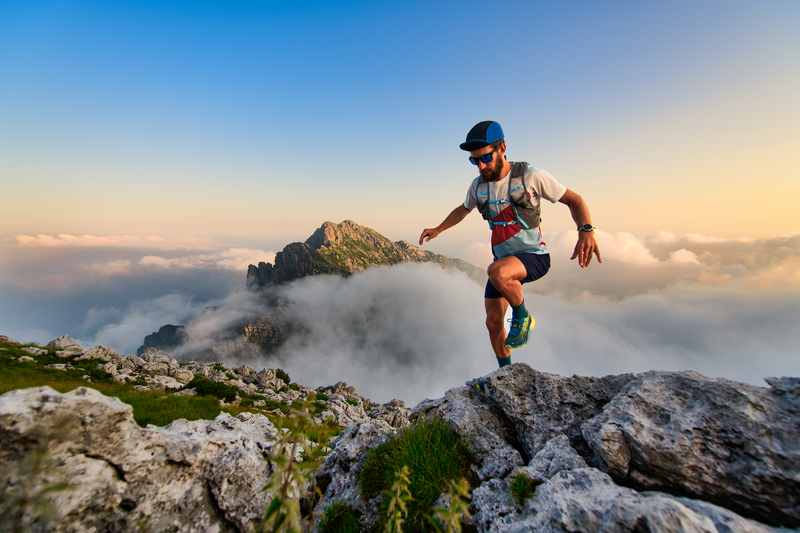
95% of researchers rate our articles as excellent or good
Learn more about the work of our research integrity team to safeguard the quality of each article we publish.
Find out more
REVIEW article
Front. Bioeng. Biotechnol. , 18 January 2024
Sec. Biomaterials
Volume 12 - 2024 | https://doi.org/10.3389/fbioe.2024.1349077
This article is part of the Research Topic Functional Biomaterials for Drug Delivery View all 8 articles
Gene therapy is a technique that rectifies defective or abnormal genes by introducing exogenous genes into target cells to cure the disease. Although gene therapy has gained some accomplishment for the diagnosis and therapy of inherited or acquired cardiovascular diseases, how to efficiently and specifically deliver targeted genes to the lesion sites without being cleared by the blood system remains challenging. Based on nanotechnology development, the non-viral vectors provide a promising strategy for overcoming the difficulties in gene therapy. At present, according to the physicochemical properties, nanotechnology-based non-viral vectors include polymers, liposomes, lipid nanoparticles, and inorganic nanoparticles. Non-viral vectors have an advantage in safety, efficiency, and easy production, possessing potential clinical application value when compared with viral vectors. Therefore, we summarized recent research progress of gene therapy for cardiovascular diseases based on commonly used non-viral vectors, hopefully providing guidance and orientation for future relevant research.
Cardiovascular disease (CVD) leads to almost a third of all deaths worldwide, resulting from atherosclerotic plaque leading to hemadostenosis and blood flow restriction (Park et al., 2020; Tsao et al., 2022). Despite progress in medical technology, CVD is still a major cause of death (Yang et al., 2023). Conventional treatment strategies for CVD include anticoagulation, antiplatelet, thrombolytics, hypolipidemic drugs, and invasive therapies like vascular bypass grafting and stent transplantation (Zhu et al., 2021). However, small molecule drug therapy in conventional treatment strategies is characterized by short half-life and low bioavailability, and long-term use of certain drugs may also lead to side effects such as drug resistance and potential hematological toxicity (Missri, 1979; Fu et al., 2014). Surgical treatment, on the other hand, is more pro-traumatic, requires a longer recovery time, and has a high risk of postoperative complications. These problems have led to the fact that conventional treatment options cannot fully meet clinical needs (Harafuji et al., 2005; Pala et al., 2020). In addition, conventional therapy focuses on palliatives to manage symptoms and slow down the disease progression, rather than disease eradication (Markina et al., 2023).
Gene therapy is a technique that rectifies defective or abnormal genes by introducing exogenous genes into target cells to cure the disease (Korpela et al., 2021). Delivering genes into targeted cells and tissues through vectors is crucial in gene therapy (Jiao et al., 2020). On the premise of the inactivation of the host immune response, transferring therapeutic genes into host cells with efficiency and sustainability is key to the success of gene therapy (Yetisgin et al., 2020). Virus and plasmid DNA are the most ordinary vectors in gene therapy agents (Hou et al., 2022). In practice, however, only a little bare plasmid DNA enters the cells, resulting in low transfection efficiency of therapeutic genes in target tissues (Ylä-Herttuala and Martin, 2000). Only adenovirus (Ad) vectors and adenovirus associated (AAV) vectors have been applied in cardiac clinical trials (Yetisgin et al., 2020), but potential risks like integration with the host cell genome and nonnegligible immunogenicity exist (Kaski and Consuegra-Sanchez, 2013; Yin et al., 2014; Lin and Qi, 2023). Non-viral vectors can effectively compensate for the shortcomings of viral vectors and safely achieve effective gene therapy (Expósito et al., 2023). As nanotechnology advances continue, gene therapy based on nanocarriers has entered clinical trials due to its unique properties and advantages.
Nanotechnology-mediated non-viral vector is a gene delivery system utilizing non-viral nanomaterials, as a safer alternative to viral vectors due to high safety and low cost (Sainz-Ramos et al., 2021). Non-viral nanocarriers generally include polymers, liposomes, lipid carriers and inorganic materials (Gu et al., 2020). Non-viral nanocarriers take advantage of physicochemical properties to modify vector structure (Yagublu et al., 2022), transferring exogenous nucleic acids to the targeted cells and assisting them to escape from the endosomes to ensure efficient expression (Yang et al., 2022). In comparison with viral vectors, non-viral nanocarriers, with higher biosafety, lower immunogenicity, and mutagenicity, own a wider application owing to convenient process, low cost, and no restriction on the target gene size (Yin et al., 2014; Ren et al., 2021). Recently, much progress has been achieved in nanotechnology-mediated gene therapy in the treatment of CVD as an intensive pathogenesis study and the development of personalized precision medicine. Based on this, this review summarized the latest researches on non-viral nanocarriers of gene therapy for CVD (Figure 1).
Polymer-based nanocarriers are fabricated from natural or synthetic polymers in terms of component variety and structure diversity (Mendes et al., 2022). As one of the most promising materials for nucleic acid nanodelivery, polymer nanocarriers have significant advantages in synthesis and functional modification, transfection efficiency, biocompatibility, etc. (Piotrowski-Daspit and Alexandra, 2020). For gene delivery, polymer nanocarriers mainly condense and encase nucleic acid through the electrostatic interaction between the cationic primary amine (cationic polymer) and the anionic phosphate group (nucleotide) without being degraded or cleared by enzymes in the reticuloendothelial system (Rupei et al., 2011; Ho et al., 2021). Furthermore, polymer nanocarriers notably enhance gene transfection efficiency by interrupting and escaping from endosomes through the “proton sponge” effect and high buffering ability within the physiological pH (Yan et al., 2022). Although they have excellent transfection efficiency, conventional cationic polymers are limited in clinical application because of non-degradability, cytotoxicity, aggregation, and lack of cell specificity (Askarian Saeedeh et al., 2015; Hao et al., 2019). Experiments aimed at polymer modification, transformation, or innovation have been conducted to overcome these deficiencies. For instance, by introducing hydrophilic polymers (typical PEG chains), serum stability and biocompatibility have been considerably improved (Mendes et al., 2017; Mendes et al., 2022). In this way, targeting moieties (antibodies, transferrin, folic acid, and glycosyl components) were attached to the surface of the polymer and then the cell uptake and target specificity were facilitated via targeted gene-molecule mutual interaction (Mitchell et al., 2021).
Boussif et al. synthesized a linear and branched polycationic polymer -polyethyleneimine (PEI) (Boussif, 1995), which has been used as a “gold standard” for gene delivery of non-viral vectors (Mitchell et al., 2021; Tong et al., 2023). Modification and functionalization of PEI have optimized efficiency and safety in gene delivery, making it widely used in CVD gene therapy. For example, bile acid-modified PEI (BA-PEI) with an amphiphilic surface was designed by (Moon et al., 2014) (Figure 2). BA-PEI successfully delivered hypoxia-induced VEGF (pHI-VEGF) into MSCs via increasing plasma membrane permeability, which enabled VEGF overexpression under ischemia and thus alleviated left ventricular remodeling after acute myocardial infarction (AMI). Wang et al. found that the positively charged hyperbranched PEI could be transformed into a non-toxic polymer material with hydrazide by neutralizing the primary amine group of dendritic macromolecules with the peripheral hydrazide group. With further modification of polypeptide ligands, a new PEI-based carrier (PEI-HYD-RGD) was created, which showed good biocompatibility and cell internalization efficiency when equipped with siRNA (PEI-HYd-RGD-SIRNA) in vitro (Wang et al., 2016). In the zebrafish cardiac injury model, PEI-HYd-RGD-SIRNA could be significantly absorbed by cardiomyocytes and endothelial cells in the injured ventricular apex region. This innovative PEI-based vector is expected to be further developed into a siRNA therapy agent for cardiovascular disease. Compared to PEI alone, Yu et al. (Yu et al., 2021) constructed a miRNA delivery system based on silica and PEI (F-silica Mir-24), which could release the loaded miRNAs (Mir-24) into the cytoplasm to inhibit apoptosis. Overexpression of miR-24 inhibited cardiomyocyte apoptosis and fibrosis by directly targeting and suppressing the expression of the pro-apoptotic protein Bim. This strategy enhanced new function and long-term prognosis 7–10 days after acute myocardial infarction, providing a valuable approach to the treatment of acute myocardial infarction.
