 2016; Mahlangu et al., 2018; Lauritzen et al., 2022). This bsAb cofactor mimetic is especially relevant for patients that develop alloantibodies against FVIII and which are therefore largely restricted from treatment with recombinant FVIII (Muto et al., 2014).
Signaling pathways can also be targeted through antagonistic bsAbs working by occupying cellular receptors and blocking binding of soluble receptor ligands. Such antagonistic bsAbs are commonly used in blocking of immune checkpoint receptors, which is an extremely successful class of anti-cancer immunotherapy targets (Robert, 2020). Examples include dual blockade of immune checkpoint pathways (Kraman et al., 2020; Chen et al., 2021; Dovedi et al., 2021) and targeting of immune checkpoints together with negative regulators of anti-tumor immunity, such as TGF-β, that is associated with poor prognosis (Bai et al., 2019; Yi et al., 2021). Antagonistic bsAbs working in-cis can also be used for targeting related or overlapping signaling pathways (Dong et al., 2011). Such bsAbs can thus be used to preempt immune evasion of cancer cells by targeting a primary cancer-related pathway as well as other connected pathways that might be upregulated by the cancer cell in response to blocking of the primary pathway. An example of antagonistic dual pathway targeting is seen for HER2xHER3 bsAbs where upregulation of HER3 is a driver in resistance to HER2 inhibiting agents (Garrett et al., 2011).
Discovery of agonistic bsAbs is generally more challenging than antagonistic bsAbs because the agonistic activity of antibodies depends on multiple factors that are difficult to properly formalize (Mayes et al., 2018). As an example, the cytotoxic activity of agonistic anti-CD3 bsAbs have been found to be influenced by both the affinity, epitope, and molecular geometry (Bluemel et al., 2010; Moore et al., 2015; Root et al., 2016). Contrarily, an effective antagonistic antibody is typically characterized by a high affinity and ability to compete with the natural ligand thereby blocking its action. Further, additional synergies in therapeutic effector mechanisms might be obtained from combinations of bsAbs although this will likely increase the MOA complexity and thus further complicate bsAb design.
All natural antibodies contain an Fc region that is paramount for the antibody effector functions, which triggers the host cell responses through binding to cognate Fc receptors and soluble immune mediators such as complement proteins (Lu et al., 2018). Fc protein engineering and glycoengineering can be used to either remove or largely enhance Fc-mediated effector functions such as antibody-dependent cellular cytotoxicity (ADCC) by NK cells, antibody-dependent cellular phagocytosis (ADCP) by macrophages and even certain CD8 T cell responses (Hart et al., 2017; Goletz et al., 2018; Liu et al., 2020). Significant efforts have been made to engineer Fc regions of conventional monospecific antibodies to obtain Fc effector functions (or lack thereof) that are not naturally observed for a given isotype (Saunders, 2019). As an example, FcγR silencing mutations can be used to create effector-reduced IgG1 antibodies that still possess the favorable characteristics that are associated with the IgG1 isotype (Tang et al., 2021; Cain et al., 2023). On the other hand, glycoengineering, such as de-core-fucosylation of Fc-IgG1 N-glycans, or mutations can increase binding to certain Fc receptors and thereby mediate increased effector functions such as FcγRIIIa mediated ADCC or ADCP. Such effector-modulating mutations or glycoengineering are typically not overlapping with common bsAb-related mutations (discussed below) such as those used for chain-steering and hence can be combined in Fc-containing bsAbs as well (Escobar-Cabrera et al., 2017). Depending on the intended MOA, certain bsAbs might be more effectively designed with a modified Fc region or without one altogether. Most clinically developed bsAbs contain a Fc region (Labrijn et al., 2019) as this domain confers easy purification using protein A (Liu et al., 2010) as well as prolonged half-life from FcRn recycling (Ghetie et al., 1996; Christianson et al., 1997). Fc-containing bsAbs are broadly categorized as symmetric or asymmetric according to their molecular geometry, as discussed below.
Despite effective engineering of the Fc region, there are cases where smaller fragment-based bsAbs offer advantages over large IgG-like bsAbs. Fragment-based bsAbs are typically constructed through genetic fusion of independent antigen-binding domains but can also be assembled through multimerization domains (Blanco-Toribio et al., 2013; Walter et al., 2022; Burke et al., 2023). This simplistic approach has the advantage that its products are typically small and without important glycosylation sites, meaning that they can be produced at high titers using simple expression systems like bacteria (Zhu et al., 1996; Lu et al., 2022) or yeast (Xiong et al., 2018). Fragment-based bsAbs typically consist of only one to two polypeptide chains, which limits the risk of antibody-related impurities due to chain mispairing, which is an issue often encountered for larger and structurally more complex bsAbs as discussed below. Further, simple genetic fusion of antigen-binding domains permits high flexibility with regard to combination of valencies and specificities as exemplified by 1 + 3 (Harwood et al., 2018) and 3 + 3 (Blanco-Toribio et al., 2013; Compte et al., 2018) bsAb formats.
Fragment-based bsAbs lacking an Fc region have traditionally been based on scFv fragments that can be combined in different ways to form, e.g., BiTE (Mack et al., 1995; Löffler et al., 2000), TandAb (Reusch et al., 2014; Reusch et al., 2015), or DART (Johnson et al., 2010; Chichili et al., 2015) molecules, which are the main classes of fragment-based bsAbs utilizing scFvs (Figure 2A). Such scFv fragments are, however, often associated with thermodynamic instability (Wörn and Plückthun, 2001) and promiscuous VH-VL pairing leading to undesired self-assembly (Weatherill et al., 2012). The limitations of scFvs have sparked interest in single-domain antibodies (sdAbs) which are also small (∼15 kDa) antigen-binding domains that can be genetically fused to form fragment-based bsAbs (Conrath et al., 2001; de Bruin et al., 2018) but because they are stable, robust, and monomeric in nature, they are not prone to undesired self-assembly. SdAbs have also been combined without linkers by fusion onto CH1 and Cκ, respectively, thus using the natural pairing of the constant domains as a natural dimerization motif to generate small Fab-like bsAbs (Rozan et al., 2013). It should of course be noted that antigen-binding domains can be combined in numerous other ways than those listed above, as previously described (Brinkmann and Kontermann, 2017). A potential drawback from fragment-based bsAbs is their faster clearance due to the small size and lack of FcRn binding regions, however, the clearance issue has been addressed for certain applications and formats by coupling of the bsAb to HSA (McDonagh et al., 2012; Mandrup et al., 2021; Hangiu et al., 2022) or including an anti-HSA antibody fragment (Austin et al., 2021; Edwards et al., 2022; Ishiwatari-Ogata et al., 2022).
FIGURE 2. Schematic overview of the most common bsAb formats categorized according to molecular configuration. (A) Fragment-based bsAbs are formed by combining small antigen-binding fragments without a Fc domain. The building blocks are typically scFv fragments or sdAbs. (B) Symmetric Fc-containing bsAbs are expressed from one to two polypeptide chains often by fusing additional antigen-binding fragments onto an IgG scaffold. (C) Asymmetric Fc-containing bsAbs assembled from two to four polypeptide chains. Asymmetric bsAbs require measures to avoid chain mispairing. The small “knob” and “hole” in the schematics are used to generically illustrate places where a chain steering technology is needed to ensure HC:HC heterodimerization and/or proper HC:LC pairing.
Symmetric bsAbs are typically IgG-like molecules that adhere to the HC2LC2 format but with additional exogenous binding domains fused to the scaffold thus adding specificity towards a second antigen to the original scaffold. These bsAbs are thus typically tetravalent 2 + 2 bispecifics (bivalent targeting of each antigen) (Pang et al., 2023) but can also be made as hexavalent 2 + 4 bsAbs by double fusions (Madsen et al., 2023). Some of the most common tetravalent 2 + 2 formats include scFv-IgG fusion (Orcutt et al., 2010; DiGiandomenico et al., 2014; Pang et al., 2023), DVD-Ig (Wu et al., 2007; Wu et al., 2009), tandem scFv-Fc (Chang et al., 2022), sdAb-IgG fusions (Madsen et al., 2022; Yanakieva et al., 2022; Madsen et al., 2023), and tetra-VH IgG (Ljungars et al., 2020; Misson Mindrebo et al., 2023) (Figure 2). A strong practical advantage of symmetric bsAbs resides in the relatively simple antibody assembly where only one or two different polypeptide chains need to be expressed. This means there is less optimization of plasmid transfection ratios in initial screens compared to co-expression of three to four chains for asymmetric bsAbs. The HC2LC2 format also greatly reduces the risk of misassembly of antibody chains and thus also simplifies the purification scheme compared to asymmetric bsAbs where additional polishing steps are often needed to remove impurities formed through incorrect assembly of antibody chains (Li, 2019). The HC2LC2 format, however, also limits the flexibility in valencies as the antigen-binding domains will always appear in pairs. This limits the application to antigens where monovalent targeting is required to prevent undesired crosslinking, such as CD3 (Lee et al., 2019). The choice of a suitable exogenous antigen-binding domain is an important consideration when designing symmetric bsAbs. Traditionally, scFvs have often been used because they can be readily derived from Fv domains of conventional antibodies. Smaller sdAbs are, however, gaining increasing attention as attractive fusion partners because they are naturally small and monomeric and thus not prone to undesired self-assembly and aggregation, which is often seen for scFvs (Cao et al., 2018; Andrade et al., 2019).
Glycine-serine linkers of 10–25 amino acids are commonly used for fusion of exogenous antigen-binding domains as these exhibit favorable flexibility and stability in aqueous solutions (Chen et al., 2013). Other bsAb constructs have utilized linkers derived from natural antibody linker regions such as the antibody hinge region (Kuo et al., 2012) or the flexible link connecting Fv and CH1/Cκ (Wu et al., 2007; Wu et al., 2009). The choice of an appropriate linker is important to ensure proper spacing and display of the antigen-binding domains (Wu et al., 2009; Madsen et al., 2023). One study found linker lengths to affect both antigen-binding and stability of DVD-Ig molecules (Wu et al., 2009).
The highly modular nature of antibodies means that the exogenous antigen-binding domains can be fused both within or at the ends of polypeptide chains of the scaffold, thus enabling formation of structurally diverse bsAbs that can be tailored to fit the purpose (DiGiandomenico et al., 2014; Swope et al., 2020). While fusions of these exogenous antigen-binding fragments are often made onto full length IgG molecules they can also be fused directly to Fc domains to keep the constructs smaller than full length IgG molecules while still including the Fc region (Asano et al., 2020). In addition to the fusion of independent antigen-binding domains onto IgG scaffolds, other strategies have been employed to develop symmetric IgG-like bsAbs. One example is formation of tetra-VH IgGs by separating out distinct binding specificities onto each variable domain of the Fv by replacing VH and VL with independent sdAbs (Ljungars et al., 2020). It has also been possible to spatially segregate the 6 complementarity-determining regions (CDRs) of a single Fab domain into a VH paratope (CDRH1, CDRL2 and CDRH3) and a VL paratope (CDRL1, CDRH2 and CDRL3) (Beckmann et al., 2021). Combining these individual paratopes allowed formation of a single bispecific Fab domain dubbed DutaFab.
