- Department of Biomechanical Engineering, Faculty of Mechanical Engineering, Delft University of Technology (TU Delft), Delft, Netherlands
The antibacterial biofunctionality of bone implants is essential for the prevention and treatment of implant-associated infections (IAI). In vitro co-culture models are utilized to assess this and study bacteria-host cell interactions at the implant interface, aiding our understanding of biomaterial and the immune response against IAI without impeding the peri-implant bone tissue regeneration. This paper reviews existing co-culture models together with their characteristics, results, and clinical relevance. A total of 36 studies were found involving in vitro co-culture models between bacteria and osteogenic or immune cells at the interface with orthopedic antibacterial biomaterials. Most studies (∼67%) involved co-culture models of osteogenic cells and bacteria (osteo-bac), while 33% were co-culture models of immune cells and bacterial cells (im-bac). All models involve direct co-culture of two different cell types. The cell seeding sequence (simultaneous, bacteria-first, and cell-first) was used to mimic clinically relevant conditions and showed the greatest effect on the outcome for both types of co-culture models. The im-bac models are considered more relevant for early peri-implant infections, whereas the osteo-bac models suit late infections. The limitations of the current models and future directions to develop more relevant co-culture models to address specific research questions are also discussed.
1 Introduction
The effectiveness of antibiotics is diminishing due to the global proliferation of drug resistance, resulting in infections that are increasingly challenging to treat. This phenomenon, known as antimicrobial resistance (AMR), is responsible for the death of approximately 1.5 million individuals annually (World Health Organization, 2012; EARS-net, 2014; O’Neill, 2014; CDC, 2017; Burnham et al., 2019). If this trend continues in the upcoming decades, it will have a strong detrimental effect on the quality of life worldwide, particularly impacting medical procedures like surgery and implant replacements, as they will pose an increased risk of infection. Therefore, continued research on understanding, prevention, and treatment of AMR-related infections is crucial.
In the field of orthopedics, implant-associated infections (IAIs) can occur as bacteria (including multidrug-resistant ones) attach to and form a biofilm on the surface of an implant. This can lead to osteomyelitis, debilitating pain, severe inflammation, swelling, septic implant loosening, and, in the worst-case scenario, limb amputation or even death (Elek, 1956; Zimmerli et al., 1982; Masters et al., 2019; Saeed et al., 2019). These infections are difficult to cure, and for the currently used cementless orthopedic implants, there are no solutions to effectively prevent them. Consequently, it is essential to devise strategies to prevent biofilm formation on the implant surface. Many different surfaces are investigated for preventing bacterial adhesion, growth, and biofilm formation, which can primarily be categorized into bacteria-repellent, bactericidal and bacteriostatic surfaces (Modaresifar et al., 2019). The former type of surfaces prevents bacteria adhesion and, thus, inhibits biofilm formation. However, these surfaces may also repel host cells, which is not favorable for permanent bone implants, where implant osseointegration is vital for long-term implant survival, especially for cementless bone implants (Wang et al., 2011). In contrast, bacteriostatic and bactericidal surfaces can respectively suppress bacterial growth or kill bacteria by releasing antimicrobials, such as antibiotics (Cicuéndez et al., 2018), antimicrobial peptides (AMPs) (Boix-Lemonche et al., 2020a), or metal ions (Guo et al., 2017; Cochis et al., 2020; Shuai et al., 2024) into the immediate microenvironment for a certain period, or through contact killing effects induced by specific surface topographies (Izquierdo-Barba et al., 2015; Damiati et al., 2022).
For orthopedic applications, much of current research is directed toward finding biomaterials that can be both antibacterial and osteogenic. Over the past 2 decades, however, the importance of the interaction between the bone and immune cells, commonly referred to as osteoimmunology, has gained increased recognition and has been considered integral to the process of osseointegration (Takayanagi, 2007; Mountziaris et al., 2011; Loi et al., 2016; Mestres et al., 2021). Acute inflammation is known to be a critical initial step in bone healing (Marsell and Einhorn, 2011), and the communication that takes place between the immune and skeletal cells upon implantation plays a vital role in determining the eventual success or failure of the implant. Despite its significance, this interaction remains a largely overlooked and elusive aspect of research in the case of antibacterial biomaterials. The immune response to these biomaterials and its modulation may help in the prevention and treatment of IAIs (Dong et al., 2022). A successful novel biomaterial for orthopedic applications should be able to modulate the immune response toward bone regeneration while minimizing the chance of peri-implant infections (Feng et al., 2023). Toward this aim, suitable in vitro co-culture models are needed to study the potential of orthopedic biomaterials to orchestrate the interactions between the immune, stromal/osteoprogenitor, and bacterial cells.
This article provides a general overview of the currently available in vitro co-culture models used to assess the antibacterial properties of orthopedic biomaterials. The review is focused on the co-culture models involving bacteria with immune and/or osteogenic cells, and describes their main characteristics, methodology, results, and clinical relevance. The findings are discussed in the context of clinical requirements and the limitations of the current models. Finally, we present a perspective on further developments in this important area of research.
2 Results
2.1 Literature search output
Based on the steps described in the Supplementary Material (Methods section), a total of 36 articles were found to fulfill the inclusion criteria (i.e., research focused on in vitro co/tri-culture of bacteria with immune and/or osteogenic cells on orthopedic biomaterials). The first study was published in 2004, and since then, the interest has increased (Figure 1). The results of the literature search are detailed in Supplementary Tables S2–S4 (see Supplementary Material) and are summarized in the following sections.
2.2 Type of co-culture models and their characteristics
The different co-culture models found in the literature have been firstly divided based on the combination of the cells used in the model. After that, several culture conditions were further considered to characterize the different co-culture models. Namely, the type of bacterial, osteogenic, and immune cells investigated, the direct/indirect culture conditions, the cell seeding sequence, the ratio between the cells used in the co-cultures, the co-culture time, and the static or dynamic nature of the culture conditions.
All the included studies used the co-culture of bacteria with either osteogenic or immune cells. No tri-culture model was found (Figure 2A). Therefore, co-culture models were categorized into two different groups: (1) co-culture of osteogenic and bacterial cells (osteo-bac) and (2) co-culture of immune and bacterial cells (im-bac). The osteo-bac models were the most frequently encountered (∼67%).
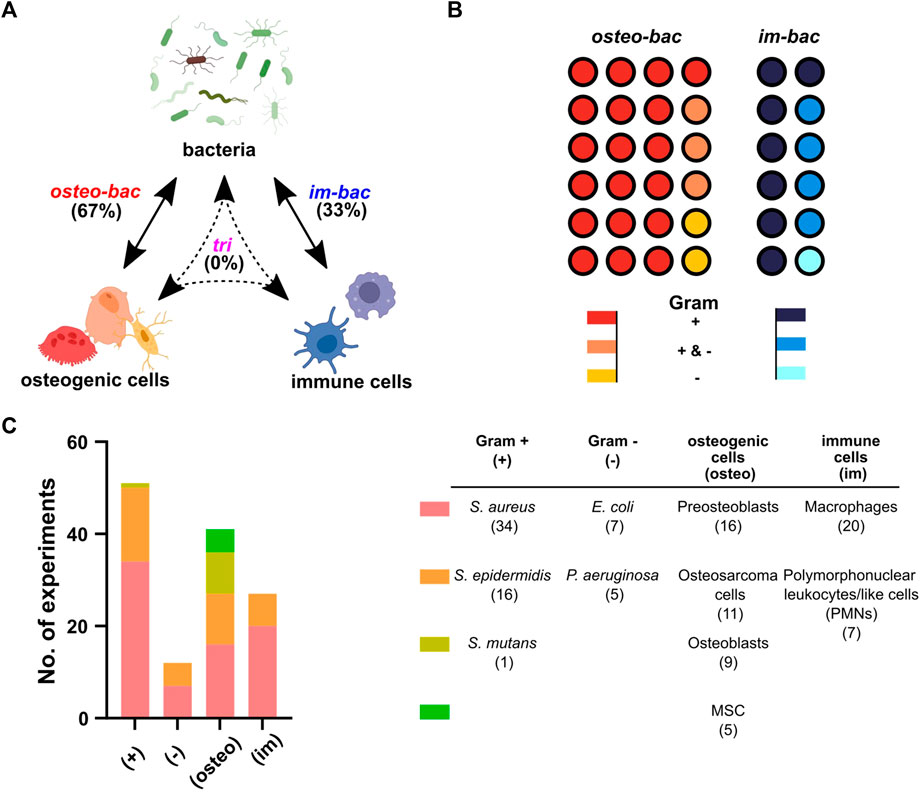
FIGURE 2. Co-culture models based on the involved cell type. (A) The terminology used in this review for the different co-culture models: in vitro models concerning osteogenic and bacteria (osteo-bac), immune cells and bacteria (im-bac) or all three in a tri-culture (tri). Created with BioRender.com (B) The distribution of the different co-culture models between the 36 articles. A distinction has been also made between the studies using Gram-positive (+) bacteria, Gram-negative (−) bacteria, or both (+&−). (C) An overview of cell types used in the experiments together with the specific cells per type.