FIGURE 2. Schematic representation of the synthesis of BA-PEI conjugates and VEGF-MSC transplantation in infarcted myocardium. BA-PEI/pHI-VEGF was used to transfect VEGF into rat MSCs to overexpress VEGF protein and play a therapeutic role in the rat myocardial ischemia-reperfusion model (Moon et al., 2014).
Polyamidoamine (PAMAM) is one of the earliest carriers used for gene delivery and one of the most commonly used dendrimeric cationic polymer carriers in biological applications (Zu and Gao, 2021). By modifying its structure and introducing antibodies on its surface, its delivery efficiency in CVD can be improved. For example, K. Zhu et al. (Zhu et al., 2013) synthesized hyperbranched PAMAM (h-PAMAM) as a novel gene delivery vector through polycondensation. The pHRE-Hvegf165 carried by h-PAMAM can resist nuclease digestion and efficiently transfect primary skeletal myoblasts (SKMs) with low cytotoxicity. In the myocardial infarction model, the transfected SkMs can reduce infarct area and interstitial fibrosis, thus inhibiting left ventricular remodeling in the post-infarction period and enhancing cardiac function. Furthermore, another study cross-linked the PAMAM-DNA complex with anti-e-selectin mab (1.2B6), delivering the PAMAM-DNA complex to the inflammatory vascular endothelium by binding the 1.2B6 antibody to E-selectin and P-selectin. As a result, the delivered anti-inflammatory genes reversed or inhibited atherosclerosis progression (Theoharis et al., 2009).
Poly (lactic-co-glycolic acid) (PLGA), one of the most successful synthetic biodegradable polymers, is hydrolyzed intracellularly to lactic acid and glycolic acid that can be metabolized by the body through the Krebs cycle, and therefore has the best biocompatibility (Kim et al., 2019). PLGAs are popular as carriers for proteins and small molecules and are now being used to deliver genetic information to cells for gene therapy. However, PLGA polymer nanoparticles, upon entering the organism, need to cross a variety of biological barriers, such as reticuloendothelial system (RES)-mediated clearance and binding of regulatory proteins in the bloodstream to PLGA that may lead to phagocytosis and clearance of nanoparticles by macrophages (Zou et al., 2009). The presence of these barriers may limit drug delivery and absorption, thus affecting drug efficacy. To overcome these barriers, several strategies have been employed to improve the effectiveness of PLGA delivery in vivo. One common strategy is to provide a hydrophilic layer on the PLGA surface to encapsulate molecules that hide the hydrophobicity, and the most commonly employed hydrophilic polymer is PEG (Danhier et al., 2012). In addition, cellular uptake can be facilitated by the addition of cationic polymers (Kim et al., 2005). X. Zhang et al. (Zhang, 2022a) prepared PP/PEI nano complexes by coupling poly (ethylene glycol) methyl ether block-PLGA (PEG-b-PLGA; PP) nanoparticle surfaces with PEI, and PP/PEI would carry the CRISPR/Cas9 gene editing plasmid induced efficient genome editing in endothelial cells of the vascular system (including lungs, heart, aorta, and peripheral vasculature) of adult mice after intravenous injection. In addition, the gene editing plasmid can induce genome editing of at least two genes at the same time or introduce both genome editing and transgene expression in vascular endothelial cells, advancing the development of cardiovascular research and potential gene therapy. Another strategy is to attach targeting motifs to increase selective cell binding and internalization through receptor-mediated endocytosis. Messerschmidt et al. (Messerschmidt et al., 2022) Coupled anti-Tie2+Tie1 antibody to the surface of PLGA and successfully delivered the plasmid encoding Notch intracellular domain (NICD) to the zebrafish endocardial layer via antibody binding to the endocardium, which resulted in the overexpression of Notch-related genes and significant improvement in cardiac function; T. Wang et al. (T. Wang et al., 2022) used platelet vesicle (PMV) camouflaged PLGA nanoparticles (PMVs@PLGA) as a carrier for miRNA inhibitors, and miRNA-targeted transport into cardiomyocytes indirectly increased the expression of Nuclear factor (erythroid-derived 2)-related factor 2 (Nrf2) by competitively binding to miR-155-5p, which protectsed myocardium in the occurrence of myocardial ischemia-reperfusion injury (MIRI), and provided a new potential pathway for the targeted treatment of MIRI.
Chitosan (CS) as a common natural carbohydrate polymers (Zu and Gao, 2021), is an alkaline polysaccharide with high biodegradability, biocompatibility, and nontoxicity and a 6.5 apparent pKa. Most of the amino groups are protonated under acidic conditions (Chronopoulou et al., 2022). Therefore, chitosan is an ideal carrier for delivering nucleic acids due to its ability of nucleic acids absorbance to form stable complexes (Ashrafizadeh, 2023). Besides, CS surface modification by PEG with different molecular weights improved aqueous solubility and prolonged half-lives (Zhang et al., 2018). Nguyen et al. used sodium tripolyphosphate (TPP) (a crosslinking agent) to synthesize polyethylene glycol chitosan polymer (chNPs) through an ester coupling reaction to enclose miR-33, and then successfully formulated an intracellular gene delivery vector targeting mouse macrophages (Nguyen et al., 2019). The results showed that chNPs successfully inhibited ABCA1 expression thereby reducing the outflow of liposterols in cholesterol metabolism, which could be used to treat atherosclerosis. Zhou et al. (Zhou et al., 2016) modified trimethyl chitosan (TMC) with the short peptide REDV (Arg-Glu-Asp-Val) and PEG to deliver miR 126 into vascular endothelial cells (VECs), which has achieved remarkable progress in promoting VECs proliferation and improving ischemic myocardial necrosis due to the high transfection efficiency.
Liposomes are closed, spherical vesicles that consist of a phospholipid bilayer with polar head groups and non-polar tail groups, along with a stabilizer like cholesterol. These liposomes are capable of delivering drugs such as nucleic acids into the cells (Mukalel et al., 2019; Li et al., 2019). Cationic liposomes are a general term for a class of positively charged liposomes, which are typically composed of diverse cationic lipid molecules alone or with neutral auxiliary lipids, such as 1,2-dioleoyl-SN-propyltriphenyl (3) phosphatidyl ethanolamine (DOPE), phosphatidylcholine (PC), cholesterol (Chol/CHO), and other common composition (Ma et al., 2021). Cationic liposomes play a crucial role in cationic liposomes by providing a positive charge, which enables them to attract, encapsulate, and compress nucleic acids (Montoto et al., 2020), while the helper lipids are in charge of improving bilayer membrane stability, reducing cationic liposome toxicity, and promoting their ability to escape from endosomes (Imani et al., 2018; Buck et al., 2019). The positively charged carrier-nucleic acid complex, through the action of electrostatic interaction, is adsorbed to the cell surface and enters the cell via endocytosis and membrane fusion, ultimately achieving transgenic expression (Shtykalova et al., 2023).
Liposomes have many merits as gene delivery vectors. Firstly, liposomes are spherical vesicles that encapsulate nucleic acids and resist nucleases (Hussen and Mahmud, 2023). Secondly, similar to cell membranes, liposomes are easy to fuse with recipient cells and have high transfection efficiency. Thirdly, as delivery systems, liposomes have no limits in the host (Lim et al., 2020). Finally, the phospholipid bilayer structure highly mimics cell membranes and exhibits excellent storage stability (Li, 2022a). Although liposomes have many advantages compared with other carriers and are widely used in in vitro cell experiments and basic experimental studies, the transfection efficiency of liposomes is low in tissues or organs with special structures and arrangements such as the heart (Wang et al., 2011). In recent years, scientists have worked to solve these difficult problems by exploring and researching new methods and techniques. Among them, the targeted delivery of drugs can be improved by using nanotechnology, and the absorption rate of drugs in heart cells can be increased by rationally designed carriers (Zhao et al., 2023). Hein et al. (Hein et al., 1998) used transferrin-modified cationic liposomes to deliver plasmids expressing vascular endothelial growth factor (pCMVbeta) into vascular endothelial cells. This approach significantly improved the delivery efficiency of the plasmid compared to unmodified vectors.
Cardiovascular diseases, such as atherosclerosis, restenosis, and inflammation, often occur in specific areas of the vasculature, offering the potential for targeted drug therapy applications. Liposomes are promising targeted drug carriers for intravascular applications that could beneficially impact the treatment of these conditions (Tang et al., 2021; Lestini et al., 2002). Liposomes utilize both passive and active targeting approaches to enhance the delivery efficiency and residence time of payload genes in the body (Li Wenpan et al., 2022). Liposome size, charge, and surface modification can significantly impact their blood clearance, cellular uptake, and distribution throughout the cardiovascular system (Khadka et al., 2020; Manners et al., 2022). Larger liposomes are prone to be engulfed by macrophages, whereas smaller ones tend to be ingested by fibroblasts (Bozzer, 2022). By modifying polyethylene glycol (PEG) on the surface of the liposome, it is disguised as an “invisible liposome” to reduce complement activation, enhance stability, and extend the cycle time of the liposome (Li et al., 2021; Zhu et al., 2017; Haghiralsadat et al., 2018). Researchers realized active targeting by adding targeting groups that improve the retention of liposomes at specific sites, increasing local concentrations and cell internalization (Bowey et al., 2012; Zhai et al., 2022). During the development of atherosclerotic lesions and injuries, the endothelium is the main location of cell extravasation and inflammation (Song et al., 2022). Leukocyte adhesion molecules (VCAM-1), ELAM-1 or E-selectin, and intercellular adhesion molecule-1 (ICAM-1) are highly expressed on the surface of vascular endothelial cells, which serve as prime targets for therapeutic delivery of CVD (Cao et al., 2021) (Figure 3). Jia et al. (X. Jia et al., 2022) designed VCAM-1 binding peptide targeting cationic liposomes (PCLs) as a siRNA delivery vector to wrap methylated NLRP3 siRNA into PCLs (NLRP3 SIRNA-PCLS). NLRP3 siRNA-PCLs can target VCAM-1 expressing endothelial cells, knockdown NLRP3, prevent TNF-α-induced NLRP3 inflammasome activation and intracellular flow of LDL, and significantly reduce the accumulation of atherosclerotic LDL in TNF-α-stimulated rat carotid endothelial cells. Another common cardiac target is myosin, which is exposed when the endothelial cell membrane is damaged. The highly specific anti-myosin monoclonal antibody 2G4 (mAb 2G4) has displayed remarkable ability in recognition and binding to ischemic cells and damaged plasma membranes, allowing intracellular myosin to be exposed to the extracellular space (Skourtis et al., 2020). Activated transcriptional activator (TAT) peptide and anti-myosin monoclonal antibody 2G4 (mAb 2G4). This construct was designed to specifically target myocardial myosin, with the aim of delivering target gene therapy to ischemic myocardium. A dual-targeted delivery system is capable of accumulating outside the cell and penetrating inside the cell to enhance the delivery of genes to target cells. Despite the many advantages of liposomes as nucleic acid carriers, the production process of preparation and nucleic acid encapsulation requires the use of organic solvent injection, which makes the production process complicated and limits the large-scale production of liposomes by their limited physical stability, low drug loading capacity, and the possibility of drug leakage (Mendes et al., 2022; Seo et al., 2023).
