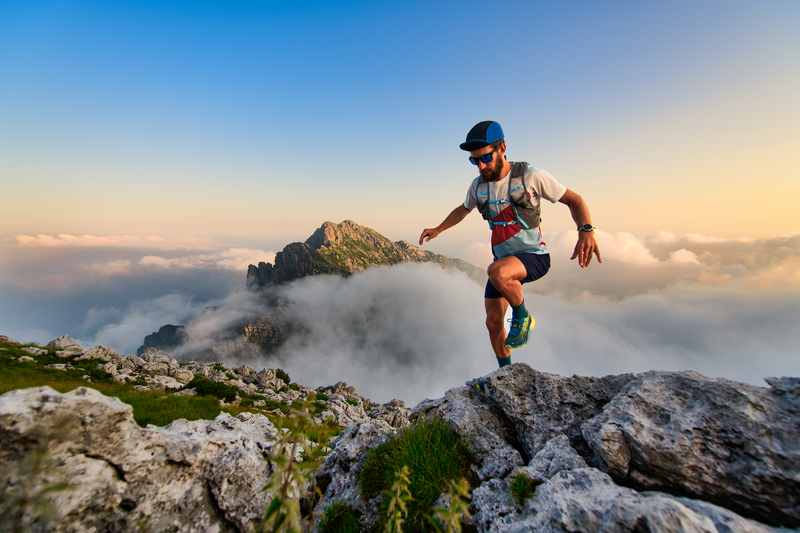
95% of researchers rate our articles as excellent or good
Learn more about the work of our research integrity team to safeguard the quality of each article we publish.
Find out more
REVIEW article
Front. Bioeng. Biotechnol. , 28 February 2024
Sec. Biomaterials
Volume 12 - 2024 | https://doi.org/10.3389/fbioe.2024.1345163
Peripheral nerve injury (PNI) is a common clinical problem, which due to poor recovery often leads to limb dysfunction and sensory abnormalities in patients. Tissue-engineered nerve guidance conduits (NGCs) that are designed and fabricated from different materials are the potential alternative to nerve autografts. However, translation of these NGCs from lab to commercial scale has not been well achieved. Complete functional recovery with the aid of NGCs in PNI becomes a topic of general interest in tissue engineering and regeneration medicine. Electrical stimulation (ES) has been widely used for many years as an effective physical method to promote nerve repair in both pre-clinical and clinical settings. Similarly, ES of conductive and electroactive materials with a broad range of electrical properties has been shown to facilitate the guidance of axons and enhance the regeneration. Graphene and its derivatives possess unique physicochemical and biological properties, which make them a promising outlook for the development of synthetic scaffolds or NGCs for PNI repair, especially in combination with ES. Considering the discussion regarding ES for the treatment of PNI must continue into further detail, herein, we focus on the role of ES in PNI repair and the molecular mechanism behind the ES therapy for PNI, providing a summary of recent advances in context of graphene-based scaffolds (GBSs) in combination with ES. Future perspectives and some challenges faced in developing GBSs are also highlighted with the aim of promoting their clinical applications.
Peripheral nerve injury (PNI) is a common and widespread clinical disease. Acute trauma, autoimmune diseases, local lesions, and infections are all triggers for injuries in the peripheral nervous system (Asthana et al., 2021). Peripheral nerves form an extensive neural network throughout the entire body, connecting the nerve center with target organs and enabling communication between them (Wieringa et al., 2018). Therefore, when PNI occurs, the lack of information transmission poses a severe threat to the mobility and sensory function in distal target organs. Even though peripheral nerves boast the intrinsic capacity to regenerate over small gaps, the slow growth rate of about 1 mm/day results in a limited regeneration of nerve function for nerve injuries more than 3 mm in length (Sunderland, 1947; Mietto et al., 2015; Brugger et al., 2017). After injury, the recovery of the innervation for the distal target organ takes a significant amount of time, and in the case of severe injuries, the distal end of the injured nerve and target organs will atrophy over time, resulting in long-term sensory and motor dysfunction (Fu and Gordon, 1995; Zhang S. et al., 2022).
The slow regeneration speed of peripheral nerves and the poor recovery of nerve function after regeneration have posed challenges in clinical practice for numerous years. Among various treatment methods, autologous nerve transplantation has been considered the gold standard for treating long-gap injuries, albeit the successful rate of recovery following surgery is only 50%, and its clinical application is limited due to the lack of donors and complications at the donor site (Lin and Marra, 2012). Electrical stimulation (ES), among the most popular non-surgical treatment methods, has been widely studied in the field of tissue engineering, both in preclinical and clinical settings. Numerous studies indicate that low-frequency ES delivered post-operatively has a certain positive effect on the repair of peripheral nerve damage, including nerve crush (Foecking et al., 2012), nerve transection (Geremia et al., 2007), and long-gap nerve defects (Huang et al., 2010) in various types of rodents. For example, ES was found to have the ability to accelerate nerve regeneration in combination with steroids, such as testosterone propionate (Sharma et al., 2010). For another example, combined with an reduced graphene oxide (rGO)-coated poly (l-lactic acid-co-caprolactone) (PLCL) microfiber scaffold, ES enhanced neurite outgrowth and alignment of PC-12 cells and primary mouse hippocampal neurons compared to control without ES stimulation (Wang et al., 2020). However, it is important to note that the directionality of the electric field exhibited little contribution to neurite alignment, especially for the neurites outgrowth on PLCL fibers with higher diameters (Wang et al., 2020), instead, it has been observed to enhance nerve fiber growth in random and lead axonal misdirection to incorrect end organs which consequently impaired functional recovery (Gordon and English, 2016). Therefore, other interventions such as tissue-engineered tubular structures, i.e., nerve guidance conduits (NGCs) were employed, which have been widely researched in terms of structural design, materials, and fabrication processes, aiming to provide multi-cuefor neural regeneration (Vijayavenkataraman, 2020; Park et al., 2022). Biocompatible and biodegradable materials with appropriate mechanical properties and desirable conductivity are highly beneficial for the establishment of NGCs in peripheral nerve regeneration. By the application of ES, conductive polymer scaffolds have a good effect on differentiation of nerve stem cells and remyelination of regenerated axons (Ghasemi-Mobarakeh et al., 2009; Song et al., 2019; Sun et al., 2019). However, the artificial polymers with excellent electrical conductivity are non-biodegradable, non-soluble, or brittleness, which inhibits them from clinical translation.
Graphene (Gr) and its derivatives graphene oxide (GO) and rGO possess numerous extraordinary properties for use in tissue engineering of the nervous system (Shin et al., 2016; Bai et al., 2018; Raslan et al., 2020; Bellier et al., 2022). As building blocks, they can assemble into various forms of graphene-based scaffolds (GBSs), such as coating, films/membranes, fibers, foams, hydrogen, conduit, 3D printing and bioprinting products. Combined with ES, the GBSs exhibit a particularly brilliant performance for the treatment of PNI (Chen et al., 2019; Dong et al., 2020; Lu et al., 2023). However, ES through conductive GBSs remains in its infancy based upon the fact that effective and safety of parameters of ES need to be confirmed; related mechanism by which ES and GBSs enhance nerve regeneration and the limitation in PNI repair need to be clarified and overcame. Therefore, this short review starts with a brief understanding the characteristics of PNI and the role of ES in repairing injured nerves, followed by a discussion on recent research advances in the preclinical phase of combining GBSs with ES in promoting nerve regeneration. The GBSs are categorized in view of the assemble precursors, i.e., Gr [including chemical vapor deposition (CVD)-G], GO, and rGO nanosheets, which are given in Table 1. This review attempts to elucidate the features of ES imposed by GBSs and prospect the application of GBSs combined with ES in the area of PNI.
TABLE 1. Graphene-based scaffolds (GBSs) combined with electrical stimulation (ES) for repairing injured peripheral nerve.
In the peripheral nervous system, a peripheral nerve is wrapped by three layers with different constituents and functions, namely, the endoneurium, perineurium, and epineurium (Liu et al., 2018). According to the Sunderland grading system (Sunderland, 1951), PNI is categorized into five types which sorts the nerve injury into five different degrees, and provides a reference for whether surgical intervention is needed: (I) temporary or reversible block (no surgical intervention required/-); (II) axons are damaged, but the endoneurium, perineurium and epineurium entire (−); (III) axons and the endoneurium are damaged, but the perineurium and epineurium are complete (−); (IV) axons, endoneurium, and the perineurium are damaged, but the epineurium is intact (surgical intervention is necessary); (V) severe nerve injury, with nerves divided into two parts (surgical intervention and nerve transplantation are necessary). The selection of repair methods after injury is usually based on the type of nerve injury, the type of target organ, the selectivity of transplanted nerve donors, the location of nerve injury, and the time interval of nerve injury (Schmidhammer et al., 2022).