As expected, the majority of the articles focused on Gram-positive bacteria (Figure 2B). The most commonly researched Gram-positive bacteria were Staphylococcus aureus (34 experiments), followed by Staphylococcus epidermidis (16 experiments), and Streptococcus mutans (1 experiment) (Figure 2C). Three articles focused only on the Gram-negative and seven on both Gram-positive and Gram-negative bacteria. The Gram-negative bacteria examined included Escherichia coli (7 experiments) and Pseudomonas aeruginosa (5 experiments). Regarding the osteogenic cells, preosteoblasts were the most frequently encountered cells. As for the immune cells, macrophages were the most prevalent cell types (Figure 2C).
Notably, all the studies were based on direct co-culture models. Interestingly, in the osteo-bac co-culture models, half of the studies used a host cell medium (αMEM or DMEM), whereas the other half used a combination of a host cell medium (αMEM or DMEM) and a bacterial-cell medium (Mueller Hinton Broth, Luria Bertoni, NB Basal Medium or Todd Hewitt Broth), with the proportion of the bacterial-cell medium ranging between 2% and 50%. In comparison, all of the im-bac studies used a host cell medium (RPMI-1640, IMDM, αMEM or DMEM), except for one study which used heparinized blood to assess its effects on the viability, phagocytic activity, and tissue regeneration capabilities of polymorphonuclear neutrophils (PMNs) as compared to the standard culture medium (RPMI-1640) (Guo et al., 2017).
Further characterization of these models was based on the cell seeding sequence and the ratio between both types of cells used in the cultures. Regarding the seeding order (Figures 3A, B), three different combinations were encountered: simultaneous seeding of both cell types (bac + osteo/im), bacteria-first (bac→osteo/im), and osteo/immune cell-first (osteo/im→bac). Most of the studies used a cell-first seeding sequence (19 studies). Alternative terms were associated with these different seeding methods to reflect some clinical scenarios. Namely, “competition” for the simultaneous seeding, “prevention” for bac→osteo/im sequence, and “protection” (of adhered host cells) for the osteo/im→bac sequence. Alternatively, the terms “race for the surface,” “perioperative,” and “post-operative” infection models were used to describe the same concepts, respectively (Yue et al., 2014; Deshmukh et al., 2016; Cochis et al., 2020).
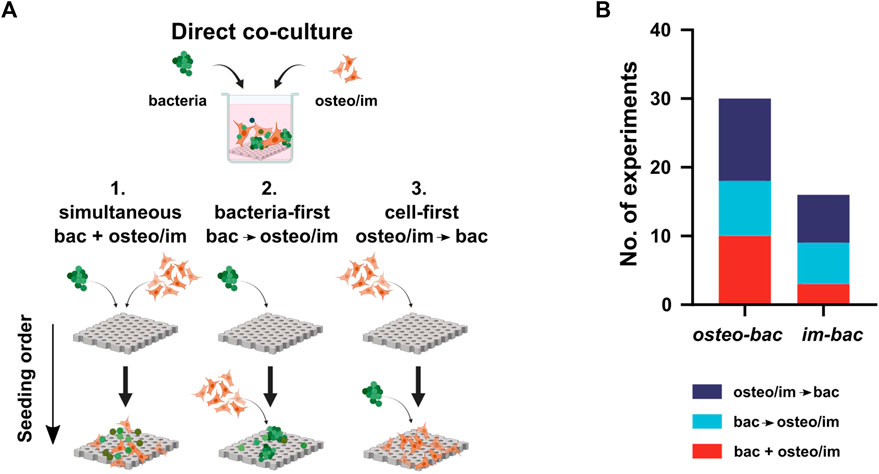
FIGURE 3. (A) The direct co-culture models use three different seeding sequences of bacteria and host cells: 1. Simultaneous (bac + osteo/im), 2. Bacteria-first, (bac→osteo/im), and 3. Cell-first (osteo/im→bac). Created with BioRender.com. (B) The distribution of the seeding sequences among the experiments.
The multiplicity of infection (MOI), particle-to-cell ratio, or infection ratio were used to define the ratio of the bacteria to the host cells in the co-culture (Wagner and Bryers, 2004; Svensson et al., 2014; Ghimire et al., 2016; Li et al., 2017; Mendoza et al., 2017; Zaatreh et al., 2017; Chu et al., 2018; Ellett et al., 2019). The MOI was calculated for all the included studies to shed light on the MOI trends between the models (Figure 4A). Remarkably, the MOIs values in the osteo-bac models were commonly within the range of 0.025–50 (∼90%), with very few exceptions (Ghimire et al., 2016; Mohiti-Asli et al., 2016; Cicuéndez et al., 2018; Sánchez-Salcedo et al., 2023). The MOIs observed in im-bac models were between 0.01 and 200 with most of the studies using a MOI between 1 and 100 (Figure 4B). In the im-bac models using simultaneous seeding, MOIs were always in the range of 10–100.
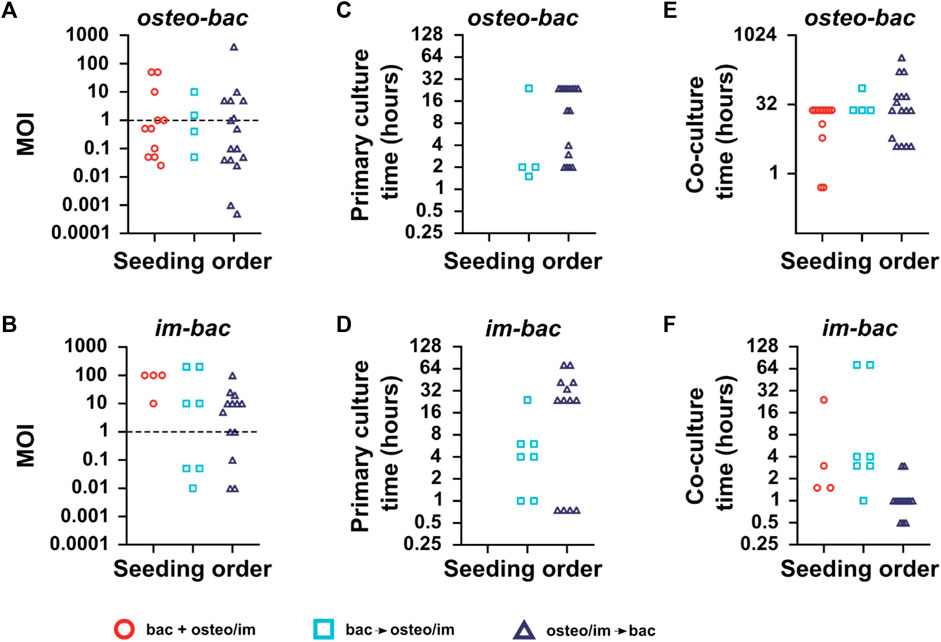
FIGURE 4. (A) The multiplicities of infection (MOIs), the ratio of bacteria to host-cells used in the direct co-culture models for each seeding sequence for osteo-bac and (B) im-bac models. (C) The primary culture time (i.e., the initial culture time for the first cell type seeded on the biomaterial). for osteo-bac models and for (D) im-bac models. (E) The co-culture time (i.e., the total co-culture time for both cell types on the biomaterial) for osteo-bac models and for (F) im-bac models. Studies involving the usage of dynamic co-culture models or experiments in which the MOI, primary culture time, or co-culture time was not mentioned are excluded.
Regarding the culture time, a distinction was made between the primary culture time and co-culture time. The former refers to the culture time of the first seeded cell type while the latter refers to the co-culture time. In the case of osteo-bac models (Figure 4C), the primary culture times varied between 0.5 and 24 h. The primary culture time of im-bac models (Figure 4D) was between 0.75 and 72 h.
