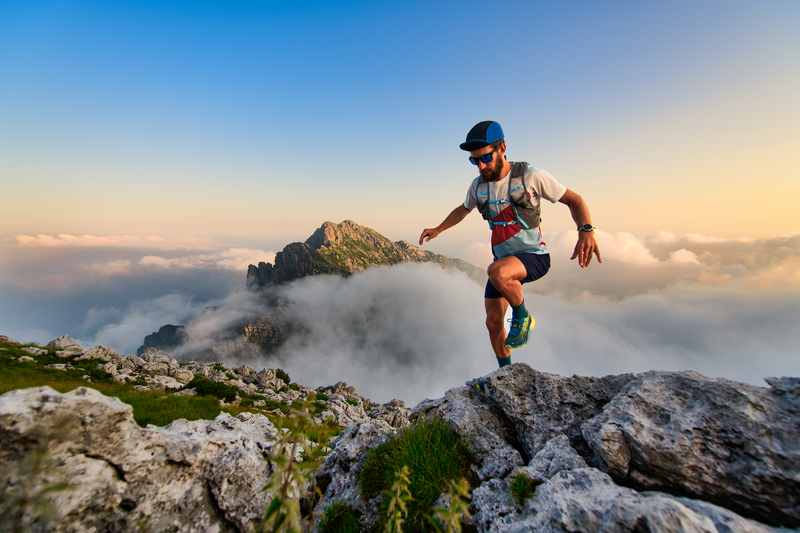
94% of researchers rate our articles as excellent or good
Learn more about the work of our research integrity team to safeguard the quality of each article we publish.
Find out more
REVIEW article
Front. Bioeng. Biotechnol. , 07 March 2024
Sec. Tissue Engineering and Regenerative Medicine
Volume 12 - 2024 | https://doi.org/10.3389/fbioe.2024.1329712
The failure of endogenous repair is the main feature of neurological diseases that cannot recover the damaged tissue and the resulting dysfunction. Currently, the range of treatment options for neurological diseases is limited, and the approved drugs are used to treat neurological diseases, but the therapeutic effect is still not ideal. In recent years, different studies have revealed that neural stem cells (NSCs) have made exciting achievements in the treatment of neurological diseases. NSCs have the potential of self-renewal and differentiation, which shows great foreground as the replacement therapy of endogenous cells in neurological diseases, which broadens a new way of cell therapy. The biological functions of NSCs in the repair of nerve injury include neuroprotection, promoting axonal regeneration and remyelination, secretion of neurotrophic factors, immune regulation, and improve the inflammatory microenvironment of nerve injury. All these reveal that NSCs play an important role in improving the progression of neurological diseases. Therefore, it is of great significance to better understand the functional role of NSCs in the treatment of neurological diseases. In view of this, we comprehensively discussed the application and value of NSCs in neurological diseases as well as the existing problems and challenges.
Neurological diseases, including Alzheimer’s disease (AD), Parkinson’s disease (PD), amyotrophic lateral sclerosis (ALS) and Huntington’s disease (HD), are highly crippled and fatal diseases that affect millions of people around the world (Heemels, 2016). The pathological mechanism of neurological diseases is mainly due to the progressive loss of neuronal structure or function, resulting in varying degrees of paralysis, cognitive, sensory and motor dysfunction, and even permanent disability (Heemels, 2016). Importantly, the ability of tissue repair and regeneration of neurological diseases is limited, and there is no radical cure that can stop or reverse their development. Clinical use of drugs to alleviate or control the symptoms of patients, but the effect of drug treatment is limited, still cannot achieve the desired effect. Importantly, drugs cannot repair damaged nerves and reconstruct neural networks. In addition, because the drug development process is complex and expensive, the clinical treatment of these diseases is limited. Therefore, there is an urgent need to explore effective treatment methods.
In recent years, with the continuous exploration of the repair of nerve injury, cell transplantation/cell therapy has entered people’s field of vision, has been widely used in neurological diseases, and broadened new treatment strategies. The therapeutic value of cell therapy in neurological diseases is to imitate the normal process of cell repair and development in the nervous system, so as to eliminate the causes of the disease, improve dysfunction and repair damaged tissue (Alessandrini et al., 2019; Andrzejewska et al., 2021). At present, researchers have achieved exciting results by transplanting different types of bioactive cells into nerve injury sites, such as spinal cord injury and sciatic nerve injury (Goulart et al., 2016; Ramalho et al., 2021). Neural stem cells are a kind of neuroblasts, which have the potential of self-renewal and differentiation, and can differentiate into neurons and glial cells (Merkle and Alvarez-Buylla, 2006). NSCs play an important role in the development of central nervous system. The functions of NSCs in the treatment of neurological diseases include promoting nerve regeneration, cell differentiation, secreting neurotrophic factors, neuroprotection and immune regulation, and producing pharmacological properties of repairing tissue damage and improving function (Dong and Yi, 2010; de Freria et al., 2021; Zhang et al., 2021). Importantly, NSCs therapy can replace endogenous deletion cells to reconstruct the function of neural network (Bellenchi et al., 2013). NSCs transplantation can replace injured or missing neurons by differentiating into neurons at the site of nerve injury, producing protective neurons and maintaining the number of neurons, which provides another attractive treatment strategy for the treatment of neurological diseases. Currently, different studies have confirmed the therapeutic effect and application value of NSCs in neurological diseases, and great progress has been made (Nizzardo et al., 2014; Ager et al., 2015; Lee et al., 2015; Mendes-Pinheiro et al., 2018). It reveals the fact that NSCs can be used in the treatment of neurological diseases. Therefore, here, we deeply discuss the application value of NSCs in the treatment of neurological diseases and some problems that need to be solved at present.
The journey of neural development begins with neuroepithelial cells, a kind of pluripotent neural stem cells, pseudo-layered cells arranged in the neural tube chamber. Neuroepithelial cells begin to divide symmetrically and maintain stem cell characteristics (Haubensak et al., 2004). After the formation of neural tube, neuroepithelial cells are transformed into radial glial cells and produce offspring and intermediate neural progenitor cells by asymmetric division, which differentiate into neurons and induce neuronal migration (Noctor et al., 2004; Gleason et al., 2008). With the development of embryo, radial glial cells can differentiate into oligodendrocytes and astrocytes (Okamoto et al., 2013). Near birth, radial glial cells change their characteristics to produce neural stem cells, which are libraries of neurogenesis and embryogenesis in adult life (Alvarez-Buylla et al., 2001; Fujita, 2003).
NSCs from adult mammals have unique characteristics, such as differentiation ability and self-renewal. They exist in specific gaps, namely, the subventricular zone (SVZ), located in the ependymal cell layer, separating the ependymal and subventricular zones, and the subgranular area (SGZ)-the dentate gyrus of the hippocampus (Gonzalez et al., 2016; Grochowski et al., 2018). The limited regeneration ability of the nervous system may be due to the limited number of NSCs in discrete locations, or surrounded by microenvironments that do not support neuronal differentiation, resulting in endogenous repair failure (Gonzalez et al., 2016). As a kind of self-renewing pluripotent cells, NSCs can produce various types of cells needed to establish the central nervous system (Kageyama et al., 2006). NSCs produce the plasticity of new neurons and glial cells through multiple processes and play an important role in embryonic development and adult neurogenesis. In recent years, NSCs transplantation has gained extensive recognition and application value in the application of tissue injury repair and nerve regeneration. Transplantation of NSCs into the injured spinal cord can increase the possibility of regeneration by replacing lost neurons or oligodendrocytes (Ishii et al., 2006; Xue et al., 2020).
In addition to the differentiation and self-renewal characteristics of NSCs, NSCs play a variety of functional characteristics in neural injury repair and regeneration, including immune regulation (the interaction between immune cells and NSCs/neural progenitor cells and their offspring seems to determine the effectiveness of endogenous regeneration response. It also determines the fate and functional integration of transplanted NSCs (Kokaia et al., 2012), secretion of neurotrophic factors (such as GDNF and BDNF) (Fu et al., 2011), anti-inflammatory regulation (Park et al., 2021), angiogenesis (Moshayedi and Carmichael, 2013), axon regeneration and myelin formation (Moshayedi and Carmichael, 2013; Chung et al., 2022) and inhibition of glial scar formation (Ishii et al., 2006) (Table 1). Moreover, in peripheral neurological diseases, implanted NSCs can differentiate into Schwann cells (SCs) and maintain a microenvironment rich in growth factors, thus promoting nerve regeneration (Lee et al., 2017). Studies have shown that IL12p80 can induce mouse NSCs to differentiate into SCs through STAT3 phosphorylation and promote the functional recovery of regenerated nerves after sciatic nerve injury (Lee et al., 2017). In view of these characteristics, the application value of NSCs in neurological diseases has a broad prospect.
NSCs mainly come from endogenous NSCs (human embryonic stem cells and human fetal brain NSCs/progenitor cells), induced pluripotent stem cells (iPSCs) and direct reprogramming (Zhang and Jiao, 2015; Grochowski et al., 2018). These provide a strong basis for the origin of NSCs and their development in the field of tissue repair and regeneration. During embryonic development, there are two key proliferative regions: the ventricular zone (VZ) and the subventricular zone (SVZ). NSCs are located in the VZ of the neural tube and produce various types of cells needed for the construction of the central nervous system (Pevny and Rao, 2003; Kageyama et al., 2006). NSCs in VZ divide symmetrically and asymmetrically to preserve stem cell banks, which then migrate to SVZ and then perform the ability to proliferate or differentiate (Guillemot, 2005). SVZ may be a special region. SVZ in the adult brain is the site of significant proliferation of neuronal cells in the early stage of development, which contains static NSCs. These endogenous resting NSCs are activated and participate in the repair process after central nervous system injury (Ito and Suda, 2014). Another major area of NSCs in the adult brain is the subgranular region of the dentate gyrus (Kriegstein and Alvarez-Buylla, 2009; Feliciano et al., 2015). NSCs can be obtained from adult subventricular zone or subgranular zone. NSCs can continuously produce and differentiate into functional neurons in the course of life, which have important functions on human body, including learning, memory and cognition (Gage and Temple, 2013).
In order to isolate, culture and obtain high yield NSCs from the subventricular zone or subgranular zone, the NSCs isolated from the subventricular zone or subgranular zone were cultured into neurospheres or monolayers, and then identified by Nestin, Sox2 or Msi1 labeling (Zhang and Jiao, 2015). The spheroids of NSCs can be well cultured and better characterized by the addition of epidermal growth factor, fibroblast growth factor 2 and B27 to the culture system in vitro (Gage and Temple, 2013; Zhang et al., 2021). After the NSCs isolated from the lateral ventricle of adult mice were cultured in a specific medium with growth factors, the number of neurospheres increased significantly (Stipcevic et al., 2013). Intracerebroventricular injection of 300,120 ng/d di-rhamnolipid could increase the number of nerve balls in the anterior lateral ventricle of adult mice (Stipcevic et al., 2013). These studies have revealed that NSCs can be obtained from endogenous, expanded or established into stable cell lines in vitro, and then transplanted to the injured site for regeneration treatment.
Direct cell reprogramming is based on the dominant role of cell pedigree transcription factors in transforming adult somatic cells into different cell types. This technique represents a promising approach in the field of regenerative medicine, with the potential to produce cell sources for cell replacement therapy (Graf and Enver, 2009; Chambers and Studer, 2011). Direct cell reprogramming can use the least set of cell line-specific transcription factors to directly transform fibroblasts into NSCs and differentiate into functional neurons and oligodendrocytes (Ring et al., 2012; Caiazzo et al., 2015). This method is fast and simple, and it takes only one step to generate the required NSCs. Adult primary fibroblasts can effectively directly transform into neural progenitor cells by timely limiting the expression of Oct4, Sox2, Klf4 and c-Myc, and show the morphological and molecular characteristics of NSCs/neural progenitor cells, such as Nestin and Sox2 (Meyer et al., 2015). Reprogrammed NSCs/neural progenitor cells can differentiate into neurons and astrocytes [Meyer S]. Studies have shown that mouse and human fibroblasts can be directly reprogrammed into NSCs without intermediate pluripotency (Kim et al., 2017). Human umbilical cord blood cells were directly reprogrammed into NSCs using pedigree-specific transcription factors SOX2 and HMGA2 with high efficiency and short turnaround time. The reprogrammed NSCs can be cloned and expanded and have the multipotential to produce neurons and glial cells (Zhang et al., 2022). Direct reprogrammed NSCs do not go through the multi-functional phase and are a safe and timely resource. This is an important progress in the field of cell transplantation therapy, and it also means that direct reprogramming of somatic cells provides a strong guarantee for NSCs for the treatment of nervous system diseases.