FIGURE 3. (A) Schematic diagram of liposomes (B) Selection of targets when endothelial damage occurs (C) Schematic diagram of the mechanism of liposomes targeting ischemic cardiomyocytes (Bowey et al., 2012).
Lipids-based nanoparticles (LNPs) are unique nanostructures, composed of various types of lipids, including cationic or ionizable lipids (CIL), structural lipids (phospholipids and cholesterol), and PEG-binding lipids (PEG-Derived phospholipids) (Patel et al., 2019). Among them, ionizable lipids are the key components that determine the titer, mRNA delivery efficiency, degradability, and reactivity (Drescher and van Hoogevest, 2020). At low pH, ionizable lipids are protonated in acidic buffers, producing a positive charge and binding to negatively charged mRNA, whereas they remain neutral at physiological pH (Chan et al., 2021; Scalzo et al., 2022). The delivery mechanism of LNPs is shown in Figure 4. Ionizable lipids are less cytotoxic than cationic lipids. This is because the positive charge of cationic lipids typically interacts with negatively charged molecules on the cell surface, leading to cell membrane damage and cell death. In contrast, ionizable lipids can dynamically regulate their charge state in the cellular environment, avoiding the continuous release of positive charges to the cell surface and reducing cytotoxicity (Sharma et al., 2014). In general, PEG-bound lipids in LNPs occupy the smallest molar percentage, which can enhance particle stability by affecting charge distribution, size, and dispersion, and hinder LNPs from aggregating, playing a crucial role in blood circulation and biological distribution (Albertsen and Camilla et al., 2022; Kon et al., 2022; Guéguen et al., 2023). Structural lipids are chiefly used to support the structure of particles and enhance stability during storage and circulation (Kulkarni et al., 2019; Witzigmann et al., 2020). According to diverse target tissues, the proportions of these four lipid components can be adjusted to change the LNP constitution (Yihunie et al., 2023). At the same time, the physical characteristics of LNPs, like particle size, morphology, encapsulation rate, and surface charge are prone to be regulated, thus producing a variety of different LNP formulations (Yang et al., 2022; Suzuki and Ishihara, 2021). Ionizable lipid nanoparticles display excellent biocompatibility, high nucleic acid encapsulation efficiency, and efficient transfection performance, releasing only under specific circumstances with low off-target effects (Yang et al., 2022). Nowadays, LNPs have become the most widely explored and applied non-viral nucleic acid delivery vector (Sun and Lu, 2023).
FIGURE 4. The Delivery mechanism of lipid nanoparticles (Suzuki and Ishihara, 2021). First, the lipid nanoparticles (LNP) completely encapsulated the RNA and prevented it from nuclease digestion. LNPs are neutral in physiological pH due to ionizable lipids and pegylated phospholipids, thus reducing non-specific interactions with serum proteins. Second, LNPs are taken up by cells via apolipoprotein E (ApoE) dependent and/or ApoE independent pathways when the pegylated phospholipids are dissociated. Finally, the protonated LNPs, after acidification in the endosomes, induce the hexagonal phase structure, destroy the cell membrane, and release RNA molecules into the cytoplasm.
Now the focus is on how to improve the delivery effect of LNPs. Modifying the surface of LNPs with targeted molecular entities becomes the most popular way (Subhan et al., 2023). In principle, peptides, antibodies, or proteins that target specific cell surface molecules are added to nanoparticles carrying mRNA to block liver accumulation and enable particular mRNA transport to target cells through high-affinity binding (Kedmi et al., 2018; Yang et al., 2022). Joel G. Rurik et al. (Rurik et al., 2022) conjured CD5 antibodies to LNP (CD5/LNP) to deliver CAR mRNA encoding fibroblast activation protein (FAP). LNP vectors specifically target T cells to generate FAP- (CAR) -T cells that recognize and attack activated cardiac fibroblasts, consequently alleviating fibrosis and regaining normal heart function in a mouse model of heart failure. Studies have shown that mannose-modified LNPs (LNP-MAN) can possibly promote uptake by APCs (Zhuang et al., 2020). Gao et al. (Gao et al., 2023) self-assembled cationic lipids (G0-C14) and poly (lactide-co-ethylacetide) -B-polyethylene glycol (PLGA-PEG) that are biodegradable to form nanoparticles (HNPs), and modified mannose on HNPs by covalent bonding, formulating macrophage-targeting nanoparticles (M-HNPs) in atherosclerotic lesions. M-HNPs coated interleukin-10 (IL-10) mRNA, mediated by mannose receptor (CD206), targeted IL-10 mRNA delivery to the site of atherosclerotic lesions and translated into anti-inflammatory factor IL-10, which increased the thickness of fiber cap by decreasing the accumulation of lipids and the size of necrotic areas, thus promoting inflammation regression, inhibiting oxidative stress and apoptosis and played an anti-atherosclerosis role. Although LNP is one of the most efficient carriers for mRNA delivery, the structural differences between DNA and mRNA result in significant differences in their delivery requirements. Further optimization of LNP formulations is required to improve DNA delivery (Algarni, 2022; Li, 2022a). Scalzo et al.‘s (Scalzo et al., 2022) research team successfully induced up to 80% transfection efficiency in cardiomyocytes in vitro by adjusting the molar ratio of ionizable lipid C12-100 to lipid combinations and the ratio of C12-100 to plasmid DNA. This study provided a new delivery vehicle for DNA delivery in cardiomyocytes. In general, the basic structure of both LNPs and liposomes are lipid molecules with similarities, yet there are significant differences (Table 1).
Gold nanoparticles, magnetic nanoparticles, and porous silicon nanoparticles are the most widely utilized inorganic nanoparticles (Sharma et al., 2021), with precise control over shape and size, non-immunogenicity, favorable biosafety, and targeting, and are apt to large-scale production, and are extensively applied in the delivery and imaging of various drugs (Wang et al., 2023; Hu et al., 2022).
Gold nanoparticles (AuNPs) are the most widely studied metal nanoparticles with excellent physical and chemical properties (Zhang, 2022b), such as bioinert, low cytotoxicity, and high stability, and AuNPs are easy to prepare and modify, which is suitable for the delivery of nucleic acid in gene therapy (Tang et al., 2022; Lee et al., 2023). AuNPs-based application in combination with existing gene therapy has been recognized as an innovative tactic with potent possibility in treating heart disease (Pala et al., 2020). Jia et al. (Jia et al., 2017) successfully constructed Antago-Mir-155-AUNps nanoparticles by covalently binding the sulfcap-modified antago-miR-155 with AuNPs. Mice received an injection of antagomir-155-aunps via the tail vein with estrogen-deficiency induced diabetic cardiomyopathy, and antago-miR-155 was steadily released in vivo and predominantly sent to macrophages through phagocytosis. Antago-miR-155 mediated an increase in M2-type macrophages, reduced inflammation and apoptosis, and restored cardiac function. Therapeutic neovascularization can be completed by delivering numerous growth factors (such as VEGF) (Kim et al., 2011; Shen et al., 2021). In clinical trials, due to inferior targeting the short circulating half-life of VEGF, and the relatively long time VEGF needs to exist to prevent the degeneration of newly formed blood vessels, it is difficult for traditional intravenous VEGF administration methods to target VEGF to damaged tissues (Kim et al., 2011; Henry et al., 2003). In the mouse model of hindlimb ischemia, AuNPs, as an excellent payload, targeted exogenous VEGF to ischemic muscle tissue via its augmented permeability and retention effect, accelerated the recovery of abundant ischemic tissue and facilitated angiogenesis (Pala et al., 2020; Kim et al., 2011).
Magnetic iron oxide nanoparticles (MNP) consisting of magnetite (Fe3O4) or magnehematite (Fe2O3) have certain superparamagnetism, and magnetic liposomes (MLs) are synthesized using magnetic nanoparticles and liposomes (Arias, 2018), which can be applied in magnetic resonance imaging and magnetism-based targeted drug delivery in CVDs (Younis et al., 2021). Molavi et al. (Marcos-Campos et al., 2011) found that angiotensin receptor 1 is overexpressed in infarcted hearts and can be used as a target for MLs. Which can effectively target growth factors, biomolecules, and cytokines in the infarcted heart muscle, minimizing the affected area of cardiac fibrosis (Namdari et al., 2017).
