- Department of Orthodontics, School of Stomatology, Capital Medical University, Beijing, China
Piezo1 (2010) was identified as a mechanically activated cation channel capable of sensing various physical forces, such as tension, osmotic pressure, and shear force. Piezo1 mediates mechanosensory transduction in different organs and tissues, including its role in maintaining bone homeostasis. This review aimed to summarize the function and possible mechanism of Piezo1 in the mechanical receptor cells in bone tissue. We found that it is a potential therapeutic target for the treatment of bone diseases.
1 Introduction
Mechanotransduction, a fundamental process conserved throughout evolution, refers to the ability to sense mechanical force and convert it into biochemical signals, ultimately achieving complex physiological functions, such as blood pressure regulation and lung relaxation. The discovery of Piezo channels in 2010 improved the understanding of the molecular and cellular mechanisms of mechanotransduction (Coste et al., 2010). The Piezo family, comprising Piezo1 and Piezo2, has the unique ability to rapidly convert diverse mechanical inputs, including tension, osmotic pressure, and shear stress, into electrical impulses.
Piezo1 is a mechanosensitive ion channel that plays a crucial role in bone remodeling, a process that involves the removal of old or damaged bone by osteoclasts and subsequent replacement with new bone formed by osteoblasts. Piezo1 is found in tissues throughout the body, including bone, and is involved in sensing changes in mechanical stress (Sun et al., 2019). Notably, Piezo1 is closely related to the development of osteoporosis (OP) (Xu et al., 2021). Furthermore, Piezo1 is expressed in both condylar cartilage and subchondral bone (Wu et al., 2022). The inhibitor GsMTx4 has recently attracted much attention as a promising treatment for cartilage injury (Li et al., 2016). GsMTx4 can weaken Piezo-mediated mechanically activated (MA) currents and reduce chondrocyte death induced by mechanical force (Lee et al., 2014).
Piezo1 is expressed and functions in various mechanical sensor cells, including osteoblasts (Sun et al., 2019; Song et al., 2020), osteoclasts (Wang et al., 2020), osteocytes (Liu Z. et al., 2022), bone marrow mesenchymal stem/stromal cells (BMSCs) (Zhou et al., 2020), chondrocytes (Hendrickx et al., 2021), periodontal ligament fibroblasts (PDLFs) (Kang et al., 2014), and periodontal ligament stem cells (PDLSCs) (Lin Y. Y. et al., 2022). These cells participate in bone formation and resorption, ultimately maintaining bone homeostasis. This study presents an overview of the structure and properties of Piezo channels, mainly focusing on recent advancements in understanding the role of Piezo1 in bone remodeling. Additionally, we explored potential signaling pathways associated with Piezo1.
2 Piezo1
2.1 Discovery of the Piezo family
Previous studies have demonstrated that MA cation channels, considered a specialized subset of mechanotransducers, are ubiquitously expressed in various cell types and can be triggered by various mechanical forces. These channels can promptly initiate cellular responses after activation. Although TRP ion channels and DEG/ENaC channels significantly promote invertebrate mechanotransduction, the mechanisms underlying mechanotransduction in mammals are unclear (Ranade et al., 2015). Therefore, identifying MA cation channels in mammals is crucial for enhancing the understanding of the mechanotransduction mechanism.
In 2010, Patapoutian and colleagues made a groundbreaking discovery: the Piezo ion channel family, comprising Piezo1 and Piezo2 (Coste et al., 2010). In that study, a significant increase in mechanosensitive currents was detected in a specific mouse neuroblastoma cell line known as Neuro2A cells. They found that the Piezo1 gene, also known as Fam38A, is essential for generating these MA currents based on RNA interference techniques. Furthermore, they found that Fam38B can encode the Piezo2 protein through homologous sequence analysis.
2.2 Structure of Piezo1
Ge et al. (2015) unveiled the high-resolution three-dimensional configuration of mouse Piezo1 using cryo-electron microscopy (cryo-EM), which resembles a three-bladed propeller (Figure 1). Piezo proteins consist of a central cap and three distal blades on the extracellular side and three elongated beams (length; about 90 nm) on the intracellular side. The transmembrane (TM) region is situated between the domains exposed on the extracellular and intracellular surfaces. The beams connect peripheral TMs and blades, linking them to the lower central axis of the channel complex. The TM region exhibits significant curvature and clockwise twist, akin to the wing-shaped blade found in propellers, comprising 9 TM helical units (THUs) (Ge et al., 2015; Zhao et al., 2016; Zhao et al., 2018).
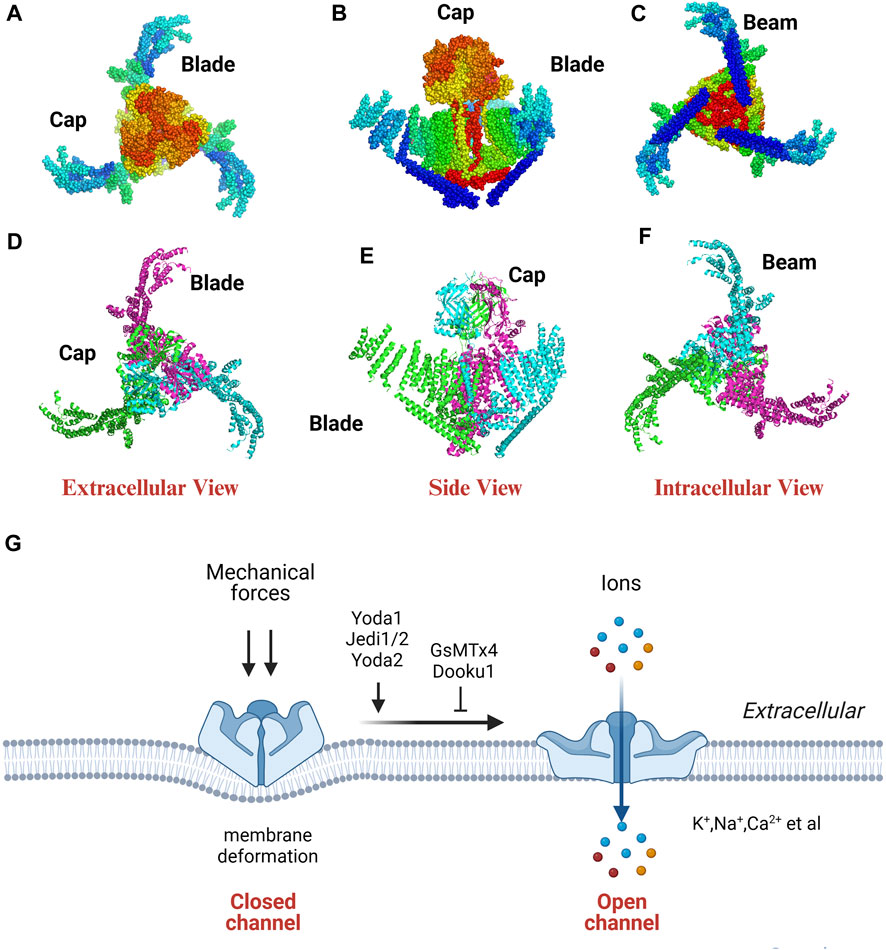
FIGURE 1. The cryo-EM structure (A–F) and property (G) of Piezo1. The extracellular, side, intracellular view of the Piezo1 channel indicated by dots (A–C) and cartoon model (D–F). PDB ID: 3jac; cited from Ge et al. (2015).
Piezo channels are dissected into two distinct functional modules (a central ion-conducting pore and a mechanotransduction module) to elucidate the correlation between the structure and function of the Piezo1 channel. The ion-conducting pore module has three primary components: the C-terminal extracellular domains (CEDs), the TM inner helices (IHs) and outer helices (OHs), and the intracellular C-terminal domains (CTDs). This module regulates ion selectivity, unitary conductance, and pore obstruction. The mechanotransduction module comprises the extracellular distal blades, the peripheral helices (PHs), the TM anchors, and the intracellular beams (Wang and Xiao, 2018). This module plays a role in the detection have a record-breaking 114 TMs based on a novel 38-TM topology (Xiao, 2020). The three large TM blades act as mechanosensors, detecting alterations in membrane tension and influencing the conformational arrangement of the channel.
Zhao et al. (2018) introduced a mechanogating mechanism that resembles a lever, categorizing the intricate and efficient process of long-distance mechanotransduction. Residues L1342 and L1345 function as pivotal points of the lever, positioned at a greater distance from the TM blade at the distal end while being closer to the central pore module of Piezo1 at the opposite end. Based on the lever principle, applying a lighter force to the TM blade with a longer force arm amplifies output force, facilitating gating of the central ion-conducting pore and selective cation penetration (Wang Y. et al., 2018; Zhao et al., 2019; Xiao, 2020). The assembled propeller-shaped machine effectively converts significant conformational alterations of the distal blades into a subtle movement of the core pore structure, transforming mechanical stimulation into ionic influx.
However, further studies should assess the mechanisms by which mechanical force modulates the activity of Piezo channels. The structure-based membrane dome mechanism suggested that Piezo protein deforms the membrane locally into a dome shape when it is in a closed state. However, this dome undergoes a relative flattening upon the application of a force. The transition of Piezo channels from a closed to an open state enhances its ability to respond to mechanical stimuli (Guo and MacKinnon, 2017). Geng et al. (2020) introduced a “plug-and-latch” mechanism in which Piezo1 outfitted three lateral ion-conducting portals with three independently positioned lateral plug gates which were strategically secured to the central axis to achieve synchronized gating of the three portals. The coordinated action of plugs and latches in the Piezo1 channel is impacted by mechanical forces acting asymmetrically on the force-sensing blades in this mechanism. Wang J. et al. (2022) found that the Piezo channels have a biochemical and functional connection to the actin cytoskeleton through the cadherin-β-catenin mechanotransduction complex. As a result, Piezo channels can effectively detect and respond to long-range mechanical disturbances within a cell.
Yang et al. (2022) elucidated the structural characteristics of Piezo1 in both curved and flattened conformations within liposome vesicles using cryo-EM. They found that Piezo1 protein has a curved conformation that can flatten. Furthermore, the protein beam can bend under mechanical stimulation while the protein cap can detach and rotate in response to mechanical stimulation. These alterations can facilitate the opening of the ion-conducting pathway, thus regulating the channel. The remarkable mechanosensitivity and specific curvature-based gating observed in lipid membranes could be due to the deformability and structural rearrangement of Piezo1. Mulhall et al. (2023) directly visualized and quantified the conformational dynamics of individual Piezo1 molecules within a cellular context using nanoscopic fluorescence imaging techniques. They found that Piezo1 blades can significantly expand while in a resting state due to the bending tension applied by the plasma membrane. Besides, the blades exhibited varying degrees of rigidity along the length. Stiffness increased at the base while the flexibility increased towards the ends. The researchers also investigated the correlation between blade growth and the activation and inhibition of channels. They found that the inhibitor of Piezo1, or the removal of the plasma membrane, can increase compaction levels in the blades. Moreover, Piezo1 activation, either through osmotic or chemical stimulation slightly expanded the blades (1–2 nm increase). These findings reveal that the conformation of the Piezo1 is more intricate than initially anticipated based solely on modeling.
2.3 Piezo1 regulation
Piezo1 protein can sense various forces, such as tension, poke force, osmotic pressure, and fluid shear force and convert mechanical stimuli into electrical signals in milliseconds (Retailleau et al., 2015). Piezo1 is a non-selective cation channel permeable to K+, Na+, Ca2+, and Mg2+, with a slight preference for Ca2+ (Coste et al., 2010) (Figure 1).
Piezo1 channels can perceive various mechanical stimuli in distinct manners. Atomic force microscopy (AFM) experiments have revealed that Piezo1 channels have distinct responses to pushing and pulling forces (Gaub and Müller, 2017). Specifically, pulling forces can more efficiently activate Piezo1 channels in the presence of extracellular matrix (ECM) proteins. Ozkan et al. (2023) monitored the local rearrangements occurring along the blades of Piezo1 under varying forces by inserting two cyclic permuted green fluorescent proteins as a probe. Significant fluorescence signals were observed from the probes upon Piezo1 activation by low-intensity fluid shear stress (FSS). However, no visible fluorescence signals were produced by the Piezo1 channel activation in response to cellular indentations, osmotic swelling, and high-intensity flow stimuli.
