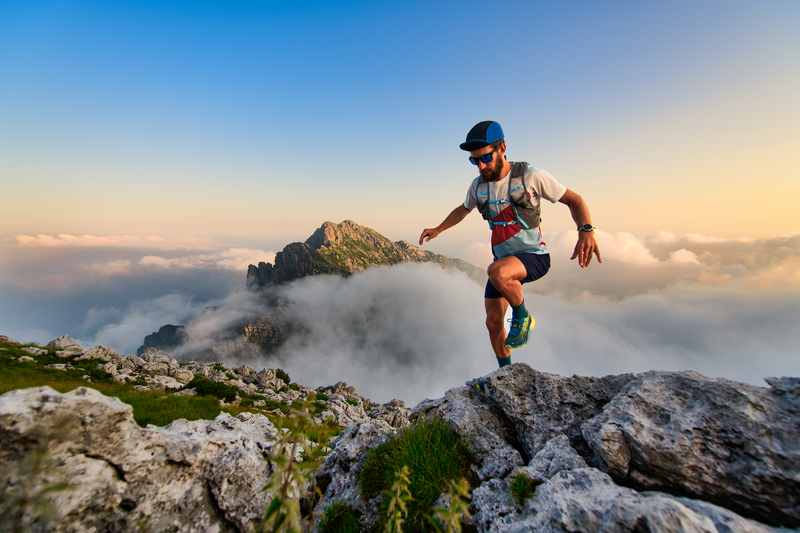
95% of researchers rate our articles as excellent or good
Learn more about the work of our research integrity team to safeguard the quality of each article we publish.
Find out more
MINI REVIEW article
Front. Bioeng. Biotechnol. , 18 January 2024
Sec. Cell and Gene Therapy
Volume 11 - 2023 | https://doi.org/10.3389/fbioe.2023.1339189
This article is part of the Research Topic CRISPR tailored gene editing – the real promise to treat human disease View all 6 articles
Over the last decade, CRISPR has revolutionized drug development due to its potential to cure genetic diseases that currently do not have any treatment. CRISPR was adapted from bacteria for gene editing in human cells in 2012 and, remarkably, only 11 years later has seen it’s very first approval as a medicine for the treatment of sickle cell disease and transfusion-dependent beta-thalassemia. However, the application of CRISPR systems is associated with unintended off-target and on-target alterations (including small indels, and structural variations such as translocations, inversions and large deletions), which are a source of risk for patients and a vital concern for the development of safe therapies. In recent years, a wide range of methods has been developed to detect unwanted effects of CRISPR-Cas nuclease activity. In this review, we summarize the different methods for off-target assessment, discuss their strengths and limitations, and highlight strategies to improve the safety of CRISPR systems. Finally, we discuss their relevance and application for the pre-clinical risk assessment of CRISPR therapeutics within the current regulatory context.
Gene editing holds great promise in treating genetic disorders that currently have no treatment available. The development of gene editing tools such as zinc finger nucleases (ZFN), transcription activator-like effector nucleases (TALENs), meganucleases and Clustered Regularly Interspaced Short Palindromic Repeat (CRISPR)-Cas systems has enabled researchers to target previously inaccessible regions of the genome. Over the last decade, CRISPR-Cas has emerged as the front runner among other gene editing modalities for use in therapeutic molecules. Indeed, at the time of revising this article, the first CRISPR-based medicine—exagamglogene autotemcel (exa-cel), an ex vivo gene edited cell therapy developed by Vertex Pharmaceuticals and CRISPR Therapeutics—had received approval for treating patients with sickle cell disease and transfusion-dependent beta-thalassemia. The success of CRISPR-Cas is linked to its relatively high efficiency in generating a variety of genomic edits, the cost-effective design of single guide RNA (sgRNA) and the ease of programming this system (Hsu et al., 2014). The most popular CRISPR system consists of the Cas9-sgRNA complex, which is able to generate specific DNA double-strand breaks (DSBs) at the target location adjacent to a protospacer-adjacent motif (PAM) (Jinek et al., 2012). This results in the activation of homology-directed repair (HDR) or non-homologous end joining (NHEJ) pathways, allowing for genome editing (Hsu et al., 2013; Mali et al., 2013). Further engineering of the Cas9 protein has enabled DSB-independent editing mechanisms, such as the use of catalytically inactive Cas9 (dCas9) in combination with effector domains to activate/repress gene expression, the use of Cas9 nickases coupled to nucleobase deaminases to produce specific nucleotide alterations via strand-biased DNA repair (base editors) and the use of Cas9 nickase coupled to a reverse transcriptase enzyme to insert sequences of interest (prime editors).
