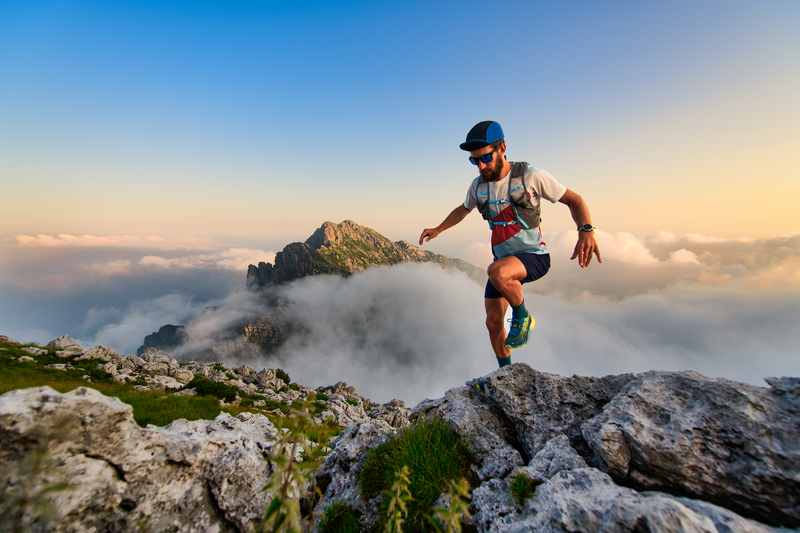
95% of researchers rate our articles as excellent or good
Learn more about the work of our research integrity team to safeguard the quality of each article we publish.
Find out more
ORIGINAL RESEARCH article
Front. Bioeng. Biotechnol. , 17 January 2023
Sec. Tissue Engineering and Regenerative Medicine
Volume 11 - 2023 | https://doi.org/10.3389/fbioe.2023.911600
This article is part of the Research Topic Biology and Clinical Applications of Adipose-Derived Cells for Skeletal Regeneration View all 5 articles
Introduction: Adipose tissue is widely exploited in regenerative medicine thanks to its trophic properties, mainly based on the presence of adipose-derived stromal cells. Numerous devices have been developed to promote its clinical use, leading to the introduction of one-step surgical procedures to obtain minimally manipulated adipose tissue derivatives. However, only a few studies compared their biological properties. This study aimed to characterize micro-fragmented (MAT) and nanofat adipose tissue (NAT) obtained with two different techniques.
Methods: MAT, NAT and unprocessed lipoaspirate were collected from surgical specimens. RNA extraction and collagenase isolation of stromal vascular fraction (SVF) were performed. Tissue sections were analysed by histological and immunohistochemical (collagen type I, CD31, CD34 and PCNA) staining to assess tissue morphology and cell content. qPCR was performed to evaluate the expression of stemness-related (SOX2, NANOG and OCT3/4), extracellular matrix (COL1A1) and inflammatory genes (IL1β, IL6 and iNOS). Furthermore, multilineage differentiation was assessed following culture in adipogenic and osteogenic media and staining with Oil Red O and Alizarin red. ASC immunophenotype was assessed by flow cytometric analysis of CD90, CD105, CD73 and CD45.
Results: Histological and immunohistochemical results showed an increased amount of stroma and a reduction of adipocytes in MAT and NAT, with the latter displaying the highest content of collagen type I, CD31, CD34 and PCNA. From LA to MAT and NAT, an increasing expression of NANOG, SOX2, OCT3/4, COL1A1 and IL6 was noted, while no significant differences in terms of IL1β and iNOS emerged. No statistically significant differences were noted between NAT and SVF in terms of stemness-related genes, while the latter demonstrated a significantly higher expression of stress-related markers. SVF cells derived from all three samples (LA, MAT, and NAT) showed a similar ASC immunoprofile as well as osteogenic and adipogenic differentiation.
Discussion: Our results showed that both MAT and NAT techniques allowed the rapid isolation of ASC-rich grafts with a high anabolic and proliferative potential. However, NAT showed the highest levels of extracellular matrix content, replicating cells, and stemness gene expression. These results may provide precious clues for the use of adipose tissue derivatives in the clinical setting.
In the last decade, mesenchymal stromal cells (MSCs) have been extensively investigated in several regenerative medicine applications for a wide variety of diseases (Vadala et al., 2007; Sowa et al., 2011; Pak et al., 2018; Viswanathan et al., 2019; Kabat et al., 2020). MSCs are non-hematopoietic cells of mesodermal origin, with stem-like potential related to self-renewal and multilineage differentiation (Vadala et al., 2008; Kulus et al., 2021). Indeed, MSCs are distributed throughout the body in almost all tissues and are mainly found in the bone marrow (BM), adipose tissue (AT) and umbilical cord (UC). Among them, AT is the most promising source due to its wide distribution, easy retrieval, minimally invasiveness of harvesting and higher number of MSC yield compared to BM (Andia et al., 2019). AT is now considered an endocrine organ capable of releasing several adipokines and is characterized by a complex biology related to the interactions between lipid-rich adipocytes and a heterogeneous population of cells composing the stromal vascular fraction (SVF). Indeed, the SVF includes preadipocytes, fibroblasts, vascular smooth muscle cells, endothelial cells, resident monocytes/macrophages, lymphocytes, and adipose-derived MSCs (ASCs) (Yoshimura et al., 2009; Sharma et al., 2021). ASCs are characterized by a high regenerative potential as reported in pre-clinical studies as well as in several clinical trials on bone and cartilage regeneration and treatment of cardiovascular, gastrointestinal and neurological disorders (Nguyen et al., 2016; Macrin et al., 2017; Bateman et al., 2018; Andia et al., 2019). Similar to MSCs, the repairing/regenerative properties of ASCs have been related to different mechanisms: differentiation towards committed resident cell phenotypes and secretion of immunomodulatory factors, cytokines, growth factors, extracellular vesicles (EVs), as well as other bioactive mediators with anti-apoptotic, antifibrotic and antioxidant effects (Wu et al., 2020; Tilotta et al., 2021; Najar et al., 2022).