The molecular geometry of asymmetric bsAbs makes them more challenging to design and produce compared to symmetric bsAbs mainly due to formation of antibody-related impurities formed through co-expression of the different antibody chains. Despite these practical issues, asymmetric bsAbs are still the major type of bsAb entering into clinical development (Labrijn et al., 2019). A notable advantage of asymmetric bsAbs is the close adherence to the native molecular geometry of IgG to harness the favorable drug-like qualities of this molecule.
The asymmetric architecture allows high flexibility in valencies, e.g., to form monovalent specificities (1 + 1) (Kienast et al., 2013; Zhang et al., 2015), which cannot be obtained for symmetric bsAbs. Further, formation of asymmetric bsAbs with high structural resemblance to IgG molecules is believed to reduce the risk of immunogenicity liabilities. It should, however, be noted that asymmetric bsAbs can also be formed to deviate from the strictly bivalent Y-shaped IgG geometry to form, e.g., trivalent 2 + 1 bsAbs (Metz et al., 2012; Bacac et al., 2016a; Bacac et al., 2016b).
The main issue associated with asymmetric bsAbs is mispairing of polypeptide chains leading to product-related impurities. Many asymmetric bsAbs rely on heterodimerization of two different HC, thus spiking interest in HC steering platforms for promoting HC heterodimerization. Numerous HC steering platforms have been developed (Liu et al., 2017), of which the majority is industry-originated, and functions by creating complementary interfaces in the CH3 domains (Ridgway et al., 1996; Atwell et al., 1997; Merchant et al., 1998; Davis et al., 2010; Gunasekaran et al., 2010; Moore et al., 2011a; Choi et al., 2013; Labrijn et al., 2013; Von Kreudenstein et al., 2013; Choi et al., 2015a; Choi et al., 2015b; Leaver-Fay et al., 2016; De Nardis et al., 2017; Skegro et al., 2017; Estes et al., 2021), which are key in governing the antibody structural integrity (Feige et al., 2010; Bertz et al., 2013). The mutations in the structurally important CH3 domain are therefore also known to often affect the bsAb thermal stability (Pomarici et al., 2022). While the detailed design principles of these CH3 steering platforms are thoroughly reviewed elsewhere (Ha et al., 2016; Brinkmann and Kontermann, 2017), it should be noted that the platforms seek to introduce structurally complementary mutations that favors HC heterodimerization while disfavoring formation of HC homodimers. The first reported, and most widely used, platform is the knob-into-hole (KiH) which introduces a large bulky tryptophan in one HC and smaller sterically complementary residues in the other HC (Atwell et al., 1997; Merchant et al., 1998). The KiH strategy is widely implemented because it is highly effective in suppressing HC homodimers (except for trace amounts of hole-hole homodimers (Chen et al., 2019; Tang et al., 2020)) and because its patent has expired. The KiH thus provides relatively easy and free access to construction of asymmetric bsAbs in a field that is otherwise highly competitive and industry-dominated as illustrated by several other patent-protected platforms (Godar et al., 2018).
When engineering HC for heterodimerization it should be realized that the relative abundance of HC heterodimer should be considered an equilibrium rather than a fixed amount. This equilibrium is affected by several factors such as the relative plasmids- and expression levels, which is typically controlled through optimizing plasmid transfection ratios (Ding et al., 2021; Ong et al., 2022). This can be time consuming especially if expressing many different bsAbs. As an example, we recently reported production of an asymmetric IgG/sdAb-Fc construct where a skewed HC plasmid transfection ratio yielded the most equal expression of the two HC chains (Madsen et al., 2022), thus suggesting the two HCs did not co-express equally well. Other studies have similarly found that the nature of the variable domains affect HC heterodimerization (Stutz and Blein, 2020; Estes et al., 2021). The CH1 domain plays an important role in the assembly and transport of IgG molecules (Hendershot et al., 1987; Feige et al., 2009), which can help explain changes in expression behavior of HCs where the Fab domain has been replaced with fragments such as sdAbs or scFvs. Interestingly, improved assembly of heterodimeric κλ bsAbs has also been obtained through codon de-optimization of the high-expressing λ chain to balance the relatively low-expressing κ thus actually increasing the yield of the target bsAb (Magistrelli et al., 2017).
In addition to HC heterodimerization, strategies also exist for ensuring correct HC:LC pairing. Selection of bsAbs with common LC eliminates the risk of HC:LC mispairing (Merchant et al., 1998; Sampei et al., 2013) although identification of common LC bsAbs can be time consuming and restrictive in terms of sequence diversity (Sampei et al., 2013; Shiraiwa et al., 2019; Ching et al., 2021), which may complicate the search for target-specific and high-affinity binders. The common LC approach further has the advantage that only three polypeptide chains need to be expressed thus easing manufacturability to a certain extent. Analogously, bsAbs with common HC can also be discovered to avoid HC mispairing (Fischer et al., 2015). Other approaches have focused on rational design of Fab steering that prevents HC:LC mispairing during co-expression of the different polypeptide chains, including Crossmab (Schaefer et al., 2011), orthogonal Fab interfaces (Lewis et al., 2014; Liu et al., 2015; Dillon et al., 2017; Froning et al., 2017; Koga et al., 2023), swapping of CH1/CL domains (Wu et al., 2015; Wozniak-Knopp et al., 2018), engineering of native interchain disulfide bonds (Mazor et al., 2015b), and grafting of IgE-derived heterodimerization domains (Kühl et al., 2022). The use of two different HC and LC allows flexible pairing of VH and VL domains and thus unrestricted access to antibody diversification when searching for target-specific bsAbs. On the other hand, balanced co-expression of all 4 polypeptide chains can be challenging thus potentially requiring additional efforts in vector design and production clone generation as well as added pressure on downstream processes. Proper HC:LC pairing can also be obtained through careful post-expression assembly where each antibody half is expressed individually and subsequently assembled to the final bsAb construct. This strategy, however, introduces additional manufacturing steps such as clone generation and manufacturing of 2 components before combination adding further challenging processing steps including sufficient reduction and oxidation of hinge disulfides (Strop et al., 2012; Labrijn et al., 2013; Spiess et al., 2013; Labrijn et al., 2014). Oher strategies include replacing one of the Fab arms with a single-chain Fab (scFab) domain so the bsAb consists of only 3 polypeptide chains and where the flexible linker of the scFab promotes proper pairing of VH/CH1 and VL/CL (Schanzer et al., 2014).
Interestingly, some Fab domains appear to exhibit inherent preferential cognate HC:LC pairing whereas other Fabs exhibit a universally more promiscuous HC:LC pairing (Dillon et al., 2017; Joshi et al., 2019; Gong et al., 2021). The determinants of pairing are mainly located in the CDRs, and such insights might be applicable in selecting compatible HC:LC pairs or identifying common LC. The issue of HC:LC mispairing can also be avoided by simply replacing one or both Fabs with antibody-fragments, such as scFv fragments or sdAbs, to ensure that the bsAb contains a single LC at most thus avoiding HC:LC mispairing (Huang et al., 2020; Ne et al., 2020; Madsen et al., 2022). The importance of proper chain pairing of bsAbs has also spiked interest in advanced analytics and efficient downstream purification processes for accurately removing and quantifying mispaired species with high throughput (Schachner et al., 2016; Yin et al., 2016; Yan et al., 2019; Ziegengeist et al., 2023).
In addition to the assembly methods described above, other strategies more heavily relying on chemical processing have also been explored. One example is expression of an IgG-like bsAb consisting of 4 different polypeptide chains expressed as a single construct where the antibody chains had been connected by linkers that steered chain assembly and could be removed post-expression (Dimasi et al., 2017). BsAb chain association has also been controlled by appending leucine zippers (Wranik et al., 2012) and full-length IgG molecules have been combined through SpyTag/SpyCatcher system (Mei et al., 2022) or using click chemistry (Wagner et al., 2014).
BsAbs are more than just the sum of their parts and selection of an optimal molecular architecture, is an important design consideration to obtain the desired functionality. This means that bsAbs that are constructed from the same molecular building blocks (and thus sharing the same total amino acid content) only differing in molecular geometry can exhibit varying activity (Dickopf et al., 2020; Madsen et al., 2023). The dual targeting is often complex because the bsAb configuration must account for both internal and external restraints to obtain the desired therapeutic functionality. Internal restraints are imposed by the molecular geometry in itself such as steric hindrance between the binding domains (Do et al., 2020; Madsen et al., 2023). One study examining a comprehensive set of symmetric sdAb-IgG bsAbs including mirroring the specificities found that the binding affinity of the antigen-binding domains was affected by inter-domain steric hindrance arising from the molecular geometry and that this effect was more pronounced when the sdAb was linked to the LC as compared to the HC (Madsen et al., 2023). Such internal restraints might be alleviated through engineering of the configuration, e.g., by extending linkers to increase the intramolecular flexibility and distance between target binding (Wu et al., 2007). External restraints, on the other hand, are imposed by the spatial organization of the target environment where the bsAb must adopt a specific conformation to achieve the desired spatiotemporal target engagement. Such external restraints are especially evident for bsAbs with (inter-)cellular activity (Croasdale et al., 2012; Kühl et al., 2023) due to the inherently complex nature of spatial cell surface receptor organization (Bethani et al., 2010). As an example, the immunological synapse required for T cell activation is dependent on the spacing of the T cell antigen receptor and the peptide-MHC ligand (Choudhuri et al., 2005). Given that artificial immunological synapses, established through T cell redirecting bsAbs, have shown high phenotypical similarity to natural immunological synapses (Offner et al., 2006) it is worthwhile considering the natural spacing restraints when designing these T cell redirecting bsAbs. In another example, FynomAbs demonstrated higher cytotoxic activity when the Fynomer (small binding protein derived from the SH3 domain from Fyn kinase) was attached N-terminally as opposed to C-terminally thus further illustrating how the geometry can be tailored for optimal interparatopic positioning (Wuellner et al., 2015). Small fragment-based configurations might also be applicable in cases where short interparatopic distances are favorable. The MOA can likewise be influenced through judicious design of the molecular configuration and interparatopic spacing of antigen-binding domains. This has been shown for T cell engaging scFv-IgG bsAbs where shorter interparatopic spacing obtained through fusion of the scFv C-terminally on the LC proved superior anti-tumor effect both in vitro and in vivo (Santich et al., 2020). Similarly, fragment-based DART molecules targeting CD19 and CD3 have shown superior cell lysis compared to a tandem scFv composed the same binding domains, thus indicating that the more compact configuration of DART molecules are favorable for maintaining cell-cell contacts (Moore et al., 2011b).
The importance of spatial receptor organization has spiked interest in structural analyses of receptor:antibody complexes aiming to uncover underlying mechanisms of effective antibody binding (Lee et al., 2016; Li et al., 2017; Chin et al., 2018). Ideally, such findings will aid the design of improved antibody therapeutics. The importance of the interparatopic distance is not only important in inter-cellular targeting but also for bsAbs where both epitopes are located on the same target cell (DiGiandomenico et al., 2014). For example, the ability of biparatopic anti-HER2 bsAbs to effectively trap HER2 in inactive crosslinked confirmations were highly dependent on the molecular geometry (Kast et al., 2021).