Induced pluripotent stem cells (iPSCs) have made an inestimable contribution to the field of regenerative medicine, bypassing ethical issues in the use of human embryos (Kim et al., 2017; Aboul-Soud et al., 2021). iPSCs have the plasticity of generating, repairing and altering nervous system function. Combined with reprogramming techniques, neural stem cells derived from human somatic cells and their offspring can more accurately simulate neurological diseases (Gage and Temple, 2013; Aboul-Soud et al., 2021). iPSCs can create specific populations of neurons, especially for neurodevelopment and the treatment of neurological diseases. iPSCs were induced from mouse embryonic or adult fibroblasts. These cells showed the morphological and growth characteristics of embryonic stem cells and expressed the characteristics of embryonic stem cell marker genes (Takahashi and Yamanaka, 2006). iPSCs were subcutaneously transplanted into nude mice to produce tumors containing various tissues of all three reproductive layers (Takahashi and Yamanaka, 2006). After further injection of these cells into blastocysts, the induction of pluripotent stem cells is helpful to the embryonic development of mice (Takahashi and Yamanaka, 2006). Studies have shown that iPSCs can produce powerful and cost-effective NSCs/early neural progenitor cells, which can differentiate into vesicular glutamate transporter 1 (VGLUT1) positive neurons (DAiuto et al., 2014). Human iPSCs-induced NSCs showed stable neural phenotypes. When these NSCs were transplanted into the model of ischemic stroke, it was found that these cells could survive and migrate to the ischemic area, and could differentiate into mature nerve cells, which was beneficial to the functional recovery (Liu, 2013). Moreover, the iPSCs derived NSCs/neural progenitor cells have low immunogenicity and can increase the survival rate after cell transplantation (Itakura et al., 2017). One of the advantages of iPSCs is that it removes ethical issues and restrictions on the use of embryonic stem cells. However, there are also some problems in iPSCs, such as genetic/epigenetic abnormalities and tumorigenesis caused by artificial induction, the progression of pluripotent state and subsequent complete differentiation to the desired lineage are still obstacles to the clinical translation of iPSCs technology, which must be solved before clinical use.
Neurological diseases include PD, AD, HD and ALS. These diseases are characterized by the loss of specific neuronal subsets, resulting in gradual loss and paralysis of sensory, cognitive and motor neurons (Fayazi et al., 2021; Liao et al., 2022). This kind of disease affects tens of millions of people around the world. with the aging of the world population, the incidence of this kind of disease continues to rise, bringing huge mental, physical and economic burden to patients (Marsh and Blurton-Jones, 2017). Despite the increasing understanding of the pathogenesis of these diseases, there is still a lack of effective and feasible treatments. Some drugs are used clinically to slow down the progression of these diseases, but the long-term use of drugs brings dependence, side effects and short-term benefits, so it is impossible to replace and recover the lost neurons and reconstruct the neural functional network. Therefore, with the continuous research and exploration of researchers, the strategy of cell therapy is introduced. People transplant functional active cells into the site of nerve injury to play a therapeutic role. Cell therapy treats neurological diseases by generating specific neurons to maintain and replace lost host neurons, establishing auxiliary connection neural networks around the affected areas, and creating a new environment to support the survival of host neurons (Sugaya and Vaidya, 2018). As a special kind of stem cells, NSCs have been widely studied in the field of nerve regeneration, which play a useful role through different mechanisms, such as the production of neurotrophic factors, reduction of neuroinflammation, neuroprotection and immune regulation (Grochowski et al., 2018; De Gioia et al., 2020; Zhang et al., 2020). In addition, NSCs must properly migrate to the injured nervous system and integrate into the host neuron circuit to reconstruct the neural network lost in the disease and enhance neural plasticity (Marsh and Blurton-Jones, 2017; De Gioia et al., 2020). Although the application of NSCs in neurological diseases is in the initial stage of exploration, it is a feasible new strategy for treatment (Table 2).
Parkinson’s disease (PD) is a common neurodegenerative disease characterized by low expression of dopamine in the striatum and degeneration of dopaminergic neurons in the substantia nigra pars compacta, leading to various motor and non-motor symptoms (Palm et al., 2012; Zhang et al., 2017). Inhibition or replacement of denatured neurons has always been considered to be an effective and standardized treatment (Palm et al., 2012). Although a variety of treatments are currently used to treat PD, such as levodopa therapy, deep brain stimulation surgery and rehabilitation, these treatments have been identified as current treatment strategies (Yasuhara et al., 2015; Yasuhara et al., 2017). Although these methods have certain therapeutic effects, they cannot prevent functional defects and disorders caused by dopamine depletion. Fortunately, cell therapy may be the key to the real recovery of degenerative/degenerative neurons. The cell therapy of PD began in 1979. The motor abnormality of PD rats was improved by transplanting dopamine-containing neurons from fetal rats. It was found that the transplanted cells survived and the axons grew well (Yasuhara et al., 2015). With the continuous study of cell therapy, researchers have transplanted different types of bioactive cells, such as olfactory sheath cells and NSCs, into the brain to produce therapeutic effects (Agrawal et al., 2004; Heris et al., 2022).
The number of neural progenitor cells/NSCs in the subependymal area, subgranular area and olfactory bulb of PD patients has been decreased after death, suggesting that the production of neural precursor cells/NSCs in patients with PD is impaired, which is the result of dopaminergic nerve loss (Höglinger et al., 2004; OSullivan et al., 2011). This suggests a close relationship between NSCs/neural progenitor cells and PD. Therefore, NSCs transplantation may be a new neural function recovery strategy for the treatment of PD. NSCs transplantation can be integrated into the surrounding environment of the central nervous system to establish an appropriate microenvironment to enhance dopaminergic nerve transmission (Chou et al., 2015). NSCs can differentiate into dopaminergic by recognizing the cellular and acellular regions of the target in the microenvironment. With the integration of differentiated dopaminergic neurons into the existing nigrostriatal dopaminergic pathway, the symptoms of PD can be alleviated (Chou et al., 2015). The NSCs/neural progenitor cells isolated from mesencephalic organs expanded stably and differentiated into mesencephalic dopamine neurons, showing better synaptic maturity and function. Further transplantation of NSCs/neural progenitor cells into the animal model of PD produced reproducible and effective therapeutic results (Kim et al., 2021). In vitro analysis showed that human NSCs line hVM1 clone 32 cells expressed standard dopamine-centered markers and other markers related to mitochondrial and peroxisome function and glucose and fat metabolism. NSCs were transplanted into PD mice to express dopamine-centered markers, the number of astrocytes and microglia increased significantly, and the weight of non-phosphorylated neurofilaments in motor cortex increased, which improved hyperactivity and gait changes in mice (Nelke et al., 2022). It is suggested that human NSCs transplantation has the function of preventing sports and non-sports injury. Moreover, in recent years, it has been found that the secretory group produced by NSCs plays a protective role in PD neurons. Injection of conditioned medium of NSCs into striatum and substantia nigra can improve motor and non-motor dysfunction in PD rats and save the loss of dopaminergic neurons (Ni et al., 2022). Conditioned medium of NSCs can reduce the apoptosis of PC12 cells induced by 6-OHDA, reduce the level of oxidative stress and improve the dysfunction of mitochondria (Ni et al., 2022). This may be related to the protective effect of conditioned medium of NSCs on 6-OHDA injured neurons through Sirt1 pathway.
Based on the role of NSCs in the treatment of PD. In order to optimize the survival of transplanted NSCs and guide their proper differentiation, researchers have combined different types of cells (such as endothelial cells and microglia) (Deng et al., 2013; Qian et al., 2020) and other factors (such as neurotrophin 3 (Gu et al., 2009)) with NSCs in the treatment of PD and achieved exciting results. Studies have shown that the combined transplantation of mesenchymal stem cells (MSCs) conditioned medium and NSCs can differentiate into dopaminergic neurons in the ventral tegmental area and medial forebrain bundle, migrate around the injured site, significantly reduce apomorphine-induced rotational asymmetry and improve spatial learning ability (Yao et al., 2016). Interestingly, combined transplantation showed higher activity in terms of cell survival, migration and behavior improvement than single NSCs transplantation (Yao et al., 2016). Other studies have shown that NSCs combined with ethyl stearate can promote the migration of NSCs from striatum to substantia nigra through CCL5/CCR5, and promote the differentiation of NSCs into dopaminergic neurons, thus improving the motor behavior of PD rats (Huang et al., 2023).
Although these studies reveal the potential value of NSCs in the treatment and application of PD, NSCs transplantation is still challenged. In the heterotopic even if the NSCs are transplanted into the primitive sites of PD, such as the substantia nigra and striatum, the new dopaminergic neurons are difficult to regenerate the axons of the striatum and are unlikely to integrate into the normal brain circuit, which leads to the main defects and obstacles of the current therapeutic effect. Another challenge is that NSCs therapy is how to target to promote the differentiation of transplanted NSCs into reliable and renewable dopamine neurons and to provide rich and effective cell substitutions for the treatment of PD.
Alzheimer’s disease (AD) can be described as a serious social, economic and medical crisis of our time. The core of the pathogenesis of AD lies in the following aspects. Tau is a microtubule-associated protein in neurons, which is very important for structural support and axonal transport. It is overphosphorylated, causing microtubules to collapse and gather into nerve fiber tangles. Secondly, β-amyloid (A-β) fragments accumulate and accumulate outside the cell. While activated microglia are closely related to β-amyloid aggregation and amyloid plaque formation. Thirdly, the loss of a large number of neurons and synapses is the most closely related factor of early cognitive decline in AD (Delbeuck et al., 2003; Salloway et al., 2014; Duncan and Valenzuela, 2017; Fang et al., 2019). AD is characterized by progressive neurodegeneration, mainly neuronal degeneration/degeneration and synaptic loss. Therefore, reversing and improving neuronal degeneration and axon loss are very important for the treatment of AD. With the emergence of cell therapy, it can not only prevent the development of the disease, but also reverse the symptoms, which has made some achievements in the application of AD.
The pathological changes of amyloid precursor protein in the progression of AD may prevent the neuronal differentiation of NSCs, while reducing the amyloid precursor protein can promote the neuronal differentiation of NSCs and migrate and differentiate into neurons in the hippocampus and cortex (Marutle et al., 2007). It also means that NSCs play an important role in AD process. NSCs transplantation plays an important therapeutic role in the application of AD by differentiating neurons, replacing and improving lost neurons. NSCs can enhance neuronal connectivity and metabolic activity in AD through compensation mechanism, thus saving the cognition of AD (Li et al., 2016). The neurospheres derived from mouse embryonic stem cells were transplanted into the frontal cortex of C57BL/6 mice. The results showed that the transplanted neurospheres survived and produced many ChAT positive neurons and a small number of serotonin positive neurons in and around the grafts (Wang et al., 2006). The working memory errors of mice were significantly reduced (Wang et al., 2006). This study revealed that the neurospheres derived from mouse embryonic stem cells significantly alleviated cholinergic deficit and short-term memory impairment in AD mice. NSCs are produced by growth factor-mediated selection in the absence of serum, and the induced NSCs can differentiate into cholinergic neurons (Moghadam et al., 2009). After transplantation of neural progenitor cells, the memory function of rats was significantly improved. Immunohistochemical analysis showed that most (about 70%) neural progenitor cells and/or primary neural progenitor cells retained neuronal phenotype after transplantation, of which about 40% had cholinergic phenotype and no tumor formation (Moghadam et al., 2009). It is suggested that NSCs transplantation is safe and effective. NSCs obtained from the hippocampus of rats were transplanted into the basal forebrain of AD rats. It was found that these cells could differentiate into neurons and astrocytes, and the number of cholinergic neurons increased, which improved the symptoms of AD rats (Xuan et al., 2008). It is interesting that further intracerebroventricular injection of brain-derived neurotrophic factor (BDNF) on the basis of NSCs transplantation can improve the differentiation of NSCs, improve the symptoms of AD rats, and improve the therapeutic effect of NSCs (Xuan et al., 2008). Further study also found that the transplanted NSCs from the hippocampus could differentiate into cholinergic neurons. NSCs transplantation could significantly increase the number of cholinergic neurons in the medial septal nucleus and vertical branch of diagonal branch, which improved the learning and memory ability of rats (Xuan et al., 2009).