Porous silicon nanoparticles (MSV) have good biocompatibility and degradability and also possess unique functions, including high porosity (up to 80%) and a unique chemical surface that can enhance the solubility of hydrophobic drugs and control drug release. These properties make MSVs highly suitable for the delivery of therapeutic agents, potentially improving treatment outcomes and reducing side effects (Wang et al., 2023; Petrisor et al., 2022). Ma et al. (Ma et al., 2016) specifically coupled e-selectin with thioaptamer (ESTA) and covalently connected it to the surface of MSV, successfully designing a nanoparticle that can target atherosclerotic inflammatory endothelial cells (ESTA-MSV). MiR-146a and miR-181b were compressed in ESTA-MSV nanoparticles and injected into ApoE gene-deficient mice through the tail vein. The findings indicated that ESTA-MSV-encapsulated mir-1461/181b could effectively suppress the expression of chemokines in aortic tissue, improve endothelial cell inflammation and significantly shorten the thickness of atherosclerotic plaques. Mesoporous silica (MSN) possesses the advantages of a large and uniform pore structure, high specific surface area, an adjustable pore structure, excellent chemical stability, and versatility, which makes it a crucial material in adsorbents, catalysts, separation materials, and drug-controlled release systems. Wang et al. (Wang et al., 2021) developed a novel biomimetic non-viral vector by wrapping the FH peptide-modified neutrophil membrane around MSNs loaded with miR-1, 133, 208, and 499 (miRCombo) (Figure 5). They injected MSNs-miR into the tail vein of a mouse model of myocardial ischemia/reperfusion injury, delivering miRCombo specifically to damaged cardiac fibroblasts (CFs) through the natural homing ability of neutrophil membrane protein and the high affinity of FH peptide to CFs. By mediating microRNA regulation, they transformed CFs into induced cardiomyocyte-like cells (iCMs), achieving in vivo cardiac reprogramming and improving heart function while reducing fibrosis. Cheang et al.’s (Cheang et al., 2012) research team used aminopropyltriethoxysilane (APTES), a common chemical, to covalently bind to the silicon atoms on the surface. By modifying the surface, APTES enhanced the binding ability to plasmid DNA. This novel gene carrier preparation improved the delivery efficiency of plasmid DNA in human vascular smooth muscle cells.
FIGURE 5. Schematic diagram of the preparation process of FNLM miR and application in myocardial ischemia/reperfusion injury model (Wang et al., 2021).
Despite the promise of inorganic nanoparticles for delivery, diagnostic, and therapeutic applications, the in vivo degradability and toxicity of inorganic nanoparticles compared to organic nanoparticles have long been an issue of concern. After intravenous injection, inorganic nanoparticles may selectively aggregate and accumulate in specific tissues or organs, and long-term deposition is often toxic, thus limiting their clinical applications (Yang et al., 2020). Accurately comparing the toxicity of inorganic nanoparticles is often challenging due to the variation in dose levels, routes, purity, and frequency of administration reported in various studies (Mohammadpour et al., 2019). Typically, inflammation and oxidative stress are common mechanisms for inducing toxicity in inorganic nanomaterials (Cheng et al., 2022). For example, iron oxide nanoparticles increase endothelial barrier permeability by inducing oxidative stress, which can lead to local inflammation (Apopa, 2009); Hsu et al. (Hsu et al., 2021) reported that exposure to silicon oxide nanoparticles lead to a significant upregulation of TNF and MAPK signaling pathways. This upregulation triggered the secretion of various pro-inflammatory cytokines by modulating the p38 and JNK signaling pathways, ultimately activating the transcription factor AP-1. In turn, AP-1 promotes apoptosis and inflammatory responses. The main molecular mechanism underlying the toxicity of gold nanoparticles is the increase in oxidative stress caused by the formation of free radicals. This leads to oxidation and damage to intracellular components, lipids, proteins, and DNA. Additionally, the aggregation of nanoparticles following systemic administration not only results in a loss of function but may also cause capillary occlusion, resulting in end-organ damage (Lanone and Boczkowski, 2006; Aillon, 2009; Thakor et al., 2011). Therefore, in the future use of inorganic nanoparticles for drug delivery and therapeutic applications, it is not only necessary to emphasize their success in applications, but also to pay attention to the toxicity of inorganic nanoparticles due to their non-degradability, and to conduct a comprehensive assessment and research to minimize the potential toxicity and side effects.
In summary, this is a review of several commonly used non-viral vectors in gene therapy of cardiovascular diseases in recent years, and the specific summary can be found in Tables 2, 3.
TABLE 3. To summarize the application strategies of non-viral vectors in the delivery of cardiovascular diseases.
Over the past decade, nanotechnology has advanced rapidly. Nanotechnology-mediated gene delivery provides a broader view of CVD gene therapy. In this paper, we reviewed the application of common non-viral nanocarries (polymers, liposomes, and inorganic nanoparticles) in CVD gene therapy. With superiority in half-life extension and biocompatibility, non-viral nanocarriers actively deliver exogenous nucleic acids to target cells or organs, marking the dawn of a new era for treating CVD. Although non-viral nanocarries have great application prospects and satisfactory preclinical results, nanotechnology-mediated non-viral CVD gene therapy has not been successfully applied clinically. Clinical translation of nanomedicine still has a long way to go. Multiple reasons account for this defeat: 1) inadequate gene delivery to target sites thus destroying the potential of transgenes. 2) insufficiency of transgenes expression time. 3) lack the recognition of underlying pathophysiological mechanisms. In addition, the scale-up of non-viral vectors is a challenge, and there is a need to develop scale-up methods that ensure consistency, reproducibility, and product quality and safety of the final product. In addition, non-viral vectors are usually made of synthetic polymers or lipids, which may not be readily degradable, leading to toxicity, an immune response, and long-term accumulation of the vector in the body. Therefore, there is a need to develop non-viral vectors that are biodegradable and have minimal toxic effects for clinical applications. Clinical regulation also plays an important role in the development of non-viral vectors for use in the field of CVD gene delivery. Regulatory agencies such as the U.S. Food and Drug Administration (FDA) require rigorous safety and efficacy evaluations of gene therapy products before they can be approved. This includes preclinical testing in animal models to assess the safety and efficacy of the product and clinical trials in humans to assess its safety and efficacy. Regulatory requirements for non-viral vectors may vary depending on the country or region in which they are used, and investigators need to ensure that local regulatory requirements are met. As nanomedicine advances, continuous improvement and optimization of nano-gene delivery vectors will help overcome these obstacles. In addition, combination therapy like gene-surgery therapy is promising to provide a comprehensive strategy for CVD treatment. More and more optimized and modified nanoscale gene delivery systems are believed to enter and successfully applied in clinics in the near future.
LJ: Conceptualization, Visualization, Writing–original draft. ZS: Visualization, Writing–original draft. ZS: Resources, Writing–review and editing, Funding acquisition. JL: Funding acquisition, Resources, Writing–review and editing. GD: Supervision, Writing–review and editing. XW: Conceptualization, Supervision, Writing–review and editing.
The author(s) declare financial support was received for the research, authorship, and/or publication of this article. This work was supported by the Yantai Science and Technology Plan Project (No: 2022MSGY074 for JL), Shandong Natural Science Foundation Youth Program (No: ZR2022QC133 for ZS).
The authors declare that the research was conducted in the absence of any commercial or financial relationships that could be construed as a potential conflict of interest.
All claims expressed in this article are solely those of the authors and do not necessarily represent those of their affiliated organizations, or those of the publisher, the editors and the reviewers. Any product that may be evaluated in this article, or claim that may be made by its manufacturer, is not guaranteed or endorsed by the publisher.