Piezo1 channels can be strongly regulated by voltage in addition to mechanical stimuli, and can even transition to a solely voltage-gated mode (Moroni et al., 2018). Moreover, stomatin-like protein 3 (STOML3) can significantly regulate Piezo channels by reducing the activation threshold of Piezo1 and Piezo2 currents (Poole et al., 2014).
Yoda1, Jedi1/2 and Yoda2 can activate Piezo1 channel. Jedi1/2, as a synthetic agonist, can activate Piezo1 by binding to the upstream blade (Wang Y. et al., 2018). Yoda1, as a synthetic small molecule, activates Piezo1 by acting as a molecular wedge, inserting itself between 2 TM regions (Syeda et al., 2015; Botello-Smith et al., 2019; Jiang et al., 2023). Yoda2, 4-Benzoic acid modification of Yoda1, can more effectively activate Piezo1 channel with better solubility and stability (Parsonage et al., 2023).
Ruthenium red, gadolinium, streptomycin, and GsMTx4 (grammostola spatulata mechanotoxin 4) can inhibit Piezo channels. Ruthenium red, gadolinium, and streptomycin are nonspecific inhibitors of Piezo1, blocking multiple cationic channels. GsMTx4, a peptide derived from spider venom, can selectively block Piezo and TRP channel families by modulating lipid bilayer fluidity within the membrane (Bae et al., 2011). Dooku1, a Yoda1 analogue, acts at the same location as Yoda1 to efficiently inhibit Yoda1-induced Piezo1 channel activation. However, Dooku1 cannot inhibit constitutive Piezo1 channel activity (Evans et al., 2018). Similarly, Tubeimoside I (TBMS1), a compound obtained from Traditional Chinese Medicine, can inhibit Yoda1-induced activation of Piezo1 channels (Liu et al., 2020).
2.4 The function of Piezo1
Piezo proteins, comprising approximately 2,500–2,800 amino acids, exhibit remarkable evolutionary conservation and lack substantial sequence homology with known ion channels (Coste et al., 2010). Notably, Piezos can detect various mechanical stimuli and produce rapid cationic currents in different mammalian cell lines (Table 1). Piezo1 is abundantly present in various organs, such as lung, bladder, intestines, and skeleton tissue. Besides, Piezo1 participates in cardiovascular mechanical transduction, immune regulation, epithelial cell homeostasis, red blood cell volume regulation, and bone formation (Coste et al., 2010) (Figure 2). Piezo2 is significantly expressed in sensory tissues, including dorsal root, trigeminal ganglia sensory neurons, and Merkel cells, where they primarily respond to touch and proprioception (Geng et al., 2016; Gottlieb, 2017).
Piezo1 mutations are linked to certain hereditary human diseases, including dehydrated hereditary stomatocytosis (DHS) (Andolfo et al., 2013) and generalised lymphatic dysplasia (GLD) (Lukacs et al., 2015). GLD is caused by loss-of-function mutations in the Piezo1 gene (Martin-Almedina et al., 2018), characterized by various symptoms, including non-immune hydrops fetalis (NIHF), lymphedema, and recurrent cellulitis (Chen Y. et al., 2021). Loss-of-function mutations in the Piezo1 gene, especially S217L and G2029R, have been shown to alter protein stability due to increased ubiquitination and subsequent proteasomal degradation (Zhou et al., 2021). Furthermore, Piezo1 loss-of-function compound heterozygous mutations have been reported in patients with Prune Belly Syndrome, which is characterized by a “Prune-like” wrinkled, flaccid ventral abdominal wall with regionally missing or hypoplastic skeletal muscle (Amado et al., 2024).
Meanwhile, DHS is associated with gain-of-function mutations in the Piezo1 gene (Fotiou et al., 2015). A recent case report showed that the c.7505A>G variant can cause a DHS phenotype. This variant is cataloged in the single nucleotide polymorphism database (dbSNP) with the reference ID rs34830861 (Martin-Almedina et al., 2018). Another gain-of-function mutation in Piezo1 gene, known as E756del has been linked to enhanced athletic performance and the ability of protection against severe malaria. When performing leaping actions requiring high tendon loading, energy storage, and return, carriers of the E756del mutation outperform non-carriers by a large margin (Passini et al., 2021).
Recent case reports have highlighted skeletal manifestations associated with Piezo1 mutations, demonstrating the diverse impact of this gene on human health. Lee et al. (2021) reported a case of a 21-year-old male diagnosed with primary lymphatic dysplasia who exhibited additional clinical features, such as a history of multiple fractures in infancy, thoracolumbar scoliosis, low height, and hypoplasia of the left-sided facial bones. Exome analysis revealed that the patient had two previously unreported pathogenic variants of Piezo1 in a trans configuration, including a heterozygous deletion spanning 93.7 kb (chr16:88,782,477-88,876,207; exon 1-50) and a single nucleotide substitution c.2858G>A (p.Arg953His). This case of compound heterozygosity, where the variants were inherited from different parents, underscores the potential impact of Piezo1 mutations on bone health. Another Piezo1 gene mutation was identified in the 61-year-old male patient with non-transfusion secondary hemochromatosis, specifically the missense mutation c.C4748T (p.A1583V). Osteoarticular involvement was indicated by thinning bone cortices and an enlarged tibial medullary cavity by computed tomography. However, it’s important to note that the search results do not provide direct evidence that the patient’s osteoarticular phenotype is caused by this mutation, or iron deposition due to the iron overload condition. To fully comprehend the possible involvement of the Piezo1 gene in bone health and illness, more investigation is required (Ruan et al., 2020).
3 Piezo1 in bone cells
Piezo1 regulates skeleton homeostasis in osteoblast lineage cells (Figure 3). The elimination of Piezo1 in mice results in fatal outcomes, due to disruption of vascular development (Ranade et al., 2015). Conditional Piezo1 deletion by various Cre strains in Osteoblast lineage cells showed reduced trabecular and cortical bone mass (Nie and Chung, 2022; Dienes et al., 2023) (Table 2).
3.1 Piezo1 in BMSCs
The BMSCs can differentiate into osteogenic, adipogenic, and chondrogenic lineages under different loading conditions (Pierce et al., 2019). Osteoblast differentiation from BMSCs occurs through various intersecting signaling pathways, including Wnt pathways, bone morphogenetic protein (BMP) pathway (James, 2013), and specific transcription factors, including runt-related transcription factor 2 (Runx2) (Zhang et al., 2010; Sun et al., 2021a).
Piezo1, in particular, has been identified as a critical mechanotransducer in various biological processes, including bone formation. It is expressed in differentiating osteoblasts and hypertrophic chondrocytes in developing skeletal structures, and its expression increases during postnatal development following elevated mechanical stress (Zhou et al., 2020). The Piezo1 agonist Yoda1 promoted bone formation and osteoblast differentiation in developing mouse limb buds even under static conditions. In contrast, Piezo2 appears to have a more redundant and dispensable function during bone formation. The deletion of Piezo2 in osteoblasts had little adverse effect on skeletal development (Zhou et al., 2020).
The absence of Piezo1 in the mesenchyme of developing limbs achieved by the utilization of Prx1-Cre result in many skeletal abnormalities in mice, including shortened long bones, diminished quantities of trabecular and cortical bone, and increased risk of spontaneous bone fractures in both newborn and early adult mice (Wang et al., 2020; Zhou et al., 2020). The observed effects are associated with heightened osteoclast function and diminished osteoblast function, as evidenced by a decrease in procollagen type I N-terminal propeptide (P1NP) and Osterix levels. Interestingly, the low bone mass phenotype in Piezo1 Prx1-Cre mice appears to be limited to long, load-bearing bones. The calvariae are not affected by Piezo1 deletion, likely due to their lower load-bearing capacity compared to long bones. To elucidate the role of mechanical stimulus in bone formation, a mechanical unloading model through tail suspension was established, revealing a reduction in bone mass in the control group, but no such effect was observed in Piezo1 Prx1-Cre mice (Wang et al., 2020).
Piezo1 plays a crucial role in BMSCs differentiation under mechanical stimulation. Sugimoto et al. (2017) discovered that hydrostatic pressure (HP) enhances the expression of Piezo1 and BMP2 in human BMSCs, thus promoting osteoblast differentiation and inhibiting adipocyte differentiation. Yoda1 treatment can significantly alleviate bone loss caused by microgravity and aging and also promote the proliferation and osteogenic differentiation of BMSCs (Hu et al., 2023). The researchers designed a wearable pulsed triboelectric nanogenerator powered by human body motion, to activate Piezo1 channel and upregulate osteogenic differentiation potential of aging BMSCs. This finding provides a possible target for bone regeneration, especially for aged people (Wang B. et al., 2022). Moreover, static magnetic field (SMF) can enhance BMSC migratory capacity through Piezo1 (Sun et al., 2023). Piezo1 also regulates the differentiation ability of BMSCs into chondrocytes. Notably, exosomes produced by siRNA-Piezo1-treated BMSCs promote BMSC development into cartilage, thus enhancing the restoration of injured cartilage in osteoarthritis (OA) (Li et al., 2021).
3.2 Piezo1 in osteoblasts
Osteoblasts are primarily found in mesenchymal stem cells (MSCs) located within and outside the periosteum and within the bone marrow matrix (Abdallah et al., 2015).
Runx2 regulates the commitment of MSCs to the osteoblastic lineage during bone development. Mice lacking Piezo1 in Runx2-expressing cells (Piezo1 Runx2-Cre) exhibited several bone abnormalities including multiple spontaneous fractures, shorter femurs, pelvic dysplasia and a considerable decrease in trabecular bone mass below the growth plates. Similarly, Piezo1 Runx2-Cre mice exhibit no calvarial bone defects at birth nor changes in calvarial thickness (Hendrickx et al., 2021). Osterix (also known as Sp7) is another critical transcription factor expressed in osteoblast progenitors and osteoblasts. Reduced trabecular and cortical bone mass has been reported in Piezo1 SP7-Cre mice, which is markedly lower than Piezo1 Prx1-Cre mice (Zhou et al., 2020). Collagen type 1 (Col1) is an important structural protein of the ECM in bone and is expressed throughout the differentiation stages from preosteoblasts to mature osteoblasts. Piezo1 Col1-CreERT mice is characterized by increased bone resorption and decreased collagen expression, which leading to decreased bone density and changes in trabecular bone structure (Wang et al., 2020). Osteocalcin (OCN) is highly expressed in mature osteoblasts and plays a role in the regulation of bone mineralization and calcium ion homeostasis. Incomplete closure of cranial sutures was observed in Piezo1 OCN-Cre mice, accompanied with shorter weight-bearing long bones and significant bone mass loss (Sun et al., 2019).
Some studies have shown that Piezo1 regulates osteoblast differentiation under different forces, including HP loading (Sugimoto et al., 2017), static magnetic force (Hao et al., 2019), low-intensity pulsed ultrasound (LIPUS) (Zhang et al., 2021) and FSS (Song et al., 2020). Sun et al. (2019) found that mechanical-loading treatment can increase Piezo1 levels and osteoblasts function, while hind-limb suspension or simulated microgravity treatment can inhibit Piezo1 expression, impairing bone integrity and strength in mice. These results demonstrate that Piezo1 effect is correlated with mechanical force in osteoblasts.
3.3 Piezo1 in osteocytes
Osteocytes, mainly found in osteoblasts, are the predominant cellular inhabitants in bone tissue. The expression of Piezo1 is significantly higher in osteocytes than Piezo2 (Li et al., 2019). Fluid-flow stimulation on mature osteocytes can activate and upregulate Piezo1 channels (Li et al., 2019; Liu Z. et al., 2022). Yoda1 can enhance intracellular calcium mobilization and inhibit sclerostin (Sost) expression in osteocytes in a dose-dependent manner, thus promoting osteoblast differentiation (Sasaki et al., 2020). Whereas Piezo1 inactivation increases the expression of receptor activator of NF-κB ligand (RANKL) and osteoclasts number and decreases the expression of osteoprotegerin(OPG), thus promoting osteoclastogenesis (Li et al., 2019; Li et al., 2023). Furthermore, Piezo1 knockdown in osteocytes can decrease the bone formation-related genes (Alkaline Phosphatase, ALP) of osteoblasts induced by ultrasound stimulation in 3D osteocyte-osteoblast co-culture (Inoue et al., 2023).