NSCs transplantation can also inhibit neuroinflammation, cholinergic dysfunction, pericyte and synaptic loss, promote adult hippocampal neurogenesis and improve the cognitive impairment of AD (Lu et al., 2021). Human NSCs were repeatedly intranasally injected into the brain of APP/PS1 transgenic AD mice. The results showed that human NSCs transplanted into nasal cavity survived and showed extensive migration and high neuronal differentiation, while glial differentiation was relatively limited (Lu et al., 2021). NSCs transplantation can differentiate into cholinergic neurons, thus saving cholinergic dysfunction in APP/PS1 mice (Lu et al., 2021). In addition, nasal transplantation of human NSCs can improve other pathological changes similar to AD, including neuroinflammation, cholinergic dysfunction, pericyte and synaptic loss, promote adult hippocampal neurogenesis, and ultimately save the cognitive impairment of AD mice (Lu et al., 2021). The mechanism of NSCs transplantation to improve the symptoms of AD may reduce the accumulation of β-amyloid protein by up-regulating the expression of β-amyloid protease, insulin-degrading enzyme and Neprilysin. Transplantation of NSCs and bone marrow MSCs reduced the number of β-amyloid plaques in the hippocampus of AD mice and increased the polarization of M2 microglia, suggesting that stem cell transplantation has anti-inflammatory effects (Campos et al., 2022). Other studies have shown that NSCs transplantation reverses the overactivity in the hippocampus of AD mice, increases the number of microglia, and improves the learning and memory ability of mice (Campos et al., 2022). These studies highlight the potential benefits of NSCs in the treatment of AD.
Amyotrophic lateral sclerosis (ALS) is a fatal disease characterized by persistent degeneration of motor neurons, which is characterized by paralysis and death by affecting motor neurons in the motor cortex, brainstem and spinal cord (Mazzini et al., 2016; Meyer, 2021). At present, some studies have proposed several pathological mechanisms of ALS-induced motoneuron death, including glutamate-induced excitotoxicity, abnormal cytoskeleton, protein aggregation, oxidative stress, mitochondrial dysfunction and extracellular SOD1 toxicity (Peters et al., 2015; Shafiq et al., 2021). There is no effective treatment for this devastating disease. With the exploration of the treatment of ALS, researchers have transplanted different types of functional cells to replace lost and degenerative neurons, resulting in therapeutic effects. In view of the unique characteristics of NSCs, which can differentiate into neurons, exciting results have been obtained in the therapeutic application of ALS (Kim et al., 2013; Baloh et al., 2018). NSCs transplanted into ALS animals can be preferentially colonized in motor cortex, hippocampus and spinal cord. Their differentiation is characterized by decreased expression of nestin, positive cells of MAP2, GFAP, O4 and CD68, and increased differentiation and survival of NSCs related to tumor necrosis factor (Mitrecić et al., 2010).
NSCs transplantation can slow down the onset and progression of ALS symptoms and prolong survival time. This beneficial effect seems to be achieved by producing nutritional factors, protecting neuromuscular function, reducing astrocyte proliferation, improving inflammation and enhancing synaptic plasticity (Teng et al., 2012). The in vitro characteristic of iPSCs-derived neural precursor cells is their ability to differentiate into neuronal phenotype. iPSCs-derived neural precursor cells transplantation normalized the expression of host genes (versican, has-1, tenascin-R, NGF, IGF-1, BDNF, bax, bcl2, and Casp-3), significantly preserved motor neurons, protected neural networks around motor neurons, slowed disease progression, and prolonged survival in all treated animals (Forostyak et al., 2020). The transplantation of human NSCs into the lumbar spinal cord of ALS rats can protect the α motoneurons near the transplanted cells and provide a transient functional improvement, but has no protective effect on the α motoneurons far away from the lumbar graft (Hefferan et al., 2012). Further studies showed that human NSCs was transplanted into the spinal cord and found that NSCs migrated and integrated widely in the spinal cord and differentiated into neurophenotypes, which delayed the loss of body weight and the deterioration of motor ability in ALS rats (Zalfa et al., 2019). NSCs transplantation reduced the number of activated astrocytes and microglia and increased the overall survival of rats. No rejection related to NSCs transplantation was observed (Zalfa et al., 2019). These studies have revealed the potential therapeutic effect of NSCs transplantation in the treatment of ALS, and it is a safe and feasible treatment.
However, people soon realized that this was an extremely difficult task, because newborn and differentiated motor neurons must project to distant axons, replace lost neurons and connect with host neurons, and reconstruct neural network function. Moreover, the toxic microenvironment formed in the process of ALS, including inflammatory attacks, has toxic effects on transplanted cells, resulting in reduced cell survival, which is also a difficult problem to be solved at present. Furthermore, how to control and enhance the targeted differentiation of transplanted NSCs into motor neuron phenotype, enhance synaptic plasticity and reduce their differentiation to other cell phenotypes is also a key issue in the treatment of ALS.
Huntington’s disease (HD) is a neurodegenerative disease caused by the CAG amplification of the Huntington protein (HTT) gene. The disease is characterized by neuronal degeneration in the striatum and cortex, leading to functional degeneration and disorders, including cognitive impairment and motor disorders (Bashir, 2019; Kim et al., 2021). With the development of the disease, the loss of neurons can affect many areas of the brain more widely (Kim et al., 2021). Since the discovery of HTT gene, no great progress has been made in the development of therapeutic strategies to prevent or slow down the progress of HD, and there is no effective treatment (Connor, 2018). With the biological mining of stem cells and their application in neurodegenerative diseases, some new and exciting results have emerged. With the biological mining of NSCs and their application in neurodegenerative diseases, some new and exciting results have emerged. The unique characteristics of NSCs provide the groundwork for the application and treatment of HD. The basis of NSCs therapy for HD is to replace or prevent cell loss. With the biological mining of NSCs and their application in neurological disease, some new and exciting results have emerged. The unique characteristics of NSCs provide the groundwork for the application and treatment of HD.
The role of NSCs transplantation in HD therapy is mainly through differentiation into neuronal phenotype, enhancement of neuronal plasticity and cell substitution (Li et al., 2020; Latoszek and Czeredys, 2021; Berlet et al., 2022). NSCs need to differentiate into gamma-aminobutyric acid (GABA) neuronal subtype before transplantation in the treatment of HD (Bosch et al., 2004). A population of homogenous functional GABA neurons was generated from neural stem cell lines. These neurons survive in HD and maintain their acquired fate (Bosch et al., 2004). In the model of HD, GABA neurons differentiated from NSCs can survive for a long time and make synaptic contact with endogenous neurons when transplanted into the striatum (Bosch et al., 2004). This reveals the possibility of using NSCs for cell replacement therapy for HD. Transplantation of NSCs derived from human embryonic stem cells into the striatum of HD model mice improved motor and cognitive impairment, saved synaptic changes, and connected with nerve endings of cells in the host (Reidling et al., 2018). Moreover, the implanted hNSCs had electrophysiological activity. HNSCs improved the motor and late cognitive impairment of HD model mice by reducing the abnormal accumulation of mutant HTT protein and the expression of brain-derived neurotrophic factor (BDNF) (Reidling et al., 2018). NSCs may reverse the progression of the disease by reducing the abnormal accumulation of mutant HTT protein and increasing the expression of brain-derived neurotrophic factor (BDNF) (Yoon et al., 2020). The immortalized NSCs could differentiate and express BDNF medium spiny neurons and GABA neurons in the striatum of HD animals, while the NSCs transplanted into the cortex formed Tbr1 positive neurons (Yoon et al., 2020). These transplanted NSCs can be stably implanted and connected in the host brain tissue, reducing the formation of glial scar and inflammation, and increasing endogenous neurogenesis and angiogenesis (Yoon et al., 2020). This study suggests that a clinical grade NSCs line is effective in preclinical trials of HD. These studies have revealed the safety and effectiveness of NSCs transplantation in HD.
Autologous or allogeneic NSCs are limited and ethically limited, while iPSCs to derive/differentiate NSCs not only provides a rich cellular guarantee in source, but also suppresses ethical problems. Indeed, NSCs derived from iPSCs have also made some achievements in HD therapy (Liu et al., 2017; Cho et al., 2019). iPSCs were induced to differentiate into NSCs in vitro. After transplantation into HD mice, it was found that these cells could survive in vivo and differentiate into region-specific neurons (moderately prickly neurons), which improved the motor dysfunction of mice (Al-Gharaibeh et al., 2017). Other studies have also found that neural progenitor cells derived from human leukocyte antigen homozygote-iPSCs are transplanted into the striatum of HD mice. It is found that the transplanted cells have multiple differentiation potential (differentiation into neurons, oligodendrocytes and astrocytes) (Park et al., 2021). These cells restored the expression of DARPP-32, the density of synaptophysin, the expression of myelin basic protein in corpus callosum and the function of astrocytes, and played a role in neuroprotection and functional recovery (Park et al., 2021). This suggests that NSCs derived from iPSCs may provide a promising therapeutic strategy for neuronal replacement of HD.
Although encouraging achievements have been made in the application of NSCs in HD therapy, the wide application and development of cell replacement therapy is limited due to some inherent problems of cells (such as genetic instability and safety of NSCs derived from iPSCs and autologous cell source) and the technical differences in the use of NSCs in laboratory. Nevertheless, NSCs are a new cell substitution strategy and have a wide range of development and application value in the application of HD therapy.
The process of regeneration and repair of PNI is a dynamic process regulated by multi-cell and multi-molecule, involving neurons, SCs (repair phenotype) and immune cells (macrophage activation and phagocytosis). The interaction between these cells regulates the process of peripheral nerve regeneration and repair (Chen et al., 2015; Modrak et al., 2020; Nocera and Jacob, 2020). In this process, neuronal degeneration, axonal atrophy and demyelination are the key factors leading to the obstruction of PNI regeneration, and other factors include inflammatory reaction, glial scar formation and immunity, which can hinder peripheral nerve regeneration and repair (Modrak et al., 2020; Zhang et al., 2023). It is understood that the inherent ability of PNI regeneration is limited, lack of regeneration basis and environment. Therefore, it is very important to improve and provide the microenvironment for PNI regeneration and promote axonal regeneration and myelination. NSCs have shown their ability to promote nerve regeneration and repair tissue injury in most studies. The mechanisms of NSCs promoting nerve regeneration include neurotrophic factors secreted by NSCs (such as BDNF, NGF, neurotrophin 3/4 and vascular growth factor), anti-inflammatory and immunomodulatory, axonal regeneration and remyelination, neuroprotection and improving the local microenvironment of nerve injury, which are beneficial to the regeneration and repair of PNI (Sullivan et al., 2016; Assinck et al., 2017; Pisciotta et al., 2020; Zhang et al., 2020).
NSCs transplantation can significantly improve the formation of axonal myelin in sciatic nerve injury and regeneration, and increase the expression of hepatocyte growth factor (HGF) and nerve growth factor (NGF) (Cheng et al., 2011; Xu et al., 2012). Extraction, isolation and identification of functional NSCs from human iPSCs injected into denervated gastrocnemius muscle enhanced neuromuscular regeneration after denervation (Li et al., 2022).