The isolation of ASCs requires several steps to disaggregate and digest the tissue. The AT is usually harvested through lipoaspiration from subcutaneous depots (Di Taranto et al., 2015). Subsequent centrifugation leads to density gradient separation of the lipoaspirated material into three layers: an upper oil layer deriving from disrupted adipocytes, an intermediate tissue layer containing the lipoaspirated AT, and a lower liquid/blood fraction. Enzymatic digestion of the intermediate layer permits the isolation of the SVF and thereafter of ASCs through the selection of cells in culture by plastic adherence (Bourin et al., 2013). These procedures (centrifugation, digestion, enzyme inactivation, filtration, etc.), are time-consuming and may increase the risk of contamination due to repeated manipulation of the graft. Therefore, strict regulatory restrictions limit the exploitation of ASCs in the clinical practice.
Interestingly, lipoaspirated AT and its ASC-rich tissue-derivatives (mainly SVF) have promoted the development of several fragmentation techniques able to reduce fat processing time to obtain ASC-rich grafts through single-session surgical procedures. These devices mainly process the AT mechanically, non-enzymatically and enzymatically, and can be performed right at the patient’s bedside (Sharma et al., 2021). Nevertheless, differences in AT processing and resulting grafts lead to different products with diverse characteristics which could affect their application and outcomes in various clinical scenarios.
The aim of this study was to characterize micro-fragmented (MAT) and nanofat AT (NAT) obtained using two different techniques, and to compare these derivatives with raw lipoaspirate (LA) and collagenase-isolated cells (SVF). In particular, differences in terms of expression of stemness and inflammatory genes, surface markers specific for different cell populations and extracellular matrix (ECM) components were investigated.
All consumables were purchased from Sigma-Aldrich (Saint Louis, MO, USA) unless otherwise specified. The study was conducted in accordance with the Declaration of Helsinki, and the protocol was approved by the Ethics Committee of Campus Bio-Medico University of Rome (n. 21.19 T). All patients signed a written informed consent.
LA was obtained from 18 patients (9 males and 9 females; mean age: 63 ± 11 years; no comorbidities) undergoing intra-articular injection of AT-derivatives (mechanically processed) for treating knee osteoarthritis (OA). Briefly, through a 1-cm infraumbilical incision, approximately 200 ml of a tumescent solution (500 ml 0.9% saline, 20 ml mepivacaine 10 mg/ml, 0.7 ml 0.1% epinephrine) were infiltrated in the abdominal subcutaneous fat and liposuction was performed until obtaining approximately 50 ml of tissue. Either a mechanical micro-fragmentation method (Lipogems© Ortho Kit, Lipogems International S.p.A, Milan, Italy) or the modified nanofat method (Persichetti et al., 2017) were used to obtain AT-derivatives from LA.
MAT was obtained using the Lipogems© processing kit as per manufacturer’s instructions (Tremolada et al., 2016b). Briefly, the LA was inserted into the device through a first size reduction filter while allowing a corresponding quantity of saline to flow out toward the waste bag. Subsequently, the device was manually shaken so that stainless steel beads inside emulsified oil residues which were thus removed, together with contaminating blood components and cellular debris, by the gravity counter-flow of saline, while washed fat clusters moved to the top of the device. When the solution appeared clear, the device was turned upside-down by 180°, and a second adipose cluster reduction was obtained by passing the graft through a second-size reduction filter and pushing additional fluid from the lower opening of the device using a 10-ml syringe.
In order to obtain the AT derivatives with the modified nanofat method, the LA was aliquoted in 10 ml Luer-lock syringes and centrifuged at 1,200 g for 3 min. After this step, a 4-phase solution was obtained: the oil in the upper part and the serum in the lower part were removed, carefully keeping the adipose part and the pellet containing the SVF. The resulting tissue was emulsified through energic mix between two 10 ml Luer-lock with a 90° stopcock. 4 ml of the resulting solution were kept for the final product. The remaining material was centrifuged at 1,200 g for 3 min to obtain a solution composed of oil and a lower portion composed of a dense NAT matrix. The final solution consisted in 4 ml previously obtained and 1 ml of NAT.
For both MAT and NAT, 5 ml of final AT derivatives were used for clinical purposes. Waste aliquots obtained by micro-fragmentation (n = 6; MAT) or by modified nanofat (n = 6; NAT) and aliquots of unprocessed LA (n = 6) were used for this study.
All tissues were processed upon arrival at the laboratory as schematized in Figure 1. Briefly, the samples were equally divided into 3 different aliquots for morphological and immunohistochemical analyses, gene expression evaluation and isolation of SVF, respectively. Considering the different volume, weight and cell density of each AT derivative obtained after processing, the starting LA volume was considered to compare AT by-products among them. For example, if 5 ml MAT were obtained from 25 ml LA and 1 ml of NAT was obtained from 50 ml LA, 5 ml MAT were compared with 0.5 ml NAT.
FIGURE 1. Schematic procedure for the processing of adipose tissue (AT) from lipoaspirate (LA), micro-fragmented (MAT) and nanofat (NAT) samples. Specimens were divided into three aliquots: 3 ml for collagenase digestion; 1 ml for RNA extraction and 1 ml for paraffin embedding. Stromal vascular fraction (SVF) pellet (n = 2 for each group) was alternatively used to isolate adipose-derived stem cells (ASCs).
A part of each specimen was fixed in 10% formalin buffered solution (Bio-Optica, Milan Italy) for 2 h, paraffin-embedded and sectioned into 4 µm-slices. The sections were stained with hematoxylin-eosin (HE; Bio-Optica) following manufacturer’s instructions. Stained slides were analysed with a Nikon A1R + confocal microscope (Nikon Instruments, Tokyo, Japan). Immunohistochemistry (IHC) was performed with the STAT-QTM IHC Staining System for human and animal tissues (INNOVEX, Tokyo, Japan) following manufacturer’s instructions. Negative controls were prepared omitting the primary antibodies. Briefly, after deparaffination 4 µm-sections were incubated for 15 min with a solution of 3% hydrogen peroxide to block endogenous peroxidase activity. Slides were then incubated with primary antibodies (Table 1) for 30 min at room temperature. After washing, sections were incubated with secondary linking antibody for 10 min, washed and incubated with peroxidase (HRP) label for 10 min. After incubation with mixed DAB/substrate solution for 3 min, sections were counterstained with haematoxylin for 1 min, dehydrated and mounted with xylene based mounting media. All tests were performed on at least three sections from each specimen.