Dentin matrix protein 1 (Dmp1) is a non-collagenous protein known to be an indicator of osteocytes. Compared to Piezo1 Runx2-Cre mice, Piezo1 Dmp1-Cre mice displayed a moderate reduction in trabecular and cortical bone mass. No significant spontaneous bone fractures were recorded (Li et al., 2019; Wang et al., 2020; Hendrickx et al., 2021). Similarly, Nottmeier et al. (2023) reported that Piezo1 deletion in osteocytes and osteoblasts suppresses the bone volume of the mandible and maxilla, as well as the height of the vertical alveolar bone. However, the morphology and length of the mandible and skull are unaffected by Piezo1 deletion.
3.4 Piezo1 in osteoclasts
Multinucleated osteoclasts are mainly found in myeloid hematopoietic precursors in the bone marrow (Boyle et al., 2003; de Vries et al., 2009). The mice with targeted deletion of Piezo1 in osteoclast lineage cells (Lyz2-Cre) (Hendrickx et al., 2021) and Ctsk-Cre (Wang et al., 2020) exhibited normal bone mass. Wang et al. (2020) also found that osteoblastic Piezo1 deficiency significantly decreases the levels of matrix proteins Col2α1 (alpha-1 type II collagen) and Col9α2 (alpha-2 type IX collagen) but markedly increases the number and activity of osteoclasts in the co-culture system of osteoclasts and Piezo-deficient osteoblasts. These findings suggest that Piezo1 can regulate osteoclast activation via type II and IX collagens, thus indirectly affecting bone resorption.
3.5 Piezo1 in PDLSCs and PDLFs
PDLSCs were initially isolated from human-impacted third molars (Seo et al., 2004). PDLSCs exhibit strong proliferation capability and high multilineage differentiation potential (Wang et al., 2011; Zhu and Liang, 2015). Mechanical force regulates the differentiation ability of PDLSCs and PDLFs and modifies associated genes (Panchamanon et al., 2019; Jin et al., 2020).
The Leptin receptor (Lepr) serves as a distinguishing factor for a distinct multipotent population of PDLSCs. Deletion of Piezo1 in Lepr + cells leads to a decrease in cellular cementum formation and alveolar bone mass, a lower ECM mass of cementum, and disorganized collagen fibrils. In contrast, femur bone mineral density are not affected. Hence, Piezo1 plays a crucial role in maintaining the equilibrium of the periodontium (Zhang et al., 2023). These findings underscore the role of Piezo1 in maintaining periodontal homeostasis.
Shen et al. (2020) showed that tension force activates and upregulates Piezo1. Besides, Piezo1 participates in periodontal ligament cells (PDLCs) mechanotransduction via the ERK signaling pathway. Piezo1 can also regulate osteoclastogenesis when the compression force is applied to PDLFs (Schröder et al., 2023). The release of adenosine triphosphate (ATP) and the activation of inflammatory genes during this process is also regulated by Piezo1 (Horie et al., 2023; Schröder et al., 2023). GsMTx4 treatment can significantly inhibit NF-kB activation and osteoclast-related factors induced by compression force, indicating that Piezo1 can regulate osteoclast differentiation via the NF-κB signaling pathway (Jin et al., 2015). Shen et al. (2023) also showed that Piezo1 inhibition can mitigate PDLFs apoptosis and damage under compression force by modulating the p38/ERK1/2 signaling pathway. In summary, Piezo1 participates in many processes that maintain the health and function of the periodontal ligament, including ATP release, osteoclastogenesis and osteogenesis.
4 Piezo1 signaling in bone remodeling
Piezo1 mediates MA cationic currents and induces Ca2+ influx (Sun et al., 2019). This influx initiates downstream Ca2+ signaling, including the activation of the nuclear factor of activated T-cells (NFAT) (Zhou et al., 2020) and Ca2+-calmodulin (CaM)-dependent protein kinase (CaMKII) (Chen et al., 2022). The Piezo-Ca2+ signaling cascade plays a crucial in bone growth and significantly enhances the understanding of fundamental molecular and biological functions in the skeletal system. The signaling pathway of Piezo1 in bone tissue is summarized in Figure 4.
4.1 Piezo1 and NFAT
Studies have shown that the Ca2+/CaN/NFAT signaling pathway regulates bone formation and bone resorption (Tomita et al., 2002; Koga et al., 2005). Elevated Ca2+ concentrations activate calcineurin (CaN), leading to NFAT dephosphorylation and subsequent nuclear translocation (Ren et al., 2021).
CaN/NFAT1 signaling axis participated in Piezo1-mediated chondrocyte apoptosis, cartilage matrix production (Ren et al., 2023), fibrochondrogenesis (Yan et al., 2023) and vascular niche regeneration (Zhang et al., 2022).
Ppp3ca, also known as CaN, is a calcium and CaM -dependent serine/threonine protein phosphatase. Notably, Zhou et al. (2020) demonstrated that Piezo1 activation leads to NFAT, YAP, and β-catenin activation in BMSCs, and then regulates gene expression during osteoblast differentiation and bone formation, which can all be prevented by knocking down Piezo1. This implies a functional relationship between these proteins in bone formation and homeostasis.
4.2 Piezo1 and CAMKII
When intracellular calcium levels rise, calcium binds to CaM, which in turn binds to CaMKII, inducing its activation. This activation leads to autophosphorylation of CaMKII, and alters its conformation, allowing it to translocate and bind to different proteins within the cell (Rostas and Skelding, 2023). The phosphorylated CaMKII can then phosphorylate CREB, which is a transcription factor that regulates the expression of genes involved in numerous cellular processes (Yan et al., 2016).
Piezo1 modulates different biological processes through CAMKII, including blood pressure regulation (Zheng et al., 2022), immune response (Geng et al., 2021), perfusion recovery after ischemia (Xie et al., 2023), chronic inflammation (Liu H. L. et al., 2022) axon regeneration (Song et al., 2019) and cardiomyocyte hypertrophy (Yu et al., 2022).
In addition, CaMKII signaling is essential for Piezo1-mediated new bone formation in ankylosing spondylitis (Chen et al., 2022). Piezo1 also regulates osteoblast differentiation via CAMKII. CaMKII and Creb phosphorylation are downregulated in osteoblasts derived from Piezo1 OCN-Cre mice with lower osteoblast activity (Sun et al., 2019).
4.3 Piezo1 and YAP
Yes-associated protein (YAP) and its paralogue transcriptional coactivator with PDZ-binding motif (TAZ) are two highly related transcriptional cofactors in Hippo signaling (Dupont et al., 2011). The activity of YAP/TAZ is regulated by a complex interplay of mechanical and biochemical signals, including the tensional state of the F-actin cytoskeleton, cell-cell and cell-ECM adhesions, and interactions with other signaling pathways (Totaro et al., 2018). This YAP/TAZ axis can promote osteoblast differentiation by activating the downstream target Runx2 (Tang et al., 2016).
The increase in intracellular calcium levels can lead to the dephosphorylation and nuclear translocation of YAP, transforming it into a transcriptional co-activator (Sayedyahossein et al., 2023). Once in the nucleus, YAP collaborates with β-catenin, forming a YAP/β-catenin complex that directly interacts and upregulates osteogenic, chondrogenic, and angiogenic factors crucial for bone repair and regeneration (Liu Y. et al., 2022). In osteoblastic cells, Piezo1 controls the YAP-dependent expression of type II and IX collagens in response to mechanical loads, influencing osteoclast development in bone remodeling (Wang et al., 2020). In addition, YAP has been found to be decreased in degenerated cartilage (Sun et al., 2021b). The activation of Piezo1 via the YAP facilitates mechanically induced cartilage degradation (Feng et al., 2023).
However, some studies demonstrates that YAP could regulate Piezo1 expression in turn. Kong et al. (2022) found the nuclear localization of YAP could activated Piezo1 and enhance osteogenesis. Additionally, YAP, in collaboration with the G protein-coupled estrogen receptor (GPER) pathway, suppresses Piezo1 activation, thereby mitigating chondrocyte apoptosis (Sun et al., 2021b).
4.4 Piezo1 and β-catenin
Zhou et al. (2022) identified several pathways, including the Wnt/β-catenin and PI3K-Akt pathways to be potential targets of Piezo1 through bioinformatic analysis.
Wnt/β-catenin pathway promotes osteoblast development and proliferation (Gong et al., 2001; Hu et al., 2005). It is known to interact with the Hippo signaling pathway, specifically with the transcription factors YAP/TAZ, which are key components of the β-catenin degradation complex in the canonical Wnt signaling pathway. Upon Wnt/Frizzled binding, the destruction complex releases β-catenin and YAP/TAZ into the cytoplasm, triggering their subsequent translocation into the nucleus (Imajo et al., 2015; Totaro et al., 2018).
YAP and Piezo1 could serve as the downstream factor of Wnt5a, which work together to encourage the 3D cell intercalations that form the mandibular arch in mice (Tao et al., 2019). Another study suggested that Piezo1 promotes Wnt1 expression partly by activating YAP1 and TAZ, and then regulates bone development and homeostasis (Li et al., 2019).
Moreover, Piezo1 regulates the stemness of BMSCs through β-catenin. Blocking Wnt/β-catenin pathway via IWR-1 treatment inhibited the Yoda1-induced osteogenic differentiation of BMSCs (Hu et al., 2023). The involvement of Piezo1 in the proliferation and osteogenic differentiation of human dental follicle cells has been shown to be mediated by the Wnt/β-catenin signaling pathway (Xing et al., 2022).
4.5 Piezo1 and AKT
AKT plays a crucial role in cell survival, proliferation, growth, and metabolism (Manning and Toker, 2017). Notably, Sasaki et al. (2020) indicated that Piezo1 activation leads to Akt phosphorylation, subsequently down-regulating Sost. This process promotes bone formation, suggesting that Piezo1 participates in osteocyte mechanotransduction by triggering downstream Piezo1-Akt signaling. The canonical Wnt/β-catenin pathway involves the Ser and Thr protein kinase glycogen synthase kinase 3 (GSK3), initially recognized as an AKT substrate (Cross et al., 1995). Phosphor-GSK-3 prevents β-catenin degradation and facilitates nuclear translocation of accumulated β-catenin, regulating downstream target genes that control bone homeostasis, including Runx2 (Cai et al., 2016). FSS induces the expression of Runx-2 in MC3T3-E1 cells via the upregulation of Piezo1. This process involves the activation of the AKT/GSK-3β/β-catenin pathway to regulate bone formation under mechanical strain (Song et al., 2020).
Phosphorylated PI3K-AKT is related to Piezo1-mediated osteoblast maturation and ossification (Chen P. et al., 2021). Moreover, Piezo1-induced increase in intracellular calcium influx activates connexin 43 hemichannels (Cx43 HCs) in osteocytes through PI3K-Akt signaling under mechanical stress, thus regulating bone anabolic function (Zeng et al., 2022). Artemisinin (ART), a highly efficacious antimalarial drug, was found to exert therapeutic effects on osteoarthritis (OA) by acting on Piezo1 and AKT proteins. ART can downregulate Yoda1-induced upregulation of OA-related genes, and inhibit PI3K and AKT phosphorylation in chondrocytes (Gan et al., 2023).
4.6 Piezo1 and MAPK
Studies have reported that the MAPK signaling pathway participates in the regulation of osteogenic differentiation (Lu and Malemud, 2019). The C-terminal region of Piezo1, containing the domain that interacts with R-Ras, modulates the influx of calcium and activation of the ERK1/2 signaling pathway. This process regulates the osteoblastic development in BMSCs (Sugimoto et al., 2023). Piezo1 can also inhibit apoptosis of the human chondrocyte via the classic MAPK/ERK1/2 signal pathway (Li et al., 2016). Activation of the Piezo1 can induce ATP release and its binding with P2 receptor, and then enhances MSC migration. Researches have shown that process could be blocked by U0126, an inhibitor of the MEK/ERK signaling pathway, suggesting MEK/ERK signaling participates in Piezo1-mediated MSC migration (Mousawi et al., 2020).