Given the influence of the bsAb configuration, the most potent and effective bsAb cannot be ascertained from analysis of parental monospecific antibodies alone. Efforts are thus made to develop functional screening strategies that goes beyond assessment of parental antibody binding alone and enables screening of combinatorial bsAb panels to also evaluate optimal molecular geometry and combinations of antigen-binding domains (Geuijen et al., 2018; Dengl et al., 2020; Segaliny et al., 2023).
Furthermore, it is not only the molecular geometry that is affecting the bsAb potency but also the relative orientation of the specificities. As an example, significantly reduced HER2 binding was observed when fusing anti-HER2 scFv to an anti-PD1 IgG scaffold compared to reverse orientation, i.e., fusion of the anti-PD1 scFv to the anti-HER2 IgG (Guling et al., 2022). Similar effects from relative orientation have also been shown for fragment-based bsAbs (de Bruin et al., 2018; Andres et al., 2019). In the design of bsAbs, it is not only the molecular configuration and the valencies that must be optimized to achieve the desired MOA but also the relative binding affinities between the different antigen-binding arms. There might thus be a need evaluate combinations of affinity variants to identify the optimal relationship. The importance of balancing the binding affinities has raised interest in mechanistic modelling for understanding the affinity interplay to allow informed bsAb design (Rhod et al., 2016; Kareva et al., 2018; Kareva et al., 2021). This affinity tuning has proven especially important for T cell engaging bsAbs, targeting CD3 and a tumor-associated antigen, and where the relative binding affinities have been shown to affect both efficacy and selectivity (Haber et al., 2021).
In addition to efficient and specific antigen-binding, successful therapeutic bsAbs must possess favorable drug-like qualities, such as high expression, good biophysical stability, low self-association, and aggregation as well as excellent solubility. These traits are commonly known as the developability profile and screening for developability is typically done early in the drug development process to avoid investing in antibodies that are unlikely to succeed as clinical candidates (Jain et al., 2017; Raybould et al., 2019). Extensive work has been aimed at developing in silico predictive tools and high-throughput assays for early screening of candidate developability liabilities (Bailly et al., 2020; Mieczkowski et al., 2023; Svilenov et al., 2023). The methods were, however, primarily developed for conventional monoclonal antibodies and extra attention might therefore be required for bsAbs because the engineering strategies used for constructing the bsAb also risk introducing unexpected liabilities. As an example, we recently showed that fusion of sdAbs onto IgG scaffolds cause changes in the expression yields and biophysical stability and that these changes were dependent on the molecular geometry, the sdAb fusion site on the IgG scaffold, and the number of domains fused (Madsen et al., 2023). This study thus highlights the importance of an optimal molecular geometry and that the bsAb developability profile cannot be ascertained from analysis of the individual building blocks or the parental antibodies alone.
Other examples of developability liabilities potentially introduced by a bsAb configuration include fragmentation, aggregation propensity as well as reduction and re-oxidation of engineered disulfides (Cao et al., 2018; Andrade et al., 2019; Swope et al., 2020; Cramer et al., 2023). Molecular geometry is thus an important design consideration also with respect to chemical and biophysical stability. Further, the molecular format has also been shown to influence the pharmacokinetics of the bsAb (Datta-Mannan et al., 2016; Datta-Mannan et al., 2021) and efforts have been made to establish in vitro assays for predicting bsAb pharmacokinetics (Müller et al., 2023).
The general developability properties of bsAbs are not very well characterized and compared across the large variation of format and geometries available potentially due to the sheer number of bsAb formats (Brinkmann and Kontermann, 2017), making it difficult to generalize on bsAb developability. While larger systematic studies would be advantageous to establish more comparative common rules and developability properties of various molecular formats and geometries the current approach often aims to identify bsAbs with favorable developability profiles within certain intellectual property platform spaces through high-throughput screening of combinatorial libraries and/or through rational multiparameter optimization (Sampei et al., 2013; Dengl et al., 2020; Furtmann et al., 2021; Root et al., 2021).
While some of the very high-throughput screening pipelines are dependent on advanced and automated instrumentation that is not readily available in most research labs, combinatorial bsAb panels for screening can also be generated through bioconjugation of individually expressed antibody components that are assembled to the final bsAb post-expression (Labrijn et al., 2014; Dengl et al., 2020; Hofmann et al., 2020). Practically, this allows construction of large bsAb panels without having to go through tedious production of each individual bsAb thus enabling screening of combinatorial binder formats.
Rational improvement of bsAb developability typically entails targeted optimization of the problematic fragment(s). Examples include engineering fragments for increased thermal stability (Miller et al., 2010; Von Kreudenstein et al., 2013; Boucher et al., 2023), solubility (Sampei et al., 2013), chemical stability (Sampei et al., 2013) and aggregation (Miller et al., 2010; Boucher et al., 2023). It is, however, important that optimized domains are subsequently re-evaluated in the context of the full length bsAb. Requirements for drug-like qualities can also be included already in the discovery, e.g., by including selective pressure for drug-like qualities in the screening process (Jespers et al., 2004).
Bispecific antibodies (bsAbs) represent a highly promising class of therapeutic modalities, demonstrating significant potential in various medical applications. The success of these molecules is highly dependent on efficient design and construction, which requires careful consideration to ensure optimal balance between therapeutic potency and favorable physicochemical properties. As previously demonstrated, the intricate interplay between the function and performance of bsAbs is intricately tied to their structural configuration. As discussed in this work, considerable efforts are directed towards the engineering of bsAbs with dual binding activity, while concurrently addressing the imperative need for developability profiles that align with, or even surpass, those of conventional monospecific antibodies. The comprehensive insights presented herein underscore the complexity of bsAb engineering, emphasizing the importance of strategic design to harness their full therapeutic potential. Notably, our exploration delves into the nuances of engineering strategies and practical considerations, shedding light on the challenges and opportunities inherent in efficient bsAb design. By dissecting the intricacies of these design principles, we contribute to the continued advancement of bsAbs as versatile and effective therapeutic agents, providing a roadmap for future research and development of improved bsAb therapeutics.
AM: Investigation, Validation, Visualization, Writing–original draft, Writing–review and editing. LP: Writing–review and editing. PK: Supervision, Writing–review and editing. SG: Conceptualization, Funding acquisition, Investigation, Supervision, Writing–original draft, Writing–review and editing.
The author(s) declare financial support was received for the research, authorship, and/or publication of this article. This work was supported by the Novo Nordisk Foundation Grant NNF19SA0056783, NNF19SA0057794, and NNF20SA0066621.
The authors declare that the research was conducted in the absence of any commercial or financial relationships that could be construed as a potential conflict of interest.
All claims expressed in this article are solely those of the authors and do not necessarily represent those of their affiliated organizations, or those of the publisher, the editors and the reviewers. Any product that may be evaluated in this article, or claim that may be made by its manufacturer, is not guaranteed or endorsed by the publisher.
Andrade, C., Arnold, L., Motabar, D., Aspelund, M., Tang, A., Hunter, A., et al. (2019). An integrated approach to aggregate control for therapeutic bispecific antibodies using an improved three column mab platform-like purification process. Biotechnol. Prog. 35, e2720. doi:10.1002/btpr.2720
Andres, F., Iamele, L., Meyer, T., Stüber, J. C., Kast, F., Gherardi, E., et al. (2019). Inhibition of the MET kinase activity and cell growth in MET-addicted cancer cells by Bi-paratopic linking. J. Mol. Biol. 431, 2020–2039. doi:10.1016/j.jmb.2019.03.024
Asano, R., Hosokawa, K., Taki, S., Konno, S., Shimomura, I., Ogata, H., et al. (2020). Build-up functionalization of anti-EGFR × anti-CD3 bispecific diabodies by integrating high-affinity mutants and functional molecular formats. Sci. Rep. 10, 4913. doi:10.1038/s41598-020-61840-3
Atwell, S., Ridgway, J. B. B., Wells, J. A., and Carter, P. (1997). Stable heterodimers from remodeling the domain interface of a homodimer using a phage display library. J. Mol. Biol. 270, 26–35. doi:10.1006/jmbi.1997.1116