Aillon, K. L., Xie, Y., El-Gendy, N., Berkland, C. J., and Forrest, M. L. (2009). Effects of nanomaterial physicochemical properties on in vivo toxicity. Adv. Drug Deliv. Rev. 61 (6), 457–466. doi:10.1016/j.addr.2009.03.010
Albertsen, H., Camilla, , Witzigmann, D., Lind, M., Petersson, K., and Simonsen, J. B. (2022). The role of lipid components in lipid nanoparticles for vaccines and gene therapy. Adv. Drug Deliv. Rev. 188 (irailak), 114416. doi:10.1016/j.addr.2022.114416
Algarni, A., Pilkington, E. H., Suys, E. J. A., Al-Wassiti, H., Pouton, C. W., and Truong, N. P. (2022). In vivo delivery of plasmid DNA by lipid nanoparticles: the influence of ionizable cationic lipids on organ-selective gene expression. Biomaterials Sci. 10 (11), 2940–2952. doi:10.1039/d2bm00168c
Arias, L. S., Pessan, J., Vieira, A., Lima, T., Delbem, A., and Monteiro, D. (2018). Iron oxide nanoparticles for biomedical applications: a perspective on synthesis, drugs, antimicrobial activity, and toxicity. Antibiot. (Basel, Switz. 7 (2), 46. doi:10.3390/antibiotics7020046
Ashrafizadeh, M., Hushmandi, K., Mirzaei, S., Bokaie, S., Bigham, A., Makvandi, P., et al. (2023). Chitosan-based nanoscale systems for doxorubicin delivery: exploring biomedical application in cancer therapy. Bioeng. Transl. Med. 8 (1), e10325. doi:10.1002/btm2.10325
Askarian, S., Abnous, K., Taghavi, S., Oskuee, R. K., and Ramezani, M. (2015). Cellular delivery of shRNA using aptamer-conjugated PLL-alkyl-PEI nanoparticles. Colloids Surfaces B Biointerfaces 136, 355–364. doi:10.1016/j.colsurfb.2015.09.023
Boussif, O., Lezoualc'h, F., Zanta, M. A., Mergny, M. D., Scherman, D., Demeneix, B., et al. (1995). A versatile vector for gene and oligonucleotide transfer into cells in culture and in vivo: polyethylenimine. Proc. Natl. Acad. Sci. 92 (16), 7297–7301. doi:10.1073/pnas.92.16.7297
Bowey, K., Tanguay, J.-F., and Tabrizian, M. (2012). Liposome technology for cardiovascular disease treatment and diagnosis. Expert Opin. Drug Deliv. 9 (2), 249–265. doi:10.1517/17425247.2012.647908
Bozzer, S., Dal Bo, M., Grimaldi, M. C., Toffoli, G., and Macor, P. (2022). Nanocarriers as a delivery platform for anticancer treatment: biological limits and perspectives in B-cell malignancies. Pharmaceutics 14 (9), 1965. doi:10.3390/pharmaceutics14091965
Buck, J., Grossen, P., Cullis, P. R., Huwyler, J., and Witzigmann, D. (2019). Lipid-based DNA therapeutics: hallmarks of non-viral gene delivery. ACS Nano 13 (4), 3754–3782. doi:10.1021/acsnano.8b07858
Cao, G., Xuan, X., Zhang, R., Hu, J., and Dong, H. (2021). Gene therapy for cardiovascular disease: basic research and clinical prospects. Front. Cardiovasc. Med. 8, 760140. doi:10.3389/fcvm.2021.760140
Chan, C., Du, S., Dong, Y., and Cheng, X. (2021). Computational and experimental approaches to investigate lipid nanoparticles as drug and gene delivery systems. Curr. Top. Med. Chem. 21 (2), 92–114. doi:10.2174/1568026620666201126162945
Cheang, T.-yun, He, W. l., Tang, B., Cheang, , Wang, S., Pro Xu, , et al. (2012). Promising plasmid DNA vector based on APTES-modified silica nanoparticles. Int. J. Nanomedicine 7, 1061–1067. doi:10.2147/IJN.S28267
Cheng, Z., Que, H., Chen, L., Sun, Q., and Wei, X. (2022). Nanomaterial-based drug delivery system targeting lymph nodes. Pharmaceutics 14 (7), 1372. doi:10.3390/pharmaceutics14071372
Chronopoulou, L., Falasca, F., Di Fonzo, F., Turriziani, O., and Palocci, C. (2022). siRNA transfection mediated by chitosan microparticles for the treatment of HIV-1 infection of human cell lines. Mater. (Basel, Switz. 15 (15), 5340. abuztuak 3. doi:10.3390/ma15155340
Danhier, F., Ansorena, E., Silva, J. M., Coco, R., Le Breton, A., and Préat, V. (2012). PLGA-based nanoparticles: an overview of biomedical applications. J. Control. Release 161 (2), 505–522. doi:10.1016/j.jconrel.2012.01.043
Danila, D., Partha, R., Elrod, D. B., Lackey, M., Casscells, S. W., and Conyers, J. L. (2009). Antibody-labeled liposomes for CT imaging of atherosclerotic plaques: in vitro investigation of an anti-ICAM antibody-labeled liposome containing iohexol for molecular imaging of atherosclerotic plaques via computed tomography. Tex. Heart Inst. J. 36 (5), 393–403.
Drescher, S., and van Hoogevest, P. (2020). The phospholipid research center: current research in phospholipids and their use in drug delivery. Pharmaceutics 12 (12), 1235. abenduak 18. doi:10.3390/pharmaceutics12121235
Expósito, J., Natera, D., Carrera, L., Armijo, J., Rios, A., Nascimento, A., et al. (2023). Gene therapy: where are we? Where are we going? Medicina 83 (Suppl. 4), 13–17. (irailak).
Fu, P., Yang, L., Sun, Y., Ye, L., Cao, Z., and Tang, K. (2014). Target network differences between western drugs and Chinese herbal ingredients in treating cardiovascular disease. BMC Bioinforma. 15 (Suppl. 4), S3. (martxoak 19. doi:10.1186/1471-2105-15-S4-S3
Gao, M., Tang, M., Ho, W., Teng, Y., Chen, Q., Bu, L., et al. (2023). Modulating plaque inflammation via targeted mRNA nanoparticles for the treatment of atherosclerosis. ACS Nano 17 (18), 17721–17739. doi:10.1021/acsnano.3c00958
Gu, Z., Da Silva, C., Van der Maaden, K., Ossendorp, F., and Cruz, L. (2020). Liposome-based drug delivery systems in cancer immunotherapy. Pharmaceutics 12 (11), 1054. azaroak 4. doi:10.3390/pharmaceutics12111054
Guéguen, C., Ben Chimol, T., Briand, M., Renaud, K., Seiler, M., Ziesel, M., et al. (2023). Evaluating how cationic lipid affects mRNA-LNP physical properties and biodistribution. Eur. J. Pharm. Biopharm., doi:10.1016/j.ejpb.2023.08.002
Haghiralsadat, F., Amoabediny, G., Naderinezhad, S., Forouzanfar, T., Helder, M. N., and Zandieh-Doulabi, B. (2018). Preparation of PEGylated cationic nanoliposome-siRNA complexes for cancer therapy. Artif. Cells, Nanomedicine, Biotechnol. 46 (Suppl. 1), 684–692. doi:10.1080/21691401.2018.1434533
Hao, F., Li, Y., Zhu, J., Sun, J., Marshall, B., Lee, R. J., et al. (2019). Polyethylenimine-based formulations for delivery of oligonucleotides. Curr. Med. Chem. 26 (13), 2264–2284. doi:10.2174/0929867325666181031094759
Harafuji, K., Chikamori, T., Kawaguchi, S., Obitsu, Y., Ito, S., Igarashi, Y., et al. (2005). Value of pharmacologic stress myocardial perfusion imaging for preoperative risk stratification for aortic surgery. Circulation J. Official J. Jpn. Circulation Soc. 69 (5), 558–563. doi:10.1253/circj.69.558
Hein, M., Ernst, M., Möller, F., and Regensburger, D. (1998). Gene transfer into rat heart-derived endothelial cells. Eur. J. Cardio-Thoracic Surg. Official J. Eur. Assoc. Cardio-Thoracic Surg. 13 (4), 460–466. doi:10.1016/s1010-7940(98)00029-3
Henry, T. D., Annex, B. H., McKendall, G. R., Azrin, M. A., Lopez, J. J., Giordano, F. J., et al. (2003). The VIVA trial: vascular endothelial growth factor in Ischemia for Vascular Angiogenesis. Circulation 107 (10), 1359–1365. doi:10.1161/01.cir.0000061911.47710.8a
Ho, W., Gao, M., Li, F., Li, Z., Zhang, X., and Xu, X. (2021). Next-Generation vaccines: nanoparticle-mediated DNA and mRNA delivery. Adv. Healthc. Mater. 10 (8), e2001812. doi:10.1002/adhm.202001812
Hou, S., Hasnat, M., Chen, Z., Liu, Y., Faran Ashraf Baig, M. M., Liu, F., et al. (2022). Application perspectives of nanomedicine in cancer treatment. Front. Pharmacol. 13, 909526. doi:10.3389/fphar.2022.909526
Hsu, S.-Yi, Morris, R., and Cheng, F. (2021). Signaling pathways regulated by silica nanoparticles. Molecules 26 (5), 1398. doi:10.3390/molecules26051398
Hu, T., Gong, H., Xu, J., Huang, Y., Wu, F., and He, Z. (2022). Nanomedicines for overcoming cancer drug resistance. Pharmaceutics 14 (8), 1606. doi:10.3390/pharmaceutics14081606
Huang, H.-C., Wang, T. Y., Rousseau, J., Mungaray, M., Michaud, C., Plaisier, C., et al. (2023). Lesion-specific suppression of YAP/TAZ by biomimetic nanodrug ameliorates atherosclerosis development. bioRxiv, 2023.04.24.537992. doi:10.1101/2023.04.24.537992
Hussen, , Mahmud, B., Abdullah, S. R., Hidayat, H. J., Faraj, G. S. H., Ali, F. A., et al. (2023). Targeting miRNA by CRISPR/Cas in cancer: advantages and challenges. Mil. Med. Res. 10 (1), 32. doi:10.1186/s40779-023-00468-6