After PNI, various metabolic, genomic, and biological mechanisms involved in the regeneration of damaged nerve’s structure and function take place (Stoll and Müller, 1999). The destruction of the integrity of the axonal plasma membrane causes a large influx of extracellular calcium and sodium ions into the cytoplasm, leading to the generation of high-frequency action potentials in the proximal axonal region of the cell body, which can retrograde to the cell body (Knott et al., 2014; Rishal and Fainzilber, 2014). Under the mediation of calcium ions (Ca2+), upregulation of regeneration-associated genes (RAGs) occurs through the cyclic adenosine monophosphate (cAMP) signaling pathway, which is crucial for the formation of growth cones (Bradke et al., 2012; Donnelly et al., 2013; Mar et al., 2014). Simultaneously, Wallerian degeneration, a series of molecular and cellular changes, providing a microenvironment conducive to axonal regeneration and reinnervation is crucial for nerve repair after PNI (Scheib and Hoeke, 2013; Conforti et al., 2014) (Figure 1). The cell fragments generated by Wallerian degeneration are cleared by Schwann cells (SCs) and macrophages. Besides, activated SCs form Bands of Büngner through the injury gap, guiding the proximal growth cone to reach the neural tube, thereby achieving regenerative innervation of the target organ (Gordon and English, 2016; Gomez-Sanchez et al., 2017). The inflammatory response is likewise an important aspect of peripheral nerve regeneration (Klimovich et al., 2021). In the early stage of injury, M1 macrophages (pro-inflammatory) are mainly recruited, which can enhance the inflammatory response and promote tissue necrosis; in the later stage, M2 macrophages (anti-inflammatory) play a crucial role in effectively responding to hypoxia, increasing the expression of vascular endothelial growth factor A (VEGF-A), and leading to the proliferation and migration of endothelial cells to the injured site (Cattin et al., 2015; Li et al., 2023).
The rate of axonal regeneration is quite slow. Furthermore, because of Wallerian degeneration, the nerve fiber tube lacks an internal structure for a long time, leading to collapse of the nerve tube and an increase in collagen fibers inside it, which results in a smaller diameter and increased difficulty of nerve regeneration (Krarup et al., 2017). The staggered growth pattern of nerve regeneration, where the regenerated axons germinate from the proximal stump at different times rather than growing all at once (Höke et al., 2002), further delays the regeneration of neurons post-PNI. Prolonged denervation impairs target organ function, leading to target muscle atrophy and persistent sensory disturbance (neuralgia or neurosensitivity), which causes long-term distress for patients (Stassart et al., 2013). Therefore, accelerating axonal regeneration speed and reducing the mismatch of regenerated nerve fibers represent the focal points of current research.
As soon as the PNI occurs, endogenous electric fields are generated correspondingly, which may take part in regulating the rate of nerve sprouting, growth, and regeneration. Applied electric fields have likewise been found to influence the regeneration of nerves after injury, i.e., promote survival, migration, and axonal elongation of neurons, either applied immediately following nerve repair or a perioperative ES (Zarrintaj et al., 2020; Kowtharapu et al., 2021; Trueman et al., 2022). In 1952, Hoffman was the first to apply ES to injured nerve. In his study, a 50–100 Hz sine-wave ES was utilized to the injured sciatic nerve of rats for 10–60 min. Results showed that germination was accelerated in the nerves of partially denervated gastrocnemius and soleus muscles (Hoffman, 1952). Subsequently, Pocket and Gavin subjected the sciatic nerve of rats to compression injury and applied ES with a frequency of 1 Hz for 15 min to 1 h, resulting in faster recovery of the toe extension reflex in the ES group (Pockett and Gavin, 1985). To date, there have been few reports of translation of intraoperative ES therapy to the clinic (Roh et al., 2022). In post-operative intervention, for instance, neuromuscular ES, transcutaneous nerve ES), and functional ES have demonstrated potential to alter neuromuscular activity through an electric field (Ni et al., 2023).
The mechanism by which ES promotes nerve regeneration is not completely understood. Nevertheless, it is widely believed that it may be related to ES promoting intracellular Ca2+ waves, cell membrane potential, membrane receptors, and gap junctions, etc. At axonal injury sites (McGregor and English, 2019; Zuo et al., 2020). The possible pathways related to biological responses to ES are given in Figure 2. For example, In vivo studies show that upregulated brain-derived neurotrophic factor (BDNF) due to the increase in Ca2+ concentration caused by ES and their high affinity receptor tropomyosin receptor kinase B (TrkB) receptor interactions increase the expression of RAGs, such as T-α-1 tubulin and GAP-43, through the cAMP pathway (English et al., 2007; Wang et al., 2011; McGregor and English, 2019). Subsequently, ES activates cAMP response element binding protein (CREB) through phosphokinase A (PKA), inhibits Rho protein expression in the p75-Nogo receptor (p75-NgR) pathway, and upregulates T-α-1 tubulin, hence enhancing cytoskeleton assembly (Sit and Manser, 2011; Yan et al., 2016). Meanwhile, ES can activate CREB to promote axonal extension through another pathway, namely, the p38 mitogen-activated protein kinase (MAPK) pathway. One study applied ES to PC-12 cells with nerve growth factor (NGF) induced axon growth impairment, and found that the CREB activation pathway could be induced by p38 MAPK to promote axon growth (Kawamura and Kano, 2019). Another study has shown that ES also promoted the induction of pluripotent stem cells into neurons, which may be related to the production of novo ciliary neurotrophic factor (CNTF) (Oh et al., 2021). In addition, in vitro experiments indicated that the application of ES (1 Hz, 5 V cm−1) promoted the secretion of neurotrophins by SCs, including NGF and NT-3, via the Ca2+ influx. Moreover, it has been observed that ES supported the transition of macrophages from M1 to M2, effectively clearing myelin debris, alleviating local inflammatory reactions, and providing a favorable microenvironment for axonal regeneration (Mc Lean and Verge, 2016).
FIGURE 2. Possible pathways related to biological responses to electrical stimulation. GF, growth factor; GR, growth receptor; CaM, calmodulin; AC, adenylyl cyclase; cAMP, cyclic adenosine monophosphate; PKA, protein kinase A; ERK, extracellular signal-regulated kinase; FAK, focal adhesion kinase; JNK, c-jun N-terminal kinase; MAPK, mitogen-activated protein kinase; PI3K, phosphatidylinositol-3 kinase; PTEN, phosphate and tensin homolog; Src, steroid receptor coactivator; YAP, yes-associated protein; TAZ, transcriptional coactivator with PDZ-binding motif; ROCK, Rho-associated protein kinase; MAP, microtubules-associated protein; mTOR, mammalian target of rapamycin; FOX1: forkhead box protein 1; GSK3β, glycogen synthase kinase-3. Adapted with permission from Ref (Liu et al., 2021). Copyright 2021, John Wiley and Sons.
In recent years, the application of electroactive materials in the field of peripheral nerve repair has received increasing attention. These materials not only are capable of connecting damaged nerves in the form of scaffolds, providing mechanical support and physical cues, but also simulate the electrophysiological microenvironment of damaged peripheral nerves and transmit biochemical signals through their own electroactive properties (Wang et al., 2022). However, there still is a long way ahead to repair long-gap PNI under ES conditions. Some possible reasons include 1) The stimulation mode (direct current, alternating current, or capacitive coupling), appropriate timing (pre-, peri-, or post-operative), and parameters of ES protocols including frequency, intensity, time, and number of ES have not been standardized (Gryshkov et al., 2021; da Silva et al., 2020), 2) The exact mechanism by which ES and electroactive materials enhance nerve regeneration is relatively unknown, 3) The misdirection of regenerated axons by ES demands of other interventions such as conduits (Gordon and English, 2016), and 4) the complex circumstances with spatial-temporal evolution of nerve injury in vivo become an evident challenge for nervous tissue engineering and regenerative medicine.