SVF was isolated from an aliquot of samples from each group (LA, MAT, NAT) through digestion as previously described (Di Taranto et al., 2015). Briefly, fat fraction was separated from each specimen, washed with phosphate-buffered saline (PBS), and then digested with 2 mg/ml collagenase (Worthington) for 40 min at 37 °C under gentle agitation. Collagenase was blocked by adding fetal bovine serum (FBS, Gibco, New York, NY, USA). The digested tissue was filtered through a 70 µm-cell strainer (Becton, Dickinson, and Company, BD, Franklin Lakes, NJ, USA) and the cell suspension was centrifuged at 300 g for 5 min. Distinct aliquots of the resulting SVF were used alternatively for RNA extraction to analyse gene expression levels (Cicione et al., 2016) or for cell culture expansion to characterize isolated ASCs (Bourin et al., 2013).
SVF-derived cells were resuspended in alpha-MEM with 15% FBS, 1% penicillin and 1% streptomycin (Gibco) and cultured in humidified atmosphere at 37 °C, with 5% CO2. After 24 h, culture medium was replaced to remove non-adherent cells and changed twice a week. Adherent cells were allowed to grow until 80%–90% of confluence and passaged up to the fourth passage. Cell pellets at passage 4 (P4) were used for the experiments.
Culture-expanded P4 ASCs were washed and analysed by flow cytometry as previously described (Cicione et al., 2013) using fluorescein isothiocyanate (FITC)- or phycoerythrin (PE)-conjugated antibodies against surface antigen markers specific for MSCs and hematopoietic stem cells (FITC-CD105, FITC-CD45, FITC-CD90, PE-CD73, BD, Table 1). A minimum of 25,000 cell events per assay were acquired using the CytoFlex (Beckman Coulter, Brea, CA, USA) and analyzed using the CytExpert Software (v.2.1, Beckman Coulter).
Adherent cells at P4 were induced toward adipogenic and osteogenic differentiation, as previously described (Cicione et al., 2016). Briefly, adipogenic medium was prepared by adding to DMEM-low glucose 10% FBS, 1 µM dexamethasone, 0.5 mM 3-isobutyl-1-methylxanthine, 10 μg/ml insulin and 100 µM indomethacin. Osteogenic medium composition was prepared by adding to DMEM-low glucose 10% FBS, 0.1 µM dexamethasone, 0.2 mM ascorbic acid 2-phosphate, and 10 mM glycerol 2-phosphate. After 21 days, cell differentiation was assessed morphologically using oil red O staining for cytoplasmic lipid droplets and alizarin red staining for mineralized matrix. Multilineage differentiation was compared with cells cultured in DMEM with 10% FBS. ImageJ software was used to quantify red colour intensity and distribution among selected pictures. Briefly, three random fields were selected from representative sections of each sample and the surface of stained matrix was compared to the whole matrix and expressed as a ratio. Sections were scored by three individuals under blinded conditions.
Total RNA was isolated from 1 ml aliquot of fresh harvested tissues (n = 7 for all three AT derivatives) and from collagenase-isolated SVF cells (n = 7). Isolation was accomplished using Trizol Reagent (Invitrogen, Waltham, MA, USA), following the manufacturer’s protocol. RNA was quantified at 260 nm using a NanoDrop™ spectrophotometer (Thermo Fisher Scientific, Waltham, MA, USA). Total RNA (1 µg) was reverse-transcribed with High-Capacity cDNA Reverse Transcription kit (Applied Biosystems, Waltham, MA, USA). cDNA samples were stored at -20 °C until used. Real-time PCR analysis (qPCR) was performed using TaqMan Gene Expression Assays listed in Table 2 and TaqMan Universal Master Mix II with UNG on a 7900HT Fast Real-Time PCR System (Thermo Fisher Scientific). Expression levels of NANOG, SOX2, OCT3/4, COL1A1, IL1β, IL6 and iNOS were investigated. GAPDH was used as a housekeeping gene to normalize the amount of target cDNAs of all other genes of interest. For each gene expression, we assigned the value 1 to the lowest level of expression and the other values were measured as relative expression levels (mRNA REL). The expression level of each target gene was calculated using the 2−ΔCt method.
Each experiment was repeated at least three times. All quantitative data are expressed as means ± standard deviations (SD). The statistical analysis of the results was performed using one-way analysis of variance (ANOVA) with Tukey post-test. Results with p < 0.05 (*), p < 0.01 (**), p < 0.001 (***) and p < 0.0001 (****) were considered statistically significant. Statistical analysis was conducted using Prism 8 (GraphPad).
Histological analysis of AT derivatives sections showed different tissue architecture and morphology. According to HE staining, only LA samples (Figure 2A) displayed a quite preserved AT morphology with a low degree of adipocyte damage confirmed by intact lobules, spherical cells and intact plasma membranes. On the other hand, MAT samples (Figure 2B) showed a lower number of intact adipocytes compared to LA samples, with increased stroma. Conversely, NAT samples (Figure 2C) almost showed the complete absence of adipocytes and the highest amount of matrix in which an elevated number of cells were dispersed. Immunohistochemistry sections of AT specimens displayed similar expression of proliferating cell nuclear antigen (PCNA), collagen type I (COL1A1), CD31 (PECAM-1) and CD34 in LA (Figures 2D–L, respectively) and MAT (Figures 2E–M, respectively) samples. Interestingly, NAT samples showed the highest amount of PCNA, COL-I, CD31 and CD34 expression (Figures 2F–N, respectively).
FIGURE 2. Histochemical and immunohistochemical analysis of lipoaspirate (LA), micro-fragmented (MAT) and nanofat (NAT) formalin-fixed paraffin-embedded sections. Images are representative for each group. (A–C): hematoxylin-eosin (HE); (D–F): Proliferating cell nuclear antigen (PCNA); (G–I): CD31 (PECAM-1); (L–N): CD34; (O–Q): Collagen type I (COLL-I). Scale bars = 250 µm.
Spindle-shaped bipolar cells were observed in the flasks after collagenase digestion in all AT derivatives under study. The only difference noticed was the starting number of attached cells which was different among MAT, NAT, and LA but without statistical significance (data not shown). After culture expansion, cells were differentiated toward adipogenic and osteogenic lineages and were analysed for the expression of surface markers (CD45, CD73, CD90, and CD105) by flow cytometry. Oil Red O and Alizarin Red staining confirmed that cells derived from the digestion of LA, MAT and NAT were able to differentiate as shown by the red lipid droplets and the red calcium depots without significant differences (Figure 3). Flow cytometric analysis confirmed the immunophenotype of ASCs with expression of CD90, CD105, CD73 cell surface antigens whereas the hematoendothelial marker CD45 was absent (Figure 4).