Imani, R., Prakash, S., Vali, H., and Faghihi, S. (2018). Polyethylene glycol and octa-arginine dual-functionalized nanographene oxide: an optimization for efficient nucleic acid delivery. Biomaterials Sci. 6 (6), 1636–1650. doi:10.1039/c8bm00058a
Jia, C., Chen, H., Wei, M., Chen, X., Zhang, Y., Cao, L., et al. (2017). Gold nanoparticle-based miR155 antagonist macrophage delivery restores the cardiac function in ovariectomized diabetic mouse model. Int. J. Nanomedicine 12, 4963–4979. doi:10.2147/IJN.S138400
Jia, X., Bai, X., Yang, X., Wang, L., Lu, Y., Zhu, L., et al. (2022). VCAM-1-binding peptide targeted cationic liposomes containing NLRP3 siRNA to modulate LDL transcytosis as a novel therapy for experimental atherosclerosis. Metabolism Clin. Exp. 135, 155274. doi:10.1016/j.metabol.2022.155274
Jiang, T., Xu, L., Zhao, M., Kong, F., Lu, X., Tang, C., et al. (2022). Dual targeted delivery of statins and nucleic acids by chitosan-based nanoparticles for enhanced antiatherosclerotic efficacy. Biomaterials 280, 121324. doi:10.1016/j.biomaterials.2021.121324
Jiao, Y., Xia, Z. L., Ze, L. J., Jing, H., Xin, B., and Fu, S. (2020). Research Progress of nucleic acid delivery vectors for gene therapy. Biomed. Microdevices 22 (1), 16. doi:10.1007/s10544-020-0469-7
Kang, D.Il, Lee, S., Lee, J. T., Sung, B. J., Yoon, J. Y., Kim, J. K., et al. (2011). Preparation and in vitro evaluation of anti-VCAM-1-Fab’-conjugated liposomes for the targeted delivery of the poorly water-soluble drug celecoxib. J. Microencapsul. 28 (3), 220–227. doi:10.3109/02652048.2011.552989
Kaski, J. C., and Consuegra-Sanchez, L. (2013). Evaluation of ASPIRE trial: a Phase III pivotal registration trial, using intracoronary administration of Generx (Ad5FGF4) to treat patients with recurrent angina pectoris. Expert Opin. Biol. Ther. 13 (12), 1749–1753. doi:10.1517/14712598.2013.827656
Kedmi, R., Veiga, N., Ramishetti, S., Goldsmith, M., Rosenblum, D., Dammes, N., et al. (2018). A modular platform for targeted RNAi therapeutics. Nat. Nanotechnol. 13 (3), 214–219. doi:10.1038/s41565-017-0043-5
Khadka, B., Lee, J. Y., Park, D. H., Kim, K. T., and Bae, J. S. (2020). The role of natural compounds and their nanocarriers in the treatment of CNS inflammation. Biomolecules 10 (10), 1401. doi:10.3390/biom10101401
Kim, I.-S., Lee, S. K., Park, Y. M., Lee, Y. B., Shin, S. C., Lee, K. C., et al. (2005). Physicochemical characterization of poly(L-lactic acid) and poly(D,L-lactide-co-glycolide) nanoparticles with polyethylenimine as gene delivery carrier. Int. J. Pharm. 298 (1), 255–262. doi:10.1016/j.ijpharm.2005.04.017
Kim, J., Cao, L., Shvartsman, D., Silva, E. A., and Mooney, D. J. (2011). Targeted delivery of nanoparticles to ischemic muscle for imaging and therapeutic angiogenesis. Nano Lett. 11 (2), 694–700. doi:10.1021/nl103812a
Kim, S.-H., Moon, J. H., Jeong, S. U., Jung, H. H., Park, C. S., Hwang, B. Y., et al. (2019). Induction of antigen-specific immune tolerance using biodegradable nanoparticles containing antigen and dexamethasone. Int. J. Nanomedicine 14, 5229–5242. doi:10.2147/IJN.S210546
Ko, Y. T., Hartner, W. C., Kale, A., and Torchilin, V. P. (2009). Gene delivery into ischemic myocardium by double-targeted lipoplexes with anti-myosin antibody and TAT peptide. Gene Ther. 16 (1), 52–59. doi:10.1038/gt.2008.135
Kon, E., Elia, U., and Peer, D. (2022). Principles for designing an optimal mRNA lipid nanoparticle vaccine. Curr. Opin. Biotechnol. 73 (otsailak), 329–336. doi:10.1016/j.copbio.2021.09.016
Korpela, H., Järveläinen, N., Siimes, S., Lampela, J., Airaksinen, J., Valli, K., et al. (2021). Gene therapy for ischaemic heart disease and heart failure. J. Intern. Med. 290 (3), 567–582. doi:10.1111/joim.13308
Kulkarni, J. A., Witzigmann, D., Leung, J., Tam, Y. Y. C., and Cullis, P. R. (2019). On the role of helper lipids in lipid nanoparticle formulations of siRNA. Nanoscale 11 (45), 21733–21739. doi:10.1039/c9nr09347h
Lanone, S., and Boczkowski, J. (2006). Biomedical applications and potential health risks of nanomaterials: molecular mechanisms. Curr. Mol. Med. 6 (6), 651–663. doi:10.2174/156652406778195026
Lee, M., Kang, S., Kim, S., and Park, N. (2023). Advances and trends in miRNA analysis using DNAzyme-based biosensors. Biosensors 13 (9), 856. doi:10.3390/bios13090856
Lestini, B. J., Sagnella, S. M., Xu, Z., Shive, M. S., Richter, N. J., Jayaseharan, J., et al. (2002). Surface modification of liposomes for selective cell targeting in cardiovascular drug delivery. J. Control. Release Official J. Control. Release Soc. 78 (1–3), 235–247. doi:10.1016/s0168-3659(01)00505-3
Li, D., Gao, C., Kuang, M., Xu, M., Wang, B., Luo, Y., et al. (2021). Nanoparticles as drug delivery systems of RNAi in cancer therapy. Mol. (Basel, Switz. 26 (8), 2380. doi:10.3390/molecules26082380
Li, M., Du, C., Guo, N., Teng, Y., Meng, X., Sun, H., et al. (2019). Composition design and medical application of liposomes. Eur. J. Med. Chem. 164, 640–653. doi:10.1016/j.ejmech.2019.01.007
Li, M., Li, Y., Li, S., Jia, L., Wang, H., et al. (2022a). The nano delivery systems and applications of mRNA. Eur. J. Med. Chem. 227, 113910. doi:10.1016/j.ejmech.2021.113910
Li, W., Gonzalez, K. M., Chung, J., Kim, M., and Lu, J. (2022b). Surface-modified nanotherapeutics targeting atherosclerosis. Biomaterials Sci. 10 (19), 5459–5471. doi:10.1039/d2bm00660j
Lim, M., Badruddoza, A. Z. M., Firdous, J., Azad, M., Mannan, A., Al-Hilal, T. A., et al. (2020). Engineered nanodelivery systems to improve DNA vaccine technologies. Pharmaceutics 12 (1), 30. doi:10.3390/pharmaceutics12010030
Lin, M., and Qi, X. (2023). Advances and challenges of stimuli-responsive nucleic acids delivery system in gene therapy. Pharmaceutics 15 (5), 1450. doi:10.3390/pharmaceutics15051450
Ma, K., Cao, X. X., and Wang, T. Y. (2021). Progress of cationic gene delivery reagents for non-viral vector. Appl. Microbiol. Biotechnol. 105 (2), 525–538. doi:10.1007/s00253-020-11028-6
Ma, S., Tian, X. Y., Zhang, Y., Mu, C., Shen, H., Bismuth, J., et al. (2016). E-selectin-targeting delivery of microRNAs by microparticles ameliorates endothelial inflammation and atherosclerosis. Sci. Rep. 6 (1), 22910. doi:10.1038/srep22910
Manners, N., Priya, V., Mehata, A., Rawat, M., Mohan, S., Makeen, H., et al. (2022). Theranostic nanomedicines for the treatment of cardiovascular and related diseases: current strategies and future perspectives. Pharm. (Basel, Switz. 15 (4), 441. apirilak 1). doi:10.3390/ph15040441
Marcos-Campos, I., Asín, L., Torres, T. E., Marquina, C., Tres, A., Ibarra, M. R., et al. (2011). Cell death induced by the application of alternating magnetic fields to nanoparticle-loaded dendritic cells. Nanotechnology 22 (20), 205101. doi:10.1088/0957-4484/22/20/205101
Markina, Y. V., Kirichenko, T. V., Tolstik, T. V., Bogatyreva, A. I., Zotova, U. S., Cherednichenko, V. R., et al. (2023). Target and cell therapy for atherosclerosis and CVD. Int. J. Mol. Sci. 24 (12), 10308. doi:10.3390/ijms241210308
Mendes, B. B., Conniot, J., Avital, A., Yao, D., Jiang, X., Zhou, X., et al. (2022). Nanodelivery of nucleic acids. Nat. Rev. Methods Prim. 2 (1), 24. apirilak 14. doi:10.1038/s43586-022-00104-y
Mendes, P., Livia, , Pan, J., and Torchilin, V. P. (2017). Dendrimers as nanocarriers for nucleic acid and drug delivery in cancer therapy. Mol. A J. Synthetic Chem. Nat. Prod. Chem. 22 (9), 1401. doi:10.3390/molecules22091401
Messerschmidt, V. L., Chintapula, U., Bonetesta, F., Laboy-Segarra, S., Naderi, A., Nguyen, K. T., et al. (2022). In vivo evaluation of non-viral NICD plasmid-loaded PLGA nanoparticles in developing zebrafish to improve cardiac functions. Front. Physiology 13, 819767. doi:10.3389/fphys.2022.819767
Missri, J. (1979). Hematologic toxicity of drugs used in cardiovascular disease. Postgrad. Med. 65 (1), 165–172. doi:10.1080/00325481.1979.11715031
Mitchell, M. J., Billingsley, M. M., Haley, R. M., Wechsler, M. E., Peppas, N. A., and Langer, R. (2021). Engineering precision nanoparticles for drug delivery. Nat. Rev. Drug Discov. 20 (2), 101–124. doi:10.1038/s41573-020-0090-8
Mohammadpour, R., Dobrovolskaia, M. A., Cheney, D. L., Greish, K. F., and Ghandehari, H. (2019). Subchronic and chronic toxicity evaluation of inorganic nanoparticles for delivery applications. Adv. Drug Deliv. Rev. 144, 112–132. doi:10.1016/j.addr.2019.07.006