It has been demonstrated that activation of Piezo channels in response to ultrasound stimulation can activate the MAPK pathway, particularly ERK1/2, in dental pulp stem cells (Gao et al., 2017). Shen et al. (2020) showed that blocking the Piezo1 channel can significantly increase the phosphorylation of GSK3α/β in PDLCs, indicating that GSK may be involved in PDLCs mechanotransduction. Also, GSK3 is a negative regulator of ERK1/2, c-Fos, and c-Jun (Wang et al., 2006; Götschel et al., 2008) and promotes β-catenin activation via Ras suppression (Liu et al., 2008). Nonetheless, further investigations should explore the potential interplay between the MAPK pathway and the Piezo1 channel in the above process.
5 Piezo1 and clinical therapy
5.1 Piezo1 and OP
OP is characterized by compromised bone strength, which substantially elevates the susceptibility to fractures, especially in the hip, spine, and wrist regions. OP is usually diagnosed after the fracture occurrence, and its etiology encompasses various elements, such as hormone fluctuations, the aging process, genetic predisposition, lifestyle choices, and certain medical disorders (Sheik Ali, 2023).
Polymorphisms in the Piezo gene are associated with human bone mineral density (BMD), a critical biomarker for the diagnosis and treatment of OP. A cross-phenotype meta-analysis for human BMD at various skeletal sites yielded the top 14 SNPs for Piezo1. Notably, the SNP rs62048221 was substantially correlated with BMD, especially around the heel, where mechanical force is applied during physical activities, such as standing. The T allele of this SNP was linked to BMD reduction, indicating that it can modulate the activity of cis-regulatory elements, thus influencing Piezo1 expression levels, which in turn affects BMD (Bai et al., 2020). OP patients have considerably lower levels of Piezo1 mRNA and protein than normal patients (Sun et al., 2019). Zhou et al. (2020) discovered that age is negatively correlated with gene expression of Piezo1 and Piezo 2 from human bone MSCs. Dzamukova et al. (2022) also discovered that mechanical forces, which increase with body weight during late adolescence, can trigger the Piezo1 activation, subsequently upregulating the kinase FAM20C within osteoblasts. Notably, FAM20C significantly regulates skeletal growth and bone mineralization by phosphorylating DMP1. Furthermore, simulated microgravity can decrease osteoblast function by inhibiting Piezo1 expression (Sun et al., 2019). Hu et al. (2023) revealed that the mitigation of bone loss in simulated microgravity conditions can be achieved through Piezo1 activation with Yoda1. Furthermore, Piezo1 activation induces a slight protective effect against bone loss in mice subjected to ovariectomy (OVX) and aging (Hu et al., 2023). Piezoelectric microvibration stimulation (PMVS) can alleviate OP induced by estrogen deficiency through Piezo1, MicroRNA-29a, and Wnt3a signaling pathways in osteoblasts, thus enhancing osteogenic activity and suppressing osteoclastic bone resorption (Wu et al., 2021). Furthermore, Sciancalepore et al. (2022) were the first to indicate that Piezo1 participates in the release of myokines. They also proposed the use of Yoda1 as a novel therapeutic intervention to augment the physiological advantages associated with exercise-induced myokine release. Guan et al. (2023) constructed a nanocarrier (ZOL-PLGA@Yoda1/SPIO) which combines the bone-targeting ability of Zoledronate (ZOL) and the magnetic properties of Superparamagnetic iron oxide (SPIO) to achieve dual-targeted administration and precise Piezo1-activated therapy for osteoporotic bone defects. In vivo and in vitro experiments have revealed that this nanocarrier not only enhances bone formation but also promotes the osteogenesis-angiogenesis coupling via the YAP/β-catenin signaling axis, providing a potentially effective strategy for the clinical treatment of osteoporotic bone defects.
The maintenance of alveolar bone homeostasis relies on occlusal force. The absence or reduction of occlusal force can lead to a disorder called alveolar bone disuse osteoporosis (ABDO), characterized by a net loss of alveolar bone. Furthermore, recombinant Slit guidance ligand 3 (SLIT3) protein into the periodontal ligament can stimulate Type H angiogenesis and osteogenesis by activating the Piezo1/Ca2+/HIF-1α/SLIT3 signaling pathway (Chen et al., 2023).
Moreover, Piezo1 is a novel biophysical intervention for OP caused by various factors, such as aging, diminished mechanical stimulation (microgravity), and estrogen insufficiency. Therefore, Piezo1 may be crucial for astronauts or persons who undergo protracted immobility for fractured bones.
5.2 Piezo1 and bone fracture
A bone fracture is widely caused by significant mechanical force or strain, such as falling, vehicular collisions, or athletic traumas. Nevertheless, specific medical diseases, such as osteoporosis and certain cancer types, can compromise bone strength, rendering them more vulnerable to fractures, even when subjected to modest pressure. Notably, the duration of the healing process often spans from 4 to 8 weeks depending on age, overall health, and the specific nature of the fracture (Einhorn and Gerstenfeld, 2015).
Piezo1 downregulation impairs fracture healing in the callus (Chen P. et al., 2021), while Piezo1 chemical activation by Yoda1 enhances fracture healing by stimulating periosteal stem cells (PSCs)-modulated chondrogenesis and osteogenesis and expediting the transformation of cartilage into bone (Liu Y. et al., 2022). Moreover, Piezo1 activation can increase the expression of vascular endothelial growth factor A, suggesting that Piezo1 may have a secondary function in angiogenesis, which creates new blood vessels to feed oxygen and nutrients to the fracture site (Liu Y. et al., 2022).
Higher-intensity ultrasound can effectively accelerate fracture healing, particularly in a mouse osteoporotic fracture model, by accelerating the process of endochondral ossification through Piezo1 activation. However, Piezo1 inhibition by a specific inhibitor (GsMTx4) negatively affects fracture healing induced by ultrasound exposure (Inoue et al., 2023). Besides, Piezo1 can sense LIPUS and regulate the proliferation of osteoblasts by triggering the activation of ERK1/2 phosphorylation and perinuclear F-actin polymerization, indicating that Piezo1 can enhance fracture repair (Zhang et al., 2021).
5.3 Piezo1 and cancer
Breast cancer metastasis, particularly in the bone, significantly limits cancer treatment. Piezo1 regulates cancer cell migration and invasion by modulating cell adhesion, stiffness, and contractility, thus influencing invadopodia formation and MMP expression (Karska et al., 2023). Piezo1 modulates breast cancer metastases in the bone by affecting osteoclast and osteocyte activity. The Piezo1 ion channel is essential for osteocyte mechanotransduction. Besides, the chemical activation of Piezo1 ion channel enhances the capacity of osteocyte to prevent cancer extravasation under low-magnitude high-frequency (LMHF) vibration (Song et al., 2022). Cancer cells can penetrate and spread to bone through osteoclasts, which are cells that tear down bone tissue. Piezo1 stimulation improves osteocytes’ mechanoresponse to LMHF vibration, thus inhibiting osteoclastogenesis and decreasing MDA-MB-231, a type of breast cancer cell, from migrating (Lin C. Y. et al., 2022). In addition, Piezo1 is highly expressed in osteosarcoma (OS) cells and regulated apoptosis, invasion, and cell proliferation of OS cells (Jiang et al., 2017).
5.4 Piezo1 and tooth movement
Understanding the mechanism of alveolar bone remodeling under mechanical force is a primary concern in orthodontics. Alveolar bone and periodontal ligament (PDL) are closely related structures in periodontium development and mechanotransduction during orthodontic tooth movement (OTM). PDL, a vital connective and supporting tissue, attaches the tooth to the adjacent bone through collagen fiber bundles, enabling the tooth to disperse and withstand loading force, including the masticatory and orthodontic force. Osteoclasts, osteoblasts, osteocytes, periodontal ligament fibroblasts, and periodontal ligament stem cells in the periodontium function as sensory cells and effectors, converting mechanical force into intracellular signals and facilitating tooth movement induced by orthodontic force (Jiang et al., 2016; Zhou et al., 2022).
The established pressure-tension hypothesis indicates that orthodontic force induces PDL compression in certain areas where blood flow is reduced, and PDL stretch in others where the blood flow is enhanced or maintained. Different force stimuli in the PDL result in diverse biological reactions and chemical environments, including oxygen concentration and transcription factor levels, leading to bone resorption on the compression side and bone creation on the tension side (Meeran, 2012; McCormack et al., 2014) (Figure 5).
Piezo1 exhibits intense immunoreactivity in both human and murine periodontal ligaments (Kang et al., 2014; Gao et al., 2017; Gaite et al., 2023). Besides, Piezo1 is crucial for sustaining the rate of OTM and promoting alveolar bone remodeling on the tension side (Jiang Y. K. et al., 2021; Du and Yang, 2023). However, Nottmeier et al. (2023) proposed a different opinion that Piezo1 impairment has minimal effect on the tooth movement distance by establishing an OTM rat model. Nonetheless, a longer testing period may reveal significant variation in bone remodeling since the OTM model was only tested for 12 days.
Furthermore, Piezo1 offers a strong theoretical foundation for the potential use of 3D-printed implants in orthopedic surgery. The low stiffness of the three-dimensionally printed Ti2448 promoted angiogenesis and osteogenesis by enhancing the Piezo1/YAP signaling axis, which in turn regulated macrophage polarization (Tang et al., 2021).
6 Discussion and conclusion
Recent research has made notable advancements in uncovering the distinct structure and function of Piezo1 in various tissues and animals. Emerging evidence suggests that Piezo1 can detect mechanical stress and convert it into biological signals, thereby maintaining bone homeostasis. The latest research on the function of the Piezo1 channel in bone remodeling is thoroughly reviewed in this article. However, further investigation is essential to fully comprehend the underlying processes behind Piezo1-mediated bone remodeling. Such insights hold promising solutions for bone diseases and may expedite advancements in OTM techniques (Figure 6).
Author contributions
YD: Writing–original draft, Writing–review and editing. BX: Visualization, Writing–review and editing. QL: Visualization, Writing–review and editing. CP: Conceptualization, Writing–original draft. KY: Funding acquisition, Supervision, Writing–review and editing.
Funding
The author(s) declare financial support was received for the research, authorship, and/or publication of this article. This study was supported by the Beijing Natural Science Foundation (Grant Number 7222077), Capital’s Funds for Health Improvements and Research (Grant Number CFH2022-2-2144), National Natural Science Foundation of China (Grant Number 81771103), and the Beijing Hospitals Authority Ascent Plan (Grant Number DFL20191501 to KY).
Acknowledgments
We would like to thank the biorender and PyMOL developer for providing tools for visualizing figures and MJE editor (www.mjeditor.com) for the English editing of the manuscript.
Conflict of interest
The authors declare that the research was conducted in the absence of any commercial or financial relationships that could be construed as a potential conflict of interest.
Publisher’s note
All claims expressed in this article are solely those of the authors and do not necessarily represent those of their affiliated organizations, or those of the publisher, the editors and the reviewers. Any product that may be evaluated in this article, or claim that may be made by its manufacturer, is not guaranteed or endorsed by the publisher.