The dry weight and muscle fiber area of gastrocnemius muscle increased significantly at 3, 5 and 7 months after NSCs transplantation. 7 months after embryonic NSCs transplantation, neurons can still be seen in the distal tibial nerve (Gu et al., 2010). It is suggested that embryonic NSCs can differentiate into neurons and form functional neuromuscular connections with denervated muscles, which may be beneficial to the treatment of muscle atrophy after peripheral nerve injury (Gu et al., 2010). All these show that NSCs have obvious advantages and therapeutic potential in promoting nerve regeneration after PNI. Interestingly, different passages of NSCs are different in the treatment of PNI. Human NSCs (fifth or sixth generation) were transplanted into 15 mm rat sciatic nerve injury model to compare the effects of two passage cell lines in the treatment of nerve injury. Compared with the fifth generation, the survival rate, SCs differentiation, axonal growth and functional results of the sixth generation of transplanted cells decreased (Du et al., 2018). The therapeutic effect of the sixth generation of NSCs on peripheral nerve regeneration was significantly lower than that of the fifth generation (Du et al., 2018). This study means that there are differences in PNI regeneration and repair among different passages of NSCs, which may be related to the decrease of cell viability and biological activity caused by long passage time of cell culture.
In view of the functional role of NSCs in the repair of PNI. Some studies explore the efficacy of NSCs in the treatment of PNI by combining other cells (such as olfactory ensheathing cells) or other factors/substances (such as overexpression of glial-derived neurotrophic factor, catheter/scaffold and matrix). These combined transplantation can enhance the therapeutic effect of NSCs and exert the characteristics of synergistic treatment (Shi et al., 2009; Syu et al., 2019; Zhang et al., 2019). In vitro, Estradiol promotes the migration and proliferation of NSC and inhibits apoptosis (Sekiguchi et al., 2013). The combined application of Estradiol and NSCs transplantation can promote the recovery of injured peripheral nerve function, increase the amount of vascular and blood perfusion in the nerve, and accelerate the motor nerve conduction velocity, voltage amplitude and exercise tolerance (Sekiguchi et al., 2013). Laminin-chitosan-PLGA nerve conduit containing SCs and NSCs can promote the regeneration of recurrent laryngeal nerve injury, and the effect is better than that of autogenous nerve transplantation (Li et al., 2018; Li et al., 2020). The diameter and area of regenerated myelin sheath of recurrent laryngeal nerve and the secretion of brain-derived neurotrophic factor and glial cell line-derived neurotrophic factor in laryngeal muscle or regenerated nerve tissue were significantly increased after transplantation of laminin-chitosan-PLGA nerve conduit containing Schwann cells and NSCs (Li et al., 2020). Co-culture of Schwann cells and NSCs in laminin-chitosan-PLGA catheter may promote the regeneration of injured nerves (Li et al., 2020). This method may be a promising alternative to repair defective nerves. Co-inoculation of NSCs and SCs into PLGA catheter carriers carrying NT-3 promoted NSCs to differentiate into neurons, enhanced synaptic connections and synaptic activity, and accompanied by SCs myelinated neurite processes (Xiong et al., 2012). This may be due to the fact that the catheter/stent can support and guide the growth of regenerated axons from one nerve stump to another, while preventing the growth of fibrous tissue and retaining neurotrophic factor components.
It is understood that SCs constitute the myelin cells of the peripheral nerve and play an important role in protecting neuronal axons and vegetative neurons. SCs play an irreplaceable role in the regeneration and repair of peripheral nerve. In addition, some researchers can promote axonal regeneration and nerve repair by transplanting SCs into PNI (Salehi et al., 2018; Yu et al., 2022; Chen et al., 2023). The differentiated NSCs showed spindle-like morphology similar to SCs and expressed glial cell markers p75 and S100 (Tong et al., 2010). SCs differentiated from NSCs can promote axonal growth (Tong et al., 2010). This suggests that NSCs can differentiate into SCs with similar morphology, phenotype and function. Interestingly, the implanted NSCs can differentiate into SCs and maintain a microenvironment rich in growth factors, thus promoting nerve regeneration (Lee et al., 2017). In vitro experiments showed that IL12p80 (homodimer of IL12p40) promoted the differentiation of mouse NSCs into SCs through signal transduction and phosphorylation of activator of transcription 3 (STAT3) (Lee et al., 2017). In the mouse model of sciatic nerve injury, the combined transplantation of NSCs with neural conduit and IL12p80 could promote motor recovery and increase the medial diameter of regenerated nerve by 4.5 times (Lee et al., 2017). Other studies have also revealed that co-culture of SCs and NSCs can promote NSCs to differentiate into neurons and secrete higher levels of neurotrophic factors including BDNF and GDNF (Yu et al., 2017). All these reveal the fact that NSCs can not only differentiate into other cell phenotypes, but also differentiate into SCs to promote and participate in the repair of PNI. The above studies show that NSCs can be used as ideal candidate cells for PNI regeneration and have a great application prospect in PNI regeneration and repair.
The treatment of neurological diseases has always been a thorny problem in clinic, and it is very important to stop and delay the progress of these diseases. Unfortunately, although drugs or other methods are often used in clinic to alleviate the progression of the disease, the therapeutic effect is still not satisfactory, which brings a great challenge to the clinic. It is important is that these methods cannot replace the lost/degenerated neurons, restore the stability of the internal environment and reconstruct the neural network, so there are great defects in the treatment. In addition, there are inherent defects in long-term drug use, such as dependence, side effects and cut-off phenomena, and huge economic burden, which lead to slow progress in clinical treatment. The emergence of cell replacement therapy has opened up a bright future for the treatment of neurological diseases. The unique biological characteristics of NSCs and their great potential in the treatment of neurological diseases have been favored by many researchers. As mentioned earlier, in different basic research fields, NSCs transplantation has a therapeutic effect on neurological diseases, which can reverse and improve disease progression and repair function. In view of the substantial results of basic research, researchers have risen from basic research to the exploration stage of clinical trials to further explore the effectiveness, safety and feasibility of NSCs in clinical trials.
Although the reports of NSCs in clinical trials of neurological diseases are limited, but some clinical trials have reported the potential value of NSCs in the treatment of neurological diseases and achieved some encouraging results (Glass et al., 2012; Luan et al., 2012; Riley et al., 2012). In a phase 1 clinical trial, 12 patients with ALS received intraspinal injection of NSCs. After a follow-up of 30 months, these patients received MRI assessment, standard clinical evaluation and regular functional evaluation, including ALSFRS-R, sitting forced vital capacity, grip strength assessment, hand dynamometer, electrical impedance myogram and bladder ultrasound. The results showed good tolerance and the feasibility and safety of cell transplantation, which delayed the progression of the disease (Feldman et al., 2014). A clinical trial in which T cell vaccines combined with autologous NSCs were transplanted into 7 patients with ALS. The patients were evaluated by using rating scale (ALSFRS-R) and neurological function. Results showed that cell transplantation could slow down or stop the progression of the disease. The median survival time of the patients was prolonged from 3.5 years to 6 years, and the improvement of neurological function lasted for at least 1 year. It is considered that cell transplantation may be a feasible, minimally invasive, safe and effective method, and can obtain lasting curative effect (Moviglia et al., 2012). Another clinical trial found that fetal NSCs were transplanted into the spinal cord of 6 patients with ALS. The main outcome measure is short-term and long-term security. mortality and severe adverse reactions of any cause, while secondary outcome measures were ALS-FRS-R scale and forced vital capacity (FVC) measurement. No increase in disease progression was found during the 18-month follow-up after transplantation (Mazzini et al., 2015). The ALS-FRS-R scale of two patients (from 1 to 2) showed a transient improvement in walking. One patient’s tibialis anterior muscle MRC score improved for 7 months (Mazzini et al., 2015). Two patients refused to accept PEG and invasive ventilator and died of respiratory failure 8 months after operation, confirming that the death was related to the progression of the disease (Mazzini et al., 2015). It is suggested that NSCs transplantation is a safe method of cell therapy. 18 patients with ALS received human NSCs transplantation. Patients before and after transplantation were monitored by clinical, psychological, neuroradiological and neurophysiological evaluation. None of the patients showed serious adverse reactions or accelerated disease progression during the 60-month follow-up (Mazzini et al., 2019). It also reflects that transplanting NSCs is a safe method and will not have significant harmful effects in the short and long term. However, other clinical trials have found that NSCs transplantation can bring related side effects. In the phase 1/2 clinical trial, it is safe and feasible that NSCs can be transplanted into 15 patients with ALS through spinal canal at high dose. The patients were followed up for up to 24 months and assessed for adverse events and disease progression, measured by the ALS functional rating scale, a revised quantitative measurement of forced vital capacity and strength (Glass et al., 2016). However, one patient has transient pain and side effects of immunosuppressive, one patient has acute neurological deterioration and the other has central pain syndrome (Glass et al., 2016).
A clinical trial reported the effect of NSCs transplantation in a patient with PD. Human neural progenitor cells/undifferentiated NSCs were transplanted into the dorsal putamen of 8 patients with moderate PD. The patients were evaluated by neurology, neuropsychology and brain imaging before operation and 1 year, 2 years and 4 years after operation. It was proved that the cell transplantation was safe, there was no immune reaction to the transplantation, and there was no adverse reaction (Madrazo et al., 2019). The patient’s neuropsychological score was not affected by the transplantation (Madrazo et al., 2019). The motor function of all but one of the patients was improved in varying degrees, and the response of five patients to L-dopa showed better curative effect (Madrazo et al., 2019). PET imaging showed a tendency to increase dopaminergic activity in the midbrain after cell transplantation (Madrazo et al., 2019). Other clinical trials reported that NSCs were transplanted into the striatum of 21 patients with PD. Unified Parkinson’s Disease Rating Scale (UPDRS), Hoehn-Yahr, PDQ-39 and Schwab-England Scores were used to evaluate the Parkinson patients’ neurofunctions. The possible side effects of transplantation were evaluated from four aspects: a) tumor formation, b) immune rejection and the use of immunosuppressants, c) graft complications and d) side effects related to delivery. The symptoms of all PD patients were significantly improved, and there were no obvious side effects including tumorigenesis, immune rejection and complications after transplantation (Lige and Zengmin, 2016) (Table 3).
These clinical trials reflect the feasibility of NSCs transplantation in clinical trials of neurological diseases, but the safety and efficacy of NSCs transplantation are still not very clear, and there is a lack of more direct clinical data to support it. Although NSCs have found great potential therapeutic value in animal basic experiments and obtained encouraging favorable results, they ignore the differences and diversity of humans. It is not clear whether the positive and beneficial results obtained in animal studies will achieve similar results in human clinical trials, and there is a lack of more clinical data. Otherwise, it is not feasible to introduce it directly into the human body for granted. At this stage, NSCs are not widely used in clinical trials, which may be related to some problems existing in stem cell transplantation and hinder the development of clinical trials.
First, a rich and effective source of cells is the primary problem that limits the wide range of clinical development. The natural limitations of cell source and in vitro expansion are still the main challenges to the development of autologous cell transplantation. The sources of autologous or allogeneic fetal NSCs are limited and ethically limited. Although NSCs can be derived from reprogramming or iPSCs, appropriate manufacturing processes must be established and certified to ensure that they range from research to therapeutic development and application. However, the lack of consensus on the characteristics of these neural stem cells and the methods used to evaluate them is still a controversial issue. Moreover, the transplantation of these derived NSCs into the body has certain safety risks and side effects, including tumor formation, genetic instability and related complications after transplantation. Therefore, for cells from these sources, cell characteristics and genetic fidelity should be evaluated to avoid cell line switching, cross contamination and carrying chromosome rearrangement. Karyotype analysis and short tandem repeat analysis are the simplest techniques to achieve this purpose. therefore, it should be carried out routinely.