Montoto, S., Sebastián, , Muraca, G., and Ruiz, M. E. (2020). Solid lipid nanoparticles for drug delivery: pharmacological and biopharmaceutical aspects. Front. Mol. Biosci. 7, 587997. doi:10.3389/fmolb.2020.587997
Moon, H.-Ho, Joo, M. K., Mok, H., Lee, M., Hwang, K. C., Kim, S. W., et al. (2014). MSC-based VEGF gene therapy in rat myocardial infarction model using facial amphipathic bile acid-conjugated polyethyleneimine. Biomaterials 35 (5), 1744–1754. doi:10.1016/j.biomaterials.2013.11.019
Mukalel, A. J., Riley, R. S., Zhang, R., and Mitchell, M. J. (2019). Nanoparticles for nucleic acid delivery: applications in cancer immunotherapy. Cancer Lett. 458, 102–112. doi:10.1016/j.canlet.2019.04.040
Namdari, M., Cheraghi, M., Negahdari, B., Eatemadi, A., and Daraee, H. (2017). Recent advances in magnetoliposome for heart drug delivery. Artif. Cells, Nanomedicine, Biotechnol. 45 (6), 1051–1057. abuztuak 18. doi:10.1080/21691401.2017.1299159
Nguyen, M.-A., Wyatt, H., Susser, L., Geoffrion, M., Rasheed, A., Duchez, A. C., et al. (2019). Delivery of MicroRNAs by chitosan nanoparticles to functionally alter macrophage cholesterol efflux in vitro and in vivo. ACS Nano 13 (6), 6491–6505. doi:10.1021/acsnano.8b09679
Pala, R., Anju, V., Dyavaiah, M., Busi, S., and Nauli, S. M. (2020). Nanoparticle-mediated drug delivery for the treatment of cardiovascular diseases. Int. J. Nanomedicine 15, 3741–3769. doi:10.2147/IJN.S250872
Park, J.Ho, Dehaini, D., Zhou, J., Holay, M., Fang, R. H., and Zhang, L. (2020). Biomimetic nanoparticle technology for cardiovascular disease detection and treatment. Nanoscale horizons 5 (1), 25–42. doi:10.1039/c9nh00291j
Patel, S., Ryals, R. C., Weller, K. K., Pennesi, M. E., and Sahay, G. (2019). Lipid nanoparticles for delivery of messenger RNA to the back of the eye. J. Control. release official J. Control. Release Soc. 303, 91–100. doi:10.1016/j.jconrel.2019.04.015
Petrisor, G., Ficai, D., Motelica, L., Trusca, R. D., Bîrcă, A. C., Vasile, B. S., et al. (2022). Mesoporous silica materials loaded with gallic acid with antimicrobial potential. Nanomaterials 12 (10), 1648. doi:10.3390/nano12101648
Piotrowski-Daspit, , Alexandra, S., Bracaglia, L. G., and Saltzman, W. M. (2020). Polymeric vehicles for nucleic acid delivery. Adv. Drug Deliv. Rev. 156, 119–132. doi:10.1016/j.addr.2020.06.014
Qian, Y., Shao, R., Guo, N. L., Schwegler-Berry, D., Pacurari, M., et al. (2009). Iron oxide nanoparticles induce human microvascular endothelial cell permeability through reactive oxygen species production and microtubule remodeling. Part. Fibre Toxicol. 6 (1), 1. doi:10.1186/1743-8977-6-1
Ren, S., Wang, M., Wang, C., Wang, Y., Sun, C., Zeng, Z., et al. (2021). Application of non-viral vectors in drug delivery and gene therapy. Polymers 13 (19), 3307. doi:10.3390/polym13193307
Rupei, T., Weihang, Ji, and Chun, W. (2011). Synthesis and characterization of new poly(ortho ester amidine) copolymers for nonviral gene delivery. Polymer 52 (4), 921–932. otsailak 17. doi:10.1016/j.polymer.2010.12.057
Rurik, J. G., Tombácz, I., Yadegari, A., Méndez Fernández, P. O., Shewale, S. V., Li, L., et al. (2022). CAR T cells produced in vivo to treat cardiac injury. Sci. (New York, N.Y.) 375 (6576), 91–96. doi:10.1126/science.abm0594
Sainz-Ramos, M., Gallego, I., Villate-Beitia, I., Zarate, J., Maldonado, I., Puras, G., et al. (2021). How far are non-viral vectors to come of age and reach clinical translation in gene therapy? Int. J. Mol. Sci. 22 (14), 7545. doi:10.3390/ijms22147545
Scalzo, S., Santos, A. K., Ferreira, H. A., Costa, P. A., Prazeres, P. H., da Silva, N. J., et al. (2022). Ionizable lipid nanoparticle-mediated delivery of plasmid DNA in cardiomyocytes. Int. J. Nanomedicine 17, 2865–2881. doi:10.2147/IJN.S366962
Scott, R. C., Rosano, J. M., Ivanov, Z., Wang, B., Chong, P. L. G., Issekutz, A. C., et al. (2009). Targeting VEGF-encapsulated immunoliposomes to MI heart improves vascularity and cardiac function. FASEB J. official Publ. Fed. Am. Soc. Exp. Biol. 23 (10), 3361–3367. doi:10.1096/fj.08-127373
Seo, Y., Lim, H., Park, H., Yu, J., An, J., Yoo, H. Y., et al. (2023). Recent progress of lipid nanoparticles-based lipophilic drug delivery: focus on surface modifications. Pharmaceutics 15 (3), 772. doi:10.3390/pharmaceutics15030772
Sharma, A. R., Kundu, S. K., Nam, J. S., Sharma, G., Priya Doss, C. G., Lee, S. S., et al. (2014). Next generation delivery system for proteins and genes of therapeutic purpose: why and how? » BioMed Res. Int. 2014, 1–11. doi:10.1155/2014/327950
Sharma, D., Arora, S., Singh, J., and Layek, B. (2021). A review of the tortuous path of nonviral gene delivery and recent progress. Int. J. Biol. Macromol. 183, 2055–2073. doi:10.1016/j.ijbiomac.2021.05.192
Shen, J., Rossato, F. A., Cano, I., and Ng, Y. S. E. (2021). Novel engineered, membrane-tethered VEGF-A variants promote formation of filopodia, proliferation, survival, and cord or tube formation by endothelial cells via persistent VEGFR2/ERK signaling and activation of CDC42/ROCK pathways. FASEB J. official Publ. Fed. Am. Soc. Exp. Biol. 35 (12), e22036. doi:10.1096/fj.202100448RR
Shtykalova, S., Deviatkin, D., Freund, S., Egorova, A., and Kiselev, A. (2023). Non-viral carriers for nucleic acids delivery: fundamentals and current applications. Life 13 (4), 903. (apirilak). doi:10.3390/life13040903
Skourtis, D., Stavroulaki, D., Athanasiou, V., Fragouli, P. G., and Iatrou, H. (2020). Nanostructured polymeric, liposomal and other materials to control the drug delivery for cardiovascular diseases. Pharmaceutics 12 (12), 1160. doi:10.3390/pharmaceutics12121160
Song, D., Li, M., Yu, X., Wang, Y., Fan, J., Yang, W., et al. (2022). The molecular pathways of pyroptosis in atherosclerosis. Front. Cell Dev. Biol. 10, 824165. doi:10.3389/fcell.2022.824165
Song, Y., Huang, Z., Xu, J., Ren, D., Wang, Y., Zheng, X., et al. (2014). Multimodal SPION-CREKA peptide based agents for molecular imaging of microthrombus in a rat myocardial ischemia-reperfusion model. Biomaterials 35 (9), 2961–2970. doi:10.1016/j.biomaterials.2013.12.038
Subhan, Md A., Filipczak, N., and Torchilin, V. P. (2023). Advances with lipid-based nanosystems for siRNA delivery to breast cancers. Pharm. (Basel, Switz. 16 (7), 970. doi:10.3390/ph16070970
Sun, Da, and Lu, Z.-R. (2023). Structure and function of cationic and ionizable lipids for nucleic acid delivery. Pharm. Res. 40 (1), 27–46. doi:10.1007/s11095-022-03460-2
Suzuki, Y., and Ishihara, H. (2021). Difference in the lipid nanoparticle technology employed in three approved siRNA (Patisiran) and mRNA (COVID-19 vaccine) drugs. Drug Metabolism Pharmacokinet. 41, 100424. doi:10.1016/j.dmpk.2021.100424
Tang, J., Rakshit, M., Chua, H. M., Darwitan, A., Nguyen, L. T. H., Muktabar, A., et al. (2021). Liposome interaction with macrophages and foam cells for atherosclerosis treatment: effects of size, surface charge and lipid composition. Nanotechnology 32 (50), 505105. doi:10.1088/1361-6528/ac2810
Tang, Lu, Zhang, A., Zhang, Z., Zhao, Q., Li, J., Mei, Y., et al. (2022). Multifunctional inorganic nanomaterials for cancer photoimmunotherapy. Cancer Commun. Lond. Engl. 42 (2), 141–163. doi:10.1002/cac2.12255
Tarin, C., Carril, M., Martin-Ventura, J. L., Markuerkiaga, I., Padro, D., Llamas-Granda, P., et al. (2015). Targeted gold-coated iron oxide nanoparticles for CD163 detection in atherosclerosis by MRI. Sci. Rep. 5, 17135. doi:10.1038/srep17135
Thakor, A. S., Jokerst, J., Zavaleta, C., Massoud, T. F., and Gambhir, S. S. (2011). Gold nanoparticles: a revival in precious metal administration to patients. Nano Lett. 11 (10), 4029–4036. doi:10.1021/nl202559p
Theoharis, S., Krueger, U., Tan, P. H., Haskard, D. O., Weber, M., and George, A. J. (2009). Targeting gene delivery to activated vascular endothelium using anti E/P-Selectin antibody linked to PAMAM dendrimers. J. Immunol. Methods 343 (2), 79–90. doi:10.1016/j.jim.2008.12.005
Tong, L., Liu, D., Cao, Z., Zheng, N., Mao, C., Liu, S., et al. (2023). Research status and prospect of non-viral vectors based on siRNA: a review. Int. J. Mol. Sci. 24 (4), 3375. doi:10.3390/ijms24043375