Austin, R. J., Lemon, B. D., Aaron, W. H., Barath, M., Culp, P. A., DuBridge, R. B., et al. (2021). Tritacs, a novel class of t-cell–engaging protein constructs designed for the treatment of solid tumors. Mol. Cancer Ther. 20, 109–120. doi:10.1158/1535-7163.mct-20-0061
Bacac, M., Fauti, T., Sam, J., Colombetti, S., Weinzierl, T., Ouaret, D., et al. (2016a). A novel carcinoembryonic antigen T-cell bispecific antibody (CEA TCB) for the treatment of solid tumors. Clin. Cancer Res. Off. J. Am. Assoc. Cancer Res. 22, 3286–3297. doi:10.1158/1078-0432.ccr-15-1696
Bacac, M., Klein, C., and Umana, P. (2016b). CEA TCB: a novel head-to-tail 2:1 T cell bispecific antibody for treatment of CEA-positive solid tumors. Oncoimmunology 5, e1203498. doi:10.1080/2162402x.2016.1203498
Bai, X., Yi, M., Jiao, Y., Chu, Q., and Wu, K. (2019). Blocking TGF-β Signaling To Enhance The Efficacy Of Immune Checkpoint Inhibitor. OncoTargets Ther. 12, 9527–9538. doi:10.2147/ott.s224013
Bailly, M., Mieczkowski, C., Juan, V., Metwally, E., Tomazela, D., Baker, J., et al. (2020). Predicting antibody developability profiles through early stage discovery screening. Mabs 12, 1743053. doi:10.1080/19420862.2020.1743053
Beckmann, R., Jensen, K., Fenn, S., Speck, J., Krause, K., Meier, A., et al. (2021). DutaFabs are engineered therapeutic Fab fragments that can bind two targets simultaneously. Nat. Commun. 12, 708. doi:10.1038/s41467-021-20949-3
Bertz, M., Buchner, J., and Rief, M. (2013). Mechanical stability of the antibody domain CH3 homodimer in different oxidation states. J. Am. Chem. Soc. 135, 15085–15091. doi:10.1021/ja405076j
Bethani, I., Skånland, S. S., Dikic, I., and Acker-Palmer, A. (2010). Spatial organization of transmembrane receptor signalling. Embo J. 29, 2677–2688. doi:10.1038/emboj.2010.175
Blanco-Toribio, A., Sainz-Pastor, N., Álvarez-Cienfuegos, A., Merino, N., Cuesta, Á. M., Sánchez-Martín, D., et al. (2013). Generation and characterization of monospecific and bispecific hexavalent trimerbodies. Mabs 5, 70–79. doi:10.4161/mabs.22698
Bluemel, C., Hausmann, S., Fluhr, P., Sriskandarajah, M., Stallcup, W. B., Baeuerle, P. A., et al. (2010). Epitope distance to the target cell membrane and antigen size determine the potency of T cell-mediated lysis by BiTE antibodies specific for a large melanoma surface antigen. Cancer Immunol. Immunother. 59, 1197–1209. doi:10.1007/s00262-010-0844-y
Borensztajn, K., Peppelenbosch, M. P., and Spek, C. A. (2008). Factor Xa: at the crossroads between coagulation and signaling in physiology and disease. Trends Mol. Med. 14, 429–440. doi:10.1016/j.molmed.2008.08.001
Boucher, L. E., Prinslow, E. G., Feldkamp, M., Yi, F., Nanjunda, R., Wu, S. J., et al. (2023). “Stapling” scFv for multispecific biotherapeutics of superior properties. Mabs 15, 2195517. doi:10.1080/19420862.2023.2195517
Brinkmann, U., and Kontermann, R. E. (2017). The making of bispecific antibodies. Mabs 9, 182–212. doi:10.1080/19420862.2016.1268307
Burke, A., Borot, F., Du, X., Churchill, M., Ding, J., Grass, A. M., et al. (2023). A novel therapeutic bispecific format based on synthetic orthogonal heterodimers enables T cell activity against Acute myeloid leukemia. Oncogene 42, 26–34. doi:10.1038/s41388-022-02532-2
Cain, P., Huang, L., Tang, Y., Anguiano, V., and Feng, Y. (2023). Impact of IgG subclass on monoclonal antibody developability. Mabs 15, 2191302. doi:10.1080/19420862.2023.2191302
Cao, M., Wang, C., Chung, W. K., Motabar, D., Wang, J., Christian, E., et al. (2018). Characterization and analysis of scFv-IgG bispecific antibody size variants. Mabs 10, 1236–1247. doi:10.1080/19420862.2018.1505398
Chang, M. R., Tomasovic, L., Kuzmina, N. A., Ronk, A. J., Byrne, P. O., Johnson, R., et al. (2022). IgG-like bispecific antibodies with potent and synergistic neutralization against circulating SARS-CoV-2 variants of concern. Nat. Commun. 13, 5814. doi:10.1038/s41467-022-33030-4
Chen, S.-H., Dominik, P. K., Stanfield, J., Ding, S., Yang, W., Kurd, N., et al. (2021). Dual checkpoint blockade of CD47 and PD-L1 using an affinity-tuned bispecific antibody maximizes antitumor immunity. J. Immunother. Cancer 9, e003464. doi:10.1136/jitc-2021-003464
Chen, T., Han, J., Guo, G., Wang, Q., Wang, Y., and Li, Y. (2019). Monitoring removal of hole-hole homodimer by analytical hydrophobic interaction chromatography in purifying a bispecific antibody. Protein Expr. Purif. 164, 105457. doi:10.1016/j.pep.2019.105457
Chen, X., Zaro, J. L., and Shen, W. C. (2013). Fusion protein linkers: property, design and functionality. Adv. Drug Deliv. Rev. 65, 1357–1369. doi:10.1016/j.addr.2012.09.039
Chichili, G. R., Huang, L., Li, H., Burke, S., He, L., Tang, Q., et al. (2015). A CD3xCD123 bispecific DART for redirecting host T cells to myelogenous leukemia: preclinical activity and safety in nonhuman primates. Sci. Transl. Med. 7, 289ra82. doi:10.1126/scitranslmed.aaa5693
Chin, S. M., Kimberlin, C. R., Roe-Zurz, Z., Zhang, P., Xu, A., Liao-Chan, S., et al. (2018). Structure of the 4-1BB/4-1BBL complex and distinct binding and functional properties of utomilumab and urelumab. Nat. Commun. 9, 4679. doi:10.1038/s41467-018-07136-7
Ching, K. H., Berg, K., Reynolds, K., Pedersen, D., Macias, A., Abdiche, Y. N., et al. (2021). Common light chain chickens produce human antibodies of high affinity and broad epitope coverage for the engineering of bispecifics. Mabs 13, 1862451. doi:10.1080/19420862.2020.1862451
Choi, H.-J., Kim, Y.-J., Choi, D.-K., and Kim, Y.-S. (2015a). Engineering of immunoglobulin fc heterodimers using yeast surface-displayed combinatorial fc library screening. PloS One 10, e0145349. doi:10.1371/journal.pone.0145349
Choi, H. J., Kim, Y. J., Lee, S., and Kim, Y. S. (2013). A heterodimeric fc-Based bispecific antibody simultaneously targeting vegfr-2 and met exhibits potent antitumor activity. Mol. Cancer Ther. 12, 2748–2759. doi:10.1158/1535-7163.mct-13-0628
Choi, H. J., Seok, S. H., Kim, Y. J., Seo, M. D., and Kim, Y. S. (2015b). Crystal structures of immunoglobulin Fc heterodimers reveal the molecular basis for heterodimer formation. Mol. Immunol. 65, 377–383. doi:10.1016/j.molimm.2015.02.017
Choudhuri, K., Wiseman, D., Brown, M. H., Gould, K., and Van Der Merwe, P. A. (2005). T-cell receptor triggering is critically dependent on the dimensions of its peptide-MHC ligand. Nature 436, 578–582. doi:10.1038/nature03843
Christianson, G. J., Brooks, W., Vekasi, S., Manolfi, E. A., Niles, J., Roopenian, S. L., et al. (1997). Beta 2-microglobulin-deficient mice are protected from hypergammaglobulinemia and have defective antibody responses because of increased IgG catabolism. J. Immunol. 159, 4781–4792. doi:10.4049/jimmunol.159.10.4781
Compte, M., Harwood, S. L., Muñoz, I. G., Navarro, R., Zonca, M., Perez-Chacon, G., et al. (2018). A tumor-targeted trimeric 4-1BB-agonistic antibody induces potent anti-tumor immunity without systemic toxicity. Nat. Commun. 9, 4809. doi:10.1038/s41467-018-07195-w
Conrath, K. E., Lauwereys, M., Wyns, L., and Muyldermans, S. (2001). Camel single-domain antibodies as modular building units in bispecific and bivalent antibody constructs. J. Biol. Chem. 276, 7346–7350. doi:10.1074/jbc.m007734200
Cramer, D. A. T., Franc, V., Heidenreich, A. K., Hook, M., Adibzadeh, M., Reusch, D., et al. (2023). Characterization of high-molecular weight by-products in the production of a trivalent bispecific 2+1 heterodimeric antibody. Mabs 15, 2175312. doi:10.1080/19420862.2023.2175312
Croasdale, R., Wartha, K., Schanzer, J. M., Kuenkele, K. P., Ries, C., Mayer, K., et al. (2012). Development of tetravalent IgG1 dual targeting IGF-1R-EGFR antibodies with potent tumor inhibition. Arch. Biochem. Biophys. 526, 206–218. doi:10.1016/j.abb.2012.03.016
Datta-Mannan, A., Brown, R., Key, S., Cain, P., and Feng, Y. (2021). Pharmacokinetic developability and disposition profiles of bispecific antibodies: a case study with two molecules. Antibodies Basel Switz., 11. doi:10.3390/antib11010002
Datta-Mannan, A., Croy, J. E., Schirtzinger, L., Torgerson, S., Breyer, M., and Wroblewski, V. J. (2016). Aberrant bispecific antibody pharmacokinetics linked to liver sinusoidal endothelium clearance mechanism in cynomolgus monkeys. mAbs 8, 969–982. doi:10.1080/19420862.2016.1178435
Davis, J. H., Aperlo, C., Li, Y., Kurosawa, E., Lan, Y., Lo, K.-M., et al. (2010). SEEDbodies: fusion proteins based on strand-exchange engineered domain (SEED) CH3 heterodimers in an Fc analogue platform for asymmetric binders or immunofusions and bispecific antibodies. Protein Eng. Des. Sel. PEDS 23, 195–202. doi:10.1093/protein/gzp094
de Bruin, R. C. G., Veluchamy, J. P., Lougheed, S. M., Schneiders, F. L., Lopez-Lastra, S., Lameris, R., et al. (2018). A bispecific nanobody approach to leverage the potent and widely applicable tumor cytolytic capacity of Vγ9Vδ2-T cells. Oncoimmunology 7, e1375641. doi:10.1080/2162402x.2017.1375641
De Gasparo, R., Pedotti, M., Simonelli, L., Nickl, P., Muecksch, F., Cassaniti, I., et al. (2021). Bispecific IgG neutralizes SARS-CoV-2 variants and prevents escape in mice. Nature. 593 7859 424-428. doi:10.1038/s41586-021-03461-y
De Nardis, C., Hendriks, L. J. A., Poirier, E., Arvinte, T., Gros, P., Bakker, A. B. H., et al. (2017). A new approach for generating bispecific antibodies based on a common light chain format and the stable architecture of human immunoglobulin G1. J. Biol. Chem. 292, 14706–14717. doi:10.1074/jbc.m117.793497
Dengl, S., Mayer, K., Bormann, F., Duerr, H., Hoffmann, E., Nussbaum, B., et al. (2020). Format chain exchange (FORCE) for high-throughput generation of bispecific antibodies in combinatorial binder-format matrices. Nat. Commun. 11, 4974. doi:10.1038/s41467-020-18477-7
Deshaies, R. J. (2020). Multispecific drugs herald a new era of biopharmaceutical innovation. Nature 580, 329–338. doi:10.1038/s41586-020-2168-1