FIGURE 3. Multilineage differentiation assay. (A–C) Oil Red O staining of cells differentiated toward adipocytes (DM), compared to control cells cultured for the same days in DMEM with 10% FBS (Ctrl); (B–D) Alizarin Red staining of cells differentiated toward osteoblasts (DM), compared to control cells cultured for the same days in DMEM with 10% FBS (Ctrl). Scale bars = 250 µm.
FIGURE 4. Surface antigen expressions of culture expanded adipose-derived stromal cells (ASCs). (A) Control cell population; (B) Dot plot of culture expanded ASCs marked with FITC-CD105 and PE-CD73; (C) Dot-plot of culture expanded ASCs marked with FITC-CD90 and PE-CD73; (D) Histogram of culture expanded ASCs marked with FITC-Isotype Control; (E) Histogram of culture expanded ASCs marked with CD45-FITC.
To explore the molecular features underlying processing differences among the AT derivatives under study, we have comparatively analysed the expression of genes responsible for stemness maintenance (Figure 5), ECM and genes involved in inflammation and oxidative stress (Figure 6). The NANOG gene (Figure 5A) was significantly overexpressed in SVF (752.8 ± 606.1) compared to LA (45.47 ± 39.46; p = 0.021) and MAT (121.6 ± 210.Six; p = 0.044) and without statistical significance compared to NAT (326.8 ± 540.9). Expression levels of SOX2 (Figure 5B) showed a similar behaviour with an overexpression in SVF samples (13,101 ± 14,050), which was significantly different from LA (116.8 ± 107.3; p = 0.048) and MAT (184.9 ± 102.8; p = 0.049), but not statistically different from NAT (2103 ± 2469). The stemness essential transcription factor OCT3/4 (Figure 5C) was overexpressed in SVF (705.0 ± 353.0) followed by NAT (586.7 ± 442.6), both significantly different from LA (15.24 ± 13.83; p = 0.007 and p = 0.026, respectively). MAT showed an intermediate amount of OCT3/4 (129.0 ± 22.9) which was significantly different only compared to SVF (p = 0.025). In addition, COL1A1 gene expression (Figure 5D) confirmed IHC findings, showing the highest levels in NAT (3.833 ± 2.203) compared to both MAT (1.739 ± 0.1252, p = 0.042) and LA (1.504 ± 0.4850, p = 0.014), while not being significantly different from SVF (1.760 ± 0.7405). IL1β, IL6 and iNOS genes were differentially expressed in all AT derivates analysed (Figure 6). In particular, IL1β levels (Figure 6A) increased in the following order: NAT (245.5 ± 378.9), MAT (431 ± 525.4), LA (641.2 ± 1,009), and lastly SVF with the highest value (22,545 ± 11,553), significantly different from all the others (p ≤ 0.0001). On the other hand, IL6 expression was significantly higher in NAT (58.25 ± 33.28) and MAT samples (53.29 ± 22.24) compared to LA (10.26 ± 4.086; p = 0.038 and p = 0.019, respectively) and SVF (1.760 ± 0.7405, p = 0.023 and p = 0.014, respectively). Finally, iNOS expression (Figure 6B) was significantly higher in SVF (10,553 ± 6,931) compared with all other samples (LA: 45.94 ± 39.37, p = 0.0004; MAT: 1,003 ± 1,123, p = 0.002; NAT: 1,138 ± 1828, p = 0.001).
FIGURE 5. Stemness-related transcription factor and extracellular matrix gene expression in lipoaspirate (LA), micro-fragmented (MAT), nanofat (NAT) and stromal vascular fraction (SVF) samples. (A) NANOG gene expression levels; (B) SOX2 gene expression levels; (C) OCT3/4 gene expression levels; (D) COL1A1 gene expression levels. Results are expressed as relative quantity (mRNA REL) of gene levels calculated by the 2−ΔDCt method, where the value one was assigned to the lowest level of expression. *p ≤ 0.05; **p ≤ 0.01.
FIGURE 6. Stress related genes expression in lipoaspirate (LA), micro-fragmented (MAT), nanofat (NAT) and stromal vascular fraction (SVF) samples. (A) Interleukin (IL)-1β gene expression levels; (B) IL-6 gene expression levels; (C) Inducible nitric oxide synthase (iNOS) gene expression levels. Results are expressed as relative quantity (mRNA REL) of gene levels calculated by the 2−ΔDCt method, where the value one was assigned to the lowest level of expression. *p ≤ 0.05; **p ≤ 0.01; ***p ≤ 0.001; ****p ≤ 0.0001.