Since Andre Geim and Konstantin Novoselov first isolated single-layer graphene in 2004, graphene has been a popular material for modern chemistry and physics applications (Novoselov et al., 2004). In recent years, graphene and its derivatives have received extensive attention as a biomaterial for use in the field of tissue engineering and regenerative medicine owing to their variety of extraordinary properties: 1) Controllable mechanical and electrical properties, either as an enhanced coating/blending in a composite or alone, providing mechanical support and physical guidance of extracellular matrix (ECM); 2) Oxygen-containing functional groups or chemical functionalization, endowing them with excellent chemical properties and hydrophilicity, which provide more chemical cues to interacting with cells of PNS; 3) High specific surface area and unique surface features, providing favorable topography and more bioactive sites for cell anchorage and cytoskeletal remodeling; 4) Antibacterial activity, preventing bacterial growth and formation of biofilm on the surface of an implant; 5) Defects and oxidation-dependence of biodegradation; 6) Facilitated fabrication of two-dimensional coatings or three/four-dimensional architectures.
The biocompatibility of graphene and its derivatives has been widely studied, and there are several related reviews on the topic (Kiew et al., 2016; Liao et al., 2018; Amani et al., 2019; Bullock and Bussy, 2019; Kumar and Parekh, 2020). The toxicity of suspended graphene-based materials was found to be highly dependent on their concentration, size of nanosheets, time of exposure, and surface chemistry. Meanwhile, either as a supporting substrate or an implantable medical device, the parameters of the material surface that influence the cellular response are significantly different from their counterparts in suspension (Kumar and Chatterjee, 2016). Reports on the application of GBSs for tissue regeneration as a promising approach found negligible toxicity on cells in vitro and in rats for periods as long as 18 months (Kumar and Chatterjee, 2016; Qian et al., 2021). Besides the biocompatibility of graphene and its derivatives for biomedical applications, their biodegradability has also been investigated. In recent in vitro and in vivo experiments, the biodegradation of GO and rGO was demonstrated to be defect- and oxidation-dependent, which may pave the way for their applications in nanomedicine and biomedical fields (Bellier et al., 2022). However, GO film is unstable in biological solutions and may lead to uncontrollable biosafety issues. Therefore, the incorporation of GO into a matrix-forming composite would be one of necessary choices. Compared with pristine graphene and GO, rGO-based scaffolds are more favorable for electrical active tissue regeneration owing to their high stability in aqueous solutions and remarkable electrical conductivity (Bullock and Bussy, 2019).
The conductive nature of graphene and its derivatives has generated significant interest in neural tissue engineering in recent years (Kumar and Parekh, 2020). They were found to improve proliferation rate of neural stem cells and induce neuronal differentiation, even without the addition of growth factors. In particular, besides electrical conductivity, GO and rGO have the ECM characteristics of PNS, which enables maintenance of high cellular viability, simulates the neurite outgrowth, regulates the degree of neurite extension and number of neuronal branches, and induces axonal alignment with or without adsorbed proteins such as poly (D-lysine) or laminin. Even though there is a great enthusiasm in exploring GBSs in this field and several review papers have been published on the study of GBSs for PNI repair, (Bei et al., 2019; Bellet et al., 2021; Grijalvo and Díaz, 2021; Aleemardani et al., 2022; Chen et al., 2022; Ławkowska et al., 2022), the discussion of ES and graphene-based materials remains only a footnote in the mentioned reviews above. Therefore, considering the significance of ES for neural scaffolds in tissue engineering and regenerative medicine, our review mainly focuses on the current exploration of GBSs combined with ES in PNI, summarizing their respective characteristics and impact on peripheral nerve repair, aiming to give guidance to current clinical potential.
Graphene is usually prepared by a mechanical and chemical exfoliation technique, and graphene films/foams with a single, few-, or multi-layer structure are prepared by CVD method (Kostarelos and Novoselov, 2014). Besides, a 3D printing technique has also been studied to fabricate 3D graphene for neuronal networks or conduit (Qian et al., 2018). Because of its two-dimensional atomic structure (Figure 3A) and unique electron distribution character, graphene has excellent physical and chemical properties, including a large specific surface area (∼2,630 m2 g−1), higher-than-diamond hardness and elastic modulus almost reaching 1 TPa, good optical behaviors (∼97.4% transmittance), high thermal conductivity (∼5,000 W m−1 K−1, higher than copper), and high electron mobility (∼2 × 105 cm2 V−1 s−1), even higher than that of carbon nanotubes and monocrystalline silicon (Yin et al., 2015; Zhang F. et al., 2022). The unique electrical properties and excellent electrochemical stability make graphene a good candidate for neuronal applications, including neural regeneration (Li et al., 2011; Bendali et al., 2013). In particular, graphene-based composite materials combined with ES accelerate the growth rate of neurons via induction of Ca2+ influx, exhibiting high levels of Tuj1 and MAP2 expressions, which led to the investigation of their potential for neural tissue engineering applications (Feng et al., 2015).
FIGURE 3. (A) Schematic diagram of graphene. Adapted with permission from Ref (Bellier et al., 2022). Copyright 2022, Springer Link. (B) Manufacture of PLCL and GN films with stripe micropatterns and PDA modification and their in vitro and in vivo applicability to accelerate nerve regeneration. Adapted with permission from Ref (Lu et al., 2023). Copyright 2023, John Wiley and Sons. (C) SCs are subjected to ES on graphene/TPU composites, and a direct current of 10 mV is more suitable for the growth and proliferation of SCs. (a) SCs stained with AO/EB. (b) MTT result for SCs proliferation with different voltage. (c) LSCM for SCs with the ES of 10 and 100 mV DC. Adapted with permission from Ref (Huang et al., 2019). Copyright 2019, Royal Society of Chemistry. (D) ES accelerates the migration of rat MSCs inoculated with GCFS, and significantly enhances the regeneration and functional recovery of the sciatic nerve implanted with GCFS nerve-guided conduit. Adapted with permission from Ref (Dong et al., 2020). Copyright 2020, Elsevier.
The combination of graphene and ES has shown significant advantages in the proliferation and differentiation of PC-12 cells, where the graphene was either used as a coating (Jung et al., 2019; Liu et al., 2022) or mixture (Golafshan et al., 2018a; Golafshan et al., 2018b; Zheng et al., 2019; Sun et al., 2021; Huang and Wang, 2023) in the polymer matrix to improve mechanical, electrical or biological properties. Along with an oriented topography, the graphene-based scaffold could further improve cell proliferation and growth direction in vitro (Golafshan et al., 2018a; Golafshan et al., 2018b; Huang and Wang, 2023; Lu et al., 2023). The in vivo implantation of PLCL/PDA/GN conduit into rat sciatic nerve defects, which exhibits both electrical conduction and an axon-guiding surface structure, promoted neural regeneration, myelination, and recovery of motor and sensory functions under the synergistic stimulation of ES (Figure 3B). Besides neurons, applying ES (10 mV, 1 h d−1, 3 times) through graphene-based conductive polymers yields a positive influence on morphologies and proliferation of SCs, which plays an important role in the process of peripheral nerve repair. Li et al. prepared a conductive composite membrane composed of graphene and TPU (Huang et al., 2019). The presence of graphene significantly improved the mechanical properties and conductivity of the membrane. By applying various voltages (10, 50, and 100 mV) of direct current (DC) ES (1 h d−1, 5 times) to the conductive composite containing SCs, a DC voltage of 10 mV was found to be most suitable for survival, synaptic stretching, and the proliferation of SCs (Figure 3C).
The combination of graphene and ES is likewise of great significance for stem cells orienting into specific cell linages. Dong et al. (2020) reported that graphene-based conductive fiber scaffolds (GCFS) prepared by combining different concentrations of graphene with COL and PCL exhibited concentration-dependent conductivity. After studying the effect of GCFS with different graphene contents on neural differentiation of MSCs, they found that a concentration of 1.0 wt% was more conducive to MSCs differentiation into mature neurons; however, higher graphene contents exhibited potential toxicity to the cells. ES promoted the secretion of MSCs and neurotrophic factors. With external stimulation (2 Hz, 20 mV cm−1) of MSCs cultured on the 1.0 wt% GCFS surfaces, a significant promotion of the migration and differentiation into neurons was achieved, even though the promoting effect could not be enhanced with increasing ES intensity. For the in vivo study, they applied a nerve guidance conduit (NGC) made of 1.0 wt% GCFS, combined with ES (2 Hz, 200 mV mm−1, 10 min d−1, 14 times), as a bridging material to the sciatic nerve defect site in rats, achieving satisfactory recovery results. ES was found to promote the regeneration and functional recovery of the sciatic nerve after nerve-guided tube implantation. Current in vitro and in vivo research on ES combined with GBSs with different electrical conductivity provides a reference for their neural tissue engineering applications (Figure 3D) (Dong et al., 2020).