References
Abdallah, B. M., Jafari, A., Zaher, W., Qiu, W., and Kassem, M. (2015). Skeletal (stromal) stem cells: an update on intracellular signaling pathways controlling osteoblast differentiation. Bone 70, 28–36. doi:10.1016/j.bone.2014.07.028
Amado, N. G., Nosyreva, E. D., Thompson, D., Egeland, T. J., Ogujiofor, O. W., Yang, M., et al. (2024). PIEZO1 loss-of-function compound heterozygous mutations in the rare congenital human disorder Prune Belly Syndrome. Nat. Commun. 15 (1), 339. doi:10.1038/s41467-023-44594-0
Andolfo, I., Alper, S. L., De Franceschi, L., Auriemma, C., Russo, R., De Falco, L., et al. (2013). Multiple clinical forms of dehydrated hereditary stomatocytosis arise from mutations in PIEZO1. Blood 121, 3925–3935. doi:10.1182/blood-2013-02-482489
Bae, C., Sachs, F., and Gottlieb, P. A. (2011). The mechanosensitive ion channel Piezo1 is inhibited by the peptide GsMTx4. Biochemistry 50, 6295–6300. doi:10.1021/bi200770q
Bai, W. Y., Wang, L., Ying, Z. M., Hu, B., Xu, L., Zhang, G. Q., et al. (2020). Identification of PIEZO1 polymorphisms for human bone mineral density. Bone 133, 115247. doi:10.1016/j.bone.2020.115247
Beech, D. J., and Kalli, A. C. (2019). Force sensing by piezo channels in cardiovascular health and disease. Arterioscler. Thromb. Vasc. Biol. 39, 2228–2239. doi:10.1161/ATVBAHA.119.313348
Botello-Smith, W. M., Jiang, W., Zhang, H., Ozkan, A. D., Lin, Y. C., Pham, C. N., et al. (2019). A mechanism for the activation of the mechanosensitive Piezo1 channel by the small molecule Yoda1. Nat. Commun. 10, 4503. doi:10.1038/s41467-019-12501-1
Boyle, W. J., Simonet, W. S., and Lacey, D. L. (2003). Osteoclast differentiation and activation. Nature 423, 337–342. doi:10.1038/nature01658
Cai, G., Lu, Y., Zhong, W., Wang, T., Li, Y., Ruan, X., et al. (2023). Piezo1-mediated M2 macrophage mechanotransduction enhances bone formation through secretion and activation of transforming growth factor-β1. Cell Prolif. 56, e13440. doi:10.1111/cpr.13440
Cai, T., Sun, D., Duan, Y., Wen, P., Dai, C., Yang, J., et al. (2016). WNT/β-catenin signaling promotes VSMCs to osteogenic transdifferentiation and calcification through directly modulating Runx2 gene expression. Exp. Cell Res. 345, 206–217. doi:10.1016/j.yexcr.2016.06.007
Chen, P., Zhang, G., Jiang, S., Ning, Y., Deng, B., Pan, X., et al. (2021). Mechanosensitive Piezo1 in endothelial cells promotes angiogenesis to support bone fracture repair. Cell Calcium 97, 102431. doi:10.1016/j.ceca.2021.102431
Chen, S., Li, Z., Chen, D., Cui, H., Wang, J., Li, Z., et al. (2022). Piezo1-mediated mechanotransduction promotes entheseal pathological new bone formation in ankylosing spondylitis. Ann. Rheum. Dis. 82, 533–545. doi:10.1136/ard-2022-223428
Chen, Y., Jiang, Y., Chen, B., Qian, Y., Liu, J., Yang, M., et al. (2021). Case report: whole exome sequencing revealed two novel mutations of PIEZO1 implicated in nonimmune hydrops fetalis. Front. Genet. 12, 684555. doi:10.3389/fgene.2021.684555
Chen, Y., Yin, Y., Luo, M., Wu, J., Chen, A., Deng, L., et al. (2023). Occlusal force maintains alveolar bone homeostasis via type H angiogenesis. J. Dent. Res. 102 (12), 1356–1365. Epub 2023 Oct 3. PMID: 37786932. doi:10.1177/00220345231191745
Choi, D., Park, E., Jung, E., Cha, B., Lee, S., Yu, J., et al. (2019). Piezo1 incorporates mechanical force signals into the genetic program that governs lymphatic valve development and maintenance. JCI Insight 4, e125068. doi:10.1172/jci.insight.125068
Coste, B., Mathur, J., Schmidt, M., Earley, T. J., Ranade, S., Petrus, M. J., et al. (2010). Piezo1 and Piezo2 are essential components of distinct mechanically activated cation channels. Science 330, 55–60. doi:10.1126/science.1193270
Cross, D. A., Alessi, D. R., Cohen, P., Andjelkovich, M., and Hemmings, B. A. (1995). Inhibition of glycogen synthase kinase-3 by insulin mediated by protein kinase B. Nature 378, 785–789. doi:10.1038/378785a0
Dalghi, M. G., Clayton, D. R., Ruiz, W. G., Al-Bataineh, M. M., Satlin, L. M., Kleyman, T. R., et al. (2019). Expression and distribution of PIEZO1 in the mouse urinary tract. Am. J. Physiol. Ren. Physiol. 317, F303–F321. doi:10.1152/ajprenal.00214.2019
de Vries, T. J., Schoenmaker, T., Hooibrink, B., Leenen, P. J., and Everts, V. (2009). Myeloid blasts are the mouse bone marrow cells prone to differentiate into osteoclasts. J. Leukoc. Biol. 85, 919–927. doi:10.1189/jlb.0708402
Dienes, B., Bazsó, T., Szabó, L., and Csernoch, L. (2023). The role of the Piezo1 mechanosensitive channel in the musculoskeletal system. Int. J. Mol. Sci. 24 (7), 6513. doi:10.3390/ijms24076513
Du, Y., and Yang, K. (2023). Role of mechanosensitive ion channel Piezo1 in tension-side orthodontic alveolar bone remodeling in rats. Arch. Oral Biol. 155, 105798. doi:10.1016/j.archoralbio.2023.105798
Dupont, S., Morsut, L., Aragona, M., Enzo, E., Giulitti, S., Cordenonsi, M., et al. (2011). Role of YAP/TAZ in mechanotransduction. Nature 474, 179–183. doi:10.1038/nature10137
Dzamukova, M., Brunner, T. M., Miotla-Zarebska, J., Heinrich, F., Brylka, L., Mashreghi, M. F., et al. (2022). Mechanical forces couple bone matrix mineralization with inhibition of angiogenesis to limit adolescent bone growth. Nat. Commun. 13 (1), 3059. doi:10.1038/s41467-022-30618-8
Einhorn, T. A., and Gerstenfeld, L. C. (2015). Fracture healing: mechanisms and interventions. Nat. Rev. Rheumatol. 11 (1), 45–54. Epub 2014 Sep 30. doi:10.1038/nrrheum.2014.164
Evans, E. L., Cuthbertson, K., Endesh, N., Rode, B., Blythe, N. M., Hyman, A. J., et al. (2018). Yoda1 analogue (Dooku1) which antagonizes Yoda1-evoked activation of Piezo1 and aortic relaxation. Br. J. Pharmacol. 175 (10), 1744–1759. doi:10.1111/bph.14188
Feng, X., Li, S., Wang, S., Meng, Y., Zheng, S., Liu, C., et al. (2023). Piezo1 mediates the degradation of cartilage extracellular matrix in malocclusion-induced TMJOA. Oral Dis. 2023, 14615. doi:10.1111/odi.14615
Fotiou, E., Martin-Almedina, S., Simpson, M., Lin, S., Gordon, K., Brice, G., et al. (2015). Novel mutations in PIEZO1 cause an autosomal recessive generalized lymphatic dysplasia with non-immune hydrops fetalis. Nat. Commun. 6, 8085. doi:10.1038/ncomms9085
Gaite, J. J., Solé-Magdalena, A., García-Mesa, Y., Cuendias, P., Martin-Cruces, J., García-Suárez, O., et al. (2023). Immunolocalization of the mechanogated ion channels PIEZO1 and PIEZO2 in human and mouse dental pulp and periodontal ligament. Anat. Rec. Hob. 2023, 25351. Epub ahead of print. PMID: 37975162. doi:10.1002/ar.25351
Gan, D., Tao, C., Jin, X., Wu, X., Yan, Q., Zhong, Y., et al. (2023). Piezo1 activation accelerates osteoarthritis progression and the targeted therapy effect of artemisinin. J. Adv. Res. 25, S2090–S1232. doi:10.1016/j.jare.2023.09.040
Gao, Q., Cooper, P. R., Walmsley, A. D., and Scheven, B. A. (2017). Role of piezo channels in ultrasound-stimulated dental stem cells. J. Endod. 43, 1130–1136. doi:10.1016/j.joen.2017.02.022
Gaub, B. M., and Müller, D. J. (2017). Mechanical stimulation of Piezo1 receptors depends on extracellular matrix proteins and directionality of force. Nano Lett. 17 (3), 2064–2072. doi:10.1021/acs.nanolett.7b00177
Ge, J., Li, W., Zhao, Q., Li, N., Chen, M., Zhi, P., et al. (2015). Architecture of the mammalian mechanosensitive Piezo1 channel. Nature 527, 64–69. doi:10.1038/nature15247
Geng, J., Liu, W., Zhou, H., Zhang, T., Wang, L., Zhang, M., et al. (2020). A plug-and-latch mechanism for gating the mechanosensitive piezo channel. Neuron 106 (3), 438–451.e6. doi:10.1016/j.neuron.2020.02.010
Geng, J., Shi, Y., Zhang, J., Yang, B., Wang, P., Yuan, W., et al. (2021). TLR4 signalling via Piezo1 engages and enhances the macrophage mediated host response during bacterial infection. Nat. Commun. 12 (1), 3519. doi:10.1038/s41467-021-23683-y
Geng, J., Zhao, Q., Zhang, T., and Xiao, B. (2016). In touch with the mechanosensitive piezo channels: structure, ion permeation, and mechanotransduction. Curr. Top. Membr. 79, 159–195. doi:10.1016/bs.ctm.2016.11.006
Gong, Y., Slee, R. B., Fukai, N., Rawadi, G., Roman-Roman, S., Reginato, A. M., et al. (2001). LDL receptor-related protein 5 (LRP5) affects bone accrual and eye development. Cell 107, 513–523. doi:10.1016/s0092-8674(01)00571-2
Götschel, F., Kern, C., Lang, S., Sparna, T., Markmann, C., Schwager, J., et al. (2008). Inhibition of GSK3 differentially modulates NF-κB, CREB, AP-1 and β-catenin signaling in hepatocytes, but fails to promote TNF-α-induced apoptosis. Exp. Cell Res. 314, 1351–1366. doi:10.1016/j.yexcr.2007.12.015
Gottlieb, P. A. (2017). A tour de Force: the discovery, properties, and function of piezo channels. Curr. Top. Membr. 79, 1–36. doi:10.1016/bs.ctm.2016.11.007
Grannemann, C., Pabst, A., Honert, A., Schieren, J., Martin, C., Hank, S., et al. (2023). Mechanical activation of lung epithelial cells through the ion channel Piezo1 activates the metalloproteinases ADAM10 and ADAM17 and promotes growth factor and adhesion molecule release. Biomater Adv. 152, 213516. doi:10.1016/j.bioadv.2023.213516
Guan, H., Wang, W., Jiang, Z., Zhang, B., Ye, Z., Zheng, J., et al. (2023). Magnetic aggregation-induced bone-targeting nanocarrier with effects of Piezo1 activation and osteogenic-angiogenic coupling for osteoporotic bone repair. Adv. Mater 2023, e2312081. Epub ahead of print. PMID: 38102981. doi:10.1002/adma.202312081
Gudipaty, S., Lindblom, J., Loftus, P., Redd, M. J., Edes, K., Davey, C. F., et al. (2017). Mechanical stretch triggers rapid epithelial cell division through Piezo1. Nature 543, 118–121. doi:10.1038/nature21407
Guo, Y. R., and MacKinnon, R. (2017). Structure-based membrane dome mechanism for Piezo mechanosensitivity. eLife 6, e33660. doi:10.7554/eLife.33660