In addition, the cell culture cycle in vitro is relatively long, the culture system is still imperfect and lack of efficiency, which may miss the key treatment window []. Therefore, in vitro and purified cells need relatively short expansion culture, enhance the viability and proliferation of cultured cells, improve the culture system in vitro, and obtain abundant cells more efficiently. The formulation of strict culture programs and strict quality control may help us to achieve this goal. Moreover, studies have shown that there are differences in the treatment of neurological diseases in different passage lines of NSCs culture (Du et al., 2018). However, it is not clear which generation of cultured cells can achieve the best therapeutic effect, which needs to be explored and completed.
Second, the method of cell transplantation and the problem of location and tracking in vivo. NSCs can be transplanted into the body through a variety of methods and techniques, including intracerebral/spinal cord direct injection, intravenous transplantation, subarachnoid space transplantation and local injury site direct transplantation (Zhang et al., 2023). Although these methods can be well implemented by surgeons, there are differences in the therapeutic effects of different transplantation methods. For example, cells from vein transplantation or subarachnoid space transplantation can flow to other parts through the vein, and few of them may be colonized in the nerve injury site to play a therapeutic role. Currently, it is uncertain which transplantation method has the best therapeutic effect, and there is a lack of consensus. In addition, the transplanted cells cannot be tracked in vivo, and it is impossible to determine whether the transplanted cells are accurately colonized at the injured site, which also leads to a problem in the application of cell transplantation at present.
Third, the stability and safety of NSCs transplantation. Studies have shown that transplantation of NSCs into patients can lead to certain side effects, such as transient pain, central pain syndrome, immunosuppression and acute neurological deterioration (Riley et al., 2014; Glass et al., 2016). Therefore, the wide application of NSCs in clinical trials requires more animal research, fully understand the biological characteristics and safety of NSCs in vivo, and reduce the risk of additional injury. Thus, a good understanding of how NSCs work in vivo and how they connect and integrate with host cells, overcome tissue and environment-specific barriers, better characterize and control NSCs differentiation in vivo, target cell subtypes (neuronal subtypes) required for differentiation, and interact with the host.
Moreover, the time and dose of cell transplantation can lead to some differences or poor therapeutic effects. At present, there is a lack of guidance and consensus on the application of transplantation time and dose, which also needs more experimental work to explore in the future. Although the replacement therapy of NSCs transplantation is in the primary stage of exploration, there are many uncertain factors and many problems that need to be solved, but these will not prevent researchers from carrying out in-depth exploration and intensified work. in the end, these problems will be well solved.
In view of the differentiation potential of NSCs and their unique characteristics in the regeneration and repair of nerve injury, NSCs have great potential in the treatment of neurological diseases. NSCs transplantation has made great progress in the treatment of neurological diseases, revealing the fact that they can be a candidate cell replacement therapy for neurological diseases. However, NSCs are not ready for clinical transplantation. Although a few clinical trials have reported that NSCs are feasible and safe in the treatment of patients with neurological diseases, their wide application is still greatly hindered, and more direct evidence is needed to support it. Although there are many problems that need to be solved at present, they will not hinder the pace of researchers’ exploration, and eventually these problems will be well solved. In a word, neural stem cells are a promising cell transplantation therapy for the treatment of neurological diseases.
LY: Supervision, Writing–review and editing. S-CL: Data curation, Methodology, Software, Writing–review and editing. Y-YL: Formal Analysis, Writing–review and editing. F-QZ: Data curation, Formal Analysis, Writing–review and editing. M-JX: Formal Analysis, Writing–review and editing. D-XH: Writing–review and editing. W-JZ: Data curation, Funding acquisition, Software, Writing–original draft, Writing–review and editing.
The author(s) declare financial support was received for the research, authorship, and/or publication of this article. These studies were supported by grants from the Natural Science Foundation of Jiangxi Province (20232BAB206048), Youth Science Foundation of Jiangxi Province (20224BAB216030).
The authors declare that the research was conducted in the absence of any commercial or financial relationships that could be construed as a potential conflict of interest.
All claims expressed in this article are solely those of the authors and do not necessarily represent those of their affiliated organizations, or those of the publisher, the editors and the reviewers. Any product that may be evaluated in this article, or claim that may be made by its manufacturer, is not guaranteed or endorsed by the publisher.
Aboul-Soud, M. A. M., Alzahrani, A. J., and Mahmoud, A. (2021). Induced pluripotent stem cells (iPSCs)-Roles in regenerative therapies, disease modelling and drug screening. Cells 10 (9), 2319. doi:10.3390/cells10092319
Ager, R. R., Davis, J. L., Agazaryan, A., Benavente, F., Poon, W. W., LaFerla, F. M., et al. (2015). Human neural stem cells improve cognition and promote synaptic growth in two complementary transgenic models of Alzheimer's disease and neuronal loss. Hippocampus 25 (7), 813–826. doi:10.1002/hipo.22405
Agrawal, A. K., Shukla, S., Chaturvedi, R. K., Seth, K., Srivastava, N., Ahmad, A., et al. (2004). Olfactory ensheathing cell transplantation restores functional deficits in rat model of Parkinson's disease: a cotransplantation approach with fetal ventral mesencephalic cells. Neurobiol. Dis. 16 (3), 516–526. doi:10.1016/j.nbd.2004.04.014
Alessandrini, M., Preynat-Seauve, O., De Bruin, K., and Pepper, M. S. (2019). Stem cell therapy for neurological disorders. S Afr. Med. J. 109 (8b), 70–77. doi:10.7196/samj.2019.v109i8b.14009
Al-Gharaibeh, A., Culver, R., Stewart, A. N., Srinageshwar, B., Spelde, K., Frollo, L., et al. (2017). Induced pluripotent stem cell-derived neural stem cell transplantations reduced behavioral deficits and ameliorated neuropathological changes in YAC128 mouse model of Huntington's disease. Front. Neurosci. 11, 628. doi:10.3389/fnins.2017.00628
Alvarez-Buylla, A., García-Verdugo, J. M., and Tramontin, A. D. (2001). A unified hypothesis on the lineage of neural stem cells. Nat. Rev. Neurosci. 2 (4), 287–293. doi:10.1038/35067582
Andrzejewska, A., Dabrowska, S., Lukomska, B., and Janowski, M. (2021). Mesenchymal stem cells for neurological disorders. Adv. Sci. (Weinh). 8 (7), 2002944. doi:10.1002/advs.202002944
Assinck, P., Duncan, G. J., Hilton, B. J., Plemel, J. R., and Tetzlaff, W. (2017). Cell transplantation therapy for spinal cord injury. Nat. Neurosci. 20 (5), 637–647. doi:10.1038/nn.4541
Baloh, R. H., Glass, J. D., and Svendsen, C. N. (2018). Stem cell transplantation for amyotrophic lateral sclerosis. Curr. Opin. Neurol. 31 (5), 655–661. doi:10.1097/wco.0000000000000598
Bashir, H. (2019). Emerging therapies in Huntington's disease. Expert Rev. Neurother. 19 (10), 983–995. doi:10.1080/14737175.2019.1631161
Bellenchi, G. C., Volpicelli, F., Piscopo, V., Perrone-Capano, C., and di Porzio, U. (2013). Adult neural stem cells: an endogenous tool to repair brain injury? J. Neurochem. 124 (2), 159–167. doi:10.1111/jnc.12084
Berlet, R., Galang Cabantan, D. A., Gonzales-Portillo, D., and Borlongan, C. V. (2022). Enriched environment and exercise enhance stem cell therapy for stroke, Parkinson's disease, and Huntington's disease. Front. Cell Dev. Biol. 10, 798826. doi:10.3389/fcell.2022.798826
Bosch, M., Pineda, J. R., Suñol, C., Petriz, J., Cattaneo, E., Alberch, J., et al. (2004). Induction of GABAergic phenotype in a neural stem cell line for transplantation in an excitotoxic model of Huntington's disease. Exp. Neurol. 190 (1), 42–58. doi:10.1016/j.expneurol.2004.06.027
Caiazzo, M., Giannelli, S., Valente, P., Lignani, G., Carissimo, A., Sessa, A., et al. (2015). Direct conversion of fibroblasts into functional astrocytes by defined transcription factors. Stem Cell Rep. 4 (1), 25–36. doi:10.1016/j.stemcr.2014.12.002
Campos, H. C., Ribeiro, D. E., Hashiguchi, D., Hukuda, D. Y., Gimenes, C., Romariz, S. A. A., et al. (2022). Distinct effects of the hippocampal transplantation of neural and mesenchymal stem cells in a transgenic model of Alzheimer's disease. Stem Cell Rev. Rep. 18 (2), 781–791. doi:10.1007/s12015-021-10321-9
Chambers, S. M., and Studer, L. (2011). Cell fate plug and play: direct reprogramming and induced pluripotency. Cell 145 (6), 827–830. doi:10.1016/j.cell.2011.05.036
Chen, P., Piao, X., and Bonaldo, P. (2015). Role of macrophages in Wallerian degeneration and axonal regeneration after peripheral nerve injury. Acta Neuropathol. 130 (5), 605–618. doi:10.1007/s00401-015-1482-4