The co-culture durations for the osteo-bac models varied depending on the culture sequence and were in the ranges of 0.5–24 h, 24–72 h, and 4 h up to 14 days for simultaneous, bacteria-first, and cell-first seeding, respectively (Figure 4E). These differences in the co-culture time were influenced by the specific research questions investigated in the studies. Some studies focused on the initial adhesion of bacteria, which could be examined within 30 min (Foss et al., 2015), while others assessed the long-term osteogenic modulation of highly antibacterial surfaces, requiring a co-culture duration of 14 days (Mohiti-Asli et al., 2016). In the case of the im-bac models (Figure 4F), the co-culture time did not exceed 72 h. For simultaneous seeding, the time varied between 1.5 and 24 h, while for bacteria-first seeding, the range extended from 1 to 72 h. In the case of the cell-first models, the primary culture duration spanned from 0.5 to 4 h. The shorter co-culture times in the cell-first studies can be explained by the focus of those studies on the immediate immunomodulatory effect of the biomaterial upon seeding. However, in the study by Zwicker and co-workers (Zwicker et al., 2022), the co-culture time was extended to 72 h to observe a polarization shift in the macrophages. No such shift was, however, observed in the co-culture while it did occur in their monoculture experiments.
Most co-cultures (∼85%) were conducted under static conditions. Among the four studies utilizing dynamic co-culture conditions, two involved osteo-bac co-culture models while the other two were im-bac models, all four of which used a bacteria-first sequence (Lee et al., 2011; Gu et al., 2014; Yue et al., 2014; Ellett et al., 2019). Interestingly, the two osteo-bac models focused on the effect of shear stress in lab-on-a-chip (LOC) devices, whereas only one im-bac model focused on the effect of flow on macrophage mobility and the other on neutrophil migration under static conditions in a LOC, to minimize the effect of fluid shear stress on cell behavior.
2.3 Effects of osteogenic and immune cells on the antibacterial properties of orthopedic biomaterials
2.3.1 Osteo-bac models
The primary research focus of the studies using the osteo-bac co-culture models has usually been to investigate the antibacterial effectiveness of the biomaterials in the presence of bone-like cells (Supplementary Table S2). This has been mainly assessed by looking at the surface coverage of both cell types.
Cell-first studies (i.e., when preosteoblasts were seeded first) (Zaatreh et al., 2016; Zaatreh et al., 2017; Tran and Tran, 2021) revealed interesting insights regarding the interaction of both cell types. Whereas the silver (Ag) nanoparticles present on the surface of titanium specimens prevented biofilm formation during bacterial monoculture, during co-culture using the cell-first sequence, S. aureus survived on top and inside preosteoblasts (MC3T3-E1), using them as a shield against the antimicrobial surface (Tran and Tran, 2021). This behavior decreased both the viability of osteogenic cells and the antibacterial efficacy of the biomaterial. The same trend has been observed for S. epidermidis in contact with a titanium surface with a rapidly corroding magnesium layer. While the coated-titanium specimens exhibited antibacterial behavior during bacterial monoculture, they were ineffective during cell-first osteo-bac co-culture (Zaatreh et al., 2016; Zaatreh et al., 2017).
When S. aureus was seeded first, higher preosteoblast (MC3T3-E1) death, as compared to simultaneous and cell-first experiments, was observed for a biomaterial consisting of hydroxyapatite (HA) to promote preosteoblast adhesion and chitosan to inhibit bacterial survival (Chu et al., 2018). Even with a low MOI (1:20), the antibacterial properties of this biomaterial were not strong enough to prevent bacteria from adhering to its surface and starting to form biofilms. This resulted in preosteoblasts not being able to attach to the biomaterial surface and proliferate.
In contrast to the static conditions, the dynamic perfusion systems allowed for a method for assessing the live behavior of bone cells during healing with and without the presence of a microbial threat. To illustrate the progression of wound healing, the effects of flow on the bone formation of MC3T3-E1 preosteoblasts were assessed. In addition, the administration of S. epidermidis into the channel led to the development of biofilm and adversely affected the osteogenic differentiation of the cells inside this 3D flow system (Lee et al., 2011). Another study, using osteosarcoma cells, also utilized a flow system to assess the surface coverage by osteosarcoma cells and S. epidermidis (MOI = 1:1) on (non-) anodized Ti and Ti6Al4V surfaces (Yue et al., 2014). Generally, the dynamic culture conditions facilitated a better distribution of bacteria at the beginning of each experiment, negatively impacting the survival of human osteosarcoma cells on non-anodized surfaces. The anodized surfaces proved to be successful in inhibiting bacterial growth and improving host cell attachment and surface coverage.
In all of the aforementioned studies, different methods were utilised for the assessment of bacterial and host cell survival on top of the implants. A basic method used for assessing the antibacterial efficiency of a biomaterial in the presence of osteogenic cells was by counting the colony-forming unit (CFU) after removing adhered bacteria from the biomaterial by sonication (Supplementary Table S3) (Lee et al., 2011; Mohiti-Asli et al., 2016; Jia et al., 2021). To compare the survival of both cell types, several studies detached the mammalian cells from the surface with trypsin, followed by cell counting using Trypan blue and a cell counter (Foss et al., 2015; Ghimire et al., 2016; Zaatreh et al., 2016; Zaatreh et al., 2017; Martínez-Pérez et al., 2019; Cochis et al., 2020). The surface coverage or morphological changes in both cell types was determined by scanning electron microscopy (SEM) (Foss et al., 2015; Jia et al., 2016; Mohiti-Asli et al., 2016; Zaatreh et al., 2016; Zaatreh et al., 2017; Chu et al., 2018; Qiu et al., 2019; Shen et al., 2020; Tan et al., 2020; Rivera et al., 2021; Damiati et al., 2022), optical microscopy (Lee et al., 2011; Jia et al., 2016; Tran and Tran, 2021), and fluorescence microscopy (Yue et al., 2014; Foss et al., 2015; Jia et al., 2016; Chu et al., 2018; Cicuéndez et al., 2018; Martínez-Pérez et al., 2019; Qiu et al., 2019; Tao et al., 2019; Boix-Lemonche et al., 2020b; Boix-Lemonche et al., 2021; Jia et al., 2021; Sánchez-Salcedo et al., 2023).
In addition to assessing host or bacterial cell survival in terms of cell number or morphological features, several studies investigated the invasion rate of bacteria, to highlight the infection rate of bacteria on different substrates. Different methods were employed to quantify the number of internalized bacteria inside bone cells, as a form of determining the invasion rate of bacteria into the host cells. Fluorescence imaging has been employed using cytoskeletal and nucleus staining combined with pre-stained bacteria (Yue et al., 2014; Foss et al., 2015; Jia et al., 2016; Chu et al., 2018; Cicuéndez et al., 2018; Qiu et al., 2019; Tao et al., 2019; Boix-Lemonche et al., 2020b; Boix-Lemonche et al., 2021; Jia et al., 2021). Additionally, acridine orange has been utilized to stain bacterial DNA and visualize the internalization of bacteria by the osteogenic cells (Chu et al., 2018; Martínez-Pérez et al., 2019; Sánchez-Salcedo et al., 2023). Another technique to quantify internalized bacteria involves destroying the cellular membrane to release all the internalized bacteria, known as the “Gentamicin protocol” (Jia et al., 2016; Tran and Tran, 2021). This method entails removing all extracellular bacteria using gentamicin or PBS, lysing the cells to release intracellular bacteria, and performing CFU counting or live/dead staining of the released bacteria.
Another technique for assessing cell death or bacterial invasion was by measuring lactate dehydrogenase (LDH), which is released by host cells when they die (Chu et al., 2018; Cicuéndez et al., 2018). Notably, one study employed qPCR to quantitatively assess biofilm formation after 24 h of co-culture (Chu et al., 2018). By sonication of the biomaterials in PBS, the biofilm was collected and biofilm-specific genes (i.e., icaA, icaD, hld, and spa) were measured. Finally, the osteogenic response of host cells to bacteria and biomaterials has been evaluated to a limited extent using ALP, OCN (Shen et al., 2020), RUNX2 (Damiati et al., 2022), and ARS staining (Lee et al., 2011; Mohiti-Asli et al., 2016).