GO is an oxidized graphene derivative produced by oxidizing graphite with sulfuric acid and potassium permanganate under acidic conditions (Dikin et al., 2007). The surface of GO sheets contains oxygen-containing functional groups such as epoxy, carboxyl, and hydroxyl groups (Figure 4A), which give it good hydrophilicity and colloidal stability, making them more suitable for adhesion, proliferation, and differentiation of cells. Simultaneously, oxygen-containing groups enable it to interact with biological molecules such as peptides, DNA, or proteins through physical adsorption or chemical binding that can be modified or functionalized (Sanchez et al., 2012; Shin et al., 2016). As one of the derivatives of graphene, GO also has unique physicochemical properties for biomedical applications, including as scaffolds in regenerative medicine (Raslan et al., 2020). Even though GO is considered to be an electrically insulating material, its electrical conductivity and piezoelectric property can be regulated by its density and types of oxygen-containing groups, and combining with ES, GO has also been explored for use in peripheral nerve repair (De et al., 2022).
FIGURE 4. (A) Schematic diagram of GO. Adapted with permission from Ref (Bellier et al., 2022). Copyright 2022, Springer Link. (B) SEM images of (a) PLLA fiber-film, (b) DBS-doped CCF, (c) DBS-GO-doped CCF, (d) DBS-CFGO-doped CCF. Adapted with permission from Ref (Shang et al., 2019). Copyright 2019, American Chemical Society. (C) Immunofluorescent images and SEM images of neurites from PC-12 cells on three CCFs with ES: (a,b) DBS-doped CCF, (c,d) DBS-GO-doped CCF, (e,f) DBS-CFGO-doped CCF. (g) Neurite alignment percentage. (h) Neurite length of PC-12 cells. Adapted with permission from Ref (Shang et al., 2019). Copyright 2019, American Chemical Society. (D) ES promotes the alignment of SCs along the current direction on PDA/CGO/PPy PLLA membranes. Adapted with permission from Ref (Li et al., 2020). Copyright 2020, Elsevier.
Huang et al. prepared polypyrrole (PPy) conductive composite films (CCFs) doped with GO nanosheets on aligned poly-l-lactic acid (PLLA) fibers using the electrochemical deposition method (Shang et al., 2019; Li et al., 2020). PC-12 cells cultured on the surface of CCFs with administered ES at an intensity of 50 mV cm−1 showed significantly higher neurite length and percentage of alignment than those without ES (Figures 4B, C). The enhanced promotion of neurite elongation and orientation was ascribed to GO sheets that coated the surface of the film, providing electrical and topographical cues for regulating PC-12 cells behavior. In another study, in vitro experiments were carried out on RSC-96 cells (Li et al., 2020). The results indicated that the film had a promoting effect on the expression of neural proteins, and the application of 1 h ES (50 mV cm−1) arranged SCs along the current direction, which was of great significance for peripheral nerve repair (Figure 4D). Furthermore, the study confirmed the synergistic stimulation of ES and conductive conduit with high tensile strength and aligned surface morphology on nerve regeneration and functional recovery (Chen et al., 2019).
rGO can be produced by reducing GO via chemical or thermal treatment. Therefore, rGO exhibits a similar structure to GO, a two-dimensional nanomaterial comprising single-layer sheets of sp2 and sp3 hybridized carbons, with the exception of the decreased amount of oxygen-containing functional groups (Figure 5A) (Hui et al., 2022). Due to the removal of oxygen-containing groups, rGO exhibits higher thermal stability, higher electrical conductivity, and lower cytotoxicity compared to GO, which are important properties for neural tissue engineering and regenerative medicine (Bellier et al., 2022). In recent years, the application of rGO combined with ES in PNI repair has gained increasing attention.
FIGURE 5. (A) Schematic diagram of rGO. Adapted with permission from Ref (Bellier et al., 2022). Copyright 2022, Springer Link. (B) Preparation of graphene IDEs and differentiation of MSC under ES. Adapted with permission from Ref (Aznar-Cervantes et al., 2017). Copyright 2017, John Wiley and Sons. (C) AP/rGO scaffold enhances the migration, proliferation, and myelin formation of SCs. PC-12 cells cultured on the conductive AP/RGO scaffold exhibit high differentiation after ES. AP/RGO neural guide conduit promotes nerve regeneration in vivo. Adapted with permission from Ref (Wang et al., 2019). Copyright 2019, Elsevier. (D) PC-12 cells cultivated with rGO-coated NF demonstrate neurogenicity upon ES. Nerve guidance conduit containing the assembly of rGO-coated NF and ADSC promote the recovery of sciatic nerve injury. Adapted with permission from Ref (Mao et al., 2023). Copyright 2023, Elsevier.
The combination of rGO and ES promotes the differentiation of MSCs into SCs. Das et al. (2017) electrically stimulated (50 Hz, 100 mV, 10 min d−1, 15 times) MSCs using inkjet-printed rGO-based electrodes. The results showed that ES enhances cellular differentiation more than conventional chemical strategies (Das et al., 2017). The circuit made of rGO ink after pulsed-laser processing displayed high conductivity, with a sheet resistance lower than 1 k Ω/sq (Figure 5B). Research has shown that combining rGO-based biomaterials with ES effectively promotes the maturation and differentiation of neurons and regulates the repair function of SCs, which plays an important role in the formation of a peripheral nerve regeneration microenvironment. Wang et al. (2019) coated the rGO onto the surface of an ApF/PLCL nanofiber scaffold through an in-situ redox reaction of GO. In vitro, the scaffold significantly promoted SC migration, proliferation, and myelin formation, including myelin specific gene expression and secretion of neurotrophic factors. PC-12 cells cultured on the conductive scaffolds also exhibited high differentiation ability with the aid of ES. The in vivo performance of implanting AP/rGO NGC into the sciatic nerve defect of rats was similar to that of autologous nerve transplantation (Figure 5C). Liu et al. prepared conductive PLA/rGO/PPy composite nanofibers by the incorporation of rGO into PLA (Dikin et al., 2007). Owing to the presence of conductive PPy and rGO, the conductivity of the composite achieved 1.46 × 10−1 S cm−1, which is higher than some of the graphene-based composites, as shown in Table 1. To study the effect of ES on the proliferation and differentiation of nerve cells seeded on scaffolds with high electrical conductivity, the authors applied electric field strength of 0, 100, 400, and 700 mV cm−1 and 50 Hz for 0.5 h d−1 for 3 days. The electric intensity of 400 mV cm−1 was found to be most favorable for cell proliferation, differentiation, and neurite growth. Most recently, Mao et al. (2023) developed a conductive NGC for better nerve regeneration compared to their previous study through filling with PCL NF that was coated by rGO layers. In vitro study demonstrated the excellent cytocompatibility of rGO@NF with 30 layers of rGO, which exhibited the highest electrical conductivity and promoted PC-12 cells extension and neurite outgrowth in the presence of ES. Further, transplantation of the NGC in vivo to bridge the nerve defect in a Sprague Dawley rat model accelerated nerve regeneration to a greater extent compared to bridging the fractured nerve by a hollow NGC (Figure 5D). All the results above indicate that ES combined with rGO is an efficient strategy to develop an artificial implant for long-gap PNI repair.
As a common clinical disease, the incidence rate of PNI has experienced an upward trend in recent years. ES is considered an effective treatment for PNI, and has been extensively studied in the preclinical stage. GBSs represent promising media and carriers for ES, owing to their excellent electrical conductivity and mechanical properties. The combination of GBSs and ES has shown encouraging effects in promoting stem cell differentiation, inducing neuronal repair, and promoting the proliferation, migration, and maturation of SCs. However, their practical applications have certain limitations that must be overcome. First, research to date is still limited to the preclinical stage, and most reports concern in vitro studies. Meanwhile, a large amount of in vivo and clinical translation data is needed to support a next-generation scaffold. Furthermore, there are still challenges in the biocompatibility of GBSs, and long-term safety in vivo is of particular significance for GBSs in future clinical applications. In addition, low-frequency or direct current electric fields are currently chosen to administer post-operational ES through GBSs. However, given the importance of balance between conductivity and ES for a proper neural regeneration, stimulation settings of the intensity of the applied ES through GBSs—in particular 3D-graphene or 3D-reduced graphene oxide with superior electrical conductivity must be carefully taken into account. In addition, the potential for GBSs piezoelectricity is still to be extensively explored to provide an effective platform for a wireless or non-invasive repair of PNI.