Hao, L., Li, L., Wang, P., Wang, Z., Shi, X., Guo, M., et al. (2019). Synergistic osteogenesis promoted by magnetically actuated nano-mechanical stimuli. Nanoscale 11, 23423–23437. doi:10.1039/C9NR07170A
Hasegawa, K., Fujii, S., Matsumoto, S., Tajiri, Y., Kikuchi, A., and Kiyoshima, T. (2021). YAP signaling induces PIEZO1 to promote oral squamous cell carcinoma cell proliferation. J. Pathol. 253, 80–93. doi:10.1002/path.5553
He, J., Xie, X., Xiao, Z., Qian, W., Zhang, L., and Hou, X. (2023). Piezo1 in digestive system function and dysfunction. Int. J. Mol. Sci. 24, 12953. doi:10.3390/ijms241612953
Hendrickx, G., Fischer, V., Liedert, A., von Kroge, S., Haffner-Luntzer, M., Brylka, L., et al. (2021). Piezo1 inactivation in chondrocytes impairs trabecular bone formation. J. Bone Min. Res. 36, 369–384. doi:10.1002/jbmr.4198
Horie, S., Nakatomi, C., Ito-Sago, M., Morii, A., Orimoto, A., Ikeda, H., et al. (2023). PIEZO1 promotes ATP release from periodontal ligament cells following compression force. Eur. J. Orthod. 45 (5), 565–574. doi:10.1093/ejo/cjad052
Hu, H., Hilton, M. J., Tu, X., Yu, K., Ornitz, D. M., and Long, F. (2005). Sequential roles of Hedgehog and Wnt signaling in osteoblast development. Development 132, 49–60. doi:10.1242/dev.01564
Hu, Y., Tian, H., Chen, W., Liu, Y., Cao, Y., Pei, H., et al. (2023). The critical role of the piezo1/β-catenin/ATF4 Axis on the stemness of Gli1+ BMSCs during simulated microgravity-induced bone loss. Adv. Sci. (Weinh) 10 (32), e2303375. doi:10.1002/advs.202303375
Imajo, M., Ebisuya, M., and Nishida, E. (2015). Dual role of YAP and TAZ in renewal of the intestinal epithelium. Nat. Cell Biol. 17, 7–19. doi:10.1038/ncb3084
Inoue, S., Li, C., Hatakeyama, J., Jiang, H., Kuroki, H., and Moriyama, H. (2023). Higher-intensity ultrasound accelerates fracture healing via mechanosensitive ion channel Piezo1. Bone 177, 116916. doi:10.1016/j.bone.2023.116916
James, A. W. (2013). Review of signaling pathways governing MSC osteogenic and adipogenic differentiation. Sci. (Cairo) 2013, 1–17. doi:10.1155/2013/684736
Jiang, N., Guo, W., Chen, M., Zheng, Y., Zhou, J., Kim, S. G., et al. (2016). Periodontal ligament and alveolar bone in health and adaptation: tooth movement. Front. Oral. Biol. 18, 1–8. doi:10.1159/000351894
Jiang, W., Wijerathne, T. D., Zhang, H., Lin, Y. C., Jo, S., Im, W., et al. (2023). Structural and thermodynamic framework for PIEZO1 modulation by small molecules. Proc. Natl. Acad. Sci. U. S. A. 12 (50), e2310933120. doi:10.1073/pnas.2310933120
Jiang, Y. D., Song, J., Xu, Y., Liu, C. Y., Qian, W., Bai, T., et al. (2021). Piezo1 regulates intestinal epithelial function by affecting the tight junction protein claudin-1 via the ROCK pathway. Life Sci. 275, 119254. doi:10.1016/j.lfs.2021.119254
Jiang, Y. K., Guan, Y., Lan, Y., Chen, S., Li, T., Zou, S., et al. (2021). Mechanosensitive Piezo1 in periodontal ligament cells promotes alveolar bone remodeling during orthodontic tooth movement. Front. Physiol. 12, 767136. doi:10.3389/fphys.2021.767136
Jiang, L., Zhao, Y. D., and Chen, W. X. (2017). The function of the novel mechanical activated ion channel Piezo1 in the human osteosarcoma cells. Med. Sci. Monit. 23, 5070–5082. doi:10.12659/msm.906959
Jin, S. S., He, D. Q., Wang, Y., Zhang, T., Yu, H. J., Li, Z. X., et al. (2020). Mechanical force modulates periodontal ligament stem cell characteristics during bone remodelling via TRPV4. Cell Prolif. 53, e12912. doi:10.1111/cpr.12912
Jin, Y., Li, J., Wang, Y., Ye, R., Feng, X., Jing, Z., et al. (2015). Functional role of mechanosensitive ion channel Piezo1 in human periodontal ligament cells. Angle Orthod. 85, 87–94. doi:10.2319/123113-955.1
Kang, T., Huang, S., Li, P., Shi, T., Yu, Q., Duan, Y., et al. (2014). Piezos’ expression in periodontal tissues of rats. Chin. J. Conserv. Dent. 24, 269–273. doi:10.15956/j.cnki.chin.j.conserv.dent.2014.05.006
Karska, J., Kowalski, S., Saczko, J., Moisescu, M. G., and Kulbacka, J. (2023). Mechanosensitive ion channels and their role in cancer cells. Membr. (Basel) 13 (2), 167. doi:10.3390/membranes13020167
Koga, T., Matsui, Y., Asagiri, M., Kodama, T., de Crombrugghe, B., Nakashima, K., et al. (2005). NFAT and Osterix cooperatively regulate bone formation. Nat. Med. 11, 880–885. doi:10.1038/nm1270
Kong, K., Chang, Y., Hu, Y., Qiao, H., Zhao, C., Rong, K., et al. (2022). TiO2 nanotubes promote osteogenic differentiation through regulation of yap and Piezo1. Front. Bioeng. Biotechnol. 10, 872088. doi:10.3389/fbioe.2022.872088
Koser, D. E., Thompson, A. J., Foster, S. K., Dwivedy, A., Pillai, E. K., Sheridan, G. K., et al. (2016). Mechanosensing is critical for axon growth in the developing brain. Nat. Neurosci. 19, 1592–1598. doi:10.1038/nn.4394
Lang, K., Breer, H., and Frick, C. (2018). Mechanosensitive ion channel Piezo1 is expressed in antral G cells of murine stomach. Cell Tissue Res. 371, 251–260. doi:10.1007/s00441-017-2755-0
Lee, S., Park, S., Kim, H. Y., Chae, J. H., and Ko, J. M. (2021). Extended phenotypes of PIEZO1-related lymphatic dysplasia caused by two novel compound heterozygous variants. Eur. J. Med. Genet. 64 (10), 104295. doi:10.1016/j.ejmg.2021.104295
Lee, W., Leddy, H. A., Chen, Y., Lee, S. H., Zelenski, N. A., McNulty, A. L., et al. (2014). Synergy between Piezo1 and Piezo2 channels confers high-strain mechanosensitivity to articular cartilage. Proc. Natl. Acad. Sci. U. S. A. 111, E5114–E5122. doi:10.1073/pnas.1414298111
Li, L. L., Li, X. F., Zhang, J. Y., Zhou, Y. W., and Yang, Q. N. (2021). Repair of osteoarthritis in animal model with exosomes derived from BMSCs transfected by the siRNA-Piezo1 through CT navigation. Zhongguo Gu Shang 34 (12), 1171–1178. doi:10.12200/j.issn.1003-0034.2021.12.015
Li, X., Han, L., Nookaew, I., Mannen, E., Silva, M. J., Almeida, M., et al. (2019). Stimulation of Piezo1 by mechanical signals promotes bone anabolism. Elife 8, e49631. doi:10.7554/eLife.49631
Li, X., Zhang, C., Bowman, H. H., Stambough, J. B., Stronach, B. M., Mears, S. C., et al. (2023). Piezo1 opposes age-associated cortical bone loss. Aging Cell 22, e13846. doi:10.1111/acel.13846
Li, X. F., Zhang, Z., Li, X. D., Wang, T. B., and Zhang, H. N. (2016). Mechanism of the Piezo1 protein-induced apoptosis of the chondrocytes through the MAPK/ERK1/2 signal pathway. Zhonghua Yi Xue Za Zhi 96, 2472–2477. doi:10.3760/cma.j.issn.0376-2491.2016.31.007
Lin, C. Y., Song, X., Ke, Y., Raha, A., Wu, Y., Wasi, M., et al. (2022). Yoda1 enhanced low-magnitude high-frequency vibration on osteocytes in regulation of MDA-MB-231 breast cancer cell migration. Cancers (Basel) 14 (14), 3395. doi:10.3390/cancers14143395
Lin, Y. Y., Ren, J., and McGrath, C. (2022). Mechanosensitive piezo1 and piezo2 ion channels in craniofacial development and dentistry: recent advances and prospects. Front. Physiol. 13, 1039714. doi:10.3389/fphys.2022.1039714
Liu, H. L., Hu, J., Zheng, Q., Feng, X., Zhan, F., Wang, X., et al. (2022). Piezo1 channels as force sensors in mechanical force-related chronic inflammation. Front. Immunol. 13, 816149. doi:10.3389/fimmu.2022.816149
Liu, Y., Tian, H., Hu, Y., Cao, Y., Song, H., Lan, S., et al. (2022). Mechanosensitive Piezo1 is crucial for periosteal stem cell-mediated fracture healing. Int. J. Biol. Sci. 18, 3961–3980. doi:10.7150/ijbs.71390
Liu, S., Fang, X., Hall, H., Yu, S., Smith, D., Lu, Z., et al. (2008). Homozygous deletion of glycogen synthase kinase 3β bypasses senescence allowing Ras transformation of primary murine fibroblasts. Proc. Natl. Acad. Sci. U. S. A. 105, 5248–5253. doi:10.1073/pnas.0704242105
Liu, S., Pan, X., Cheng, W., Deng, B., He, Y., Zhang, L., et al. (2020). Tubeimoside I antagonizes yoda1-evoked Piezo1 channel activation. Front. Pharmacol. 11, 768. doi:10.3389/fphar.2020.00768
Liu, Z., Tang, Y., He, L., Geng, B., Lu, F., He, J., et al. (2022). Piezo1-mediated fluid shear stress promotes OPG and inhibits RANKL via NOTCH3 in MLO-Y4 osteocytes. Channels (Austin) 16, 127–136. doi:10.1080/19336950.2022.2085379
Lu, N., and Malemud, C. J. (2019). Extracellular signal-regulated kinase: a regulator of cell growth, inflammation, chondrocyte and bone cell receptor-mediated gene expression. Int. J. Mol. Sci. 20 (15), 3792. doi:10.3390/ijms20153792
Lukacs, V., Mathur, J., Mao, R., Bayrak-Toydemir, P., Procter, M., Cahalan, S. M., et al. (2015). Impaired PIEZO1 function in patients with a novel autosomal recessive congenital lymphatic dysplasia. Nat. Commun. 6, 8329. doi:10.1038/ncomms9329
Mammoto, T., Hunyenyiwa, T., Kyi, P., Hendee, K., Matus, K., Rao, S., et al. (2022). Hydrostatic Pressure Controls Angiogenesis Through Endothelial YAP1 During Lung Regeneration. Front. Bioeng. Biotechnol. 10, 823642. doi:10.3389/fbioe.2022.823642
Manning, B. D., and Toker, A. (2017). AKT/PKB signaling: navigating the network. Cell 169, 381–405. doi:10.1016/j.cell.2017.04.001
Martin-Almedina, S., Mansour, S., and Ostergaard, P. (2018). Human phenotypes caused by PIEZO1 mutations; one gene, two overlapping phenotypes? J. Physiol. 596 (6), 985–992. doi:10.1113/JP275718
Matsunaga, M., Kimura, M., Ouchi, T., Nakamura, T., Ohyama, S., Ando, M., et al. (2021). Mechanical stimulation-induced calcium signaling by Piezo1 channel activation in human odontoblast reduces dentin mineralization. Front. Physiol. 12, 704518. doi:10.3389/fphys.2021.704518
McCormack, S. W., Witzel, U., Watson, P. J., Fagan, M. J., and Gröning, F. (2014). The biomechanical function of periodontal ligament fibres in orthodontic tooth movement. PLoS One 9 (7), e102387. doi:10.1371/journal.pone.0102387
Meeran, N. A. (2012). Biological response at the cellular level within the periodontal ligament on application of orthodontic force - An update. J. Orthod. Sci. 1 (1), 2–10. doi:10.4103/2278-0203.94769