2.3.2 Im-bac models
All the im-bac co-culture studies, except for one, focused on the recruitment and pro-inflammatory response of immune cells (PMNs and macrophages) and how the biomaterial affects this response in a co-culture model (Supplementary Table S2).
Yang et al. (Yang et al., 2021) showed the effects of the three different seeding sequences, all using the same MOI (10:1), with S. aureus and Raw 264.7 macrophages on UV/ozone irradiated antibacterial titanium surfaces (Ti-UV). The strongest antibacterial effects, as determined by the increased reactive oxygen species (ROS) production of macrophages in combination with more internalized bacteria, were achieved when macrophages were seeded first on the biomaterial surface followed by bacterial seeding after 24 h. In the case of the simultaneous and bacteria-first model, the macrophages did not have adequate space to survive and proliferate on the surface, leading to a reduced rate of bacterial killing by macrophages.
One interesting aspect of this study was that during the monoculture of macrophages on the Ti-UV surface, expression of pro-inflammatory genes (TNF-α and IL-6) were downregulated, whereas IL-10 and Arg-1 were upregulated, indicating a reduced foreign body response (FBR). Reduced early production of excessive ROS by macrophages in response to the Ti-UV surface helped create a favorable environment for the macrophages as compared to Ti. This controlled regulation of ROS production facilitated and enhanced the antibacterial and phagocytic activity of macrophages against bacteria.
By modulating the macrophage response in this way, the Ti-UV surface demonstrated potential benefits for promoting a favorable immune response, reducing the foreign body response, and potentially improving the biocompatibility of this biomaterial for this application.
In addition, this was the only study which also tested the osteoimmunomodulatory effect of the Ti-UV biomaterial on MSCs. They found that the conditioned medium (CM) from LPS-stimulated macrophages cultured on this biomaterial improves the osteogenic response of the MSCs.
Only one im-bac study has directly focused on macrophage polarization to limit the foreign body response toward a contact-killing surface instead of focusing on enhanced phagocytosis (Zwicker et al., 2022). The monoculture of THP-1 stimulated macrophages on Ti6Al4V, with and without Poly (hexamethylene) biguanide hydrochloride (PHMB) coating, showed that the layer did not induce a pro-inflammatory response. During bacteria-first co-culture, this material enabled macrophage attachment, viability, and improved tissue regeneration through early killing of the bacteria.
In two im-bac studies, LOC devices were developed with a focus on achieving high-output systems capable of simultaneous handling of a large number of experiments (Gu et al., 2014; Ellett et al., 2019). These devices were specifically designed to enhance the throughput and efficiency of the experiments. For example, a chip was created with a chitosan and glass-patterned surface to reveal macrophage mobility and phagocytic activity in response to bacterial presence (Gu et al., 2014). Under a constant flow to refresh the culture media, it was seen that the macrophage mobility was increased on chitosan as compared to glass, which reduced the bacterial presence on chitosan. Using a patterned surface, multiple parts on the same chip were used to compare the differences between macrophages on both surfaces. Finally, another LOC device allowed for quick and easy assessment of neutrophil recruitment to bacteria (Ellett et al., 2019). This device consisted of multiple smaller inner and one large outer compartment, which were only connected with a channel. The recruitment of neutrophils from the outer part toward the inner compartments, in which bacteria were present, was assessed using ROS staining.
Similar to osteo-bac models, the combination of CFU count (Deshmukh et al., 2016; Chen et al., 2017) with cell count (Wagner and Bryers, 2004; Gu et al., 2014; Svensson et al., 2014; Guo et al., 2017; Reigada et al., 2020; Zwicker et al., 2022) has been the most commonly used approach to evaluate the antibacterial effectiveness of biomaterials in the presence of the immune cells (Supplementary Table S4).
SEM imaging to see lysed cells or bacteria on the surface or on top of immune cells was a commonly used method to assess the survival of immune and bacterial cells (Svensson et al., 2014; Mendoza et al., 2017; Hou et al., 2019; Reigada et al., 2020; Yang et al., 2021). The staining of both macrophages and bacteria, followed by confocal microscopy (Zwicker et al., 2022) or, in some cases, pre-staining of bacteria (Wagner and Bryers, 2004; Chen et al., 2017) or flow cytometry (Chen et al., 2017; Mendoza et al., 2017), have been used to assess the number of internalized bacteria inside macrophages. In addition, the previously mentioned gentamycin protocol has also been used to determine the rate of phagocytosis by macrophages (Li et al., 2017; Mendoza et al., 2017), instead of determining the rate of invasion, as was the case in the osteo-bac studies.
Remarkably, while LDH measurements were used to determine the number of lysed host cells in osteo-bac models, it was used to determine bacterial death in im-bac models (Svensson et al., 2014; Guo et al., 2017). A final method of assessing phagocytosis was by looking at the ROS activity of the immune cells while being co-cultured with bacteria. The level of oxidative stress inside the immune cells is a direct indication of an enhanced pro-inflammatory response (Wagner and Bryers, 2004; Svensson et al., 2014; Ellett et al., 2019; Yang et al., 2021).
Only a few studies have looked into the effects of biomaterials on immune response by measuring the expression of pro- (IL-1β, TNF-α, IL-6) and anti- (IL-8, IL-10) inflammatory markers, using qPCR or ELISA (Wagner and Bryers, 2004; Svensson et al., 2014). One study has specifically tried to use these markers to assess the polarization shift after 1, 2, and 3 days of co-culture (Zwicker et al., 2022).
3 Discussion
Assessment of antibacterial biomaterials under more relevant clinical conditions requires novel in vitro culture models that incorporate different cell types, ECM components, and soluble factors. Here, we reviewed the co-culture models currently used for the investigation of orthopedic antibacterial biomaterials. It is worth noting that the early results published at the beginning of the 2000s used im-bac models. Later, the osteo-bac models started to be also used and quickly exceeded the im-bac models in number. The slow pace of new studies in this field indicates a lack of recognition within the biomedical community regarding the magnitude of this issue. It underscores not only the necessity for developing better biomaterials but also emphasizes the requirement for enhanced in vitro investigations to narrow the gap between biomaterial development and clinical applications. The knowledge resulting from such models has created new frontiers in the study of cell-surface and cell-cell interactions, which could impact the future design of orthopedic antibacterial biomaterials. In particular, the dominant paradigm is expected to shift from dual (i.e., osteogenic and antibacterial) functionalities to a triple-biofunctionality paradigm encompassing immuno-modulatory, osteogenic, and antibacterial responses. Such a more comprehensive paradigm is likely to improve the prevention and treatment of implant-associated infections particularly in the light of the emergence of antibiotic-resistant strains. Furthermore, the findings of the included studies point toward new approaches and methodologies that may improve the current models and their output, by more strongly embracing emerging technologies, such as microfluidics, 3D printing, and the use of advanced bioreactors.
The results of this review revealed that all the co-culture models used to date are based on direct cultures of bacteria with either osteogenic or immune cells. In general, the direct co-culture models are considered more relevant than the indirect co-culture models, primarily because the cells are subjected to both physical and paracrine interactions during the cell culture experiments (Hayrapetyan et al., 2016; Sakthivel et al., 2019). This is also true for co-culture models involving bacteria and host cells, where direct interactions between these cell types are critical in defining the effectiveness of the biomaterial concerning the viability and functional efficacy of host cells as well as the targeted antibacterial biofunctionality. It is, therefore, not surprising that no studies have used indirect co-culture models. Nevertheless, indirect co-culture models may be of relevance when addressing specific research questions, such as how to delineate the effects of an antibacterial agent released from the biomaterial surface from the contact-killing effects, as shown in a study by Sánchez-Salcedo et al. (Sánchez-Salcedo et al., 2023). The indirect osteo-immunomodulatory effects of an im-bac co-culture on MSCs is another such example (Yang et al., 2021).