Chen, Y. S., Ng, H. Y., Chen, Y. W., Cho, D. Y., Ho, C. C., Chen, C. Y., et al. (2023). Additive manufacturing of Schwann cell-laden collagen/alginate nerve guidance conduits by freeform reversible embedding regulate neurogenesis via exosomes secretion towards peripheral nerve regeneration. Biomater. Adv. 146, 213276. doi:10.1016/j.bioadv.2022.213276
Cheng, L. N., Duan, X. H., Zhong, X. M., Guo, R. M., Zhang, F., Zhou, C. P., et al. (2011). Transplanted neural stem cells promote nerve regeneration in acute peripheral nerve traction injury: assessment using MRI. AJR Am. J. Roentgenol. 196 (6), 1381–1387. doi:10.2214/ajr.10.5495
Cho, I. K., Yang, B., Forest, C., Qian, L., and Chan, A. W. S. (2019). Amelioration of Huntington's disease phenotype in astrocytes derived from iPSC-derived neural progenitor cells of Huntington's disease monkeys. PLoS One 14 (3), e0214156. doi:10.1371/journal.pone.0214156
Chou, C. H., Fan, H. C., and Hueng, D. Y. (2015). Potential of neural stem cell-based therapy for Parkinson's disease. Park. Dis. 2015, 1–9. doi:10.1155/2015/571475
Chung, Y. F., Chen, J. H., Li, C. W., Hsu, H. Y., Chen, Y. P., Wang, C. C., et al. (2022). Human IL12p80 promotes murine oligodendrocyte differentiation to repair nerve injury. Int. J. Mol. Sci. 23 (13), 7002. doi:10.3390/ijms23137002
Connor, B. (2018). Concise review: the use of stem cells for understanding and treating Huntington's disease. Stem Cells 36 (2), 146–160. doi:10.1002/stem.2747
DAiuto, L., Zhi, Y., Kumar Das, D., Wilcox, M. R., Johnson, J. W., McClain, L., et al. (2014). Large-scale generation of human iPSC-derived neural stem cells/early neural progenitor cells and their neuronal differentiation. Organogenesis 10 (4), 365–377. doi:10.1080/15476278.2015.1011921
de Freria, C. M., Van Niekerk, E., Blesch, A., and Lu, P. (2021). Neural stem cells: promoting axonal regeneration and spinal cord connectivity. Cells 10 (12), 3296. doi:10.3390/cells10123296
De Gioia, R., Biella, F., Citterio, G., Rizzo, F., Abati, E., Nizzardo, M., et al. (2020). Neural stem cell transplantation for neurodegenerative diseases. Int. J. Mol. Sci. 21 (9), 3103. doi:10.3390/ijms21093103
Delbeuck, X., Van der Linden, M., and Collette, F. (2003). Alzheimer's disease as a disconnection syndrome? Neuropsychol. Rev. 13 (2), 79–92. doi:10.1023/a:1023832305702
Deng, X., Liang, Y., Lu, H., Yang, Z., Liu, R., Wang, J., et al. (2013). Co-transplantation of GDNF-overexpressing neural stem cells and fetal dopaminergic neurons mitigates motor symptoms in a rat model of Parkinson's disease. PLoS One 8 (12), e80880. doi:10.1371/journal.pone.0080880
Dong, M. M., and Yi, T. H. (2010). Stem cell and peripheral nerve injury and repair. Facial Plast. Surg. 26 (5), 421–428. doi:10.1055/s-0030-1265023
Du, J., Chen, H., Zhou, K., and Jia, X. (2018). Quantitative multimodal evaluation of passaging human neural crest stem cells for peripheral nerve regeneration. Stem Cell Rev. Rep. 14 (1), 92–100. doi:10.1007/s12015-017-9758-9
Duncan, T., and Valenzuela, M. (2017). Alzheimer's disease, dementia, and stem cell therapy. Stem Cell Res. Ther. 8 (1), 111. doi:10.1186/s13287-017-0567-5
Fang, E. F., Hou, Y., Palikaras, K., Adriaanse, B. A., Kerr, J. S., Yang, B., et al. (2019). Mitophagy inhibits amyloid-β and tau pathology and reverses cognitive deficits in models of Alzheimer’s disease. Nat. Neurosci. 22 (3), 401–412. doi:10.1038/s41593-018-0332-9
Fayazi, N., Sheykhhasan, M., Soleimani Asl, S., and Najafi, R. (2021). Stem cell-derived exosomes: a new strategy of neurodegenerative disease treatment. Mol. Neurobiol. 58 (7), 3494–3514. doi:10.1007/s12035-021-02324-x
Feldman, E. L., Boulis, N. M., Hur, J., Johe, K., Rutkove, S. B., Federici, T., et al. (2014). Intraspinal neural stem cell transplantation in amyotrophic lateral sclerosis: phase 1 trial outcomes. Ann. Neurol. 75 (3), 363–373. doi:10.1002/ana.24113
Feliciano, D. M., Bordey, A., and Bonfanti, L. (2015). Noncanonical sites of adult neurogenesis in the mammalian brain. Cold Spring Harb. Perspect. Biol. 7 (10), a018846. doi:10.1101/cshperspect.a018846
Forostyak, S., Forostyak, O., Kwok, J. C. F., Romanyuk, N., Rehorova, M., Kriska, J., et al. (2020). Transplantation of neural precursors derived from induced pluripotent cells preserve perineuronal nets and stimulate neural plasticity in ALS rats. Int. J. Mol. Sci. 21 (24), 9593. doi:10.3390/ijms21249593
Fu, K. Y., Dai, L. G., Chiu, I. M., Chen, J. R., and Hsu, S. H. (2011). Sciatic nerve regeneration by microporous nerve conduits seeded with glial cell line-derived neurotrophic factor or brain-derived neurotrophic factor gene transfected neural stem cells. Artif. Organs 35 (4), 363–372. doi:10.1111/j.1525-1594.2010.01105.x
Fujita, S. (2003). The discovery of the matrix cell, the identification of the multipotent neural stem cell and the development of the central nervous system. Cell Struct. Funct. 28 (4), 205–228. doi:10.1247/csf.28.205
Gage, F. H., and Temple, S. (2013). Neural stem cells: generating and regenerating the brain. Neuron 80 (3), 588–601. doi:10.1016/j.neuron.2013.10.037
Glass, J. D., Boulis, N. M., Johe, K., Rutkove, S. B., Federici, T., Polak, M., et al. (2012). Lumbar intraspinal injection of neural stem cells in patients with amyotrophic lateral sclerosis: results of a phase I trial in 12 patients. Stem Cells 30 (6), 1144–1151. doi:10.1002/stem.1079
Glass, J. D., Hertzberg, V. S., Boulis, N. M., Riley, J., Federici, T., Polak, M., et al. (2016). Transplantation of spinal cord-derived neural stem cells for ALS: analysis of phase 1 and 2 trials. Neurology 87 (4), 392–400. doi:10.1212/wnl.0000000000002889
Gleason, D., Fallon, J. H., Guerra, M., Liu, J. C., and Bryant, P. J. (2008). Ependymal stem cells divide asymmetrically and transfer progeny into the subventricular zone when activated by injury. Neuroscience 156 (1), 81–88. doi:10.1016/j.neuroscience.2008.06.065
Gonzalez, R., Hamblin, M. H., and Lee, J. P. (2016). Neural stem cell transplantation and CNS diseases. CNS Neurol. Disord. Drug Targets 15 (8), 881–886. doi:10.2174/1871527315666160815164247
Goulart, C. O., Ângelo Durço, D. F., de Carvalho, L. A., Oliveira, J. T., Alves, L., Cavalcante, L. A., et al. (2016). Olfactory ensheathing glia cell therapy and tubular conduit enhance nerve regeneration after mouse sciatic nerve transection. Brain Res. 1650, 243–251. doi:10.1016/j.brainres.2016.09.021
Graf, T., and Enver, T. (2009). Forcing cells to change lineages. Nature 462 (7273), 587–594. doi:10.1038/nature08533
Grochowski, C., Radzikowska, E., and Maciejewski, R. (2018). Neural stem cell therapy-Brief review. Clin. Neurol. Neurosurg. 173, 8–14. doi:10.1016/j.clineuro.2018.07.013
Gu, S., Huang, H., Bi, J., Yao, Y., and Wen, T. (2009). Combined treatment of neurotrophin-3 gene and neural stem cells is ameliorative to behavior recovery of Parkinson's disease rat model. Brain Res. 1257, 1–9. doi:10.1016/j.brainres.2008.12.016
Gu, S., Shen, Y., Xu, W., Xu, L., Li, X., Zhou, G., et al. (2010). Application of fetal neural stem cells transplantation in delaying denervated muscle atrophy in rats with peripheral nerve injury. Microsurgery 30 (4), 266–274. doi:10.1002/micr.20722
Guillemot, F. (2005). Cellular and molecular control of neurogenesis in the mammalian telencephalon. Curr. Opin. Cell Biol. 17 (6), 639–647. doi:10.1016/j.ceb.2005.09.006
Haubensak, W., Attardo, A., Denk, W., and Huttner, W. B. (2004). Neurons arise in the basal neuroepithelium of the early mammalian telencephalon: a major site of neurogenesis. Proc. Natl. Acad. Sci. U. S. A. 101 (9), 3196–3201. doi:10.1073/pnas.0308600100
Hefferan, M. P., Galik, J., Kakinohana, O., Sekerkova, G., Santucci, C., Marsala, S., et al. (2012). Human neural stem cell replacement therapy for amyotrophic lateral sclerosis by spinal transplantation. PLoS One 7 (8), e42614. doi:10.1371/journal.pone.0042614
Heris, R. M., Shirvaliloo, M., Abbaspour-Aghdam, S., Hazrati, A., Shariati, A., Youshanlouei, H. R., et al. (2022). The potential use of mesenchymal stem cells and their exosomes in Parkinson's disease treatment. Stem Cell Res. Ther. 13 (1), 371. doi:10.1186/s13287-022-03050-4
Höglinger, G. U., Rizk, P., Muriel, M. P., Duyckaerts, C., Oertel, W. H., Caille, I., et al. (2004). Dopamine depletion impairs precursor cell proliferation in Parkinson disease. Nat. Neurosci. 7 (7), 726–735. doi:10.1038/nn1265
Huang, J., Yi, L., Yang, X., Zheng, Q., Zhong, J., Ye, S., et al. (2023). Neural stem cells transplantation combined with ethyl stearate improve PD rats motor behavior by promoting NSCs migration and differentiation. CNS Neurosci. Ther. 29 (6), 1571–1584. doi:10.1111/cns.14119
Ishii, K., Nakamura, M., Dai, H., Finn, T. P., Okano, H., Toyama, Y., et al. (2006). Neutralization of ciliary neurotrophic factor reduces astrocyte production from transplanted neural stem cells and promotes regeneration of corticospinal tract fibers in spinal cord injury. J. Neurosci. Res. 84 (8), 1669–1681. doi:10.1002/jnr.21079
Itakura, G., Ozaki, M., Nagoshi, N., Kawabata, S., Nishiyama, Y., Sugai, K., et al. (2017). Low immunogenicity of mouse induced pluripotent stem cell-derived neural stem/progenitor cells. Sci. Rep. 7 (1), 12996. doi:10.1038/s41598-017-13522-w
Ito, K., and Suda, T. (2014). Metabolic requirements for the maintenance of self-renewing stem cells. Nat. Rev. Mol. Cell Biol. 15 (4), 243–256. doi:10.1038/nrm3772
Kageyama, R., Hatakeyama, J., and Ohtsuka, T. (2006). Transcription factors in the nervous system: development, brain function, and diseases. Wiley; Roles Hes bHLH factors neural Dev. 3–22.