For a long time, AT has been mainly regarded as an inactive tissue, mainly serving as an energy storage of the fatty acids contained in mature adipocytes. However, later research demonstrated that AT is indeed an endocrine organ secreting factors involved in several biological and regulatory processes and also contains other cell types including preadipocytes, pericytes, fibroblasts, endothelial cells, hematopoietic cells, macrophages, and ASCs (Gimble et al., 2014). ASCs can be obtained through enzymatic digestion of LA samples, isolated from SVF by plastic adherence and culture expanded in vitro (Fraser et al., 2007; Lee et al., 2020; Harrison et al., 2022). Interestingly, ASCs isolated from different subcutaneous fat depots (i.e., abdomen, gluteal region, thigh) show different marker expression and multi-differentiation potential, suggesting that ASCs harvested from different anatomical niches may present unique properties (Ong et al., 2014; Tsuji, 2014; Di Taranto et al., 2015; Vadala et al., 2015). Despite this, ASCs and AT derivatives have already been successfully used in tissue engineering applications and clinical trials thanks to their regenerative potential (Zuk et al., 2001; Si et al., 2019). Indeed, the Food and Drug Administration (FDA) and the European Medicines Agency (EMA) have allowed the use of such AT derivatives for specific therapeutic purposes (Johnson et al., 2017; Raposio and Ciliberti, 2017). In order to be exploited, these AT-derivatives must not be subjected to extensive manipulations, such as collagenase digestion which could cause immune reactions in the host (Kim and Jeong, 2014), thence incompatible with the European Good Manufacturing Guidelines (eGMP) (Regulation (EC) No. 1394/2007 of the Parliament European Union and the European Council). Several devices have been developed to separate and isolate SVF from AT relying on mechanical or physical forces to manipulate the structural integrity of the AT (Oberbauer et al., 2015; Gentile et al., 2019; Rihani, 2019). The final product obtained from these procedures is not a cellular stromal vascular portion, as it occurs from enzymatic digestion, but a combination of cellular debris, blood cells and ECM components (Condé-Green et al., 2016). Among the various non-enzymatic procedures proposed, there are several operator-dependent devices and tools that mechanically dissociate the lipoaspirate (Coccè et al., 2018). Currently, the Lipogems© device is one of the most commonly used in the clinical practice. It consists of a closed device in which intraoperatively lipoaspirated fat is introduced and microfragmented. The final product is a fatty tissue reduced to microfragments (400–600 μm-wide), without oil or blood residues and rich in ASCs (Tremolada et al., 2016a). Tonnard et al. developed a subproduct of AT called “nanofat” by mechanical disintegration (emulsification and filtration), further reducing particle size and making it easily injectable (Gentile et al., 2017; Grünherz et al., 2019). This AT-derivative is devoid of mature adipocytes and contains populations of CD31+ and CD34+ stromal cells of vascular origin (derived from fragments of arterioles, venules and capillaries), growth factors and naïve cell matrix components (Lo Furno et al., 2017). Preclinical and preliminary clinical studies have demonstrated the feasibility of using nanofat for its reparative capabilities at the graft site by boosting ECM remodeling and angiogenesis, as well as modulating immune system and cell turnover (Tamburino et al., 2016; Chen et al., 2021). However, a few studies have compared the various methods used to obtain AT-derivatives from LA and their effectiveness.
In this study, we analysed the morphology of LA, MAT and NAT through HE staining, which showed that the amount of intact adipocytes drastically decreased from LA, MAT to NAT samples, respectively. Subsequently, IHC analysis showed that NAT samples were characterized by the highest expression of PCNA, COL1A1, CD31 and CD34, thus demonstrating an increase of cell proliferation, ECM anabolism and vascular marker expression, as well as a higher content of cells and ECM compared to LA and MAT samples. Moreover, we compared the expression of stemness genes NANOG, SOX2 and OCT3/4 among LA, MAT, NAT and SVF isolated after enzymatic digestion. Interestingly, these markers were significantly overexpressed in SVF compared to LA and MAT, while not being statistically different from NAT. In addition, OCT3/4 expression level was also significantly upregulated in NAT samples compared to LA. This may suggest that NAT prepared with the modified nanofat technique may produce a graft with a high regenerative potential without the need to perform additional manipulation (i.e., enzymatic digestion). Moreover, NAT also showed the highest gene expression of COL1A1, further confirming IHC findings.
Although AT is considered a structural organ, recent evidence has demonstrated its endocrine and paracrine functions exerted by the secretion anti-inflammatory and vasorelaxant adipokines (such as adiponectin, omentin, angiotensin, methyl palmitate and nitric oxide, NO), which contribute to its anti-contractile, anti-inflammatory and antiatherogenic actions (Vezzani et al., 2018; Toussirot, 2020). However, in some circumstances (e.g., obesity, metabolic syndrome) adipokines, cytokines and other factors produced and released by AT may contribute to generate a chronic inflammatory state and promote the development of hypertension, dyslipidaemia, type II diabetes and other metabolic and cardiovascular diseases (Fantuzzi, 2005). Nonetheless, mechanical manipulation of AT itself may activate oxidative stress and inflammation. Our results demonstrated that inflammatory and oxidative stress markers such as IL1β and iNOS were differentially expressed in the AT derivates under study. We found the lowest levels of IL1β and iNOS gene expression in NAT and LA, respectively, whereas in both cases SVF showed the highest values compared to all other samples. Therefore, enzymatic digestion performed to obtain SVF resulted in a consistent increase of inflammatory and oxidative stress markers, while NAT processing better maintained their levels compared to MAT. However, our findings also showed that IL6 was significantly more expressed in NAT and MAT compared to LA and SVF. Differently from IL-1β, IL-6 holds a dual biological role: while it has been traditionally involved in the inflammatory response and release of matrix-degrading enzymes, recent findings have also demonstrated that IL-6 may upregulate anti-catabolic factors, suggesting a protective role (Wiegertjes et al., 2020).
Following enzymatic digestion to obtain SVF, flow cytometric analysis and differentiation towards the adipogenic and osteogenic lineage in vitro showed comparable results in all samples derived from the three derivatives under study. This may be explained by the loss of distinct cell features following in vitro expansion.
Among the AT derivatives under investigation, NAT showed a dense ECM with a low number of adipocytes and a high content of ASCs and cells of vascular origin, with a reduced expression of pro-inflammatory and oxidative stress-related factors compared to other minimally manipulated and non-enzymatically treated AT-derivatives. Conversely, MAT was mainly composed of mature adipocytes with a lower expression of stemness-related genes, thus resembling the characteristics of LA. Furthermore, the differences in the relative amount of the different types of cells contained in the three set of samples under study may lead to different outcomes.
This study presents some limitations. The main limitation is related to patients’ variability. Indeed, we did not specifically select patients undergoing the regenerative treatment, therefore the collection of AT samples was random. In this regard, we did not evaluate if the presence of comorbidities (diabetes, hypertension, dyslipidaemia) might affect the regenerative potential of AT-derivatives in clinical practice. Furthermore, the majority of LA, MAT and NAT samples analysed did not belong to the same patients (only two LA and MAT samples were matched). However, recent evidence has demonstrated that decreased proliferation and differentiation potential in ASCs has been associated with increasing age, body mass index, diabetes mellitus, exposure to radiotherapy and Tamoxifen, while no significant influence was reported in association with gender, donor site preference, HIV status and chemotherapy. As stated above, our cohort was balanced in terms of male to female ratio (1:1), presented a similar age span, was affected by OA and not by comorbidities (including obesity), did not report a previous history of cancer and related treatments, and underwent fat grafting from the same site through the same incision (Varghese et al., 2017). Therefore, despite patient variability and the small sample size, we expect our data to be effectively representing the biological characteristics of the AT-derivatives under investigation. In addition, some inherent imprecision exists concerning AT derivatives and their nomenclature, mainly due to the wide availability of different harvesting techniques and processing. Indeed, while MAT is defined as such due to the presence of micrometric (∼300 μm) adipose clusters (Tremolada et al., 2016b), no specific component in the nanometric scale is present in NAT. The term NAT was introduced to depict the high degree of emulsification of this by-product and the possibility to inject it through finer sharp needles compared to MAT (Tonnard et al., 2013). Furthermore, the heterogeneity of quality and quantity among analysed AT-derivatives may not accurately represent the biological diversity of cells populating the tissue. Finally, as only a limited number of genes was investigated, analyses of wider inflammatory gene panel would have been needed to better define the immunomodulatory effects of AT derivatives under study.