The selection of the direct co-culture models should be based on the research questions addressed. From a biomaterial perspective, it may be more relevant to make a distinction between early and late IAIs (Trampuz et al., 2005; Ribeiro et al., 2012; Cochis et al., 2020) and develop (co-culture) models that address each specific type of infection (Figure 5). The micro-environment in vivo is far more complicated than in vitro. Focusing on a specific type of infection may enable researchers to better mimic the most relevant aspects of in vivo conditions in their in vitro co-culture models. The phases following implantation are hemostasis, inflammation, tissue repair, and tissue remodeling (Marsell and Einhorn, 2011; Ribeiro et al., 2012; Carnicer-Lombarte et al., 2021). An early in vitro infection model should, therefore, include bacteria and inflammatory cells (im-bac models) with the possible addition of blood components (to represent the early hemostasis phase), followed by the addition of stromal/progenitor cells at a later time point. Until now, only a few studies have focused on the hemostasis phase and the effects of serum or blood on the antibacterial efficiency of a biomaterial, even though the addition of blood provides a clinically relevant microenvironment, which is beneficial for reducing the discrepancy between in vitro and in vivo outcomes (Wagner and Bryers, 2004; Guo et al., 2017; Li et al., 2017; Hou et al., 2019).
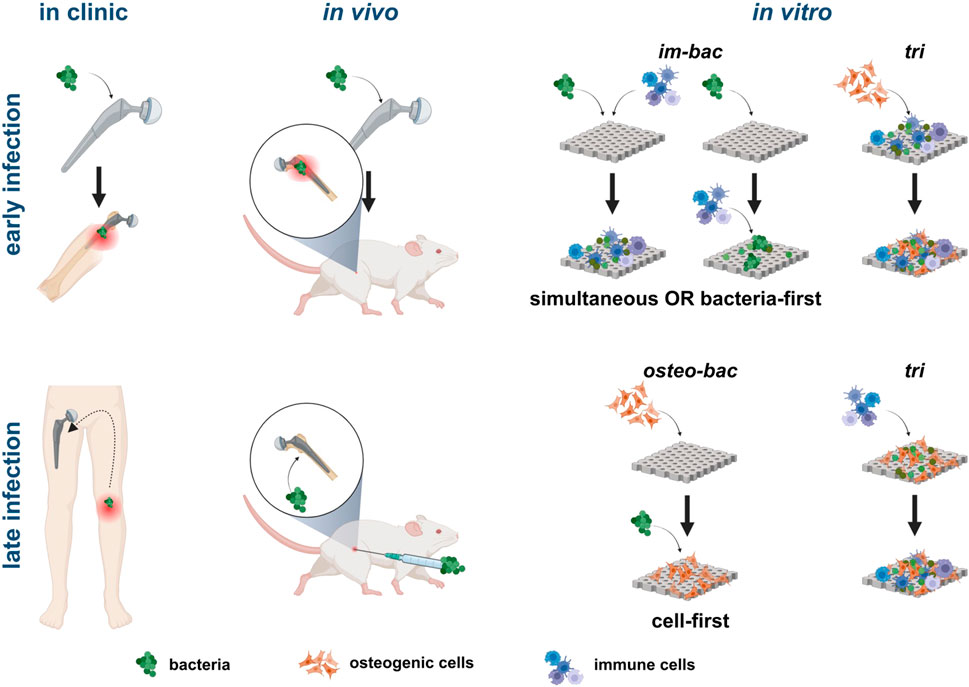
FIGURE 5. Illustration of clinical and in vivo scenarios directly related to different in vitro co-culture models. For simulating early infections, models where bacteria and immune cells are introduced simultaneously or with bacteria first are more relevant. The addition of bone-forming cells in a triple culture model can simulate the progression of the infection in the peri-implant area. For late-stage infections, seeding bone cells first before adding bacteria is more clinically relevant with the subsequent addition of immune cells in a triple culture adding complexity to the system. Created with BioRender.com.
The inflammatory response following the hemostasis phase during an early infection can be best mimicked by im-bac models in which the macrophages will be present within hours after infection (Martinez, 2008). These time points are also seen in the included im-bac models, where the focus has been on the pro-inflammatory response during this initial inflammatory response toward a foreign body or bacterial infection (Arciola et al., 2012). Longer co-culture (>7 days) for macrophages may result in automatic phenotype change toward M2, altering their behavior and function (Chamberlain et al., 2015). Overall, optimal culture times depend on the bacterial and immune cell types used, each strain having distinct proliferation times, and the research question addressed.
Although the seeding sequence can be established based on the type of infections addressed, the in vitro results reviewed here reveal a few interesting findings related to the effects of the immune cells on the bacteria during co-culture experiments. In im-bac cell-first models, for example, despite enough room being available for bacteria, fewer S. aureus adhered on the biomaterial surface as most were attached to or were found inside macrophages. While im-bac cell-first models may not have direct relevance to in vivo investigations, they hold significant potential in understanding the immunomodulatory effects of biomaterials and their implications for antibacterial efficacy. As for the MOI, a low MOI is more clinically relevant for early infections as compared to high MOIs that are more representative of late infections (Fitzgerald, 1979; Ribeiro et al., 2012). In addition, a low MOI also helps researchers to achieve successful bacteria-first co-culture, as the early adhesion and surface coverage of bacteria can create a cytotoxic microenvironment and compromise the adhesion of host cells to the biomaterial while also adversely affecting the proliferation of such cells (Yue et al., 2014).
In the case of late infections, the bone tissue is already present on the implant surface. The co-culture models should, therefore, include bone/osteoprogenitors cells, bacteria, and the (vascularized) bone matrix, followed by the addition of immune cells within a few hours after the onset of the infection. The osteo-bac models combined with a cell-first seeding sequence can closely mimic this situation. Furthermore, in post-operative infections, high MOIs can be expected since the bacterial load could go toward 104–106 bacteria (Fitzgerald, 1979; Ribeiro et al., 2012).
The primary culture time of the osteo-bac models reviewed here did not exceed 24 h, meaning that no bone formation could have taken place. Such experiments are, however, crucial in initial assessments of the effectiveness of antibacterial surfaces. While in some cases the biomaterial is observed to suppress the biofilm formation in monocultures, bacteria were observed on top of osteogenic cells in the co-culture assessments of the same biomaterials (Zaatreh et al., 2016; Zaatreh et al., 2017; Tran and Tran, 2021). This phenomenon could be due to the preference of bacteria for attaching to eukaryotic surfaces rather than to biomaterials (Alkow and Portnoy, 1992). Knowledge of this bacterial survival mechanism is essential because the bacteria attached to host cells may survive even if the substrate has antibacterial properties (Tran et al., 2020). The common perspective of assessing the “race for the surface” does not apply in such cases (Gristina et al., 1989). The battle is not only for the surface but also for survival, as adhered osteogenic cells alone may not effectively combat bacterial infections. This highlights the importance of having surfaces that can both directly kill the bacteria on their surface and release antibacterial agents in the bone tissue surrounding the implant without affecting the viability of the host cells. Such observations could have major implications for the future directions of biomaterial research. The addition of immune cells or using an early infection model followed by the addition of osteoprogenitor cells could better mimic the battle for survival.
In future co-culture models of both early and late infections, immune cells from the innate and/or adaptive immune system should be considered. Such an approach will enable us to better understand and harness the endogenous antibacterial and regenerative potential of the host organism. Such advanced models can lead to a reduction of animal studies while shedding light on relevant fundamental questions related to IAIs.
Based on the recent fundamental knowledge generated in the field, investigations using relatively less complex models may be helpful to investigate, for example, the role of immune cells as antibacterial “drugs,” the surface-induced pro-inflammatory effects for a controlled duration, and the phagocytosis vs invasion processes. While internalized bacteria inside immune cells can indicate successful phagocytosis (Yang et al., 2021), they could also suggest successful invasion (Li et al., 2017; Mendoza et al., 2017). CFU counting of internalized bacteria in lysed macrophages can be argued to assess the incapability of macrophages to eradicate the threat, as the bacteria are still alive inside them, thus measuring the invasion rate (Mendoza et al., 2017). Conversely, a reduction in the number of invading bacteria may be due to the biomaterial’s success in managing cell invasion (Li et al., 2017). Understanding such aspects could lead to novel design criteria for antibacterial biomaterials.
Additional possibilities to scavenge the bacteria from or kill them within the tissue are relevant and may include the modulation of the response of the immune cells to maximize their antibacterial function. The results of the included co-culture models seem to indicate that the surface/biomaterial antibacterial effects are surmounted by cell-cell communication. More detailed investigations are, however, needed to elucidate the effects of the antibacterial surface per se in such models. The existing co-culture models should, therefore, be further improved to accommodate relevant tissue components and microenvironmental conditions (such as flow) for each infection type. This could be achieved by using emerging technologies such as 3D bioprinting of microtissues, organ-on-chip models, advanced bioreactors, and perfusion systems. In addition, advanced single-cell studies and the associated methodologies offer new tools for fundamental understanding of cell-biomaterial interactions. Applying these tools to novel and relevant co- and tri-culture models is essential for the assessment of multifunctional biomaterials and should become part of the research methodology used for developing such biomaterials. This approach will not only reveal unprecedented fundamental knowledge on the working mechanism of such biomaterials but will also contribute to decreasing the dependence on in vivo models thereby fostering the research towards the clinics. Future orthopedic antibacterial biomaterials should be able to prevent both early and late IAIs. This may require more advanced concepts, such as on-demand drug delivery upon external/endogenous stimuli. Such advancements are to be expected in the near future.