One of the major concerns in the development of CRISPR-based therapeutics is the specificity of CRISPR systems. This concern stems from the well-documented evidence that CRISPR-Cas is prone to cause unwanted DNA alterations (Hsu et al., 2013; Mali et al., 2013). These undesirable effects may be the result of on-target or off-target alterations that lead to small insertions and deletions (indels) or large structural variations (SVs). Therefore, it is critical to assess the genome-wide specificity of CRISPR tools and ensure that they are safe for therapeutic applications. In this article, we provide an overview of the methods that have been used to identify and characterize on-target and off-target effects caused by CRISPR systems. We discuss their strengths and limitations, and highlight strategies to improve the safety of CRISPR systems. Finally, we discuss their relevance and application for the pre-clinical risk assessment of CRISPR therapeutics within the current regulatory framework of the United States Food and Drug Administration (FDA) and the European Medicines Agency (EMA).
Off-target effects can be minimized effectively through the in silico prediction of CRISPR-Cas cleavage specificity and the strategic design of optimal sgRNAs. Two primary methods have been developed for predicting the specificity of CRISPR sgRNAs (Table 1). The first are alignment-based methods that employ either conventional or specialized algorithms to align sgRNAs with a given genome. As a result of this alignment, potential off-target sites and sequences are identified. This approach is mainly utilized for screening sgRNA designs and identifying all potential off-targets. The second are scoring-based methods that use complex models to score and rank sgRNAs based on the off-targets identified through the alignment process. The goal of scoring-based methods is selecting the sgRNA with the highest specificity for experimental use. Among these tools, we highlight Cas-OFFinder (Bae et al., 2014) since it is one of the most popular tools for identifying potential off-target sites. Importantly, in silico methods do not account for the intricate cellular environment and, therefore, their predictions need to be validated experimentally. For additional details, we refer to comprehensive reviews on this topic (Bao et al., 2021).
Several experimental methods have been developed to identify off-target (and on-target) edits induced by CRISPR systems. These can be broadly divided into in vitro cell-free methods and cell-based methods. More recently, methods have also been developed that can be applied in vivo in pre-clinical animal studies. Table 2 summarizes the most commonly used methods for off-target assessment as well as some relatively new methods that enable in vivo analyses. Cell-free methods such as CIRCLE-seq or SITE-seq, which are based on the use of isolated genomic DNA (gDNA), tend to be more sensitive than cell-based methods such as TTISS or IDLV and allow easy assessment of dose response. However, in vitro cell-free methods are prone to lower validation rates than cell-based methods due to the lack of chromatin context in gDNA. Indeed, chromatin structure has been shown to influence CRISPR off-targeting (Kuscu et al., 2014). Furthermore, some methods such as CHANGE-seq or GUIDE-seq are “unbiased” in that they can identify genome-wide off-targets, whereas others such as UdiTas or LAM-HTGTS require a priori knowledge of off-target sites (from in silico predictions for instance). However, these latter two techniques have the added advantage of being able to identify SVs in addition to indels induced by Cas nucleases. For detailed descriptions and comparisons of the different methods, we invite the reader to refer to Table 2 and some recent reviews (Kim et al., 2019; Atkins et al., 2021; Guo et al., 2023). Each method has its own set of advantages and limitations; hence, no single method can provide a comprehensive assessment of CRISPR-Cas associated off-targets. Developers of CRISPR therapeutics will have to use a combination of methods to assess off-target editing activity of their molecules. We further discuss off-target assessment strategies for the pre-clinical development of CRISPR therapeutics in Section 4.
The RNA-guided CRISPR-Cas9 nuclease from Streptococcus pyogenes (SpCas9) has been extensively repurposed for genome editing. Early studies revealed its high DNA cleavage activity but also associated it with increased off-target events (Hsu et al., 2013; Mali et al., 2013). To address this, SpCas9 mutants with greater specificity were developed, including enhanced SpCas9 (Slaymaker et al., 2016), high-fidelity SpCas9 (Kleinstiver et al., 2016), and hyper-accurate Cas9 (Chen et al., 2017). These variants displayed significantly fewer off-target effects than the original SpCas9. A recent innovation, SuperFi-Cas9 (Bravo et al., 2022), can discriminate between on- and off-target DNA substrates without compromising DNA cleavage, offering high fidelity. However, it exhibits relatively low on-target activity, limiting its general use in gene editing applications (Kulcsar et al., 2022). Nevertheless, in certain scenarios, SuperFi-Cas9 can complement super-active adenine base editors, counteracting mutant deaminase partners. Other lower-activity, higher-fidelity SpCas9 variants, such as HeFSpCas9 (Kulcsar et al., 2017), also enhance the precision of hyperactive editors. While high-fidelity Cas9 variants excel in specificity, they may exhibit reduced on-target activity when delivered as ribonucleoprotein (RNP) complexes, which is a therapeutically relevant formulation. In this context, HiFi Cas9 shows improved on-to-off-target ratio when delivered as an RNP, facilitating robust gene targeting for the correction of disease-causing mutations (Vakulskas et al., 2018). Finally, Cas9 nickases, which cut only one DNA strand, generate DSBs with reduced off-target effects when guided by two sgRNAs (Ran et al., 2013; Frock et al., 2015). However, challenges arise in identifying properly positioned sgRNAs due to the requirement of PAM sequences. While the diversity of genome editing tools continues to expand (Altae-Tran et al., 2021; Saito et al., 2023), complete elimination of off-target effects remains a major challenge in the field.