Our results indicate that AT-derivatives differ in terms of stemness gene expression, cell content and levels of inflammatory and oxidative stress markers. Interestingly, NAT showed the highest concentration of ASCs and the lowest expression of IL1β compared to other grafts. Therefore, considering the cost-effectiveness and ease of processing characterizing the NAT here obtained, such AT-derivative may be ideal for future applications in regenerative medicine. However, further studies are needed to encourage the routine standardized use of NAT in the clinical setting.
The raw data supporting the conclusions of this article will be made available by the authors, without undue reservation.
The studies involving human participants were reviewed and approved by Ethics Committee of Campus Bio-Medico University of Rome. The patients/participants provided their written informed consent to participate in this study.
All authors contributed to the study design. FR, LA, and GV provided surgical waste specimens to perform this study. CC, GD, and VT performed the experimental part. CC performed data and statistical analysis. CC and VT wrote the first draft of the article. GD, LA, FR, and GV revised the manuscript and refined it. All authors contributed to discussions and development of this paper. All authors contributed to the interpretation of the data and the generation of the manuscript.
The authors declare that the research was conducted in the absence of any commercial or financial relationships that could be construed as a potential conflict of interest.
All claims expressed in this article are solely those of the authors and do not necessarily represent those of their affiliated organizations, or those of the publisher, the editors and the reviewers. Any product that may be evaluated in this article, or claim that may be made by its manufacturer, is not guaranteed or endorsed by the publisher.
Andia, I., Maffulli, N., and Burgos-Alonso, N. (2019). Stromal vascular fraction technologies and clinical applications. Expert Opin. Biol. Ther. 19 (12), 1289–1305. doi:10.1080/14712598.2019.1671970
Bateman, M. E., Strong, A. L., Gimble, J. M., and Bunnell, B. A. (2018). Concise review: Using fat to fight disease: A systematic review of nonhomologous adipose-derived stromal/stem cell therapies. Stem Cells 36 (9), 1311–1328. doi:10.1002/stem.2847
Bourin, P., Bunnell, B. A., Casteilla, L., Dominici, M., Katz, A. J., March, K. L., et al. (2013). Stromal cells from the adipose tissue-derived stromal vascular fraction and culture expanded adipose tissue-derived stromal/stem cells: A joint statement of the international federation for adipose therapeutics and science (IFATS) and the international society for cellular therapy (ISCT). Cytotherapy 15 (6), 641–648. doi:10.1016/j.jcyt.2013.02.006
Chen, Z., Ge, Y., Zhou, L., Li, T., Yan, B., Chen, J., et al. (2021). Pain relief and cartilage repair by nanofat against osteoarthritis: Preclinical and clinical evidence. Stem Cell Res. Ther. 12 (1), 477. doi:10.1186/s13287-021-02538-9
Cicione, C., Di Taranto, G., Barba, M., Isgrò, M. A., D’Alessio, A., Cervelli, D., et al. (2016). In vitro validation of a closed device enabling the purification of the fluid portion of liposuction aspirates. Plastic Reconstr. Surg. 137 (4), 1157–1167. doi:10.1097/prs.0000000000002014
Cicione, C., Muiños-López, E., Hermida-Gómez, T., Fuentes-Boquete, I., Díaz-Prado, S., and Blanco, F. J. (2013). Effects of severe hypoxia on bone marrow mesenchymal stem cells differentiation potential. Stem Cells Int. 2013, 1–11. doi:10.1155/2013/232896
Coccè, V., Brini, A., Giannì, A. B., Sordi, V., Berenzi, A., Alessandri, G., et al. (2018). A nonenzymatic and automated closed-cycle process for the isolation of mesenchymal stromal cells in Drug delivery applications. Stem Cells Int. 2018, 1–10. doi:10.1155/2018/4098140
Condé-Green, A., Kotamarti, V. S., Sherman, L. S., Keith, J. D., Lee, E. S., Granick, M. S., et al. (2016). Shift toward mechanical isolation of adipose-derived stromal vascular fraction. Plastic Reconstr. Surg. - Glob. Open 4 (9), e1017. doi:10.1097/gox.0000000000001017
Di Taranto, G., Cicione, C., Visconti, G., Isgrò, M. A., Barba, M., Di Stasio, E., et al. (2015). Qualitative and quantitative differences of adipose-derived stromal cells from superficial and deep subcutaneous lipoaspirates: A matter of fat. Cytotherapy 17 (8), 1076–1089. doi:10.1016/j.jcyt.2015.04.004
Fantuzzi, G. (2005). Adipose tissue, adipokines, and inflammation. J. Allergy Clin. Immunol. 115 (5), 911–919. doi:10.1016/j.jaci.2005.02.023
Fraser, J. K., Wulur, I., Alfonso, Z., Zhu, M., and Wheeler, E. S. (2007). Differences in stem and progenitor cell yield in different subcutaneous adipose tissue depots. Cytotherapy 9 (5), 459–467. doi:10.1080/14653240701358460
Gentile, P., Calabrese, C., De Angelis, B., Pizzicannella, J., Kothari, A., and Garcovich, S. (2019). Impact of the different preparation methods to obtain human adipose-derived stromal vascular fraction cells (AD-SVFs) and human adipose-derived mesenchymal stem cells (AD-MSCs): Enzymatic digestion versus mechanical centrifugation. Int. J. Mol. Sci. 20 (21), 5471. doi:10.3390/ijms20215471
Gentile, P., Scioli, M. G., Bielli, A., Orlandi, A., and Cervelli, V. (2017). Comparing different nanofat procedures on scars: Role of the stromal vascular fraction and its clinical implications. Regen. Med. 12 (8), 939–952. doi:10.2217/rme-2017-0076
Gimble, J. M., Bunnell, B. A., Frazier, T., Rowan, B., Shah, F., Thomas-Porch, C., et al. (2014). Adipose-derived stromal/stem cells. Organogenesis 9 (1), 3–10. doi:10.4161/org.24279
Grünherz, L., Sanchez-Macedo, N., Frueh, F. S., McLuckie, M., and Lindenblatt, N. (2019). Nanofat applications: From clinical esthetics to regenerative research. Curr. Opin. Biomed. Eng. 10, 174–180. doi:10.1016/j.cobme.2019.07.002
Harrison, M. A. A., Al-Ghadban, S. I., O’Donnell, B. T., Mohiuddin, O. A., Wise, R. M., Sullivan, B. N., et al. (2022). “Establishing the adipose stem cell identity: Characterization assays and functional properties,” in Scientific principles of adipose stem cells, 23–56.