4 Conclusion
The development of multifunctional biomaterials presents significant challenges in identifying suitable in vitro culture models to effectively evaluate their capacity to regulate immune responses, enhance bone regeneration, and prevent peri-implant infections. This review focused on the co-culture models currently used for the assessment of the efficacy of antibacterial orthopedic biomaterials. The findings have revealed the use of either im-bac (co-culture of immune cells and bacteria) or osteo-bac (co-culture of osteogenic cells and bacteria) models, all of which have been direct co-culture models. The seeding sequence employed in these models was different depending on the type of peri-implant infection investigated (e.g., early vs. late infections). The im-bac models are considered better suited for the study of early peri-implant infections, while osteo-bac models are particularly relevant to late infections. Future developments of such co-culture models are expected to include the incorporation of more relevant components and cell types.
Author contributions
BIME: Conceptualization, Data curation, Formal Analysis, Methodology, Writing–original draft, Writing–review and editing. IA: Conceptualization, Funding acquisition, Project administration, Supervision, Writing–review and editing. LEF-A: Conceptualization, Formal Analysis, Funding acquisition, Methodology, Supervision, Writing–review and editing. AAZ: Conceptualization, Funding acquisition, Project administration, Supervision, Writing–review and editing.
Funding
The author(s) declare financial support was received for the research, authorship, and/or publication of this article. This publication is part of the project DARTBAC (with project number NWA.1292.19.354 of the research programme NWA-ORC which is (partly) financed by the Dutch Research Council (NWO).
Conflict of interest
The authors declare that the research was conducted in the absence of any commercial or financial relationships that could be construed as a potential conflict of interest.
The author(s) declared that they were an editorial board member of Frontiers, at the time of submission. This had no impact on the peer review process and the final decision.
Publisher’s note
All claims expressed in this article are solely those of the authors and do not necessarily represent those of their affiliated organizations, or those of the publisher, the editors and the reviewers. Any product that may be evaluated in this article, or claim that may be made by its manufacturer, is not guaranteed or endorsed by the publisher.
Supplementary material
The Supplementary Material for this article can be found online at: https://www.frontiersin.org/articles/10.3389/fbioe.2024.1332771/full#supplementary-material
References
Alkow, S. F., and Portnoy, D. A. (1992). THE INTERACTION OF BACTERIA WITH MAMMALIAN CELLS. Available at: www.annualreviews.org (Accessed March 1, 2023).
Arciola, C. R., Hänsch, G. M., Visai, L., Testoni, F., Maurer, S., Campoccia, D., et al. (2012). Interactions of staphylococci with osteoblasts and phagocytes in the pathogenesis of implant-associated osteomyelitis. Int. J. Artif. Organs 35, 713–726. doi:10.5301/IJAO.5000158
Boix-Lemonche, G., Guillem-Marti, J., D’Este, F., Manero, J. M., and Skerlavaj, B. (2020a). Covalent grafting of titanium with a cathelicidin peptide produces an osteoblast compatible surface with antistaphylococcal activity. Colloids Surfaces B Biointerfaces 185, 110586. doi:10.1016/j.colsurfb.2019.110586
Boix-Lemonche, G., Guillem-Marti, J., D’Este, F., Manero, J. M., and Skerlavaj, B. (2020b). Covalent grafting of titanium with a cathelicidin peptide produces an osteoblast compatible surface with antistaphylococcal activity. Colloids Surfaces B Biointerfaces 185, 110586. doi:10.1016/J.COLSURFB.2019.110586
Boix-Lemonche, G., Guillem-Marti, J., Lekka, M., D’Este, F., Guida, F., Manero, J. M., et al. (2021). Membrane perturbation, altered morphology and killing of Staphylococcus epidermidis upon contact with a cytocompatible peptide-based antibacterial surface. Colloids Surfaces B Biointerfaces 203, 111745. doi:10.1016/j.colsurfb.2021.111745
Burnham, J. P., Olsen, M. A., and Kollef, M. H. (2019). Re-estimating annual deaths due to multidrug-resistant organism infections. Infect. Control Hosp. Epidemiol. 40, 112–113. doi:10.1017/ice.2018.304
Carnicer-Lombarte, A., Chen, S.-T., Malliaras, G. G., and Barone, D. G. (2021). Foreign body reaction to implanted biomaterials and its impact in nerve neuroprosthetics. Front. Bioeng. Biotechnol. 9, 622524. doi:10.3389/fbioe.2021.622524
CDC (2017). Antibiotic use in the United States, 2017: progress and opportunities. Atlanta, GA: US Department of Health and Human Services.
Chamberlain, L. M., Holt-Casper, D., Gonzalez-Juarrero, M., and Grainger, D. W. (2015). Extended culture of macrophages from different sources and maturation results in a common M2 phenotype. J. Biomed. Mat. Res. Part A 103, 2864–2874. doi:10.1002/jbm.a.35415
Chen, J., Howell, C., Haller, C. A., Patel, M. S., Ayala, P., Moravec, K. A., et al. (2017). An immobilized liquid interface prevents device associated bacterial infection in vivo. Biomaterials 113, 80–92. doi:10.1016/j.biomaterials.2016.09.028
Chu, L., Yang, Y., Yang, S., Fan, Q., Yu, Z., Hu, X.-L., et al. (2018). Preferential colonization of osteoblasts over Co-cultured bacteria on a bifunctional biomaterial surface. Front. Microbiol. 9, 2219–2313. doi:10.3389/fmicb.2018.02219
Cicuéndez, M., Doadrio, J. C., Hernández, A., Portolés, M. T., Izquierdo-Barba, I., and Vallet-Regí, M. (2018). Multifunctional pH sensitive 3D scaffolds for treatment and prevention of bone infection. Acta Biomater. 65, 450–461. doi:10.1016/j.actbio.2017.11.009
Cochis, A., Barberi, J., Ferraris, S., Miola, M., Rimondini, L., Vernè, E., et al. (2020). Competitive surface colonization of antibacterial and bioactive materials doped with strontium and/or silver ions. Nanomaterials 10, 120. doi:10.3390/nano10010120
Damiati, L. A., Tsimbouri, M. P., Hernandez, V.-L., Jayawarna, V., Ginty, M., Childs, P., et al. (2022). Materials-driven fibronectin assembly on nanoscale topography enhances mesenchymal stem cell adhesion, protecting cells from bacterial virulence factors and preventing biofilm formation. Biomaterials 280, 121263. doi:10.1016/j.biomaterials.2021.121263
Deshmukh, K. R., Ramanan, S. R., and Kowshik, M. (2016). Low-temperature-processed biocompatible Ag-HAp nanoparticles with anti-biofilm efficacy for tissue engineering applications. J. Sol-Gel Sci. Technol. 80, 738–747. doi:10.1007/s10971-016-4149-2
Dong, J., Wang, W., Zhou, W., Zhang, S., Li, M., Li, N., et al. (2022). Immunomodulatory biomaterials for implant-associated infections: from conventional to advanced therapeutic strategies. Biomater. Res. 26, 72. doi:10.1186/S40824-022-00326-X
Ears-net, (2014). Antimicrobial resistance - annual epidemiological report for 2014. Available at: https://www.ecdc.europa.eu/en/publications-data/antimicrobial-resistance-ears-net-annual-epidemiological-report-2014 (Accessed August 24, 2022).