Kim, A., Lalonde, K., Truesdell, A., Gomes Welter, P., Brocardo, P. S., Rosenstock, T. R., et al. (2021b). New avenues for the treatment of Huntington's disease. Int. J. Mol. Sci. 22 (16), 8363. doi:10.3390/ijms22168363
Kim, J. J., Shin, J. H., Yu, K. R., Lee, B. C., Kang, I., Lee, J. Y., et al. (2017). Direct conversion of human umbilical cord blood into induced neural stem cells with SOX2 and HMGA2. Int. J. Stem Cells 10 (2), 227–234. doi:10.15283/ijsc17025
Kim, S. U., Lee, H. J., and Kim, Y. B. (2013). Neural stem cell-based treatment for neurodegenerative diseases. Neuropathology 33 (5), 491–504. doi:10.1111/neup.12020
Kim, S. W., Woo, H. J., Kim, E. H., Kim, H. S., Suh, H. N., Kim, S. H., et al. (2021a). Neural stem cells derived from human midbrain organoids as a stable source for treating Parkinson's disease: midbrain organoid-NSCs (Og-NSC) as a stable source for PD treatment. Prog. Neurobiol. 204, 102086. doi:10.1016/j.pneurobio.2021.102086
Kokaia, Z., Martino, G., Schwartz, M., and Lindvall, O. (2012). Cross-talk between neural stem cells and immune cells: the key to better brain repair? Nat. Neurosci. 15 (8), 1078–1087. doi:10.1038/nn.3163
Kriegstein, A., and Alvarez-Buylla, A. (2009). The glial nature of embryonic and adult neural stem cells. Annu. Rev. Neurosci. 32, 149–184. doi:10.1146/annurev.neuro.051508.135600
Latoszek, E., and Czeredys, M. (2021). Molecular components of store-operated calcium channels in the regulation of neural stem cell physiology, neurogenesis, and the pathology of Huntington's disease. Front. Cell Dev. Biol. 9, 657337. doi:10.3389/fcell.2021.657337
Lee, D. C., Chen, J. H., Hsu, T. Y., Chang, L. H., Chang, H., Chi, Y. H., et al. (2017). Neural stem cells promote nerve regeneration through IL12-induced Schwann cell differentiation. Mol. Cell Neurosci. 79, 1–11. doi:10.1016/j.mcn.2016.11.007
Lee, I. S., Jung, K., Kim, I. S., Lee, H., Kim, M., Yun, S., et al. (2015). Human neural stem cells alleviate Alzheimer-like pathology in a mouse model. Mol. Neurodegener. 10, 38. doi:10.1186/s13024-015-0035-6
Li, E., Park, H. R., Hong, C. P., Kim, Y., Choi, J., Lee, S., et al. (2020a). Neural stem cells derived from the developing forebrain of YAC128 mice exhibit pathological features of Huntington's disease. Cell Prolif. 53 (10), e12893. doi:10.1111/cpr.12893
Li, L. K., Huang, W. C., Hsueh, Y. Y., Yamauchi, K., Olivares, N., Davila, R., et al. (2022). Intramuscular delivery of neural crest stem cell spheroids enhances neuromuscular regeneration after denervation injury. Stem Cell Res. Ther. 13 (1), 205. doi:10.1186/s13287-022-02877-1
Li, X., Zhu, H., Sun, X., Zuo, F., Lei, J., Wang, Z., et al. (2016). Human neural stem cell transplantation rescues cognitive defects in APP/PS1 model of Alzheimer's disease by enhancing neuronal connectivity and metabolic activity. Front. Aging Neurosci. 8, 282. doi:10.3389/fnagi.2016.00282
Li, Y., Men, Y., Wang, B., Chen, X., and Yu, Z. (2020b). Co-transplantation of Schwann cells and neural stem cells in the laminin-chitosan-PLGA nerve conduit to repair the injured recurrent laryngeal nerve in SD rats. J. Mater Sci. Mater Med. 31 (11), 99. doi:10.1007/s10856-020-06436-z
Li, Y., Yu, Z., Men, Y., Chen, X., and Wang, B. (2018). Laminin-chitosan-PLGA conduit co-transplanted with Schwann and neural stem cells to repair the injured recurrent laryngeal nerve. Exp. Ther. Med. 16 (2), 1250–1258. doi:10.3892/etm.2018.6343
Liao, Y. Z., Ma, J., and Dou, J. Z. (2022). The role of TDP-43 in neurodegenerative disease. Mol. Neurobiol. 59 (7), 4223–4241. doi:10.1007/s12035-022-02847-x
Lige, L., and Zengmin, T. (2016). Transplantation of neural precursor cells in the treatment of Parkinson disease: an efficacy and safety analysis. Turk Neurosurg. 26 (3), 378–383. doi:10.5137/1019-5149.jtn.10747-14.4
Liu, J. (2013). Induced pluripotent stem cell-derived neural stem cells: new hope for stroke? Stem Cell Res. Ther. 4 (5), 115. doi:10.1186/scrt326
Liu, Y., Qiao, F., Leiferman, P. C., Ross, A., Schlenker, E. H., and Wang, H. (2017). FOXOs modulate proteasome activity in human-induced pluripotent stem cells of Huntington's disease and their derived neural cells. Hum. Mol. Genet. 26 (22), 4416–4428. doi:10.1093/hmg/ddx327
Lu, M. H., Ji, W. L., Chen, H., Sun, Y. Y., Zhao, X. Y., Wang, F., et al. (2021). Intranasal transplantation of human neural stem cells ameliorates Alzheimer's disease-like pathology in a mouse model. Front. Aging Neurosci. 13, 650103. doi:10.3389/fnagi.2021.650103
Luan, Z., Liu, W., Qu, S., Du, K., He, S., Wang, Z., et al. (2012). Effects of neural progenitor cell transplantation in children with severe cerebral palsy. Cell Transpl. 21 (Suppl. 1), S91–S98. doi:10.3727/096368912x633806
Madrazo, I., Kopyov, O., Ávila-Rodríguez, M. A., Ostrosky, F., Carrasco, H., Kopyov, A., et al. (2019). Transplantation of human neural progenitor cells (NPC) into putamina of parkinsonian patients: a case series study, safety and efficacy four years after surgery. Cell Transpl. 28 (3), 269–285. doi:10.1177/0963689718820271
Marsh, S. E., and Blurton-Jones, M. (2017). Neural stem cell therapy for neurodegenerative disorders: the role of neurotrophic support. Neurochem. Int. 106, 94–100. doi:10.1016/j.neuint.2017.02.006
Marutle, A., Ohmitsu, M., Nilbratt, M., Greig, N. H., Nordberg, A., and Sugaya, K. (2007). Modulation of human neural stem cell differentiation in Alzheimer (APP23) transgenic mice by phenserine. Proc. Natl. Acad. Sci. U. S. A. 104 (30), 12506–12511. doi:10.1073/pnas.0705346104
Mazzini, L., Gelati, M., Profico, D. C., Sgaravizzi, G., Projetti Pensi, M., Muzi, G., et al. (2015). Human neural stem cell transplantation in ALS: initial results from a phase I trial. J. Transl. Med. 13, 17. doi:10.1186/s12967-014-0371-2
Mazzini, L., Gelati, M., Profico, D. C., Sorarù, G., Ferrari, D., Copetti, M., et al. (2019). Results from phase I clinical trial with intraspinal injection of neural stem cells in amyotrophic lateral sclerosis: a long-term outcome. Stem Cells Transl. Med. 8 (9), 887–897. doi:10.1002/sctm.18-0154
Mazzini, L., Vescovi, A., Cantello, R., Gelati, M., and Vercelli, A. (2016). Stem cells therapy for ALS. Expert Opin. Biol. Ther. 16 (2), 187–199. doi:10.1517/14712598.2016.1116516
Mendes-Pinheiro, B., Teixeira, F. G., Anjo, S. I., Manadas, B., Behie, L. A., and Salgado, A. J. (2018). Secretome of undifferentiated neural progenitor cells induces histological and motor improvements in a rat model of Parkinson's disease. Stem Cells Transl. Med. 7 (11), 829–838. doi:10.1002/sctm.18-0009
Merkle, F. T., and Alvarez-Buylla, A. (2006). Neural stem cells in mammalian development. Curr. Opin. Cell Biol. 18 (6), 704–709. doi:10.1016/j.ceb.2006.09.008
Meyer, S., Wörsdörfer, P., Günther, K., Thier, M., and Edenhofer, F. (2015). Derivation of adult human fibroblasts and their direct conversion into expandable neural progenitor cells. J. Vis. Exp. (101), e52831. doi:10.3791/52831
Meyer, T. (2021). Amyotrophe Lateralsklerose (ALS) – diagnose, Verlauf und neue Behandlungsoptionen [Amyotrophic lateral sclerosis (ALS) - diagnosis, course of disease and treatment options]. Dtsch. Med. Wochenschr 146 (24-25), 1613–1618. doi:10.1055/a-1562-7882
Mitrecić, D., Nicaise, C., Gajović, S., and Pochet, R. (2010). Distribution, differentiation, and survival of intravenously administered neural stem cells in a rat model of amyotrophic lateral sclerosis. Cell Transpl. 19 (5), 537–548. doi:10.3727/096368910x498269
Modrak, M., Talukder, M. A. H., Gurgenashvili, K., Noble, M., and Elfar, J. C. (2020). Peripheral nerve injury and myelination: potential therapeutic strategies. J. Neurosci. Res. 98 (5), 780–795. doi:10.1002/jnr.24538
Moghadam, F. H., Alaie, H., Karbalaie, K., Tanhaei, S., Nasr Esfahani, M. H., and Baharvand, H. (2009). Transplantation of primed or unprimed mouse embryonic stem cell-derived neural precursor cells improves cognitive function in Alzheimerian rats. Differentiation 78 (2-3), 59–68. doi:10.1016/j.diff.2009.06.005
Moshayedi, P., and Carmichael, S. T. (2013). Hyaluronan, neural stem cells and tissue reconstruction after acute ischemic stroke. Biomatter 3 (1), e23863. doi:10.4161/biom.23863
Moviglia, G. A., Moviglia-Brandolino, M. T., Varela, G. S., Albanese, G., Piccone, S., Echegaray, G., et al. (2012). Feasibility, safety, and preliminary proof of principles of autologous neural stem cell treatment combined with T-cell vaccination for ALS patients. Cell Transpl. 21 (Suppl. 1), S57–S63. doi:10.3727/096368912X633770
Nelke, A., García-López, S., Martínez-Serrano, A., and Pereira, M. P. (2022). Multifactoriality of Parkinson's disease as explored through human neural stem cells and their transplantation in middle-aged parkinsonian mice. Front. Pharmacol. 12, 773925. doi:10.3389/fphar.2021.773925
Ni, W., Zhou, J., Ling, Y., Lu, X., Niu, D., Zeng, Y., et al. (2022). Neural stem cell secretome exerts a protective effect on damaged neuron mitochondria in Parkinson's disease model. Brain Res., 1790, 147978. doi:10.1016/j.brainres.2022.147978
Nizzardo, M., Simone, C., Rizzo, F., Ruggieri, M., Salani, S., Riboldi, G., et al. (2014). Minimally invasive transplantation of iPSC-derived ALDHhiSSCloVLA4+ neural stem cells effectively improves the phenotype of an amyotrophic lateral sclerosis model. Hum. Mol. Genet. 23 (2), 342–354. doi:10.1093/hmg/ddt425
Nocera, G., and Jacob, C. (2020). Mechanisms of Schwann cell plasticity involved in peripheral nerve repair after injury. Cell Mol. Life Sci. 77 (20), 3977–3989. doi:10.1007/s00018-020-03516-9
Noctor, S. C., Martínez-Cerdeño, V., Ivic, L., and Kriegstein, A. R. (2004). Cortical neurons arise in symmetric and asymmetric division zones and migrate through specific phases. Nat. Neurosci. 7 (2), 136–144. doi:10.1038/nn1172
Okamoto, M., Namba, T., Shinoda, T., Kondo, T., Watanabe, T., Inoue, Y., et al. (2013). TAG-1-assisted progenitor elongation streamlines nuclear migration to optimize subapical crowding. Nat. Neurosci. 16 (11), 1556–1566. doi:10.1038/nn.3525
OSullivan, S. S., Johnson, M., Williams, D. R., Revesz, T., Holton, J. L., Lees, A. J., et al. (2011). The effect of drug treatment on neurogenesis in Parkinson's disease. Mov. Disord. 26 (1), 45–50. doi:10.1002/mds.23340
Palm, T., Bahnassawy, L., and Schwamborn, J. (2012). MiRNAs and neural stem cells: a team to treat Parkinson's disease? RNA Biol. 9 (6), 720–730. doi:10.4161/rna.19984
Park, H. J., Jeon, J., Choi, J., Kim, J. Y., Kim, H. S., Huh, J. Y., et al. (2021b). Human iPSC-derived neural precursor cells differentiate into multiple cell types to delay disease progression following transplantation into YAC128 Huntington's disease mouse model. Cell Prolif. 54 (8), e13082. doi:10.1111/cpr.13082
Park, M., Kim, H. M., Shin, H. A., Lee, S. H., Hwang, D. Y., and Lew, H. (2021a). Human pluripotent stem cell-derived neural progenitor cells promote retinal ganglion cell survival and axon recovery in an optic nerve compression animal model. Int. J. Mol. Sci. 22 (22), 12529. doi:10.3390/ijms222212529
Peters, O. M., Ghasemi, M., and Brown, R. H. (2015). Emerging mechanisms of molecular pathology in ALS. J. Clin. Invest. 125 (5), 1767–1779. doi:10.1172/jci71601