GBSs have been reported to assist in regulating neuronal excitability, which has a significant impact on neuronal repair and axonal regeneration. Simultaneously, studies indicate that GBSs can serve as a bridge to connect nerve defect sites and help transmit chemical signals between cells, promoting nerve regeneration. Further, reports showed their ability to improve the microenvironment of nerve repair, promote angiogenesis, and regulate immune responses. The GBSs with their unique topography and surface structure exhibit strong effect on the morphology and differentiation of stem cells into neurons. Even though the biodegradation of graphene and its derivatives is still a challenging issue for applications in tissue engineered grafts, the development of neural prosthesis or non-degradable flexible electronics for long-term applications for next stage of nerve guidance conduit or neural electrodes may have opened up numerous opportunities to GBSs for an optimal recovery in patients with PNI.
YZ: Formal Analysis, Resources, Writing–original draft, Writing–review and editing. YL: Data curation, Funding acquisition, Project administration, Writing–original draft. SK: Methodology, Software, Writing–original draft. DS: Data curation, Writing–review and editing. YL: Data curation, Writing–original draft. XW: Supervision, Writing–review and editing. LL: Supervision, Writing–review and editing.
The author(s) declare financial support was received for the research, authorship, and/or publication of this article. This work was financially supported by the Project of the Natural Science Foundation of Jilin Province (20210101381JC), the Department of Science and Technology of Jilin Province (YDZJ202201ZYTS673), and the National Natural Science Foundation of China (No. 81901245).
The authors declare that the research was conducted in the absence of any commercial or financial relationships that could be construed as a potential conflict of interest.
All claims expressed in this article are solely those of the authors and do not necessarily represent those of their affiliated organizations, or those of the publisher, the editors and the reviewers. Any product that may be evaluated in this article, or claim that may be made by its manufacturer, is not guaranteed or endorsed by the publisher.
Aleemardani, M., Zare, P., Seifalian, A., Bagher, Z., and Seifalian, A. M. (2022). Graphene-based materials prove to Be a promising candidate for nerve regeneration following peripheral nerve injury. Biomedicines 10, 73. doi:10.3390/biomedicines10010073
Amani, H., Mostafavi, E., Arzaghi, H., Davaran, S., Akbarzadeh, A., Akhavan, O., et al. (2019). Three-dimensional graphene foams: synthesis, properties, biocompatibility, biodegradability, and applications in tissue engineering. Acs Biomaterials Sci. Eng. 5, 193–214. doi:10.1021/acsbiomaterials.8b00658
Asthana, P., Zhang, G., Sheikh, K. A., and Ma, C. H. E. (2021). Heat shock protein is a key therapeutic target for nerve repair in autoimmune peripheral neuropathy and severe peripheral nerve injury. Brain Behav. Immun. 91, 48–64. doi:10.1016/j.bbi.2020.08.020
Aznar-Cervantes, S., Pagan, A., Martinez, J. G., Bernabeu-Esclapez, A., Otero, T. F., Meseguer-Olmo, L., et al. (2017). Electrospun silk fibroin scaffolds coated with reduced graphene promote neurite outgrowth of PC-12 cells under electrical stimulation. Mater. Sci. Eng. C-Materials Biol. Appl. 79, 315–325. doi:10.1016/j.msec.2017.05.055
Bai, R. G., Ninan, N., Muthoosamy, K., and Manickam, S. (2018). Graphene: a versatile platform for nanotheranostics and tissue engineering. Prog. Mater. Sci. 91, 24–69. doi:10.1016/j.pmatsci.2017.08.004
Bei, H. P., Yang, Y., Zhang, Q., Tian, Y., Luo, X., Yang, M., et al. (2019). Graphene-based nanocomposites for neural tissue engineering. Molecules 24, 658. doi:10.3390/molecules24040658
Bellet, P., Gasparotto, M., Pressi, S., Fortunato, A., Scapin, G., Mba, M., et al. (2021). Graphene-based scaffolds for regenerative medicine. Nanomaterials 11, 404. doi:10.3390/nano11020404
Bellier, N., Baipaywad, P., Ryu, N., Lee, J. Y., and Park, H. (2022). Recent biomedical advancements in graphene oxide- and reduced graphene oxide-based nanocomposite nanocarriers. Biomaterials Res. 26, 65. doi:10.1186/s40824-022-00313-2
Bendali, A., Hess, L. H., Seifert, M., Forster, V., Stephan, A.-F., Garrido, J. A., et al. (2013). Purified neurons can survive on peptide-free graphene layers. Adv. Healthc. Mater. 2, 929–933. doi:10.1002/adhm.201200347
Bradke, F., Fawcett, J. W., and Spira, M. E. (2012). Assembly of a new growth cone after axotomy: the precursor to axon regeneration. Nat. Rev. Neurosci. 13, 183–193. doi:10.1038/nrn3176
Brugger, V., Duman, M., Bochud, M., Munger, E., Heller, M., Ruff, S., et al. (2017). Delaying histone deacetylase response to injury accelerates conversion into repair Schwann cells and nerve regeneration. Nat. Commun. 8, 14272. doi:10.1038/ncomms14272
Bullock, C. J., and Bussy, C. (2019). Biocompatibility considerations in the design of graphene biomedical materials. Adv. Mater. Interfaces 6. doi:10.1002/admi.201900229
Cattin, A.-L., Burden, J. J., Van Emmenis, L., Mackenzie, F. E., Hoving, J. J. A., Calavia, N. G., et al. (2015). Macrophage-induced blood vessels guide Schwann cell-mediated regeneration of peripheral nerves. Cell 162, 1127–1139. doi:10.1016/j.cell.2015.07.021
Chen, C., Xi, Y., and Weng, Y. (2022). Progress in the development of graphene-based biomaterials for tissue engineering and regeneration. Materials 15, 2164. doi:10.3390/ma15062164
Chen, X., Liu, C., Huang, Z., Pu, X., Shang, L., Yin, G., et al. (2019). Preparation of carboxylic graphene oxide-composited polypyrrole conduits and their effect on sciatic nerve repair under electrical stimulation. J. Biomed. Mater. Res. Part A 107, 2784–2795. doi:10.1002/jbm.a.36781
Chen, X., Ranjan, V. D., Liu, S., Liang, Y. N., Lim, J. S. K., Chen, H., et al. (2021). In situ formation of 3D conductive and cell-laden graphene hydrogel for electrically regulating cellular behavior. Macromol. Biosci. 21, e2000374. doi:10.1002/mabi.202000374
Conforti, L., Gilley, J., and Coleman, M. P. (2014). Wallerian degeneration: an emerging axon death pathway linking injury and disease. Nat. Rev. Neurosci. 15, 394–409. doi:10.1038/nrn3680
Das, S. R., Uz, M., Ding, S., Lentner, M. T., Hondred, J. A., Cargill, A. A., et al. (2017). Electrical differentiation of mesenchymal stem cells into schwann-cell-like phenotypes using inkjet-printed graphene circuits. Adv. Healthc. Mater. 6. doi:10.1002/adhm.201601087
da Silva, L. P., Kundu, S. C., Reis, R. L., and Correlo, V. M. (2020). Electric phenomenon: a disregarded tool in tissue engineering and regenerative medicine. Trends Biotechnol. 38, 24–49. doi:10.1016/j.tibtech.2019.07.002
De, I., Sharma, P., and Singh, M. (2022). Emerging approaches of neural regeneration using physical stimulations solely or coupled with smart piezoelectric nano-biomaterials. Eur. J. Pharm. Biopharm. 173, 73–91. doi:10.1016/j.ejpb.2022.02.016
Dikin, D. A., Stankovich, S., Zimney, E. J., Piner, R. D., Dommett, G. H. B., Evmenenko, G., et al. (2007). Preparation and characterization of graphene oxide paper. Nature 448, 457–460. doi:10.1038/nature06016