Elek, S. D. (1956). Experimental staphylococcal infections in the skin of man. Ann. N. Y. Acad. Sci. 65, 85–90. doi:10.1111/J.1749-6632.1956.TB36626.X
Ellett, F., Jalali, F., Marand, A. L., Jorgensen, J., Mutlu, B. R., Lee, J., et al. (2019). Microfluidic arenas for war games between neutrophils and microbes. Lab. Chip 19, 1205–1216. doi:10.1039/C8LC01263F
Feng, P., He, R., Gu, Y., Yang, F., Pan, H., and Shuai, C. (2023). Construction of antibacterial bone implants and their application in bone regeneration. Mat. Horizons. doi:10.1039/d3mh01298k
Fitzgerald, R. H. (1979). Microbiologic environment of the conventional operating room. Arch. Surg. 114, 772. doi:10.1001/archsurg.1979.01370310014003
Foss, B. L., Ghimire, N., Tang, R., Sun, Y., and Deng, Y. (2015). Bacteria and osteoblast adhesion to chitosan immobilized titanium surface: a race for the surface. Colloids Surfaces B Biointerfaces 134, 370–376. doi:10.1016/j.colsurfb.2015.07.014
Ghimire, N., Foss, B. L., Sun, Y., and Deng, Y. (2016). Interactions among osteoblastic cells, Staphylococcus aureus, and chitosan-immobilized titanium implants in a postoperative coculture system: an in vitro study. J. Biomed. Mat. Res. Part A 104, 586–594. doi:10.1002/jbm.a.35597
Gristina, A. G., Naylor, P. T., and Myrvik, Q. (1989). “The race for the surface: microbes, tissue cells, and biomaterials,” in Molecular mechanisms of microbial adhesion (New York, NY: Springer New York), 177–211. doi:10.1007/978-1-4612-3590-3_15
Gu, Y., Zhang, W., Wang, H., and Lee, W. Y. (2014). Chitosan surface enhances the mobility, cytoplasm spreading, and phagocytosis of macrophages. Colloids Surfaces B Biointerfaces 117, 42–50. doi:10.1016/j.colsurfb.2014.01.051
Guo, G., Zhou, H., Wang, Q., Wang, J., Tan, J., Li, J., et al. (2017). Nano-layered magnesium fluoride reservoirs on biomaterial surfaces strengthen polymorphonuclear leukocyte resistance to bacterial pathogens. Nanoscale 9, 875–892. doi:10.1039/C6NR07729C
Hayrapetyan, A., Surjandi, S., Lemsom, E. E. P. J., Wolters, M. M. M. W., Jansen, J. A., and van den Beucken, J. J. J. P. (2016). Coculture effects on the osteogenic differentiation of human mesenchymal stromal cells. Tissue Eng. Regen. Med. 13, 713–723. doi:10.1007/s13770-016-0008-z
Hou, W., Liu, Y., Wu, S., Zhang, H., Guo, B., Zhang, B., et al. (2019). Preadsorption of serum proteins regulates bacterial infections and subsequent macrophage phagocytosis on biomaterial surfaces. ACS Appl. Bio Mat. 2, 5957–5964. doi:10.1021/ACSABM.9B00890
Izquierdo-Barba, I., García-Martín, J. M., Álvarez, R., Palmero, A., Esteban, J., Pérez-Jorge, C., et al. (2015). Nanocolumnar coatings with selective behavior towards osteoblast and Staphylococcus aureus proliferation. Acta Biomater. 15, 20–28. doi:10.1016/J.ACTBIO.2014.12.023
Jia, Z., Wen, M., Xiong, P., Yan, J., Zhou, W., Cheng, Y., et al. (2021). Mussel bioinspired morphosynthesis of substrate anchored core–shell silver self-assemblies with multifunctionality for bioapplications. Mat. Sci. Eng. C 123, 112025. doi:10.1016/j.msec.2021.112025
Jia, Z., Xiu, P., Xiong, P., Zhou, W., Cheng, Y., Wei, S., et al. (2016). Additively manufactured macroporous titanium with silver-releasing micro-/nanoporous surface for multipurpose infection control and bone repair - a proof of concept. ACS Appl. Mat. Interfaces 8, 28495–28510. doi:10.1021/acsami.6b10473
Lee, J.-H., Wang, H., Kaplan, J. B., and Lee, W. Y. (2011). Microfluidic approach to create three-dimensional tissue models for biofilm-related infection of orthopaedic implants. Tissue Eng. Part C Methods 17, 39–48. doi:10.1089/ten.tec.2010.0285
Li, C., Ding, Y., Kuddannaya, S., Zhang, Y., and Yang, L. (2017). Anti-bacterial properties of collagen-coated glass and polydimethylsiloxane substrates. J. Mat. Sci. 52, 9963–9978. doi:10.1007/s10853-017-1175-6
Loi, F., Córdova, L. A., Pajarinen, J., Lin, T., Yao, Z., and Goodman, S. B. (2016). Inflammation, fracture and bone repair. Bone 86, 119–130. doi:10.1016/j.bone.2016.02.020
Marsell, R., and Einhorn, T. A. (2011). The biology of fracture healing. Injury 42, 551–555. doi:10.1016/j.injury.2011.03.031
Martinez, F. O. (2008). Macrophage activation and polarization. Front. Biosci. 13, 453. doi:10.2741/2692
Martínez-Pérez, M., Conde, A., Arenas, M.-A., Mahíllo-Fernandez, I., De-Damborenea, J.-J., Pérez-Tanoira, R., et al. (2019). The “Race for the Surface” experimentally studied: in vitro assessment of Staphylococcus spp. adhesion and preosteoblastic cells integration to doped Ti-6Al-4V alloys. Colloids Surfaces B Biointerfaces 173, 876–883. doi:10.1016/j.colsurfb.2018.10.076
Masters, E. A., Trombetta, R. P., de Mesy Bentley, K. L., Boyce, B. F., Gill, A. L., Gill, S. R., et al. (2019). Evolving concepts in bone infection: redefining “biofilm”, “acute vs. chronic osteomyelitis”, “the immune proteome” and “local antibiotic therapy.”. Bone Res. 7, 20. doi:10.1038/s41413-019-0061-z
Mendoza, G., Regiel-Futyra, A., Andreu, V., Sebastián, V., Kyzioł, A., Stochel, G., et al. (2017). Bactericidal effect of gold-chitosan nanocomposites in coculture models of pathogenic bacteria and human macrophages. ACS Appl. Mat. Interfaces 9, 17693–17701. doi:10.1021/acsami.6b15123
Mestres, G., Carter, S.-S. D., Hailer, N. P., and Diez-Escudero, A. (2021). A practical guide for evaluating the osteoimmunomodulatory properties of biomaterials. Acta Biomater. 130, 115–137. doi:10.1016/j.actbio.2021.05.038
Modaresifar, K., Azizian, S., Ganjian, M., Fratila-Apachitei, L. E., and Zadpoor, A. A. (2019). Bactericidal effects of nanopatterns: a systematic review. Acta Biomater. 83, 29–36. doi:10.1016/j.actbio.2018.09.059
Mohiti-Asli, M., Molina, C., Diteepeng, T., Pourdeyhimi, B., and Loboa, E. G. (2016). Evaluation of silver ion-releasing scaffolds in a 3D coculture system of MRSA and human adipose-derived stem cells for their potential use in treatment or prevention of osteomyelitis. Tissue Eng. Part A 22, 1258–1263. doi:10.1089/ten.tea.2016.0063
Mountziaris, P. M., Spicer, P. P., Kasper, F. K., and Mikos, A. G. (2011). Harnessing and modulating inflammation in strategies for bone regeneration. Tissue Eng. Part B Rev. 17, 393–402. doi:10.1089/ten.teb.2011.0182
O’Neill, J. (2014). Antimicrobial resistance: tackling a crisis for the health and wealth of nations. Available at: https://amr-review.org/sites/default/files/AMR Review Paper - Tackling a crisis for the health and wealth of nations_1.pdf (Accessed August 24, 2022).