Michishita, M., Yano, K., Tomita, K. I., Matsuzaki, O., and Kasahara, K. I. (2016). Piezo1 expression increases in rat bladder after partial bladder outlet obstruction. Life Sci. 166, 1–7. doi:10.1016/j.lfs.2016.10.017
Moroni, M., Servin-Vences, M. R., Fleischer, R., Sánchez-Carranza, O., and Lewin, G. R. (2018). Voltage gating of mechanosensitive PIEZO channels. Nat. Commun. 9 (1), 1096. doi:10.1038/s41467-018-03502-7
Mousawi, F., Peng, H., Li, J., Ponnambalam, S., Roger, S., Zhao, H., et al. (2020). Chemical activation of the Piezo1 channel drives mesenchymal stem cell migration via inducing ATP release and activation of P2 receptor purinergic signaling. Stem Cells 38, 410–421. doi:10.1002/stem.3114
Mulhall, E. M., Gharpure, A., Lee, R. M., Dubin, A. E., Aaron, J. S., Marshall, K. L., et al. (2023). Direct observation of the conformational states of PIEZO1. Nature 620, 1117–1125. doi:10.1038/s41586-023-06427-4
Murthy, S. E., Dubin, A. E., and Patapoutian, A. (2017). Piezos thrive under pressure: mechanically activated ion channels in health and disease. Nat. Rev. Mol. Cell Biol. 18, 771–783. doi:10.1038/nrm.2017.92
Nagai, S., Kitamura, K., Kimura, M., Yamamoto, H., Katakura, A., and Shibukawa, Y. (2023). Functional expression of mechanosensitive piezo1/TRPV4 channels in mouse osteoblasts. Bull. Tokyo Dent. Coll. 64, 1–11. doi:10.2209/tdcpublication.2022-0015
Nie, X., and Chung, M. K. (2022). Piezo channels for skeletal development and homeostasis: insights from mouse genetic models. Differentiation 126, 10–15. doi:10.1016/j.diff.2022.06.001
Nonomura, K., Lukacs, V., Sweet, D. T., Goddard, L. M., Kanie, A., Whitwam, T., et al. (2018). Mechanically activated ion channel PIEZO1 is required for lymphatic valve formation. Proc. Natl. Acad. Sci. U. S. A. 115 (50), 12817–12822. doi:10.1073/pnas.1817070115
Nottmeier, C., Lavicky, J., Lopez, M. G., Knauth, S., Kahl-Nieke, B., Amling, M., et al. (2023). Mechanical-induced bone remodeling does not depend on Piezo1 in dentoalveolar hard tissue. Sci. Rep. 13 (1), 9563. doi:10.1038/s41598-023-36699-9
Ozkan, A. D., Wijerathne, T. D., Gettas, T., and Lacroix, J. J. (2023). Force-induced motions of the PIEZO1 blade probed with fluorimetry. Cell Rep. 42 (8), 112837. doi:10.1016/j.celrep.2023.112837
Panchamanon, P., Pavasant, P., and Leethanakul, C. (2019). Periostin plays role in force-induced stem cell potential by periodontal ligament stem cells. Cell Biol. Int. 43, 506–515. doi:10.1002/cbin.11116
Parsonage, G., Cuthbertson, K., Endesh, N., Murciano, N., Hyman, A. J., Revill, C. H., et al. (2023). Improved PIEZO1 agonism through 4-benzoic acid modification of Yoda1. Br. J. Pharmacol. 180 (16), 2039–2063. doi:10.1111/bph.15996
Passini, F. S., Jaeger, P. K., Saab, A. S., Hanlon, S., Chittim, N. A., Arlt, M. J., et al. (2021). Shear-stress sensing by PIEZO1 regulates tendon stiffness in rodents and influences jumping performance in humans. Nat. Biomed. Eng. 5 (12), 1457–1471. Epub 2021 May 24. PMID: 34031557; PMCID: PMC7612848. doi:10.1038/s41551-021-00716-x
Pierce, J. L., Begun, D. L., Westendorf, J. J., and McGee-Lawrence, M. E. (2019). Defining osteoblast and adipocyte lineages in the bone marrow. Bone 118, 2–7. doi:10.1016/j.bone.2018.05.019
Poole, K., Herget, R., Lapatsina, L., Ngo, H. D., and Lewin, G. R. (2014). Tuning Piezo ion channels to detect molecular-scale movements relevant for fine touch. Nat. Commun. 5, 3520. doi:10.1038/ncomms4520
Ranade, S. S., Qiu, Z., Woo, S. H., Hur, S. S., Murthy, S. E., Cahalan, S. M., et al. (2014). Piezo1, a mechanically activated ion channel, is required for vascular development in mice. Proc. Natl. Acad. Sci. U. S. A. 111, 10347–10352. doi:10.1073/pnas.1409233111
Ranade, S. S., Syeda, R., and Patapoutian, A. (2015). Mechanically activated ion channels. Neuron 87, 1162–1179. doi:10.1016/j.neuron.2015.08.032
Ren, R., Guo, J., Chen, Y., Zhang, Y., Chen, L., and Xiong, W. (2021). The role of Ca (2+)/Calcineurin/NFAT signalling pathway in osteoblastogenesis. Cell Prolif. 54, e13122. doi:10.1111/cpr.13122
Ren, X., Zhuang, H., Li, B., Jiang, F., Zhang, Y., Zhou, P., et al. (2023). Gsmtx4 alleviated osteoarthritis through piezo1/calcineurin/NFAT1 signaling Axis under excessive mechanical strain. Int. J. Mol. Sci. 24 (4), 4022. doi:10.3390/ijms24044022
Retailleau, K., Duprat, F., Arhatte, M., Ranade, S. S., Peyronnet, R., Martins, J. R., et al. (2015). Piezo1 in smooth muscle cells is involved in hypertension-dependent arterial remodeling. Cell Rep. 13, 1161–1171. doi:10.1016/j.celrep.2015.09.072
Rostas, J. A. P., and Skelding, K. A. (2023). Calcium/calmodulin-Stimulated protein kinase II (CaMKII): different functional outcomes from activation, depending on the cellular microenvironment. Cells 12 (3), 401. doi:10.3390/cells12030401
Ruan, D. D., Gan, Y. M., Lu, T., Yang, X., Zhu, Y. B., Yu, Q. H., et al. (2020). Genetic diagnosis history and osteoarticular phenotype of a non-transfusion secondary hemochromatosis. World J. Clin. Cases 8 (23), 5962–5975. doi:10.12998/wjcc.v8.i23.5962
Sasaki, F., Hayashi, M., Mouri, Y., Nakamura, S., Adachi, T., and Nakashima, T. (2020). Mechanotransduction via the Piezo1-Akt pathway underlies Sost suppression in osteocytes. Biochem. Biophys. Res. Commun. 521, 806–813. doi:10.1016/j.bbrc.2019.10.174
Sato, M., Ogura, K., Kimura, M., Nishi, K., Ando, M., Tazaki, M., et al. (2018). Activation of mechanosensitive transient receptor potential/piezo channels in odontoblasts generates action potentials in cocultured isolectin B (4)-negative medium-sized trigeminal ganglion neurons. J. Endod. 44, 984–991.e2. doi:10.1016/j.joen.2018.02.020
Sayedyahossein, S., Thines, L., and Sacks, D. B. (2023). Ca2+ signaling and the Hippo pathway: intersections in cellular regulation. Cell Signal 110, 110846. doi:10.1016/j.cellsig.2023.110846
Schröder, A., Neher, K., Krenmayr, B., Paddenberg, E., Spanier, G., Proff, P., et al. (2023). Impact of PIEZO1-channel on inflammation and osteoclastogenesis mediated via periodontal ligament fibroblasts during mechanical loading. Eur. J. Oral Sci. 131 (1), e12913. doi:10.1111/eos.12913
Sciancalepore, M., Massaria, G., Tramer, F., Zacchi, P., Lorenzon, P., and Bernareggi, A. (2022). A preliminary study on the role of Piezo1 channels in myokine release from cultured mouse myotubes. Biochem. Biophys. Res. Commun. 623, 148–153. doi:10.1016/j.bbrc.2022.07.059
Seo, B. M., Miura, M., Gronthos, S., Bartold, P. M., Batouli, S., Brahim, J., et al. (2004). Investigation of multipotent postnatal stem cells from human periodontal ligament. Lancet 364, 149–155. doi:10.1016/S0140-6736(04)16627-0
Sheik Ali, A. (2023). Osteoporosis: a narrative review. Cureus 15 (8), e43031. doi:10.7759/cureus.43031
Shen, X., Wu, W., Ying, Y., Zhou, L., and Zhu, H. (2023). A regulatory role of Piezo1 in apoptosis of periodontal tissue and periodontal ligament fibroblasts during orthodontic tooth movement. Aust. Endod. J. 49 (1), 228–237. doi:10.1111/aej.12721
Shen, Y., Pan, Y., Guo, S., Sun, L., Zhang, C., and Wang, L. (2020). The roles of mechanosensitive ion channels and associated downstream MAPK signaling pathways in PDLC mechanotransduction. Mol. Med. Rep. 21, 2113–2122. doi:10.3892/mmr.2020.11006
Song, J., Liu, L., Lv, L., Hu, S., Tariq, A., Wang, W., et al. (2020). Fluid shear stress induces Runx-2 expression via upregulation of PIEZO1 in MC3T3-E1 cells. Cell Biol. Int. 44, 1491–1502. doi:10.1002/cbin.11344
Song, X., Lin, C. Y., Mei, X., Wang, L., and You, L. (2022). Reduction of breast cancer extravasation via vibration activated osteocyte regulation. iScience 25 (12), 105500. doi:10.1016/j.isci.2022.105500
Song, Y., Li, D., Farrelly, O., Miles, L., Li, F., Kim, S. E., et al. (2019). The mechanosensitive ion channel piezo inhibits axon regeneration. Neuron 102, 373–389.e6. doi:10.1016/j.neuron.2019.01.050
Sugimoto, A., Iwata, K., Kurogoushi, R., Tanaka, M., Nakashima, Y., Yamakawa, Y., et al. (2023). C-terminus of PIEZO1 governs Ca2+ influx and intracellular ERK1/2 signaling pathway in mechanotransduction. Biochem. Biophys. Res. Commun. 682, 39–45. doi:10.1016/j.bbrc.2023.09.080
Sugimoto, A., Miyazaki, A., Kawarabayashi, K., Shono, M., Akazawa, Y., Hasegawa, T., et al. (2017). Piezo type mechanosensitive ion channel component 1 functions as a regulator of the cell fate determination of mesenchymal stem cells. Sci. Rep. 7, 17696. doi:10.1038/s41598-017-18089-0
Sun, W., Chi, S., Li, Y., Ling, S., Tan, Y., Xu, Y., et al. (2019). The mechanosensitive Piezo1 channel is required for bone formation. Elife 8, e47454. doi:10.7554/eLife.47454
Sun, Y., Fang, Y., Li, X., Li, J., Liu, D., Wei, M., et al. (2023). A static magnetic field enhances the repair of osteoarthritic cartilage by promoting the migration of stem cells and chondrogenesis. J. Orthop. Transl. 39, 43–54. doi:10.1016/j.jot.2022.11.007
Sun, Y., Leng, P., Guo, P., Gao, H., Liu, Y., Li, C., et al. (2021b). G protein coupled estrogen receptor attenuates mechanical stress-mediated apoptosis of chondrocyte in osteoarthritis via suppression of Piezo1. Mol. Med. 27 (1), 96. doi:10.1186/s10020-021-00360-w
Sun, Y., Yuan, Y., Wu, W., Lei, L., and Zhang, L. (2021a). The effects of locomotion on bone marrow mesenchymal stem cell fate: insight into mechanical regulation and bone formation. Cell Biosci. 11, 88. doi:10.1186/s13578-021-00601-9
Syeda, R., Xu, J., Dubin, A. E., Coste, B., Mathur, J., Huynh, T., et al. (2015). Chemical activation of the mechanotransduction channel Piezo1. Elife 4, e07369. doi:10.7554/eLife.07369
Tang, Y., Feinberg, T., Keller, E. T., Li, X. Y., and Weiss, S. J. (2016). Snail/Slug binding interactions with YAP/TAZ control skeletal stem cell self-renewal and differentiation. Nat. Cell Biol. 18, 917–929. doi:10.1038/ncb3394