Johnson, M. L., Johnson, L., Mahabir, R. C., and Bernard, R. (2017). Perspectives on the FDA draft guidances for use of adipose tissue. Aesthetic Surg. J. 37 (5), 622–625. doi:10.1093/asj/sjx049
Kabat, M., Bobkov, I., Kumar, S., and Grumet, M. (2020). Trends in mesenchymal stem cell clinical trials 2004-2018: Is efficacy optimal in a narrow dose range? Stem Cells Transl. Med. 9 (1), 17–27. doi:10.1002/sctm.19-0202
Kim, Y.-J., and Jeong, J.-H. (2014). Clinical application of adipose stem cells in plastic surgery. J. Korean Med. Sci. 29 (4), 462. doi:10.3346/jkms.2014.29.4.462
Kulus, M., Sibiak, R., Stefańska, K., Zdun, M., Wieczorkiewicz, M., Piotrowska-Kempisty, H., et al. (2021). Mesenchymal stem/stromal cells derived from human and animal perinatal tissues—origins, characteristics, signaling pathways, and clinical trials. Cells 10 (12), 3278. doi:10.3390/cells10123278
Lee, J., Lee, C. Y., Park, J.-H., Seo, H.-H., Shin, S., Song, B.-W., et al. (2020). Differentiation of adipose-derived stem cells into functional chondrocytes by a small molecule that induces Sox9. Exp. Mol. Med. 52 (4), 672–681. doi:10.1038/s12276-020-0424-y
Lo Furno, D., Tamburino, S., Mannino, G., Gilia, E., Lombardo, G., Tarico, M. S., et al. (2017). Nanofat 2.0: Experimental evidence for a fat grafting rich in mesenchymal stem cells. Physiological Res. 66, 663–671. doi:10.33549/physiolres.933451
Macrin, D., Joseph, J. P., Pillai, A. A., and Devi, A. (2017). Eminent sources of adult mesenchymal stem cells and their therapeutic imminence. Stem Cell Rev. Rep. 13 (6), 741–756. doi:10.1007/s12015-017-9759-8
Najar, M., Melki, R., Khalife, F., Lagneaux, L., Bouhtit, F., Moussa Agha, D., et al. (2022). Therapeutic mesenchymal stem/stromal cells: Value, challenges and optimization. Front. Cell Dev. Biol. 9, 716853. doi:10.3389/fcell.2021.716853
Nguyen, A., Guo, J., Banyard, D. A., Fadavi, D., Toranto, J. D., Wirth, G. A., et al. (2016). Stromal vascular fraction: A regenerative reality? Part 1: Current concepts and review of the literature. J. Plastic, Reconstr. Aesthetic Surg. 69 (2), 170–179. doi:10.1016/j.bjps.2015.10.015
Oberbauer, E., Steffenhagen, C., Wurzer, C., Gabriel, C., Redl, H., and Wolbank, S. (2015). Enzymatic and non-enzymatic isolation systems for adipose tissue-derived cells: Current state of the art. Cell Regen. 4 (1), 4–7. doi:10.1186/s13619-015-0020-0
Ong, W. K., Tan, C. S., Chan, K. L., Goesantoso, G. G., Chan, X. H. D., Chan, E., et al. (2014). Identification of specific cell-surface markers of adipose-derived stem cells from subcutaneous and visceral fat depots. Stem Cell Rep. 2 (2), 171–179. doi:10.1016/j.stemcr.2014.01.002
Pak, J., Lee, J., Pak, N., Pak, Y., Park, K., Jeon, J., et al. (2018). Cartilage regeneration in humans with adipose tissue-derived stem cells and adipose stromal vascular fraction cells: Updated status. Int. J. Mol. Sci. 19 (7), 2146. doi:10.3390/ijms19072146
Persichetti, P., Marangi, G. F., Segreto, F., Pantano, F., Tirindelli, M. C., and Gregorj, C. (2017). Adipose tissue purification technique to obtain high concentration of adipose stem cells. European Patent Office. Available at: https://worldwide.espacenet.com/patent/search/family/058779263/publication/IT201700003805A1?q=pn%3DIT201700003805A1 (E.U. Patent no. IT201700003805A1).