Qiu, J., Qian, W., Zhang, J., Chen, D., Yeung, K. W. K., and Liu, X. (2019). Minocycline hydrochloride loaded graphene oxide enables enhanced osteogenic activity in the presence of Gram-positive bacteria, Staphylococcus aureus. J. Mat. Chem. B 7, 3590–3598. doi:10.1039/C9TB00405J
Reigada, I., Guarch-Pérez, C., Patel, J. Z., Riool, M., Savijoki, K., Yli-Kauhaluoma, J., et al. (2020). Combined effect of naturally-derived biofilm inhibitors and differentiated HL-60 cells in the prevention of Staphylococcus aureus biofilm formation. Microorganisms 8, 1757. doi:10.3390/microorganisms8111757
Ribeiro, M., Monteiro, F. J., and Ferraz, M. P. (2012). Infection of orthopedic implants with emphasis on bacterial adhesion process and techniques used in studying bacterial-material interactions. Biomatter 2, 176–194. doi:10.4161/biom.22905
Rivera, L. R., Cochis, A., Biser, S., Canciani, E., Ferraris, S., Rimondini, L., et al. (2021). Antibacterial, pro-angiogenic and pro-osteointegrative zein-bioactive glass/copper based coatings for implantable stainless steel aimed at bone healing. Bioact. Mat. 6, 1479–1490. doi:10.1016/j.bioactmat.2020.11.001
Saeed, K., McLaren, A. C., Schwarz, E. M., Antoci, V., Arnold, W. V., Chen, A. F., et al. (2019). 2018 international consensus meeting on musculoskeletal infection: summary from the biofilm workgroup and consensus on biofilm related musculoskeletal infections. J. Orthop. Res. 37, 1007–1017. doi:10.1002/jor.24229
Sakthivel, K., O’Brien, A., Kim, K., and Hoorfar, M. (2019). Microfluidic analysis of heterotypic cellular interactions: a review of techniques and applications. Trac. Trends Anal. Chem. 117, 166–185. doi:10.1016/j.trac.2019.03.026
Sánchez-Salcedo, S., García, A., González-Jiménez, A., and Vallet-Regí, M. (2023). Antibacterial effect of 3D printed mesoporous bioactive glass scaffolds doped with metallic silver nanoparticles. Acta Biomater. 155, 654–666. doi:10.1016/j.actbio.2022.10.045
Shen, X., Hu, W., Ping, L., Liu, C., Yao, L., Deng, Z., et al. (2020). Antibacterial and osteogenic functionalization of titanium with silicon/copper-doped high-energy shot peening-assisted micro-arc oxidation technique. Front. Bioeng. Biotechnol. 8, 573464. doi:10.3389/FBIOE.2020.573464
Shuai, C., Shi, X., Yang, F., Tian, H., and Feng, P. (2024). Oxygen vacancy boosting Fenton reaction in bone scaffold towards fighting bacterial infection. Int. J. Extrem. Manuf. 6, 015101. doi:10.1088/2631-7990/ad01fd
Svensson, S., Forsberg, M., Hulander, M., Vazirisani, F., Thomsen, P., Trobos, M., et al. (2014). Role of nanostructured gold surfaces on monocyte activation and Staphylococcus epidermidis biofilm formation. Int. J. Nanomedicine 9, 775–794. doi:10.2147/IJN.S51465
Takayanagi, H. (2007). Osteoimmunology: shared mechanisms and crosstalk between the immune and bone systems. Nat. Rev. Immunol. 7, 292–304. doi:10.1038/nri2062
Tan, J., Liu, Z., Wang, D., Zhang, X., Qian, S., and Liu, X. (2020). A facile and universal strategy to endow implant materials with antibacterial ability via alkalinity disturbing bacterial respiration. Biomater. Sci. 8, 1815–1829. doi:10.1039/C9BM01793C
Tao, B., Deng, Y., Song, L., Ma, W., Qian, Y., Lin, C., et al. (2019). BMP2-loaded titania nanotubes coating with pH-responsive multilayers for bacterial infections inhibition and osteogenic activity improvement. Colloids Surfaces B Biointerfaces 177, 242–252. doi:10.1016/j.colsurfb.2019.02.014
Trampuz, A., Ly, W. K., and Zimmerli, W. (2005). Prosthetic joint infections: update in diagnosis and treatment Phage Therapy in Orthopaedic Implant-Associated Infections View project Antiinfektiöse Strategien in der Unfallchirurgie Teil 1 und Teil 2 View project Prosthetic joint infections: update in diagnosis and treatment. Available at: https://www.researchgate.net/publication/7777205 (Accessed September 29, 2022).
Tran, H. A., Tran, M. H., and Tran, P. A. (2020). Antibiotic resistance of S. aureus on a ‘bifunctional’ surface: an in vitro coculture study. Mat. Lett. 280, 128542. doi:10.1016/j.matlet.2020.128542
Tran, H. A., and Tran, P. A. (2021). In situ coatings of silver nanoparticles for biofilm treatment in implant-retention surgeries: antimicrobial activities in monoculture and coculture. ACS Appl. Mat. Interfaces 13, 41435–41444. doi:10.1021/acsami.1c08239
Wagner, V. E., and Bryers, J. D. (2004). Poly(ethylene glycol)-polyacrylate copolymers modified to control adherent monocyte-macrophage physiology: interactions with attachingStaphylococcus epidermidis orPseudomonas aeruginosa bacteria. J. Biomed. Mat. Res. 69A, 79–90. doi:10.1002/jbm.a.20115
Wang, Y., Subbiahdoss, G., Swartjes, J., Van Der Mei, H. C., Busscher, H. J., and Libera, M. (2011). Length-scale mediated differential adhesion of mammalian cells and microbes. Adv. Funct. Mat. 21, 3916–3923. doi:10.1002/ADFM.201100659
World Health Organization (2012). The evolving threat of antimicrobial resistance Options for action. Available at: www.who.int/patientsafety/en/ (Accessed August 24, 2022).
Yang, Y., Zhang, H., Komasa, S., Morimoto, Y., Sekino, T., Kawazoe, T., et al. (2021). UV/ozone irradiation manipulates immune response for antibacterial activity and bone regeneration on titanium. Mat. Sci. Eng. C 129, 112377. doi:10.1016/j.msec.2021.112377
Yue, C., Kuijer, R., Kaper, H. J., van der Mei, H. C., and Busscher, H. J. (2014). Simultaneous interaction of bacteria and tissue cells with photocatalytically activated, anodized titanium surfaces. Biomaterials 35, 2580–2587. doi:10.1016/j.biomaterials.2013.12.036
Zaatreh, S., Haffner, D., Strauss, M., Dauben, T., Zamponi, C., Mittelmeier, W., et al. (2017). Thin magnesium layer confirmed as an antibacterial and biocompatible implant coating in a co-culture model. Mol. Med. Rep. 15, 1624–1630. doi:10.3892/mmr.2017.6218
Zaatreh, S., Wegner, K., Strauß, M., Pasold, J., Mittelmeier, W., Podbielski, A., et al. (2016). Co-culture of S. Epidermidis and human osteoblasts on implant surfaces: an advanced in vitro model for implant-associated infections. PLoS One 11, e0151534. doi:10.1371/journal.pone.0151534
Zimmerli, W., Waldvoge, F. A., Vaudaux, P., and Nydegger, D. E. (1982). Pathogenesis of foreign body infection: description and characteristics of an animal model. J. Infect. Dis. 146, 487–497. doi:10.1093/INFDIS/146.4.487
Zwicker, P., Schmidt, T., Hornschuh, M., Lode, H., Kramer, A., and Müller, G. (2022). In vitro response of THP-1 derived macrophages to antimicrobially effective PHMB-coated Ti6Al4V alloy implant material with and without contamination with S. epidermidis and P. aeruginosa. Biomater. Res. 26, 1. doi:10.1186/s40824-021-00247-1
Keywords: implant-associated infections, antibacterial biomaterials, in vitro co-culture models, osteogenic cells, immune cells
Citation: Eijkel BIM, Apachitei I, Fratila-Apachitei LE and Zadpoor AA (2024) In vitro co-culture models for the assessment of orthopedic antibacterial biomaterials. Front. Bioeng. Biotechnol. 12:1332771. doi: 10.3389/fbioe.2024.1332771
Received: 03 November 2023; Accepted: 15 January 2024;
Published: 05 February 2024.
Edited by:
Bin Li, Soochow University, ChinaReviewed by:
Jochen Salber, Ruhr-University Bochum, GermanyCijun Shuai, Jiangxi University of Science and Technology, China
Copyright © 2024 Eijkel, Apachitei, Fratila-Apachitei and Zadpoor. This is an open-access article distributed under the terms of the Creative Commons Attribution License (CC BY). The use, distribution or reproduction in other forums is permitted, provided the original author(s) and the copyright owner(s) are credited and that the original publication in this journal is cited, in accordance with accepted academic practice. No use, distribution or reproduction is permitted which does not comply with these terms.
*Correspondence: Benedictus I. M. Eijkel, b.i.m.eijkel@tudelft.nl; Lidy E. Fratila-Apachitei, e.l.fratila-apachitei@tudelft.nl