Tsao, C. W., Aday, A. W., Almarzooq, Z. I., Alonso, A., Beaton, A. Z., Bittencourt, M. S., et al. (2022). Heart disease and stroke statistics—2022 update: a report from the American heart association. Circulation 145 (8), e153–e639. doi:10.1161/CIR.0000000000001052
Wang, F., Gao, L., Meng, L. Y., Xie, J. M., Xiong, J. W., and Luo, Y. (2016). A neutralized noncharged polyethylenimine-based system for efficient delivery of siRNA into heart without toxicity. ACS Appl. Mater. Interfaces 8 (49), 33529–33538. doi:10.1021/acsami.6b13295
Wang, N., Chen, C., Ren, J., and Dai, D. (2023). MicroRNA delivery based on nanoparticles of cardiovascular diseases. Mol. Cell. Biochem., doi:10.1007/s11010-023-04821-0
Wang, Q., Song, Y., Chen, J., Li, Q., Gao, J., Tan, H., et al. (2021). Direct in vivo reprogramming with non-viral sequential targeting nanoparticles promotes cardiac regeneration. Biomaterials 276, 121028. doi:10.1016/j.biomaterials.2021.121028
Wang, T., Zhou, T., Xu, M., Wang, S., Wu, A., Zhang, M., et al. (2022). Platelet membrane-camouflaged nanoparticles carry microRNA inhibitor against myocardial ischaemia‒reperfusion injury. J. Nanobiotechnology 20, 434. doi:10.1186/s12951-022-01639-8
Wang, Z., Tiruppathi, C., Cho, J., Minshall, R. D., and Malik, A. B. (2011). Delivery of nanoparticle: complexed drugs across the vascular endothelial barrier via caveolae. IUBMB life 63 (8), 659–667. doi:10.1002/iub.485
Witzigmann, D., Kulkarni, J. A., Leung, J., Chen, S., Cullis, P. R., and van der Meel, R. (2020). Lipid nanoparticle technology for therapeutic gene regulation in the liver. Adv. Drug Deliv. Rev. 159, 344–363. doi:10.1016/j.addr.2020.06.026
Yagublu, V., Karimova, A., Hajibabazadeh, J., Reissfelder, C., Muradov, M., Bellucci, S., et al. (2022). Overview of physicochemical properties of nanoparticles as drug carriers for targeted cancer therapy. J. Funct. Biomaterials 13 (4), 196. doi:10.3390/jfb13040196
Yan, Yi, Liu, X. Y., Lu, A., Wang, X. Y., Jiang, L. X., and Wang, J. C. (2022). Non-viral vectors for RNA delivery. J. Control. Release Official J. Control. Release Soc. 342, 241–279. doi:10.1016/j.jconrel.2022.01.008
Yang, L., Gong, L., Wang, P., Zhao, X., Zhao, F., Zhang, Z., et al. (2022). Recent advances in lipid nanoparticles for delivery of mRNA. Pharmaceutics 14 (12), 2682. doi:10.3390/pharmaceutics14122682
Yang, P., Ren, J., and Yang, L. (2023). Nanoparticles in the new era of cardiovascular therapeutics: challenges and opportunities. Int. J. Mol. Sci. 24 (6), 5205. doi:10.3390/ijms24065205
Yang, W., Yang, S., Jiang, L., Zhou, Y., Yang, C., and Deng, C. (2020). Tumor microenvironment triggered biodegradation of inorganic nanoparticles for enhanced tumor theranostics. RSC Adv. 10 (45), 26742–26751. doi:10.1039/d0ra04651e
Yetisgin, A. A., Cetinel, S., Zuvin, M., Kosar, A., and Kutlu, O. (2020). Therapeutic nanoparticles and their targeted delivery applications. Molecules 25 (9), 2193. doi:10.3390/molecules25092193
Yihunie, W., Nibret, G., and Aschale, Y. (2023). Recent advances in messenger ribonucleic acid (mRNA) vaccines and their delivery systems: a review. Clin. Pharmacol. Adv. Appl. 15, 77–98. doi:10.2147/CPAA.S418314
Yin, H., Kanasty, R. L., Eltoukhy, A. A., Vegas, A. J., Dorkin, J. R., and Anderson, D. G. (2014). Non-viral vectors for gene-based therapy. Nat. Rev. Genet. 15 (8), 541–555. doi:10.1038/nrg3763
Ylä-Herttuala, S., and Martin, J. F. (2000). Cardiovascular gene therapy. Lancet 355 (9199), 213–222. doi:10.1016/S0140-6736(99)04180-X
Younis, N. K., Ghoubaira, J. A., Bassil, E. P., Tantawi, H. N., and Eid, A. H. (2021). Metal-based nanoparticles: promising tools for the management of cardiovascular diseases. Nanomedicine Nanotechnol. Biol. Med. 36, 102433. doi:10.1016/j.nano.2021.102433
Yu, H., Zhang, R., Shen, M., Zhu, Y., Zhang, Q., et al. (2021). Inhibition of cardiomyocyte apoptosis post-acute myocardial infarction through the efficient delivery of microRNA-24 by silica nanoparticles. Nanoscale Adv. 3 (22), 6379–6385. doi:10.1039/D1NA00568E
Zhai, B.-T., Sun, J., Shi, Y. J., Zhang, X. F., Zou, J. B., Cheng, J. X., et al. (2022). Review targeted drug delivery systems for norcantharidin in cancer therapy. J. Nanobiotechnology 20 (1), 509. doi:10.1186/s12951-022-01703-3
Zhang, H., Bahamondez-Canas, T. F., Zhang, Y., Leal, J., and Smyth, H. D. (2018). PEGylated chitosan for nonviral aerosol and mucosal delivery of the CRISPR/Cas9 system in vitro. Mol. Pharm. 15 (11), 4814–4826. doi:10.1021/acs.molpharmaceut.8b00434
Zhang, J., Lei, W., Meng, Y., Zhou, C., Zhang, B., Yuan, J., et al. (2022a). Expression of PEI-coated gold nanoparticles carrying exogenous gene in periwinkle mesophyll cells and its practice in huanglongbing research. iScience 25 (6), 104479. doi:10.1016/j.isci.2022.104479
Zhang, X., Jin, H., Huang, X., Chaurasiya, B., Dong, D., Shanley, T. P., et al. (2022b). Robust genome editing in adult vascular endothelium by nanoparticle delivery of CRISPR-Cas9 plasmid DNA. Cell Rep. 38 (1), 110196. doi:10.1016/j.celrep.2021.110196
Zhao, Q., Cheng, N., Sun, X., Yan, L., and Li, W. (2023). The application of nanomedicine in clinical settings. Front. Bioeng. Biotechnol. 11, 1219054. doi:10.3389/fbioe.2023.1219054
Zhou, F., Jia, X., Yang, Q., Yang, Y., Zhao, Y., Fan, Y., et al. (2016). Targeted delivery of microRNA-126 to vascular endothelial cells via REDV peptide modified PEG-trimethyl chitosan. Biomaterials Sci. 4 (5), 849–856. doi:10.1039/C5BM00629E
Zhu, C., Ma, J., Ji, Z., Shen, J., and Wang, Q. (2021). Recent advances of cell membrane coated nanoparticles in treating cardiovascular disorders. Molecules 26 (11), 3428. doi:10.3390/molecules26113428
Zhu, K., Guo, C., Xia, Y., Lai, H., Yang, W., Wang, Y., et al. (2013). Transplantation of novel vascular endothelial growth factor gene delivery system manipulated skeletal myoblasts promote myocardial repair. Int. J. Cardiol. 168 (3), 2622–2631. doi:10.1016/j.ijcard.2013.03.041
Zhu, Xi, Tao, W., Liu, D., Wu, J., Guo, Z., Ji, X., et al. (2017). Surface de-PEGylation controls nanoparticle-mediated siRNA delivery in vitro and in vivo. Theranostics 7 (7), 1990–2002. doi:10.7150/thno.18136
Zhuang, X., Qi, Y., Wang, M., Nan, F., Zhang, H., et al. (2020). mRNA vaccines encoding the ha protein of influenza A H1N1 virus delivered by cationic lipid nanoparticles induce protective immune responses in mice. Vaccines 8 (1), 123. (martxoak 10). doi:10.3390/vaccines8010123
Zou, W., Liu, C., Chen, Z., and Zhang, N. (2009). Preparation and characterization of cationic PLA-PEG nanoparticles for delivery of plasmid DNA. Nanoscale Res. Lett. 4 (9), 982–992. doi:10.1007/s11671-009-9345-3
Keywords: non-viral vector, gene therapy, cardiovascular disease, gene transfer, nanotechnology
Citation: Jiao L, Sun Z, Sun Z, Liu J, Deng G and Wang X (2024) Nanotechnology-based non-viral vectors for gene delivery in cardiovascular diseases. Front. Bioeng. Biotechnol. 12:1349077. doi: 10.3389/fbioe.2024.1349077
Received: 04 December 2023; Accepted: 08 January 2024;
Published: 18 January 2024.
Edited by:
Yawei Du, Shanghai Jiao Tong University, ChinaReviewed by:
Uday Chintapula, University of Pennsylvania, United StatesCopyright © 2024 Jiao, Sun, Sun, Liu, Deng and Wang. This is an open-access article distributed under the terms of the Creative Commons Attribution License (CC BY). The use, distribution or reproduction in other forums is permitted, provided the original author(s) and the copyright owner(s) are credited and that the original publication in this journal is cited, in accordance with accepted academic practice. No use, distribution or reproduction is permitted which does not comply with these terms.
*Correspondence: Xiaozhong Wang, d2FuZ3hpYW96aG9uZ0BuY3UuZWR1LmNu
†These authors have contributed equally to this work
Disclaimer: All claims expressed in this article are solely those of the authors and do not necessarily represent those of their affiliated organizations, or those of the publisher, the editors and the reviewers. Any product that may be evaluated in this article or claim that may be made by its manufacturer is not guaranteed or endorsed by the publisher.
Research integrity at Frontiers
Learn more about the work of our research integrity team to safeguard the quality of each article we publish.