Dickopf, S., Georges, G. J., and Brinkmann, U. (2020). Format and geometries matter: structure-based design defines the functionality of bispecific antibodies. Comput. Struct. Biotechnol. J. 18, 1221–1227. doi:10.1016/j.csbj.2020.05.006
DiGiandomenico, A., Keller, A. E., Gao, C., Rainey, G. J., Warrener, P., Camara, M. M., et al. (2014). A multifunctional bispecific antibody protects against Pseudomonas aeruginosa. Sci. Transl. Med. 6, 262ra155. doi:10.1126/scitranslmed.3009655
Dillon, M., Yin, Y., Zhou, J., McCarty, L., Ellerman, D., Slaga, D., et al. (2017). Efficient production of bispecific IgG of different isotypes and species of origin in single mammalian cells. Mabs 9, 213–230. doi:10.1080/19420862.2016.1267089
Dimasi, N., Fleming, R., Sachsenmeier, K. F., Bezabeh, B., Hay, C., Wu, J., et al. (2017). Guiding bispecific monovalent antibody formation through proteolysis of IgG1 single-chain. Mabs 9, 438–454. doi:10.1080/19420862.2016.1277301
Ding, M., Shen, L., Xiao, L., Liu, X., and Hu, J. (2021). A cell line development strategy to improve a bispecific antibody expression purity in CHO cells. Biochem. Eng. J. 166, 107857. doi:10.1016/j.bej.2020.107857
Do, T. M., Capdevila, C., Pradier, L., Blanchard, V., Lopez-Grancha, M., Schussler, N., et al. (2020). Tetravalent bispecific tandem antibodies improve brain exposure and efficacy in an amyloid transgenic mouse model. Mol. Ther. - Methods Clin. Dev. 19, 58–77. doi:10.1016/j.omtm.2020.08.014
Dong, J., Sereno, A., Aivazian, D., Langley, E., Miller, B. R., Snyder, W. B., et al. (2011). A stable IgG-Like bispecific antibody targeting the epidermal growth factor receptor and the type I insulin-like growth factor receptor demonstrates superior anti-tumor activity. Mabs 3, 273–288. doi:10.4161/mabs.3.3.15188
Dovedi, S. J., Elder, M. J., Yang, C., Sitnikova, S. I., Irving, L., Hansen, A., et al. (2021). Design and efficacy of a monovalent bispecific PD-1/CTLA4 antibody that enhances CTLA4 blockade on PD-1(+) activated T cells. Cancer Discov. 11, 1100–1117. doi:10.1158/2159-8290.cd-20-1445
Edwards, C. J., Sette, A., Cox, C., Di Fiore, B., Wyre, C., Sydoruk, D., et al. (2022). The multi-specific VH-based Humabody CB213 co-targets PD1 and LAG3 on T cells to promote anti-tumour activity. Br. J. Cancer 126, 1168–1177. doi:10.1038/s41416-021-01684-4
Escobar-Cabrera, E., Lario, P., Baardsnes, J., Schrag, J., Durocher, Y., and Dixit, S. (2017). Asymmetric fc engineering for bispecific antibodies with reduced effector function. Antibodies 6, 7. doi:10.3390/antib6020007
Estes, B., Sudom, A., Gong, D., Whittington, D. A., Li, V., Mohr, C., et al. (2021). Next generation Fc scaffold for multispecific antibodies. Iscience 24, 103447. doi:10.1016/j.isci.2021.103447
Feige, M. J., Groscurth, S., Marcinowski, M., Shimizu, Y., Kessler, H., Hendershot, L. M., et al. (2009). An unfolded CH1 domain controls the assembly and secretion of IgG antibodies. Mol. Cell 34, 569–579. doi:10.1016/j.molcel.2009.04.028
Feige, M. J., Hendershot, L. M., and Buchner, J. (2010). How antibodies fold. Trends Biochem. Sci. 35, 189–198. doi:10.1016/j.tibs.2009.11.005
Fischer, N., Elson, G., Magistrelli, G., Dheilly, E., Fouque, N., Laurendon, A., et al. (2015). Exploiting light chains for the scalable generation and platform purification of native human bispecific IgG. Nat. Commun. 6, 6113. doi:10.1038/ncomms7113
Froning, K. J., Leaver-Fay, A., Wu, X., Phan, S., Gao, L., Huang, F., et al. (2017). Computational design of a specific heavy chain/κ light chain interface for expressing fully IgG bispecific antibodies. Protein Sci. 26, 2021–2038. doi:10.1002/pro.3240
Furtmann, N., Schneider, M., Spindler, N., Steinmann, B., Li, Z., Focken, I., et al. (2021). An end-to-end automated platform process for high-throughput engineering of next-generation multi-specific antibody therapeutics. Mabs 13, 1955433. doi:10.1080/19420862.2021.1955433
Garrett, J. T., Olivares, M. G., Rinehart, C., Granja-Ingram, N. D., Sánchez, V., Chakrabarty, A., et al. (2011). Transcriptional and posttranslational up-regulation of HER3 (ErbB3) compensates for inhibition of the HER2 tyrosine kinase. Proc. Natl. Acad. Sci. U. S. A. 108, 5021–5026. doi:10.1073/pnas.1016140108
Geuijen, C. A. W., De Nardis, C., Maussang, D., Rovers, E., Gallenne, T., Hendriks, L. J. A., et al. (2018). Unbiased combinatorial screening identifies a bispecific IgG1 that potently inhibits HER3 signaling via HER2-guided ligand blockade. Cancer Cell 33, 922–936.e10. doi:10.1016/j.ccell.2018.04.003
Ghetie, V., Hubbard, J. G., Kim, J. K., Tsen, M. F., Lee, Y., and Ward, E. S. (1996). Abnormally short serum half-lives of IgG in β2-microglobulin-deficient mice. Eur. J. Immunol. 26, 690–696. doi:10.1002/eji.1830260327
Godar, M., de Haard, H., Blanchetot, C., and Rasser, J. (2018). Therapeutic bispecific antibody formats: a patent applications review (1994-2017). Expert Opin. Ther. Pat. 28, 251–276. doi:10.1080/13543776.2018.1428307
Goletz, C., Lischke, T., Harnack, U., Schiele, P., Danielczyk, A., Rühmann, J., et al. (2018). Glyco-engineered anti-human programmed death-ligand 1 antibody mediates stronger CD8 T cell activation than its normal glycosylated and non-glycosylated counterparts. Front. Immunol. 9, 1614. doi:10.3389/fimmu.2018.01614
Gong, D., Riley, T. P., Bzymek, K. P., Correia, A. R., Li, D., Spahr, C., et al. (2021). Rational selection of building blocks for the assembly of bispecific antibodies. Mabs 13, 1870058. doi:10.1080/19420862.2020.1870058
Guling, C., Zhuxia, H., Deng, L., qing, M. X., Li, K., Xu, W., et al. (2022). Bispecific antibody simultaneously targeting PD1 and HER2 inhibits tumor growth via direct tumor cell killing in combination with PD1/PDL1 blockade and HER2 inhibition. Acta Pharmacol. Sin. 43, 672–680. doi:10.1038/s41401-021-00683-8
Gunasekaran, K., Pentony, M., Shen, M., Garrett, L., Forte, C., Woodward, A., et al. (2010). Enhancing antibody Fc heterodimer formation through electrostatic steering effects: applications to bispecific molecules and monovalent IgG. J. Biol. Chem. 285, 19637–19646. doi:10.1074/jbc.m110.117382
Ha, J. H., Kim, J. E., and Kim, Y. S. (2016). Immunoglobulin Fc heterodimer platform technology: from design to applications in therapeutic antibodies and proteins. Front. Immunol. 7, 394. doi:10.3389/fimmu.2016.00394
Haber, L., Olson, K., Kelly, M. P., Crawford, A., DiLillo, D. J., Tavaré, R., et al. (2021). Generation of T-cell-redirecting bispecific antibodies with differentiated profiles of cytokine release and biodistribution by CD3 affinity tuning. Sci. Rep. 11, 14397. doi:10.1038/s41598-021-93842-0
Hangiu, O., Compte, M., Dinesen, A., Navarro, R., Tapia-Galisteo, A., Mandrup, O. A., et al. (2022). Tumor targeted 4-1BB agonist antibody-albumin fusions with high affinity to FcRn induce anti-tumor immunity without toxicity. Iscience 25, 104958. doi:10.1016/j.isci.2022.104958
Harris, K. E., Lorentsen, K. J., Malik-Chaudhry, H. K., Loughlin, K., Basappa, H. M., Hartstein, S., et al. (2021). A bispecific antibody agonist of the IL-2 heterodimeric receptor preferentially promotes in vivo expansion of CD8 and NK cells. Sci. Rep. 11, 10592. doi:10.1038/s41598-021-90096-8
Hart, F., Danielczyk, A., and Goletz, S. (2017). Human cell line-derived monoclonal IgA antibodies for cancer immunotherapy. Bioeng. Basel Switz. 4, 42. doi:10.3390/bioengineering4020042
Harwood, S. L., Alvarez-Cienfuegos, A., Nuñez-Prado, N., Compte, M., Hernández-Pérez, S., Merino, N., et al. (2018). ATTACK, a novel bispecific T cell-recruiting antibody with trivalent EGFR binding and monovalent CD3 binding for cancer immunotherapy. Oncoimmunology 7, e1377874. doi:10.1080/2162402x.2017.1377874
Hendershot, L., Bole, D., Köhler, G., and Kearney, J. F. (1987). Assembly and secretion of heavy chains that do not associate posttranslationally with immunoglobulin heavy chain-binding protein. J. Cell Biol. 104, 761–767. doi:10.1083/jcb.104.3.761
Hmila, I., Saerens, D., Abderrazek, R. B., Vincke, C., Abidi, N., Benlasfar, Z., et al. (2010). A bispecific nanobody to provide full protection against lethal scorpion envenoming. Faseb J. 24, 3479–3489. doi:10.1096/fj.09-148213
Hoffmann, P., Hofmeister, R., Brischwein, K., Brandl, C., Crommer, S., Bargou, R., et al. (2005). Serial killing of tumor cells by cytotoxic T cells redirected with a CD19-/CD3-bispecific single-chain antibody construct. Int. J. Cancer 115, 98–104. doi:10.1002/ijc.20908
Hofmann, T., Schmidt, J., Ciesielski, E., Becker, S., Rysiok, T., Schütte, M., et al. (2020). Intein mediated high throughput screening for bispecific antibodies. mAbs 12, 1731938. doi:10.1080/19420862.2020.1731938
Huang, S., Segués, A., Hulsik, D. L., Zaiss, D. M., Sijts, AJAM, van Duijnhoven, S. M. J., et al. (2020). A novel efficient bispecific antibody format, combining a conventional antigen-binding fragment with a single domain antibody, avoids potential heavy-light chain mis-pairing. J. Immunol. Methods 483, 112811. doi:10.1016/j.jim.2020.112811
Ishiguro, T., Sano, Y., Komatsu, S. I., Kamata-Sakurai, M., Kaneko, A., Kinoshita, Y., et al. (2017). An anti-glypican 3/CD3 bispecific T cell-redirecting antibody for treatment of solid tumors. Sci. Transl. Med. 9, aal4291. doi:10.1126/scitranslmed.aal4291
Ishiwatari-Ogata, C., Kyuuma, M., Ogata, H., Yamakawa, M., Iwata, K., Ochi, M., et al. (2022). Ozoralizumab, a humanized anti-tnfα NANOBODY® compound, exhibits efficacy not only at the onset of arthritis in a human TNF transgenic mouse but also during secondary failure of administration of an anti-tnfα IgG. Front. Immunol. 13, 853008. doi:10.3389/fimmu.2022.853008
Jain, T., Sun, T., Durand, S., Hall, A., Houston, N. R., Nett, J. H., et al. (2017). Biophysical properties of the clinical-stage antibody landscape. Proc. Natl. Acad. Sci. U. S. A. 114, 944–949. doi:10.1073/pnas.1616408114