Tang, Z., Wei, X., Li, T., Wu, H., Xiao, X., Hao, Y., et al. (2021). Three-dimensionally printed Ti2448 with low stiffness enhanced angiogenesis and osteogenesis by regulating macrophage polarization via Piezo1/YAP signaling Axis. Front. Cell Dev. Biol. 9, 750948. doi:10.3389/fcell.2021.750948
Tao, H., Zhu, M., Lau, K., Whitley, O. K. W., Samani, M., Xiao, X., et al. (2019). Oscillatory cortical forces promote three dimensional cell intercalations that shape the murine mandibular arch. Nat. Commun. 10, 1703. doi:10.1038/s41467-019-09540-z
Tomita, M., Reinhold, M. I., Molkentin, J. D., and Naski, M. C. (2002). Calcineurin and NFAT4 induce chondrogenesis. J. Biol. Chem. 277, 42214–42218. doi:10.1074/jbc.C200504200
Totaro, A., Panciera, T., and Piccolo, S. (2018). YAP/TAZ upstream signals and downstream responses. Nat. Cell Biol. 20, 888–899. doi:10.1038/s41556-018-0142-z
Wang, B., Li, G., Zhu, Q., Liu, W., Ke, W., Hua, W., et al. (2022). Bone repairment via mechanosensation of Piezo1 using wearable pulsed triboelectric nanogenerator. Small 18 (30), e2201056. doi:10.1002/smll.202201056
Wang, J., Jiang, J., Yang, X., Zhou, G., Wang, L., Xiao, B., et al. (2022). Tethering Piezo channels to the actin cytoskeleton for mechanogating via the cadherin-β-catenin mechanotransduction complex. Cell Rep. 38 (6), 110342. doi:10.1016/j.celrep.2022.110342
Wang, L., Shen, H., Zheng, W., Tang, L., Yang, Z., Gao, Y., et al. (2011). Characterization of stem cells from alveolar periodontal ligament. Tissue Eng. Part A 17, 1015–1026. doi:10.1089/ten.tea.2010.0140
Wang, L., You, X., Lotinun, S., Zhang, L., Wu, N., and Zou, W. (2020). Mechanical sensing protein PIEZO1 regulates bone homeostasis via osteoblast-osteoclast crosstalk. Nat. Commun. 11, 282. doi:10.1038/s41467-019-14146-6
Wang, Q., Zhou, Y., Wang, X., and Evers, B. M. (2006). Glycogen synthase kinase-3 is a negative regulator of extracellular signal-regulated kinase. Oncogene 25, 43–50. doi:10.1038/sj.onc.1209004
Wang, S., Chennupati, R., Kaur, H., Iring, A., Wettschureck, N., and Offermanns, S. (2016). Endothelial cation channel PIEZO1 controls blood pressure by mediating flow-induced ATP release. J. Clin. Invest. 126, 4527–4536. doi:10.1172/JCI87343
Wang, Y., Chi, S., Guo, H., Li, G., Wang, L., Zhao, Q., et al. (2018). A lever-like transduction pathway for long-distance chemical- and mechano-gating of the mechanosensitive Piezo1 channel. Nat. Commun. 9, 1300. doi:10.1038/s41467-018-03570-9
Wang, Y., and Xiao, B. (2018). The mechanosensitive Piezo1 channel: structural features and molecular bases underlying its ion permeation and mechanotransduction. J. Physiol. 596 (6), 969–978. doi:10.1113/JP274404
Wong, T. Y., Juang, W. C., Tsai, C. T., Tseng, C. J., Lee, W. H., Chang, S. N., et al. (2018). Mechanical stretching simulates cardiac physiology and pathology through mechanosensor Piezo1. J. Clin. Med. 7, 410. doi:10.3390/jcm7110410
Wu, C. B., Ma, T., Ma, L., Wang, Q., and Zhou, Q. (2022). Piezo1 affects temporomandibular joint osteoarthritis by influencing pSmad3. Front. Physiol. 13, 892089. doi:10.3389/fphys.2022.892089
Wu, J., Lewis, A. H., and Grandl, J. (2017). Touch, tension, and transduction – the function and regulation of piezo ion channels. Trends Biochem. Sci. 42, 57–71. doi:10.1016/j.tibs.2016.09.004
Wu, R. W., Lian, W. S., Chen, Y. S., Ko, J. Y., Wang, S. Y., Jahr, H., et al. (2021). Piezoelectric microvibration mitigates estrogen loss-induced osteoporosis and promotes Piezo1, MicroRNA-29a, and Wnt3a signaling in osteoblasts. Int. J. Mol. Sci. 22 (17), 9476. doi:10.3390/ijms22179476
Xiao, B. (2020). Levering mechanically activated piezo channels for potential pharmacological intervention. Annu. Rev. Pharmacol. Toxicol. 60, 195–218. doi:10.1146/annurev-pharmtox-010919-023703
Xie, L., Wang, X., Ma, Y., Ma, H., Shen, J., Chen, J., et al. (2023). Piezo1 (Piezo-Type mechanosensitive ion channel component 1)-mediated mechanosensation in macrophages impairs perfusion recovery after hindlimb ischemia in mice. Arterioscler. Thromb. Vasc. Biol. 43 (4), 504–518. doi:10.1161/ATVBAHA.122.318625
Xing, Y., Yang, B., He, Y., Xie, B., Zhao, T., and Chen, J. (2022). Effects of mechanosensitive ion channel Piezo1 on proliferation and osteogenic differentiation of human dental follicle cells. Ann. Anat. 239, 151847. doi:10.1016/j.aanat.2021.151847
Xu, X., Liu, S., Liu, H., Ru, K., Jia, Y., Wu, Z., et al. (2021). Piezo channels: awesome mechanosensitive structures in cellular mechanotransduction and their role in bone. Int. J. Mol. Sci. 22, 6429. doi:10.3390/ijms22126429
Yan, W., Maimaitimin, M., Wu, Y., Fan, Y., Ren, S., Zhao, F., et al. (2023). Meniscal fibrocartilage regeneration inspired by meniscal maturational and regenerative process. Sci. Adv. 9 (45), eadg8138. doi:10.1126/sciadv.adg8138
Yan, X., Liu, J., Ye, Z., Huang, J., He, F., Xiao, W., et al. (2016). CaMKII-mediated CREB phosphorylation is involved in Ca2+-induced BDNF mRNA transcription and neurite outgrowth promoted by electrical stimulation. PLoS One 11 (9), e0162784. doi:10.1371/journal.pone.0162784
Yang, X., Lin, C., Chen, X., Li, S., Li, X., and Xiao, B. (2022). Structure deformation and curvature sensing of PIEZO1 in lipid membranes. Nature 604 (7905), 377–383. doi:10.1038/s41586-022-04574-8
Yu, Z. Y., Gong, H., Kesteven, S., Guo, Y., Wu, J., Li, J. V., et al. (2022). Piezo1 is the cardiac mechanosensor that initiates the cardiomyocyte hypertrophic response to pressure overload in adult mice. Nat. Cardiovasc Res. 1, 577–591. doi:10.1038/s44161-022-00082-0
Zeng, Y., Riquelme, M. A., Hua, R., Zhang, J., Acosta, F. A., Gu, S., et al. (2022). Mechanosensitive piezo1 calcium channel activates connexin 43 hemichannels through PI3K signaling pathway in bone. Cell Biosci. 12, 191. doi:10.1186/s13578-022-00929-w
Zhang, D., Lin, W., Jiang, S., Deng, P., Liu, L., Wang, Q., et al. (2023). Lepr-expressing PDLSCs contribute to periodontal homeostasis and respond to mechanical force by Piezo1. Adv. Sci. (Weinh) 10 (29), e2303291. doi:10.1002/advs.202303291
Zhang, G., Li, X., Wu, L., and Qian, Y. X. (2021). Piezo1 channel activation in response to mechanobiological acoustic radiation force in osteoblastic cells. Bone Res. 9, 16. doi:10.1038/s41413-020-00124-y
Zhang, L., Su, P., Xu, C., Chen, C., Liang, A., Du, K., et al. (2010). Melatonin inhibits adipogenesis and enhances osteogenesis of human mesenchymal stem cells by suppressing PPARγ expression and enhancing Runx2 expression. J. Pineal Res. 49, 364–372. doi:10.1111/j.1600-079X.2010.00803.x
Zhang, X., Hou, L., Li, F., Zhang, W., Wu, C., Xiang, L., et al. (2022). Piezo1-mediated mechanosensation in bone marrow macrophages promotes vascular niche regeneration after irradiation injury. Theranostics 12 (4), 1621–1638. doi:10.7150/thno.64963
Zhao, Q., Wu, K., Geng, J., Chi, S., Wang, Y., Zhi, P., et al. (2016). Ion permeation and mechanotransduction mechanisms of mechanosensitive piezo channels. Neuron 89, 1248–1263. doi:10.1016/j.neuron.2016.01.046
Zhao, Q., Zhou, H., Chi, S., Wang, F., Wang, J., Geng, J., et al. (2018). Structure and mechanogating mechanism of the Piezo1 channel. Nature 554, 487–492. doi:10.1038/nature25743
Zhao, Q., Zhou, H., Li, X., and Xiao, B. (2019). The mechanosensitive Piezo1 channel: a three-bladed propeller-like structure and a lever-like mechanogating mechanism. FEBS J. 286, 2461–2470. doi:10.1111/febs.14711
Zheng, Q., Zou, Y., Teng, P., Chen, Z., Wu, Y., Dai, X., et al. (2022). Mechanosensitive Channel PIEZO1 senses shear force to induce KLF2/4 expression via CaMKII/MEKK3/ERK5 Axis in endothelial cells. Cells 11 (14), 2191. doi:10.3390/cells11142191
Zhou, T., Gao, B., Fan, Y., Liu, Y., Feng, S., Cong, Q., et al. (2020). Piezo1/2 mediate mechanotransduction essential for bone formation through concerted activation of NFAT-YAP1-ß-catenin. Elife 9, e52779. doi:10.7554/eLife.52779
Zhou, M., and Graves, D. T. (2022). Impact of the host response and osteoblast lineage cells on periodontal disease. Front. Immunol. 13, 998244. doi:10.3389/fimmu.2022.998244
Zhou, Y., Zhang, C., Zhou, Z., and Wang, J. (2022). Identification of key genes and pathways associated with PIEZO1 in bone-related disease based on bioinformatics. Int. J. Mol. Sci. 23 (9), 5250. doi:10.3390/ijms23095250
Zhou, Z., Li, J. V., Martinac, B., and Cox, C. D. (2021). Loss-of-Function Piezo1 mutations display altered stability driven by ubiquitination and proteasomal degradation. Front. Pharmacol. 12, 766416. doi:10.3389/fphar.2021.766416
Keywords: Piezo1, bone remodeling, osteoblasts, osteoclasts, mechanical force
Citation: Du Y, Xu B, Li Q, Peng C and Yang K (2024) The role of mechanically sensitive ion channel Piezo1 in bone remodeling. Front. Bioeng. Biotechnol. 12:1342149. doi: 10.3389/fbioe.2024.1342149
Received: 21 November 2023; Accepted: 16 January 2024;
Published: 08 February 2024.
Edited by:
Jerome J. Lacroix, Western University of Health Sciences, United StatesReviewed by:
Jian Shi, University of Leeds, United KingdomTibor Rohacs, Rutgers, The State University of New Jersey, United States
Copyright © 2024 Du, Xu, Li, Peng and Yang. This is an open-access article distributed under the terms of the Creative Commons Attribution License (CC BY). The use, distribution or reproduction in other forums is permitted, provided the original author(s) and the copyright owner(s) are credited and that the original publication in this journal is cited, in accordance with accepted academic practice. No use, distribution or reproduction is permitted which does not comply with these terms.
*Correspondence: Kai Yang, ZHJfeWFuZ2thaUAxNjMuY29t