Pevny, L., and Rao, M. S. (2003). The stem-cell menagerie. Trends Neurosci. 26 (7), 351–359. doi:10.1016/s0166-2236(03)00169-3
Pisciotta, A., Bertoni, L., Vallarola, A., Bertani, G., Mecugni, D., and Carnevale, G. (2020). Neural crest derived stem cells from dental pulp and tooth-associated stem cells for peripheral nerve regeneration. Neural Regen. Res. 15 (3), 373–381. doi:10.4103/1673-5374.266043
Qian, Y., Chen, X. X., Wang, W., Li, J. J., Wang, X. P., Tang, Z. W., et al. (2020). Transplantation of Nurr1-overexpressing neural stem cells and microglia for treating parkinsonian rats. CNS Neurosci. Ther. 26 (1), 55–65. doi:10.1111/cns.13149
Ramalho, B. D. S., de Almeida, F. M., and Martinez, A. M. B. (2021). Cell therapy and delivery strategies for spinal cord injury. Histol. Histopathol. 36 (9), 907–920. doi:10.14670/HH-18-350
Reidling, J. C., Relaño-Ginés, A., Holley, S. M., Ochaba, J., Moore, C., Fury, B., et al. (2018). Human neural stem cell transplantation rescues functional deficits in R6/2 and Q140 Huntington's disease mice. Stem Cell Rep. 10 (1), 58–72. doi:10.1016/j.stemcr.2017.11.005
Riley, J., Federici, T., Polak, M., Kelly, C., Glass, J., Raore, B., et al. (2012). Intraspinal stem cell transplantation in amyotrophic lateral sclerosis: a phase I safety trial, technical note, and lumbar safety outcomes. Neurosurgery 71 (2), 405–416. doi:10.1227/neu.0b013e31825ca05f
Riley, J., Glass, J., Feldman, E. L., Polak, M., Bordeau, J., Federici, T., et al. (2014). Intraspinal stem cell transplantation in amyotrophic lateral sclerosis: a phase I trial, cervical microinjection, and final surgical safety outcomes. Neurosurgery 74 (1), 77–87. doi:10.1227/neu.0000000000000156
Ring, K. L., Tong, L. M., Balestra, M. E., Javier, R., Andrews-Zwilling, Y., Li, G., et al. (2012). Direct reprogramming of mouse and human fibroblasts into multipotent neural stem cells with a single factor. Cell Stem Cell 11 (1), 100–109. doi:10.1016/j.stem.2012.05.018
Salehi, M., Naseri-Nosar, M., Ebrahimi-Barough, S., Nourani, M., Khojasteh, A., Hamidieh, A. A., et al. (2018). Sciatic nerve regeneration by transplantation of Schwann cells via erythropoietin controlled-releasing polylactic acid/multiwalled carbon nanotubes/gelatin nanofibrils neural guidance conduit. J. Biomed. Mater Res. B Appl. Biomater. 106 (4), 1463–1476. doi:10.1002/jbm.b.33952
Salloway, S., Sperling, R., Fox, N. C., Blennow, K., Klunk, W., Raskind, M., et al. (2014). Two phase 3 trials of bapineuzumab in mild-to-moderate Alzheimer's disease. N. Engl. J. Med. 370 (4), 322–333. doi:10.1056/nejmoa1304839
Sekiguchi, H., Ii, M., Jujo, K., Thorne, T., Ito, A., Klyachko, E., et al. (2013). Estradiol promotes neural stem cell differentiation into endothelial lineage and angiogenesis in injured peripheral nerve. Angiogenesis 16 (1), 45–58. doi:10.1007/s10456-012-9298-5
Shafiq, K., Sanghai, N., Guo, Y., and Kong, J. (2021). Implication of post-translationally modified SOD1 in pathological aging. Geroscience 43 (2), 507–515. doi:10.1007/s11357-021-00332-2
Shi, Y., Zhou, L., Tian, J., and Wang, Y. (2009). Transplantation of neural stem cells overexpressing glia-derived neurotrophic factor promotes facial nerve regeneration. Acta Otolaryngol. 129 (8), 906–914. doi:10.1080/00016480802468153
Stipcevic, T., Knight, C. P., and Kippin, T. E. (2013). Stimulation of adult neural stem cells with a novel glycolipid biosurfactant. Acta Neurol. Belg 113 (4), 501–506. doi:10.1007/s13760-013-0232-4
Sugaya, K., and Vaidya, M. (2018). Stem cell therapies for neurodegenerative diseases. Adv. Exp. Med. Biol. 1056, 61–84. doi:10.1007/978-3-319-74470-4_5
Sullivan, R., Dailey, T., Duncan, K., Abel, N., and Borlongan, C. V. (2016). Peripheral nerve injury: stem cell therapy and peripheral nerve transfer. Int. J. Mol. Sci. 17 (12), 2101. doi:10.3390/ijms17122101
Syu, W. Z., Hueng, D. Y., Chen, W. L., Chan, J. Y., Chen, S. G., and Huang, S. M. (2019). Adipose-derived neural stem cells combined with acellular dermal matrix as a neural conduit enhances peripheral nerve repair. Cell Transpl. 28 (9-10), 1220–1230. doi:10.1177/0963689719853512
Takahashi, K., and Yamanaka, S. (2006). Induction of pluripotent stem cells from mouse embryonic and adult fibroblast cultures by defined factors. Cell 126 (4), 663–676. doi:10.1016/j.cell.2006.07.024
Teng, Y. D., Benn, S. C., Kalkanis, S. N., Shefner, J. M., Onario, R. C., Cheng, B., et al. (2012). Multimodal actions of neural stem cells in a mouse model of ALS: a meta-analysis. Sci. Transl. Med. 4 (165), 165ra164. doi:10.1126/scitranslmed.3004579
Tong, L., Ji, L., Wang, Z., Tong, X., Zhang, L., and Sun, X. (2010). Differentiation of neural stem cells into Schwann-like cells in vitro. Biochem. Biophys. Res. Commun. 401 (4), 592–597. doi:10.1016/j.bbrc.2010.09.107
Wang, Q., Matsumoto, Y., Shindo, T., Miyake, K., Shindo, A., Kawanishi, M., et al. (2006). Neural stem cells transplantation in cortex in a mouse model of Alzheimer’s disease. J. Med. Invest. 53 (1-2), 61–69. doi:10.2152/jmi.53.61
Xiong, Y., Zhu, J. X., Fang, Z. Y., Zeng, C. G., Zhang, C., Qi, G. L., et al. (2012). Coseeded Schwann cells myelinate neurites from differentiated neural stem cells in neurotrophin-3-loaded PLGA carriers. Int. J. Nanomedicine 7, 1977–1989. doi:10.2147/ijn.s30706
Xu, L., Zhou, S., Feng, G. Y., Zhang, L. P., Zhao, D. M., Sun, Y., et al. (2012). Neural stem cells enhance nerve regeneration after sciatic nerve injury in rats. Mol. Neurobiol. 46 (2), 265–274. doi:10.1007/s12035-012-8292-7
Xuan, A. G., Long, D. H., Gu, H. G., Yang, D. D., Hong, L. P., and Leng, S. L. (2008). BDNF improves the effects of neural stem cells on the rat model of Alzheimer's disease with unilateral lesion of fimbria-fornix. Neurosci. Lett. 440 (3), 331–335. doi:10.1016/j.neulet.2008.05.107
Xuan, A. G., Luo, M., Ji, W. D., and Long, D. H. (2009). Effects of engrafted neural stem cells in Alzheimer's disease rats. Neurosci. Lett. 450 (2), 167–171. doi:10.1016/j.neulet.2008.12.001
Xue, X., Zhang, L., Yin, X., Chen, X. X., Chen, Z. F., Wang, C. X., et al. (2020). Transplantation of neural stem cells preconditioned with high-mobility group box 1 facilitates functional recovery after spinal cord injury in rats. Mol. Med. Rep. 22 (6), 4725–4733. doi:10.3892/mmr.2020.11565
Yao, Y., Huang, C., Gu, P., and Wen, T. (2016). Combined MSC-secreted factors and neural stem cell transplantation promote functional recovery of PD rats. Cell Transpl. 25 (6), 1101–1113. doi:10.3727/096368915x689938
Yasuhara, T., Kameda, M., Agari, T., and Date, I. (2015). Regenerative medicine for Parkinson's disease. Neurol. Med. Chir. (Tokyo) 55 (2), 113–123. doi:10.2176/nmc.ra.2014-0264
Yasuhara, T., Kameda, M., Sasaki, T., Tajiri, N., and Date, I. (2017). Cell therapy for Parkinson's disease. Cell Transpl. 26 (9), 1551–1559. doi:10.1177/0963689717735411
Yoon, Y., Kim, H. S., Jeon, I., Noh, J. E., Park, H. J., Lee, S., et al. (2020). Implantation of the clinical-grade human neural stem cell line, CTX0E03, rescues the behavioral and pathological deficits in the quinolinic acid-lesioned rodent model of Huntington's disease. Stem Cells 38 (8), 936–947. doi:10.1002/stem.3191
Yu, J., Ye, K., Li, J., Wei, Y., Zhou, J., Ni, W., et al. (2022). Schwann-like cell conditioned medium promotes angiogenesis and nerve regeneration. Cell Tissue Bank. 23 (1), 101–118. doi:10.1007/s10561-021-09920-0
Yu, Z., Men, Y., and Dong, P. (2017). Schwann cells promote the capability of neural stem cells to differentiate into neurons and secret neurotrophic factors. Exp. Ther. Med. 13 (5), 2029–2035. doi:10.3892/etm.2017.4183
Zalfa, C., Rota Nodari, L., Vacchi, E., Gelati, M., Profico, D., Boido, M., et al. (2019). Transplantation of clinical-grade human neural stem cells reduces neuroinflammation, prolongs survival and delays disease progression in the SOD1 rats. Cell Death Dis. 10 (5), 345. doi:10.1038/s41419-019-1582-5
Zhang, J., and Jiao, J. (2015). Molecular biomarkers for embryonic and adult neural stem cell and neurogenesis. Biomed. Res. Int. 2015, 1–14. doi:10.1155/2015/727542
Zhang, L., Li, B., Liu, B., and Dong, Z. (2019). Co-Transplantation of epidermal neural crest stem cells and olfactory ensheathing cells repairs sciatic nerve defects in rats. Front. Cell Neurosci. 13, 253. doi:10.3389/fncel.2019.00253
Zhang, L., Ma, X. J., Fei, Y. Y., Han, H. T., Xu, J., Cheng, L., et al. (2022). Stem cell therapy in liver regeneration: focus on mesenchymal stem cells and induced pluripotent stem cells. Pharmacol. Ther. 232, 108004. doi:10.1016/j.pharmthera.2021.108004
Zhang, L. P., Liao, J. X., Liu, Y. Y., Luo, H. L., and Zhang, W. J. (2023b). Potential therapeutic effect of olfactory ensheathing cells in neurological diseases: neurodegenerative diseases and peripheral nerve injuries. Front. Immunol. 14, 1280186. doi:10.3389/fimmu.2023.1280186
Zhang, Q., Chen, W., Tan, S., and Lin, T. (2017). Stem cells for modeling and therapy of Parkinson's disease. Hum. Gene Ther. 28 (1), 85–98. doi:10.1089/hum.2016.116
Zhang, W. J., Luo, C., Huang, C., Liu, S. C., and Luo, H. L. (2021). Microencapsulated neural stem cells inhibit sciatic nerve injury-induced pain by reducing P2 × 4 receptor expression. Front. Cell Dev. Biol. 9, 656780. doi:10.3389/fcell.2021.656780
Zhang, W. J., Wu, C. L., and Liu, J. P. (2023a). Schwann cells as a target cell for the treatment of cancer pain. Glia 71 (10), 2309–2322. doi:10.1002/glia.24391
Keywords: NSCs, neurological diseases, treatment, transplantation, challenge
Citation: Yang L, Liu S-C, Liu Y-Y, Zhu F-Q, Xiong M-J, Hu D-X and Zhang W-J (2024) Therapeutic role of neural stem cells in neurological diseases. Front. Bioeng. Biotechnol. 12:1329712. doi: 10.3389/fbioe.2024.1329712
Received: 02 November 2023; Accepted: 12 February 2024;
Published: 07 March 2024.
Edited by:
Silvia Barbon, University of Padua, ItalyReviewed by:
Elham Jamshidi, Johns Hopkins University, United StatesCopyright © 2024 Yang, Liu, Liu, Zhu, Xiong, Hu and Zhang. This is an open-access article distributed under the terms of the Creative Commons Attribution License (CC BY). The use, distribution or reproduction in other forums is permitted, provided the original author(s) and the copyright owner(s) are credited and that the original publication in this journal is cited, in accordance with accepted academic practice. No use, distribution or reproduction is permitted which does not comply with these terms.
*Correspondence: Wen-Jun Zhang, bmRlZnkyMjA1N0BuY3UuZWR1LmNu; Dong-Xia Hu, aHVkb25neGlhNTQ4OUBzaW5hLmNvbQ==
Disclaimer: All claims expressed in this article are solely those of the authors and do not necessarily represent those of their affiliated organizations, or those of the publisher, the editors and the reviewers. Any product that may be evaluated in this article or claim that may be made by its manufacturer is not guaranteed or endorsed by the publisher.
Research integrity at Frontiers
Learn more about the work of our research integrity team to safeguard the quality of each article we publish.