Jespers, L., Schon, O., Famm, K., and Winter, G. (2004). Aggregation-resistant domain antibodies selected on phage by heat denaturation. Nat. Biotechnol. 22, 1161–1165. doi:10.1038/nbt1000
Johnson, S., Burke, S., Huang, L., Gorlatov, S., Li, H., Wang, W., et al. (2010). Effector cell recruitment with novel Fv-based dual-affinity re-targeting protein leads to potent tumor cytolysis and in vivo B-cell depletion. J. Mol. Biol. 399, 436–449. doi:10.1016/j.jmb.2010.04.001
Joshi, K. K., Phung, W., Han, G., Yin, Y., Kim, I., Sandoval, W., et al. (2019). Elucidating heavy/light chain pairing preferences to facilitate the assembly of bispecific IgG in single cells. Mabs 11, 1254–1265. doi:10.1080/19420862.2019.1640549
Kareva, I., Zutshi, A., Gupta, P., and Kabilan, S. (2021). Bispecific antibodies: a guide to model informed drug discovery and development. Heliyon 7, e07649. doi:10.1016/j.heliyon.2021.e07649
Kareva, I., Zutshi, A., and Kabilan, S. (2018). Guiding principles for mechanistic modeling of bispecific antibodies. Prog. Biophys. Mol. Biol. 139, 59–72. doi:10.1016/j.pbiomolbio.2018.08.011
Kast, F., Schwill, M., Stüber, J. C., Pfundstein, S., Nagy-Davidescu, G., Rodríguez, J. M. M., et al. (2021). Engineering an anti-HER2 biparatopic antibody with a multimodal mechanism of action. Nat. Commun. 12, 3790. doi:10.1038/s41467-021-23948-6
Kienast, Y., Klein, C., Scheuer, W., Raemsch, R., Lorenzon, E., Bernicke, D., et al. (2013). Ang-2-VEGF-A CrossMab, a novel bispecific human IgG1 antibody blocking VEGF-A and Ang-2 functions simultaneously, mediates potent antitumor, antiangiogenic, and antimetastatic efficacy. Clin. Cancer Res. Off. J. Am. Assoc. Cancer Res. 19, 6730–6740. doi:10.1158/1078-0432.ccr-13-0081
Koga, H., Yamano, T., Betancur, J., Nagatomo, S., Ikeda, Y., Yamaguchi, K., et al. (2023). Efficient production of bispecific antibody by FAST-IgTM and its application to NXT007 for the treatment of hemophilia A. Mabs 15, 2222441. doi:10.1080/19420862.2023.2222441
Kraman, M., Faroudi, M., Allen, N. L., Kmiecik, K., Gliddon, D., Seal, C., et al. (2020). FS118, a bispecific antibody targeting LAG-3 and PD-L1, enhances T-cell activation resulting in potent antitumor activity. Clin. Cancer Res. Off. J. Am. Assoc. Cancer Res. 26, 3333–3344. doi:10.1158/1078-0432.ccr-19-3548
Krogsgaard, M., and Davis, M. M. (2005). How T cells “see” antigen. Nat. Immunol. 6, 239–245. doi:10.1038/ni1173
Kühl, L., Aschmoneit, N., Kontermann, R. E., and Seifert, O. (2022). The eIg technology to generate Ig-like bispecific antibodies. Mabs 14, 2063043. doi:10.1080/19420862.2022.2063043
Kühl, L., Schäfer, A. K., Kraft, S., Aschmoneit, N., Kontermann, R. E., and Seifert, O. (2023). eIg-based bispecific T-cell engagers targeting EGFR: format matters. Mabs 15, 2183540. doi:10.1080/19420862.2023.2183540
Kuo, S. R., Wong, L., and Liu, J. S. (2012). Engineering a CD123xCD3 bispecific scFv immunofusion for the treatment of leukemia and elimination of leukemia stem cells. Protein Eng. Des. Sel. 25, 561–570. doi:10.1093/protein/gzs040
Labrijn, A. F., Janmaat, M. L., Reichert, J. M., and Parren, PWHI (2019). Bispecific antibodies: a mechanistic review of the pipeline. Nat. Rev. Drug Discov. 18, 585–608. doi:10.1038/s41573-019-0028-1
Labrijn, A. F., Meesters, J. I., De Goeij, BECG, Van Den Bremer, E. T. J., Neijssen, J., Van Kampen, M. D., et al. (2013). Efficient generation of stable bispecific IgG1 by controlled Fab-arm exchange. Proc. Natl. Acad. Sci. U. S. A. 110, 5145–5150. doi:10.1073/pnas.1220145110
Labrijn, A. F., Meesters, J. I., Priem, P., de Jong, R. N., van den Bremer, E. T. J., van Kampen, M. D., et al. (2014). Controlled Fab-arm exchange for the generation of stable bispecific IgG1. Nat. Protoc. 9, 2450–2463. doi:10.1038/nprot.2014.169
Lauritzen, B., Bjelke, M., Björkdahl, O., Bloem, E., Keane, K., Kjalke, M., et al. (2022). A novel next-generation FVIIIa mimetic, Mim8, has a favorable safety profile and displays potent pharmacodynamic effects: results from safety studies in cynomolgus monkeys. J. Thromb. Haemost. 20, 1312–1324. doi:10.1111/jth.15682
Leaver-Fay, A., Froning, K. J., Atwell, S., Aldaz, H., Pustilnik, A., Lu, F., et al. (2016). Computationally designed bispecific antibodies using negative state repertoires. Structure 24, 641–651. doi:10.1016/j.str.2016.02.013
Lee, H. Y., Contreras, E., Register, A. C., Wu, Q., Abadie, K., Garcia, K., et al. (2019). Development of a bioassay to detect T-cell-activating impurities for T-cell-dependent bispecific antibodies. Sci. Rep. 9, 3900. doi:10.1038/s41598-019-40689-1
Lee, J. Y., Lee, H. T., Shin, W., Chae, J., Choi, J., Kim, S. H., et al. (2016). Structural basis of checkpoint blockade by monoclonal antibodies in cancer immunotherapy. Nat. Commun. 7, 13354. doi:10.1038/ncomms13354
Lewis, S. M., Wu, X., Pustilnik, A., Sereno, A., Huang, F., Rick, H. L., et al. (2014). Generation of bispecific IgG antibodies by structure-based design of an orthogonal Fab interface. Nat. Biotechnol. 32, 191–198. doi:10.1038/nbt.2797
Li, J., Stagg, N. J., Johnston, J., Harris, M. J., Menzies, S. A., DiCara, D., et al. (2017). Membrane-proximal epitope facilitates efficient T cell synapse formation by anti-FcRH5/CD3 and is a requirement for myeloma cell killing. Cancer Cell 31, 383–395. doi:10.1016/j.ccell.2017.02.001
Li, L., Deng, L., Meng, X., Gu, C., Meng, L., Li, K., et al. (2021). Tumor-targeting anti-EGFR x anti-PD1 bispecific antibody inhibits EGFR-overexpressing tumor growth by combining EGFR blockade and immune activation with direct tumor cell killing. Transl. Oncol. 14, 100916. doi:10.1016/j.tranon.2020.100916
Li, Y. (2019). A brief introduction of IgG-like bispecific antibody purification: methods for removing product-related impurities. Protein Expr. Purif. 155, 112–119. doi:10.1016/j.pep.2018.11.011
Liu, H., Saxena, A., Sidhu, S. S., and Wu, D. (2017). Fc engineering for developing therapeutic bispecific antibodies and novel scaffolds. Front. Immunol. 8, 38. doi:10.3389/fimmu.2017.00038
Liu, H. F., Ma, J., Winter, C., and Bayer, R. (2010). Recovery and purification process development for monoclonal antibody production. Mabs 2, 480–499. doi:10.4161/mabs.2.5.12645
Liu, R., Oldham, R. J., Teal, E., Beers, S. A., and Cragg, M. S. (2020). Fc-engineering for modulated effector functions-improving antibodies for cancer treatment. Antibodies Basel Switz. 9, 64. doi:10.3390/antib9040064
Liu, Z., Leng, E. C., Gunasekaran, K., Pentony, M., Shen, M., Howard, M., et al. (2015). A novel antibody engineering strategy for making monovalent bispecific heterodimeric IgG antibodies by electrostatic steering mechanism. J. Biol. Chem. 290, 7535–7562. doi:10.1074/jbc.m114.620260
Ljungars, A., Schiött, T., Mattson, U., Steppa, J., Hambe, B., Semmrich, M., et al. (2020). A bispecific IgG format containing four independent antigen binding sites. Sci. Rep. 10, 1546. doi:10.1038/s41598-020-58150-z
Löffler, A., Kufer, P., Lutterbüse, R., Zettl, F., Daniel, P. T., Schwenkenbecher, J. M., et al. (2000). A recombinant bispecific single-chain antibody, CD19 × CD3, induces rapid and high lymphoma-directed cytotoxicity by unstimulated T lymphocytes. Blood 95, 2098–2103. doi:10.1182/blood.v95.6.2098
Lu, L. L., Suscovich, T. J., Fortune, S. M., and Alter, G. (2018). Beyond binding: antibody effector functions in infectious diseases. Nat. Rev. Immunol. 18, 46–61. doi:10.1038/nri.2017.106
Lu, Z., Liu, Z., Li, X., Qin, X., Hong, H., Zhou, Z., et al. (2022). Nanobody-based bispecific neutralizer for shiga toxin-producing E. coli. Acs Infect. Dis. 8, 321–329. doi:10.1021/acsinfecdis.1c00456
Mack, M., Riethmüller, G., and Kufer, P. (1995). A small bispecific antibody construct expressed as a functional single-chain molecule with high tumor cell cytotoxicity. Proc. Natl. Acad. Sci. U. S. A. 92, 7021–7025. doi:10.1073/pnas.92.15.7021
Madsen, A. V., Kristensen, P., Buell, A. K., and Goletz, S. (2023). Generation of robust bispecific antibodies through fusion of single-domain antibodies on IgG scaffolds: a comprehensive comparison of formats. Mabs 15, 2189432. doi:10.1080/19420862.2023.2189432
Madsen, A. V., Mejias-Gomez, O., Pedersen, L. E., Skovgaard, K., Kristensen, P., and Goletz, S. (2022). Immobilization-free binding and affinity characterization of higher order bispecific antibody complexes using size-based microfluidics. Anal. Chem. 94, 13652–13658. doi:10.1021/acs.analchem.2c02705
Magistrelli, G., Poitevin, Y., Schlosser, F., Pontini, G., Malinge, P., Josserand, S., et al. (2017). Optimizing assembly and production of native bispecific antibodies by codon de-optimization. Mabs 9, 231–239. doi:10.1080/19420862.2016.1267088
Mahlangu, J., Oldenburg, J., Paz-Priel, I., Negrier, C., Niggli, M., Mancuso, M. E., et al. (2018). Emicizumab prophylaxis in patients who have hemophilia a without inhibitors. N. Engl. J. Med. 379, 811–822. doi:10.1056/nejmoa1803550
Mandrup, O. A., Ong, S. C., Lykkemark, S., Dinesen, A., Rudnik-Jansen, I., Dagnæs-Hansen, N. F., et al. (2021). Programmable half-life and anti-tumour effects of bispecific T-cell engager-albumin fusions with tuned FcRn affinity. Commun. Biol. 4, 310. doi:10.1038/s42003-021-01790-2
Mayes, P. A., Hance, K. W., and Hoos, A. (2018). The promise and challenges of immune agonist antibody development in cancer. Nat. Rev. Drug Discov. 17, 509–527. doi:10.1038/nrd.2018.75
Mazor, Y., Hansen, A., Yang, C., Chowdhury, P. S., Wang, J., Stephens, G., et al. (2015a). Insights into the molecular basis of a bispecific antibody’s target selectivity. Mabs 7, 461–469. doi:10.1080/19420862.2015.1022695
Mazor, Y., Oganesyan, V., Yang, C., Hansen, A., Wang, J., Liu, H., et al. (2015b). Improving target cell specificity using a novel monovalent bispecific IgG design. Mabs 7, 377–389. doi:10.1080/19420862.2015.1007816