Dong, C., Qiao, F., Hou, W., Yang, L., and Lv, Y. (2020). Graphene-based conductive fibrous scaffold boosts sciatic nerve regeneration and functional recovery upon electrical stimulation. Appl. Mater. Today 21, 100870. doi:10.1016/j.apmt.2020.100870
Donnelly, C. J., Park, M., Spillane, M., Yoo, S., Pacheco, A., Gomes, C., et al. (2013). Axonally synthesized β-actin and GAP-43 proteins support distinct modes of axonal growth. J. Neurosci. 33, 3311–3322. doi:10.1523/jneurosci.1722-12.2013
English, A. W., Schwartz, G., Meador, W., Sabatier, M. J., and Mulligan, A. (2007). Electrical stimulation promotes peripheral axon regeneration by enhanced neuronal neurotrophin signaling. Dev. Neurobiol. 67, 158–172. doi:10.1002/dneu.20339
Feng, Z.-Q., Wang, T., Zhao, B., Li, J., and Jin, L. (2015). Soft graphene nanofibers designed for the acceleration of nerve growth and development. Adv. Mater. 27, 6462–6468. doi:10.1002/adma.201503319
Foecking, E. M., Fargo, K. N., Coughlin, L. M., Kim, J. T., Marzo, S. J., and Jones, K. J. (2012). Single session of brief electrical stimulation immediately following crush injury enhances functional recovery of rat facial nerve. J. Rehabilitation Res. Dev. 49, 451–458. doi:10.1682/jrrd.2011.03.0033
Fu, S. Y., and Gordon, T. (1995). Contributing factors to poor functional recovery after delayed nerve repair - prolonged denervation. J. Neurosci. 15, 3886–3895. doi:10.1523/jneurosci.15-05-03886.1995
Geremia, N. M., Gordon, T., Brushart, T. M., Al-Majed, A. A., and Verge, V. M. K. (2007). Electrical stimulation promotes sensory neuron regeneration and growth-associated gene expression. Exp. Neurol. 205, 347–359. doi:10.1016/j.expneurol.2007.01.040
Ghasemi-Mobarakeh, L., Prabhakaran, M. P., Morshed, M., Nasr-Esfahani, M. H., and Ramakrishna, S. (2009). Electrical stimulation of nerve cells using conductive nanofibrous scaffolds for nerve tissue engineering. Tissue Eng. Part A 15, 3605–3619. doi:10.1089/ten.tea.2008.0689
Golafshan, N., Kharaziha, M., and Alehosseini, M. (2018a). A three-layered hollow tubular scaffold as an enhancement of nerve regeneration potential. Biomed. Mater. 13, 065005. doi:10.1088/1748-605X/aad8da
Golafshan, N., Kharaziha, M., Fathi, M., Larson, B. L., Giatsidis, G., and Masoumi, N. (2018b). Anisotropic architecture and electrical stimulation enhance neuron cell behaviour on a tough graphene embedded PVA: alginate fibrous scaffold. Rsc Adv. 8, 6381–6389. doi:10.1039/c7ra13136d
Gomez-Sanchez, J. A., Pilch, K. S., van der Lans, M., Fazal, S. V., Benito, C., Wagstaff, L. J., et al. (2017). After nerve injury, lineage tracing shows that myelin and remak Schwann cells elongate extensively and branch to form repair Schwann cells, which shorten radically on remyelination. J. Neurosci. 37, 9086–9099. doi:10.1523/jneurosci.1453-17.2017
Gordon, T., and English, A. W. (2016). Strategies to promote peripheral nerve regeneration: electrical stimulation and/or exercise. Eur. J. Neurosci. 43, 336–350. doi:10.1111/ejn.13005
Grijalvo, S., and Díaz, D. D. (2021). Graphene-based hybrid materials as promising scaffolds for peripheral nerve regeneration. Neurochem. Int. 147, 105005. doi:10.1016/j.neuint.2021.105005
Gryshkov, O., Al Halabi, F., Kuhn, A. I., Leal-Marin, S., Freund, L. J., Forthmann, M., et al. (2021). PVDF and P(VDF-TrFE) electrospun scaffolds for nerve graft engineering: a comparative study on piezoelectric and structural properties, and in vitro biocompatibility. Int. J. Mol. Sci. 22, 11373. doi:10.3390/ijms222111373
Hoffman, H., and Binet, F. (1952). Acceleration and retardatton of the process of axon-sprouttng in partially denervated muscles. Aust. J. Exp. Biol. Med. Sci. 30, 541–566. doi:10.1038/icb.1952.52
Höke, A., Gordon, T., Zochodne, D. W., and Sulaiman, O. A. R. (2002). A decline in glial cell-line-derived neurotrophic factor expression is associated with impaired regeneration after long-term Schwann cell denervation. Exp. Neurol. 173, 77–85. doi:10.1006/exnr.2001.7826
Huang, J., Lu, L., Hu, X., Ye, Z., Peng, Y., Yan, X., et al. (2010). Electrical stimulation accelerates motor functional recovery in the rat model of 15-mm sciatic nerve gap bridged by scaffolds with longitudinally oriented microchannels. Neurorehabilitation Neural Repair 24, 736–745. doi:10.1177/1545968310368686
Huang, W.-J., and Wang, J. (2023). Development of 3D-printed, biodegradable, conductive PGSA composites for nerve tissue regeneration. Macromol. Biosci. 23, e2200470. doi:10.1002/mabi.202200470
Huang, Z., Guo, Z., Sun, M., Fang, S., and Li, H. (2019). A study on graphene composites for peripheral nerve injury repair under electrical stimulation. Rsc Adv. 9, 28627–28635. doi:10.1039/c9ra04855c
Huang, Z., Sun, M., Li, Y., Guo, Z., and Li, H. (2021). Reduced graphene oxide-coated electrospun fibre: effect of orientation, coverage and electrical stimulation on Schwann cells behavior. J. Mater. Chem. B 9, 2656–2665. doi:10.1039/d1tb00054c
Hui, Y., Yan, Z., Yang, H., Xu, X., Yuan, W.-E., and Qian, Y. (2022). Graphene family nanomaterials for stem cell neurogenic differentiation and peripheral nerve regeneration. Acs Appl. Bio Mater. 5, 4741–4759. doi:10.1021/acsabm.2c00663
Jung, H. S., Kim, H. H., Shin, M. H., Kim, S., Kim, K. S., Cho, K., et al. (2019). Electroceutical residue-free graphene device for dopamine monitoring and neural stimulation. Acs Biomaterials Sci. Eng. 5, 2013–2020. doi:10.1021/acsbiomaterials.8b01488
Kawamura, K., and Kano, Y. (2019). Electrical stimulation induces neurite outgrowth in PC12m3 cells via the p38 mitogen-activated protein kinase pathway. Neurosci. Lett. 698, 81–84. doi:10.1016/j.neulet.2019.01.015
Kiew, S. F., Kiew, L. V., Lee, H. B., Imae, T., and Chung, L. Y. (2016). Assessing biocompatibility of graphene oxide-based nanocarriers: a review. J. Control. Release 226, 217–228. doi:10.1016/j.jconrel.2016.02.015
Klimovich, P., Rubina, K., Sysoeva, V., and Semina, E. (2021). New Frontiers in peripheral nerve regeneration: concerns and remedies. Int. J. Mol. Sci. 22, 13380. doi:10.3390/ijms222413380
Knott, E. P., Assi, M., and Pearse, D. D. (2014). Cyclic amp signaling: a molecular determinant of peripheral nerve regeneration. Biomed Res. Int. 2014, 1–8. doi:10.1155/2014/651625
Kostarelos, K., and Novoselov, K. S. (2014). Exploring the interface of graphene and biology. Science 344, 261–263. doi:10.1126/science.1246736
Kowtharapu, B. S., Damaraju, J., Singh, N. K., Ziebart, J., Bader, R., Koczan, D., et al. (2021). Analysis of the differential gene and protein expression profiles of corneal epithelial cells stimulated with alternating current electric fields. Genes 12, 299. doi:10.3390/genes12020299
Krarup, C., Rosen, B., Boeckstyns, M., Sorensen, A. I., Lundborg, G., Moldovan, M., et al. (2017). Sensation, mechanoreceptor, and nerve fiber function after nerve regeneration. Ann. Neurology 82, 940–950. doi:10.1002/ana.25102
Kumar, S., and Chatterjee, K. (2016). Comprehensive review on the use of graphene-based substrates for regenerative medicine and biomedical devices. Acs Appl. Mater. Interfaces 8, 26431–26457. doi:10.1021/acsami.6b09801