Raposio, E., and Ciliberti, R. (2017). Clinical use of adipose-derived stem cells: European legislative issues. Ann. Med. Surg. 24, 61–64. doi:10.1016/j.amsu.2017.11.002
Rihani, J. (2019). Microfat and nanofat. Fac. Plas. Surg. Clin. N. Am. 27 (3), 321–330. doi:10.1016/j.fsc.2019.03.004
Sharma, S., Muthu, S., Jeyaraman, M., Ranjan, R., and Jha, S. K. (2021). Translational products of adipose tissue-derived mesenchymal stem cells: Bench to bedside applications. World J. Stem Cells 13 (10), 1360–1381. doi:10.4252/wjsc.v13.i10.1360
Si, Z., Wang, X., Sun, C., Kang, Y., Xu, J., Wang, X., et al. (2019). Adipose-derived stem cells: Sources, potency, and implications for regenerative therapies. Biomed. Pharmacother. 114, 108765. doi:10.1016/j.biopha.2019.108765
Sowa, G., Westrick, E., Pacek, C., Coelho, P., Patel, D., Vadala, G., et al. (2011). In vitro and in vivo testing of a novel regulatory system for gene therapy for intervertebral disc degeneration. Spine (Phila Pa 1976) 36 (10), E623–E628. doi:10.1097/BRS.0b013e3181ed11c1
Tamburino, S., Lombardo, G. A. G., Tarico, M. S., and Perrotta, R. E. (2016). The role of nanofat grafting in vulvar lichen sclerosus: A preliminary report. Archives Plastic Surg. 43 (1), 93–95. doi:10.5999/aps.2016.43.1.93
Tilotta, V., Vadalà, G., Ambrosio, L., Russo, F., Cicione, C., Di Giacomo, G., et al. (2021). Mesenchymal stem cell-derived exosomes: The New frontier for the treatment of intervertebral disc degeneration. Appl. Sci. 11 (23), 11222. doi:10.3390/app112311222
Tonnard, P., Verpaele, A., Peeters, G., Hamdi, M., Cornelissen, M., and Declercq, H. (2013). Nanofat grafting: Basic research and clinical applications. Plast. Reconstr. Surg. 132 (4), 1017–1026. doi:10.1097/PRS.0b013e31829fe1b0
Toussirot, E. (2020). Mini-Review: The contribution of adipokines to joint inflammation in inflammatory rheumatic diseases. Front. Endocrinol. 11, 606560. doi:10.3389/fendo.2020.606560
Tremolada, C., Colombo, V., and Ventura, C. (2016a). Adipose tissue and mesenchymal stem cells: State of the art and Lipogems® Technology development. Curr. Stem Cell Rep. 2, 304–312. doi:10.1007/s40778-016-0053-5
Tremolada, C., Ricordi, C., Caplan, A. I., and Ventura, C. (2016b). Mesenchymal stem cells in Lipogems, a reverse story: From clinical practice to basic science. Methods Mol. Biol. 1416, 109–122. doi:10.1007/978-1-4939-3584-0_6
Tsuji, W. (2014). Adipose-derived stem cells: Implications in tissue regeneration. World J. Stem Cells 6 (3), 312. doi:10.4252/wjsc.v6.i3.312
Vadala, G., Di Martino, A., Tirindelli, M. C., Denaro, L., and Denaro, V. (2008). Use of autologous bone marrow cells concentrate enriched with platelet-rich fibrin on corticocancellous bone allograft for posterolateral multilevel cervical fusion. J. Tissue Eng. Regen. Med. 2 (8), 515–520. doi:10.1002/term.121
Vadala, G., Russo, F., Ambrosio, L., Di Martino, A., Papalia, R., and Denaro, V. (2015). Biotechnologies and biomaterials in spine surgery. J. Biol. Regul. Homeost. Agents 29 (4), 137–147.
Vadala, G., Sowa, G. A., and Kang, J. D. (2007). Gene therapy for disc degeneration. Expert Opin. Biol. Ther. 7 (2), 185–196. doi:10.1517/14712598.7.2.185
Varghese, J., Griffin, M., Mosahebi, A., and Butler, P. (2017). Systematic review of patient factors affecting adipose stem cell viability and function: Implications for regenerative therapy. Stem Cell Res. Ther. 8 (1), 45. doi:10.1186/s13287-017-0483-8
Vezzani, B., Shaw, I., Lesme, H., Yong, L., Khan, N., Tremolada, C., et al. (2018). Higher pericyte content and secretory activity of microfragmented human adipose tissue compared to enzymatically derived stromal vascular fraction. Stem Cells Transl. Med. 7 (12), 876–886. doi:10.1002/sctm.18-0051
Viswanathan, S., Shi, Y., Galipeau, J., Krampera, M., Leblanc, K., Martin, I., et al. (2019). Mesenchymal stem versus stromal cells: International society for cell & gene therapy (ISCT®) mesenchymal stromal cell committee position statement on nomenclature. Cytotherapy 21 (10), 1019–1024. doi:10.1016/j.jcyt.2019.08.002
Wiegertjes, R., van de Loo, F. A. J., and Blaney Davidson, E. N. (2020). A roadmap to target interleukin-6 in osteoarthritis. Rheumatology 59 (10), 2681–2694. doi:10.1093/rheumatology/keaa248
Wu, X., Jiang, J., Gu, Z., Zhang, J., Chen, Y., and Liu, X. (2020). Mesenchymal stromal cell therapies: Immunomodulatory properties and clinical progress. Stem Cell Res. Ther. 11 (1), 345. doi:10.1186/s13287-020-01855-9
Yoshimura, K., Suga, H., and Eto, H. (2009). Adipose-derived stem/progenitor cells: Roles in adipose tissue remodeling and potential use for soft tissue augmentation. Regen. Med. 4 (2), 265–273. doi:10.2217/17460751.4.2.265
Keywords: adipose tissue, mesenchymal stromal cells, cell therapy, stromal vascular fraction, regenerative medicine
Citation: Cicione C, Vadalà G, Di Giacomo G, Tilotta V, Ambrosio L, Russo F, Zampogna B, Cannata F, Papalia R and Denaro V (2023) Micro-fragmented and nanofat adipose tissue derivatives: In vitro qualitative and quantitative analysis. Front. Bioeng. Biotechnol. 11:911600. doi: 10.3389/fbioe.2023.911600
Received: 02 April 2022; Accepted: 06 January 2023;
Published: 17 January 2023.
Edited by:
Martijn van Griensven, Maastricht University, NetherlandsReviewed by:
Ramon Llull, Wake Forest University, United StatesCopyright © 2023 Cicione, Vadalà, Di Giacomo, Tilotta, Ambrosio, Russo, Zampogna, Cannata, Papalia and Denaro. This is an open-access article distributed under the terms of the Creative Commons Attribution License (CC BY). The use, distribution or reproduction in other forums is permitted, provided the original author(s) and the copyright owner(s) are credited and that the original publication in this journal is cited, in accordance with accepted academic practice. No use, distribution or reproduction is permitted which does not comply with these terms.
*Correspondence: Gianluca Vadalà, Zy52YWRhbGFAcG9saWNsaW5pY29jYW1wdXMuaXQ=
Disclaimer: All claims expressed in this article are solely those of the authors and do not necessarily represent those of their affiliated organizations, or those of the publisher, the editors and the reviewers. Any product that may be evaluated in this article or claim that may be made by its manufacturer is not guaranteed or endorsed by the publisher.
Research integrity at Frontiers
Learn more about the work of our research integrity team to safeguard the quality of each article we publish.