Mazor, Y., Sachsenmeier, K. F., Yang, C., Hansen, A., Filderman, J., Mulgrew, K., et al. (2017). Enhanced tumor-targeting selectivity by modulating bispecific antibody binding affinity and format valence. Sci. Rep. 7, 40098. doi:10.1038/srep40098
McDonagh, C. F., Huhalov, A., Harms, B. D., Adams, S., Paragas, V., Oyama, S., et al. (2012). Antitumor activity of a novel bispecific antibody that targets the ErbB2/ErbB3 oncogenic unit and inhibits heregulin-induced activation of ErbB3. Mol. Cancer Ther. 11, 582–593. doi:10.1158/1535-7163.mct-11-0820
Mei, L., Zappala, F., and Tsourkas, A. (2022). Rapid production of bispecific antibodies from off-the-shelf IgGs with high yield and purity. Bioconjug Chem. 33, 134–141. doi:10.1021/acs.bioconjchem.1c00476
Merchant, A. M., Zhu, Z., Yuan, J. Q., Goddard, A., Adams, C. W., Presta, L. G., et al. (1998). An efficient route to human bispecific IgG. Nat. Biotechnol. 16, 677–681. doi:10.1038/nbt0798-677
Metz, S., Panke, C., Haas, A. K., Schanzer, J., Lau, W., Croasdale, R., et al. (2012). Bispecific antibody derivatives with restricted binding functionalities that are activated by proteolytic processing. Protein Eng. Des. Sel. PEDS 25, 571–580. doi:10.1093/protein/gzs064
Mieczkowski, C., Zhang, X., Lee, D., Nguyen, K., Lv, W., Wang, Y., et al. (2023). Blueprint for antibody biologics developability. Mabs 15, 2185924. doi:10.1080/19420862.2023.2185924
Miller, B. R., Demarest, S. J., Lugovskoy, A., Huang, F., Wu, X., Snyder, W. B., et al. (2010). Stability engineering of scFvs for the development of bispecific and multivalent antibodies. Protein Eng. Des. Sel. PEDS 23, 549–557. doi:10.1093/protein/gzq028
Milutinovic, S., Kashyap, A. K., Yanagi, T., Wimer, C., Zhou, S., O’Neil, R., et al. (2016). Dual agonist surrobody simultaneously activates death receptors DR4 and DR5 to induce cancer cell death. Mol. Cancer Ther. 15, 114–124. doi:10.1158/1535-7163.mct-15-0400
Misson Mindrebo, L., Liu, H., Ozorowski, G., Tran, Q., Woehl, J., Khalek, I., et al. (2023). Fully synthetic platform to rapidly generate tetravalent bispecific nanobody-based immunoglobulins. Proc. Natl. Acad. Sci. U. S. A. 120, e2216612120. doi:10.1073/pnas.2216612120
Moore, G. L., Bautista, C., Pong, E., Nguyen, D. H. T., Jacinto, J., Eivazi, A., et al. (2011a). A novel bispecific antibody format enables simultaneous bivalent and monovalent co-engagement of distinct target antigens. Mabs 3, 546–557. doi:10.4161/mabs.3.6.18123
Moore, G. L., Lee, S.-H., Schubbert, S., Miranda, Y., Rashid, R., Pong, E., et al. (2015). Tuning T cell affinity improves efficacy and safety of anti-CD38 × anti-CD3 bispecific antibodies in monkeys - a potential therapy for multiple myeloma. Blood 126, 1798. doi:10.1182/blood.v126.23.1798.1798
Moore, P. A., Zhang, W., Rainey, G. J., Burke, S., Li, H., Huang, L., et al. (2011b). Application of dual affinity retargeting molecules to achieve optimal redirected T-cell killing of B-cell lymphoma. Blood 117, 4542–4551. doi:10.1182/blood-2010-09-306449
Müller, T., Tasser, C., Tesar, M., Fucek, I., Schniegler-Mattox, U., Koch, J., et al. (2023). Selection of bispecific antibodies with optimal developability using FcRn-Ph-HPLC as an optimized FcRn affinity chromatography method. mAbs 15, 2245519. doi:10.1080/19420862.2023.2245519
Muto, A., Yoshihashi, K., Takeda, M., Kitazawa, T., Soeda, T., Igawa, T., et al. (2014). Anti-factor IXa/X bispecific antibody ACE910 prevents joint bleeds in a long-term primate model of acquired hemophilia A. Blood 124, 3165–3171. doi:10.1182/blood-2014-07-585737
Nesspor, T. C., Kinealy, K., Mazzanti, N., Diem, M. D., Boye, K., Hoffman, H., et al. (2020). High-throughput generation of bipod (fab × scFv) bispecific antibodies exploits differential chain expression and affinity capture. Sci. Rep. 10, 7557. doi:10.1038/s41598-020-64536-w
Offner, S., Hofmeister, R., Romaniuk, A., Kufer, P., and Baeuerle, P. A. (2006). Induction of regular cytolytic T cell synapses by bispecific single-chain antibody constructs on MHC class I-negative tumor cells. Mol. Immunol. 43, 763–771. doi:10.1016/j.molimm.2005.03.007
Ong, H. K., Nguyen, N. T. B., Bi, J., and Yang, Y. (2022). Vector design for enhancing expression level and assembly of knob-into-hole based FabscFv-Fc bispecific antibodies in CHO cells. Antib. Ther. 5, 288–300. doi:10.1093/abt/tbac025
Orcutt, K. D., Ackerman, M. E., Cieslewicz, M., Quiroz, E., Slusarczyk, A. L., Frangioni, J. V., et al. (2010). A modular IgG-scFv bispecific antibody topology. Protein Eng. Des. Sel. 23, 221–228. doi:10.1093/protein/gzp077
Østergaard, H., Lund, J., Greisen, P. J., Kjellev, S., Henriksen, A., Lorenzen, N., et al. (2021). A factor VIIIa–mimetic bispecific antibody, Mim8, ameliorates bleeding upon severe vascular challenge in hemophilia A mice. Blood 138, 1258–1268. doi:10.1182/blood.2020010331
Pang, X., Huang, Z., Zhong, T., Zhang, P., Wang, Z. M., Xia, M., et al. (2023). Cadonilimab, a tetravalent PD-1/CTLA-4 bispecific antibody with trans-binding and enhanced target binding avidity. Mabs 15, 2180794. doi:10.1080/19420862.2023.2180794
Pomarici, N. D., Fernández-Quintero, M. L., Quoika, P. K., Waibl, F., Bujotzek, A., Georges, G., et al. (2022). Bispecific antibodies-effects of point mutations on CH3-CH3 interface stability. Protein Eng. Des. Sel. PEDS 35, gzac012. doi:10.1093/protein/gzac012
Raybould, M. I. J., Marks, C., Krawczyk, K., Taddese, B., Nowak, J., Lewis, A. P., et al. (2019). Five computational developability guidelines for therapeutic antibody profiling. Proc. Natl. Acad. Sci. U. S. A. 116, 4025–4030. doi:10.1073/pnas.1810576116
Reusch, U., Burkhardt, C., Fucek, I., Le Gall, F., Le Gall, M., Hoffmann, K., et al. (2014). A novel tetravalent bispecific TandAb (CD30/CD16A) efficiently recruits NK cells for the lysis of CD30+ tumor cells. Mabs 6, 727–738. doi:10.4161/mabs.28591
Reusch, U., Duell, J., Ellwanger, K., Herbrecht, C., Knackmuss, S. H. J., Fucek, I., et al. (2015). A tetravalent bispecific TandAb (CD19/CD3), AFM11, efficiently recruits T cells for the potent lysis of CD19+ tumor cells. Mabs 7, 584–604. doi:10.1080/19420862.2015.1029216
Rhoden, J. J., Dyas, G. L., and Wroblewski, V. J. (2016). A modeling and experimental investigation of the effects of antigen density, binding affinity, and antigen expression ratio on bispecific antibody binding to cell surface targets. J. Biol. Chem. 291, 11337–11347. doi:10.1074/jbc.m116.714287
Ridgway, J. B., Presta, L. G., and Carter, P. (1996). “Knobs-into-holes” engineering of antibody CH3 domains for heavy chain heterodimerization. Protein Eng. 9, 617–621. doi:10.1093/protein/9.7.617
Rispens, T., Ooijevaar-de Heer, P., Bende, O., and Aalberse, R. C. (2011). Mechanism of immunoglobulin G4 Fab-arm exchange. J. Am. Chem. Soc. 133, 10302–10311. doi:10.1021/ja203638y
Robert, C. (2020). A decade of immune-checkpoint inhibitors in cancer therapy. Nat. Commun. 11, 3801. doi:10.1038/s41467-020-17670-y
Root, A. R., Cao, W., Li, B., LaPan, P., Meade, C., Sanford, J., et al. (2016). Development of PF-06671008, a highly potent anti-P-cadherin/anti-CD3 bispecific DART molecule with extended half-life for the treatment of cancer. Antibodies 5, 6. doi:10.3390/antib5010006
Root, A. R., Guntas, G., Katragadda, M., Apgar, J. R., Narula, J., Chang, C. S., et al. (2021). Discovery and optimization of a novel anti-GUCY2c x CD3 bispecific antibody for the treatment of solid tumors. Mabs 13, 1850395. doi:10.1080/19420862.2020.1850395
Rozan, C., Cornillon, A., Petiard, C., Chartier, M., Behar, G., Boix, C., et al. (2013). Single-domain antibody–based and linker-free bispecific antibodies targeting FcγRIII induce potent antitumor activity without recruiting regulatory T cells. Mol. Cancer Ther. 12, 1481–1491. doi:10.1158/1535-7163.mct-12-1012
Sampei, Z., Igawa, T., Soeda, T., Okuyama-Nishida, Y., Moriyama, C., Wakabayashi, T., et al. (2013). Identification and multidimensional optimization of an asymmetric bispecific IgG antibody mimicking the function of factor VIII cofactor activity. Plos One 8, e57479. doi:10.1371/journal.pone.0057479
Santich, B. H., Park, J. A., Tran, H., Guo, H.-F., Huse, M., and Cheung, N.-K. V. (2020). Interdomain spacing and spatial configuration drive the potency of IgG-[L]-scFv T cell bispecific antibodies. Sci. Transl. Med. 12, eaax1315. doi:10.1126/scitranslmed.aax1315
Saunders, K. O. (2019). Conceptual approaches to modulating antibody effector functions and circulation half-life. Front. Immunol. 10, 1296. doi:10.3389/fimmu.2019.01296
Schachner, L., Han, G., Dillon, M., Zhou, J., McCarty, L., Ellerman, D., et al. (2016). Characterization of chain pairing variants of bispecific IgG expressed in a single host cell by high-resolution native and denaturing mass spectrometry. Anal. Chem. 88, 12122–12127. doi:10.1021/acs.analchem.6b02866
Schaefer, W., Regula, J. T., Bähner, M., Schanzer, J., Croasdale, R., Dürr, H., et al. (2011). Immunoglobulin domain crossover as a generic approach for the production of bispecific IgG antibodies. Proc. Natl. Acad. Sci. U. S. A. 108, 11187–11192. doi:10.1073/pnas.1019002108
Schanzer, J. M., Wartha, K., Croasdale, R., Moser, S., Künkele, K. P., Ries, C., et al. (2014). A novel glycoengineered bispecific antibody format for targeted inhibition of epidermal growth factor receptor (EGFR) and insulin-like growth factor receptor type i (IGF-1R) demonstrating unique molecular properties. J. Biol. Chem. 289, 18693–18706. doi:10.1074/jbc.m113.528109
Segaliny, A. I., Jayaraman, J., Chen, X., Chong, J., Luxon, R., Fung, A., et al. (2023). A high throughput bispecific antibody discovery pipeline. Commun. Biol. 6, 380. doi:10.1038/s42003-023-04746-w