Kumar, S., and Parekh, S. H. (2020). Linking graphene-based material physicochemical properties with molecular adsorption, structure and cell fate. Commun. Chem. 3, 8. doi:10.1038/s42004-019-0254-9
Ławkowska, K., Pokrywczynska, M., Koper, K., Kluth, L. A., Drewa, T., and Adamowicz, J. (2022). Application of graphene in tissue engineering of the nervous system. Int. J. Mol. Sci. 23, 33. doi:10.3390/ijms23010033
Li, N., Zhang, X., Song, Q., Su, R., Zhang, Q., Kong, T., et al. (2011). The promotion of neurite sprouting and outgrowth of mouse hippocampal cells in culture by graphene substrates. Biomaterials 32, 9374–9382. doi:10.1016/j.biomaterials.2011.08.065
Li, Y., Huang, Z., Pu, X., Chen, X., Yin, G., Wang, Y., et al. (2020). Polydopamine/carboxylic graphene oxide-composited polypyrrole films for promoting adhesion and alignment of Schwann cells. Colloids Surfaces B-Biointerfaces 191, 110972. doi:10.1016/j.colsurfb.2020.110972
Li, Z., Jiang, Z., Lu, L., and Liu, Y. (2023). Microfluidic manipulation for biomedical applications in the central and peripheral nervous systems. Pharmaceutics 15, 210. doi:10.3390/pharmaceutics15010210
Liao, C., Li, Y., and Tjong, S. C. (2018). Graphene nanomaterials: synthesis, biocompatibility, and cytotoxicity. Int. J. Mol. Sci. 19, 3564. doi:10.3390/ijms19113564
Lin, Y.-C., and Marra, K. G. (2012). Injectable systems and implantable conduits for peripheral nerve repair. Biomed. Mater. 7, 024102. doi:10.1088/1748-6041/7/2/024102
Liu, Q., Wang, X., and Yi, S. (2018). Pathophysiological changes of physical barriers of peripheral nerves after injury. Front. Neurosci. 12, 597. doi:10.3389/fnins.2018.00597
Liu, R., Huang, X., Wang, X., Peng, X., Zhang, S., Liu, Y., et al. (2021). Electrical stimulation mediated the neurite outgrowth of PC-12 cells on the conductive polylactic acid/reduced graphene oxide/polypyrrole composite nanofibers. Appl. Surf. Sci. 560, 149965. doi:10.1016/j.apsusc.2021.149965
Liu, R., Xu, Z., Zhao, C., Zhang, S., Zhou, H., Zhou, L., et al. (2022). Mediate neurite outgrowth of PC-12 cells using polypyrrole-assisted laser-induced graphene flexible composite electrodes combined with electrical stimulation. Eur. Polym. J. 181, 111634. doi:10.1016/j.eurpolymj.2022.111634
Lu, S., Chen, W., Wang, J., Guo, Z., Xiao, L., Wei, L., et al. (2023). Polydopamine-decorated PLCL conduit to induce synergetic effect of electrical stimulation and topological morphology for peripheral nerve regeneration. Small Methods 7, e2200883. doi:10.1002/smtd.202200883
Mao, W., Lee, E., Cho, W., Kang, B.-J., and Yoo, H. S. (2023). Cell-directed assembly of luminal nanofibril fillers in nerve conduits for peripheral nerve repair. Biomaterials 301, 122209. doi:10.1016/j.biomaterials.2023.122209
Mar, F. M., Bonni, A., and Sousa, M. M. (2014). Cell intrinsic control of axon regeneration. Embo Rep. 15, 254–263. doi:10.1002/embr.201337723
McGregor, C. E., and English, A. W. (2019). The role of BDNF in peripheral nerve regeneration: activity-dependent treatments and Val66Met. Front. Cell. Neurosci. 12, 522. doi:10.3389/fncel.2018.00522
Mc Lean, N. A., and Verge, V. M. K. (2016). Dynamic impact of brief electrical nerve stimulation on the neural immune axis-polarization of macrophages toward a pro-repair phenotype in demyelinated peripheral nerve. Glia 64, 1546–1561. doi:10.1002/glia.23021
Mietto, B. S., Mostacada, K., and Blanco Martinez, A. M. (2015). Neurotrauma and inflammation: CNS and PNS responses. Mediat. Inflamm. 2015, 1–14. doi:10.1155/2015/251204
Ni, L., Yao, Z., Zhao, Y., Zhang, T., Wang, J., Li, S., et al. (2023). Electrical stimulation therapy for peripheral nerve injury. Front. Neurology 14, 1081458. doi:10.3389/fneur.2023.1081458
Novoselov, K. S., Geim, A. K., Morozov, S. V., Jiang, D., Zhang, Y., Dubonos, S. V., et al. (2004). Electric field effect in atomically thin carbon films. Science 306, 666–669. doi:10.1126/science.1102896
Oh, B., Wu, Y., Swaminathan, V., Lam, V., Ding, J., and George, P. M. (2021). Modulating the electrical and mechanical microenvironment to guide neuronal stem cell differentiation. Adv. Sci. 8 (7), 2002112. doi:10.1002/advs.202002112
Parandeh, S., Kharaziha, M., Karimzadeh, F., and Hosseinabadi, F. (2020). Triboelectric nanogenerators based on graphene oxide coated nanocomposite fibers for biomedical applications. Nanotechnology 31, 385402. doi:10.1088/1361-6528/ab9972
Park, H.-J., Hong, H., Thangam, R., Song, M.-G., Kim, J.-E., Jo, E.-H., et al. (2022). Static and dynamic biomaterial engineering for cell modulation. Nanomaterials 12, 1377. doi:10.3390/nano12081377
Pockett, S., and Gavin, R. M. (1985). Acceleration of peripheral nerve regeneration after crush injury in rat. Neurosci. Lett. 59, 221–224. doi:10.1016/0304-3940(85)90203-4
Qian, Y., Wang, X., Song, J., Chen, W., Chen, S., Jin, Y., et al. (2021). Preclinical assessment on neuronal regeneration in the injury-related microenvironment of graphene-based scaffolds. Npj Regen. Med. 6, 31. doi:10.1038/s41536-021-00142-2
Qian, Y., Zhao, X., Han, Q., Chen, W., Li, H., and Yuan, W. (2018). An integrated multi-layer 3D-fabrication of PDA/RGD coated graphene loaded PCL nanoscaffold for peripheral nerve restoration. Nat. Commun. 9, 323. doi:10.1038/s41467-017-02598-7
Raslan, A., Saenz del Burgo, L., Ciriza, J., and Luis Pedraz, J. (2020). Graphene oxide and reduced graphene oxide-based scaffolds in regenerative medicine. Int. J. Pharm. 580, 119226. doi:10.1016/j.ijpharm.2020.119226
Rishal, I., and Fainzilber, M. (2014). Axon-soma communication in neuronal injury. Nat. Rev. Neurosci. 15, 32–42. doi:10.1038/nrn3609
Roh, J., Schellhardt, L., Keane, G. C., Hunter, D. A., Moore, A. M., Snyder-Warwick, A. K., et al. (2022). Short-duration, pulsatile, electrical stimulation therapy accelerates axon regeneration and recovery following tibial nerve injury and repair in rats. Plastic Reconstr. Surg. 149, 681E–690E. doi:10.1097/prs.0000000000008924
Sanchez, V. C., Jachak, A., Hurt, R. H., and Kane, A. B. (2012). Biological interactions of graphene-family nanomaterials: an interdisciplinary review. Chem. Res. Toxicol. 25, 15–34. doi:10.1021/tx200339h
Scheib, J., and Hoeke, A. (2013). Advances in peripheral nerve regeneration. Nat. Rev. Neurol. 9, 668–676. doi:10.1038/nrneurol.2013.227
Schmidhammer, R., Rosenauer, R., and Hausner, T. (2022). “Surgical techniques in nerve repair,” in Peripheral nerve tissue engineering and regeneration. Editors J. B. Phillips, D. Hercher, and T. Hausner (Cham: Springer International Publishing), 467–490. doi:10.1007/978-3-030-21052-6_13
Shang, L., Huang, Z., Pu, X., Yin, G., and Chen, X. (2019). Preparation of graphene oxide-doped polypyrrole composite films with stable conductivity and their effect on the elongation and alignment of neurite. Acs Biomaterials Sci. Eng. 5, 1268–1278. doi:10.1021/acsbiomaterials.8b01326
Sharma, N., Marzo, S. J., Jones, K. J., and Foecking, E. M. (2010). Electrical stimulation and testosterone differentially enhance expression of regeneration-associated genes. Exp. Neurol. 223, 183–191. doi:10.1016/j.expneurol.2009.04.031