Shima, M., Hanabusa, H., Taki, M., Matsushita, T., Sato, T., Fukutake, K., et al. (2016). Factor VIII-mimetic function of humanized bispecific antibody in hemophilia A. N. Engl. J. Med. 374, 2044–2053. doi:10.1056/nejmoa1511769
Shiraiwa, H., Narita, A., Kamata-Sakurai, M., Ishiguro, T., Sano, Y., Hironiwa, N., et al. (2019). Engineering a bispecific antibody with a common light chain: identification and optimization of an anti-CD3 epsilon and anti-GPC3 bispecific antibody, ERY974. Methods 154, 10–20. doi:10.1016/j.ymeth.2018.10.005
Skegro, D., Stutz, C., Ollier, R., Svensson, E., Wassmann, P., Bourquin, F., et al. (2017). Immunoglobulin domain interface exchange as a platform technology for the generation of Fc heterodimers and bispecific antibodies. J. Biol. Chem. 292, 9745–9759. doi:10.1074/jbc.m117.782433
Spiess, C., Merchant, M., Huang, A., Zheng, Z., Yang, N.-Y., Peng, J., et al. (2013). Bispecific antibodies with natural architecture produced by co-culture of bacteria expressing two distinct half-antibodies. Nat. Biotechnol. 31, 753–758. doi:10.1038/nbt.2621
Spiess, C., Zhai, Q., and Carter, P. J. (2015). Alternative molecular formats and therapeutic applications for bispecific antibodies. Mol. Immunol. 67, 95–106. doi:10.1016/j.molimm.2015.01.003
Strop, P., Ho, W. H., Boustany, L. M., Abdiche, Y. N., Lindquist, K. C., Farias, S. E., et al. (2012). Generating bispecific human IgG1 and IgG2 antibodies from any antibody pair. J. Mol. Biol. 420, 204–219. doi:10.1016/j.jmb.2012.04.020
Stutz, C., and Blein, S. (2020). A single mutation increases heavy-chain heterodimer assembly of bispecific antibodies by inducing structural disorder in one homodimer species. J. Biol. Chem. 295, 9392–9408. doi:10.1074/jbc.ra119.012335
Svilenov, H. L., Arosio, P., Menzen, T., Tessier, P., and Sormanni, P. (2023). Approaches to expand the conventional toolbox for discovery and selection of antibodies with drug-like physicochemical properties. Mabs 15, 2164459. doi:10.1080/19420862.2022.2164459
Swope, N., Chung, W. K., Cao, M., Motabar, D., Liu, D., Ahuja, S., et al. (2020). Impact of enzymatic reduction on bivalent bispecific antibody fragmentation and loss of product purity upon reoxidation. Biotechnol. Bioeng. 117, 1063–1071. doi:10.1002/bit.27264
Tang, J., Zhang, X., Chen, T., Wang, Y., and Li, Y. (2020). Removal of half antibody, hole-hole homodimer and aggregates during bispecific antibody purification using MMC ImpRes mixed-mode chromatography. Protein Expr. Purif. 167, 105529. doi:10.1016/j.pep.2019.105529
Tang, Y., Cain, P., Anguiano, V., Shih, J. J., Chai, Q., and Feng, Y. (2021). Impact of IgG subclass on molecular properties of monoclonal antibodies. Mabs 13, 1993768. doi:10.1080/19420862.2021.1993768
Von Kreudenstein, T. S., Escobar-Carbrera, E., Lario, P. I., D’Angelo, I., Brault, K., Kelly, J., et al. (2013). Improving biophysical properties of a bispecific antibody scaffold to aid developability: quality by molecular design. mAbs 5, 646–654. doi:10.4161/mabs.25632
Wagner, K., Kwakkenbos, M. J., Claassena, Y. B., Maijoor, K., Böhne, M., Van Der Sluijs, K. F., et al. (2014). Bispecific antibody generated with sortase and click chemistry has broad antiinfluenza virus activity. Proc. Natl. Acad. Sci. U. S. A. 111, 16820–16825. doi:10.1073/pnas.1408605111
Walter, J. D., Scherer, M., Hutter, C. A. J., Garaeva, A. A., Zimmermann, I., Wyss, M., et al. (2022). Biparatopic sybodies neutralize SARS-CoV-2 variants of concern and mitigate drug resistance. Embo Rep. 23, e54199. doi:10.15252/embr.202154199
Weatherill, E. E., Cain, K. L., Heywood, S. P., Compson, J. E., Heads, J. T., Adams, R., et al. (2012). Towards a universal disulphide stabilised single chain Fv format: importance of interchain disulphide bond location and vLvH orientation. Protein Eng. Des. Sel. 25, 321–329. doi:10.1093/protein/gzs021
Wec, A. Z., Nyakatura, E. K., Herbert, A. S., Howell, K. A., Holtsberg, F. W., Bakken, R. R., et al. (2016). A “Trojan horse” bispecific-antibody strategy for broad protection against ebolaviruses. Science 354, 350–354. doi:10.1126/science.aag3267
Wei, J., Yang, Y., Wang, G., and Liu, M. (2022). Current landscape and future directions of bispecific antibodies in cancer immunotherapy. Front. Immunol. 13, 1035276. doi:10.3389/fimmu.2022.1035276
Weisser, N. E., Sanches, M., Escobar-Cabrera, E., O’Toole, J., Whalen, E., Chan, P. W. Y., et al. (2023). An anti-HER2 biparatopic antibody that induces unique HER2 clustering and complement-dependent cytotoxicity. Nat. Commun. 14, 1394. doi:10.1038/s41467-023-37029-3
Wörn, A., and Plückthun, A. (2001). Stability engineering of antibody single-chain Fv fragments. J. Mol. Biol. 305, 989–1010. doi:10.1006/jmbi.2000.4265
Wozniak-Knopp, G., Stadlmayr, G., Perthold, J. W., Stadlbauer, K., Gotsmy, M., Becker, S., et al. (2018). An antibody with fab-constant domains exchanged for a pair of CH3 domains. Plos One 13, e0195442. doi:10.1371/journal.pone.0195442
Wranik, B. J., Christensen, E. L., Schaefer, G., Jackman, J. K., Vendel, A. C., and Eaton, D. (2012). LUZ-Y, a novel platform for the mammalian cell production of full-length IgG-bispecific antibodies. J. Biol. Chem. 287, 43331–43339. doi:10.1074/jbc.m112.397869
Wu, C., Ying, H., Bose, S., Miller, R., Medina, L., Santora, L., et al. (2009). Molecular construction and optimization of anti-human IL-1α/β dual variable domain immunoglobulin (DVD-IgTM) molecules. Mabs 1, 339–347. doi:10.4161/mabs.1.4.8755
Wu, C., Ying, H., Grinnell, C., Bryant, S., Miller, R., Clabbers, A., et al. (2007). Simultaneous targeting of multiple disease mediators by a dual-variable-domain immunoglobulin. Nat. Biotechnol. 25, 1290–1297. doi:10.1038/nbt1345
Wu, X., Sereno, A. J., Huang, F., Zhang, K., Batt, M., Fitchett, J. R., et al. (2015). Protein design of IgG/TCR chimeras for the co-expression of Fab-like moieties within bispecific antibodies. Mabs 7, 364–376. doi:10.1080/19420862.2015.1007826
Wuellner, U., Klupsch, K., Buller, F., Attinger-Toller, I., Santimari, R., Zbinden, I., et al. (2015). Bispecific CD3/HER2 targeting FynomAb induces redirected t cell-mediated cytolysis with high potency and enhanced tumor selectivity. Antibodies 4, 426–440. doi:10.3390/antib4040426
Xiong, C., Mao, Y., Wu, T., Kang, N., Zhao, M., Di, R., et al. (2018). Optimized expression and characterization of a novel fully human bispecific single-chain diabody targeting vascular endothelial growth factor165 and programmed death-1 in Pichia pastoris and evaluation of antitumor activity in vivo. Int. J. Mol. Sci. 19, 2900. doi:10.3390/ijms19102900
Yan, Y., Xing, T., Wang, S., Daly, T. J., and Li, N. (2019). Coupling mixed-mode size exclusion chromatography with native mass spectrometry for sensitive detection and quantitation of homodimer impurities in bispecific IgG. Anal. Chem. 91, 11417–11424. doi:10.1021/acs.analchem.9b02793
Yanakieva, D., Pekar, L., Evers, A., Fleischer, M., Keller, S., Mueller-Pompalla, D., et al. (2022). Beyond bispecificity: controlled Fab arm exchange for the generation of antibodies with multiple specificities. Mabs 14, 2018960. doi:10.1080/19420862.2021.2018960
Yi, M., Zhang, J., Li, A., Niu, M., Yan, Y., Jiao, Y., et al. (2021). The construction, expression, and enhanced anti-tumor activity of YM101: a bispecific antibody simultaneously targeting TGF-β and PD-L1. J. Hematol. OncolJ Hematol. Oncol. 14, 27. doi:10.1186/s13045-021-01045-x
Yin, Y., Han, G., Zhou, J., Dillon, M., McCarty, L., Gavino, L., et al. (2016). Precise quantification of mixtures of bispecific IgG produced in single host cells by liquid chromatography-Orbitrap high-resolution mass spectrometry. Mabs 8, 1467–1476. doi:10.1080/19420862.2016.1232217
Zhang, F., Zhang, J., Liu, M., Zhao, L., LingHu, R., Feng, F., et al. (2015). Combating HER2-overexpressing breast cancer through induction of calreticulin exposure by Tras-Permut CrossMab. Oncoimmunology 4, e994391. doi:10.4161/2162402x.2014.994391
Zhu, Z., Zapata, G., Shalaby, R., Snedecor, B., Chert, M., and Carter, P. (1996). High level secretion of a humanized bispecific diabody from Escherichia coli. Bio/technology 14, 192–196. doi:10.1038/nbt0296-192
Keywords: bispecific, antibody, immunoglobulin, IgG fusion, sdAb, scFv, HC heterodimerization
Citation: Madsen AV, Pedersen LE, Kristensen P and Goletz S (2024) Design and engineering of bispecific antibodies: insights and practical considerations. Front. Bioeng. Biotechnol. 12:1352014. doi: 10.3389/fbioe.2024.1352014
Received: 07 December 2023; Accepted: 15 January 2024;
Published: 25 January 2024.
Edited by:
Michel Eppink, Wageningen University and Research, NetherlandsReviewed by:
Chang-Han Lee, Seoul National University, Republic of KoreaCopyright © 2024 Madsen, Pedersen, Kristensen and Goletz. This is an open-access article distributed under the terms of the Creative Commons Attribution License (CC BY). The use, distribution or reproduction in other forums is permitted, provided the original author(s) and the copyright owner(s) are credited and that the original publication in this journal is cited, in accordance with accepted academic practice. No use, distribution or reproduction is permitted which does not comply with these terms.
*Correspondence: Steffen Goletz, c2dvbGV0ekBkdHUuZGs=
Disclaimer: All claims expressed in this article are solely those of the authors and do not necessarily represent those of their affiliated organizations, or those of the publisher, the editors and the reviewers. Any product that may be evaluated in this article or claim that may be made by its manufacturer is not guaranteed or endorsed by the publisher.
Research integrity at Frontiers
Learn more about the work of our research integrity team to safeguard the quality of each article we publish.