Shin, S. R., Li, Y.-C., Jang, H. L., Khoshakhlagh, P., Akbari, M., Nasajpour, A., et al. (2016). Graphene-based materials for tissue engineering. Adv. Drug Deliv. Rev. 105, 255–274. doi:10.1016/j.addr.2016.03.007
Sit, S.-T., and Manser, E. (2011). Rho GTPases and their role in organizing the actin cytoskeleton. J. Cell Sci. 124, 679–683. doi:10.1242/jcs.064964
Song, S., Amores, D., Chen, C., McConnell, K., Oh, B., Poon, A., et al. (2019). Controlling properties of human neural progenitor cells using 2D and 3D conductive polymer scaffolds. Sci. Rep. 9, 19565. doi:10.1038/s41598-019-56021-w
Stassart, R. M., Fledrich, R., Velanac, V., Brinkmann, B. G., Schwab, M. H., Meijer, D., et al. (2013). A role for Schwann cell-derived neuregulin-1 in remyelination. Nat. Neurosci. 16, 48–54. doi:10.1038/nn.3281
Stoll, G., and Müller, H. W. (1999). Nerve injury, axonal degeneration and neural regeneration:: basic insights. Brain Pathol. 9, 313–325. doi:10.1111/j.1750-3639.1999.tb00229.x
Sun, Y., Liu, X., George, M. N., Park, S., Gaihre, B., Terzic, A., et al. (2021). Enhanced nerve cell proliferation and differentiation on electrically conductive scaffolds embedded with graphene and carbon nanotubes. J. Biomed. Mater. Res. Part A 109, 193–206. doi:10.1002/jbm.a.37016
Sun, Y., Quan, Q., Meng, H., Zheng, Y., Peng, J., Hu, Y., et al. (2019). Enhanced neurite outgrowth on a multiblock conductive nerve scaffold with self-powered electrical stimulation. Adv. Healthc. Mater. 8, e1900127. doi:10.1002/adhm.201900127
Sunderland, S. (1947). Rate of regeneration in human peripheral nerves; analysis of the interval between injury and onset of recovery. Archives neurology psychiatry 58, 251–295. doi:10.1001/archneurpsyc.1947.02300320002001
Sunderland, S. (1951). A classification of peripheral nerve injuries producing loss of function. Brain 74, 491–516. doi:10.1093/brain/74.4.491
Trueman, R. P., Ahlawat, A. S., and Phillips, J. B. (2022). A shock to the (nervous) system: bioelectricity within peripheral nerve tissue engineering. Tissue Eng. Part B-Reviews 28, 1137–1150. doi:10.1089/ten.teb.2021.0159
Vijayavenkataraman, S. (2020). Nerve guide conduits for peripheral nerve injury repair: a review on design, materials and fabrication methods. Acta Biomater. 106, 54–69. doi:10.1016/j.actbio.2020.02.003
Wang, J., Cheng, Y., Chen, L., Zhu, T., Ye, K., Jia, C., et al. (2019). In vitro and in vivo studies of electroactive reduced graphene oxide-modified nanofiber scaffolds for peripheral nerve regeneration. Acta Biomater. 84, 98–113. doi:10.1016/j.actbio.2018.11.032
Wang, J., Wang, H., Mo, X., and Wang, H. (2020). Reduced graphene oxide-encapsulated microfiber patterns enable controllable formation of neuronal-like networks. Adv. Mater. 32, e2004555. doi:10.1002/adma.202004555
Wang, Q., Wang, H., Ma, Y., Cao, X., and Gao, H. (2022). Effects of electroactive materials on nerve cell behaviors and applications in peripheral nerve repair. Biomaterials Sci. 10, 6061–6076. doi:10.1039/d2bm01216b
Wang, W., Liu, W., Zhu, H., Li, F., Wo, Y., Shi, W., et al. (2011). Electrical stimulation promotes BDNF expression in spinal cord neurons through Ca2+- and erk-dependent signaling pathways. Cell. Mol. Neurobiol. 31, 459–467. doi:10.1007/s10571-010-9639-0
Wieringa, P. A., de Pinho, A. R. G., Micera, S., van Wezel, R. J. A., and Moroni, L. (2018). Biomimetic architectures for peripheral nerve repair: a review of biofabrication strategies. Adv. Healthc. Mater. 7, e1701164. doi:10.1002/adhm.201701164
Yan, X., Liu, J., Ye, Z., Huang, J., He, F., Xiao, W., et al. (2016). CaMKII-Mediated CREB phosphorylation is involved in Ca2+-induced BDNF mRNA transcription and neurite outgrowth promoted by electrical stimulation. Plos One 11, e0162784. doi:10.1371/journal.pone.0162784
Yang, J.-W., Chen, C.-Y., Yu, Z.-Y., Chung, J. H. Y., Liu, X., Wu, C.-Y., et al. (2022). An electroactive hybrid biointerface for enhancing neuronal differentiation and axonal outgrowth on bio-subretinal chip. Mater. Today Bio 14, 100253. doi:10.1016/j.mtbio.2022.100253
Yin, P. T., Shah, S., Chhowalla, M., and Lee, K.-B. (2015). Design, synthesis, and characterization of graphene-nanoparticle hybrid materials for bioapplications. Chem. Rev. 115, 2483–2531. doi:10.1021/cr500537t
Zarrintaj, P., Zangene, E., Manouchehri, S., Amirabad, L. M., Baheiraei, N., Hadjighasem, M. R., et al. (2020). Conductive biomaterials as nerve conduits: recent advances and future challenges. Appl. Mater. Today 20, 100784. doi:10.1016/j.apmt.2020.100784
Zhang, F., Yang, K., Liu, G., Chen, Y., Wang, M., Li, S., et al. (2022a). Recent advances on graphene: synthesis, properties and applications. Compos. Part a-Applied Sci. Manuf. 160, 107051. doi:10.1016/j.compositesa.2022.107051
Zhang, S., Huang, M., Zhi, J., Wu, S., Wang, Y., and Pei, F. (2022b). Research hotspots and trends of peripheral nerve injuries based on web of science from 2017 to 2021: a bibliometric analysis. Front. Neurology 13, 872261. doi:10.3389/fneur.2022.872261
Zheng, F., Li, R., He, Q., Koral, K., Tao, J., Fan, L., et al. (2020). The electrostimulation and scar inhibition effect of chitosan/oxidized hydroxyethyl cellulose/reduced graphene oxide/asiaticoside liposome based hydrogel on peripheral nerve regeneration in vitro. Mater. Sci. Eng. C-Materials Biol. Appl. 109, 110560. doi:10.1016/j.msec.2019.110560
Zheng, Z., Huang, L., Yan, L., Yuan, F., Wang, L., Wang, K., et al. (2019). Polyaniline functionalized graphene nanoelectrodes for the regeneration of PC12 cells via electrical stimulation. Int. J. Mol. Sci. 20, 2013. doi:10.3390/ijms20082013
Keywords: peripheral nerve injury, electrical stimulation, graphene-based scaffolds, neural tissue engineering, nerve regeneration
Citation: Zhao Y, Liu Y, Kang S, Sun D, Liu Y, Wang X and Lu L (2024) Peripheral nerve injury repair by electrical stimulation combined with graphene-based scaffolds. Front. Bioeng. Biotechnol. 12:1345163. doi: 10.3389/fbioe.2024.1345163
Received: 27 November 2023; Accepted: 14 February 2024;
Published: 28 February 2024.
Edited by:
Víctor Carriel, University of Granada, SpainReviewed by:
Óscar Darío García García, University of Granada, SpainCopyright © 2024 Zhao, Liu, Kang, Sun, Liu, Wang and Lu. This is an open-access article distributed under the terms of the Creative Commons Attribution License (CC BY). The use, distribution or reproduction in other forums is permitted, provided the original author(s) and the copyright owner(s) are credited and that the original publication in this journal is cited, in accordance with accepted academic practice. No use, distribution or reproduction is permitted which does not comply with these terms.
*Correspondence: Xin Wang, d2FuZ194aW5Aamx1LmVkdS5jbg==; Laijin Lu, bGpsdUBqbHUuZWR1LmNu
Disclaimer: All claims expressed in this article are solely those of the authors and do not necessarily represent those of their affiliated organizations, or those of the publisher, the editors and the reviewers. Any product that may be evaluated in this article or claim that may be made by its manufacturer is not guaranteed or endorsed by the publisher.
Research integrity at Frontiers
Learn more about the work of our research integrity team to safeguard the quality of each article we publish.