The design and engineering of sgRNAs are critical factors that can significantly impact the fidelity of genome editing when using CRISPR systems. Genome-wide CRISPR studies have revealed that sgRNAs targeting the same gene locus can yield different results, underscoring the importance of carefully selecting sgRNAs in silico (Shalem et al., 2014; Wang et al., 2014; Doench et al., 2016). Beyond sgRNA sequence, modifying its length can enhance Cas9 specificity. This can be done by either extending sgRNAs with the addition of two guanine nucleotides at the 5′ end (Cho et al., 2014) or by truncating sgRNAs by removing 2–3 nucleotides from the 5′ end (Fu et al., 2014). Additionally, modifications like 2′-O-methyl-3′-phosphonoacetate (Ryan et al., 2018), next-generation bridged nucleic acids (Cromwell et al., 2018) and locked nucleic acids (Cromwell et al., 2018), reduce off-target effects while maintaining on-target efficiency. However, these modifications are primarily suitable for sgRNAs delivered as a RNA molecule, but not for sgRNAs encoded as a transgene.
Cas9-mediated DSBs have been a major source of off-target effects in CRISPR-Cas9 genome editing. Emerging gene editing tools aim to sidestep DSBs, thus enhancing specificity. One such innovation is base editors (BEs), which employ Cas9 nickases fused with nucleotide deaminases to induce single nucleotide changes via single-strand breaks (SSBs). There are two primary types: cytosine base editors (CBEs) (Komor et al., 2016), that convert cytosine to thymine, and adenine base editors (ABEs) (Gaudelli et al., 2017), that convert adenine to guanine. Additionally, the development of novel BEs capable of C>G transversions have expanded their utility (Kurt et al., 2021).
Prime editing (PE) represents a groundbreaking advancement as this can accurately create various genomic alterations, including substitutions and small insertions/deletions (Anzalone et al., 2019; Chen et al., 2021; Doman et al., 2023). This technology employs a Cas9 nickase-reverse transcriptase fusion protein and an engineered prime editing guide RNA (pegRNA). Notably, PEs exhibit reduced off-target activity compared to CRISPR-Cas9, enhancing their safety for therapeutic applications, and they have shown promise in treating sickle cell disease by correcting its primary genetic cause (requiring a transversion in HBB) (Anzalone et al., 2019).
Despite BEs and PEs mitigating large-scale genomic changes linked to DSBs, they are not without off-target risks. BEs have been associated with unintended off-target effects, such as RNA off-target activity, including self-editing of BE transcripts (Grunewald et al., 2019) and sgRNA-independent DNA editing (Jin et al., 2019; Zuo et al., 2019). Off-targets effects at the RNA level are likely transient and detectable via RNA-seq, whereas sgRNA-independent editing occurs at sites with exposed single-stranded DNA and is typically assessed by methods based on whole genome sequencing (WGS) (Table 2).
An alternative strategy to minimize off-target genomic edits is through epigenetic editors. These tools, using dCas9 fused with epigenetic modifiers, can modulate endogenous gene expression (Qi et al., 2013; Chavez et al., 2015; Hilton et al., 2015; Thakore et al., 2015; Liu et al., 2016) and they have been employed successfully in vivo to treat diseases such as diabetes mellitus, muscular dystrophy, acute kidney injury, obesity, and inherited blindness (Liao et al., 2017; Kemaladewi et al., 2019; Matharu et al., 2019; Bohm et al., 2020). It is important to note that while these tools don’t induce permanent DNA changes, they can impact the epigenome, potentially affecting daughter cells. Methods such as Chromatin Immunoprecipitation (ChIP)-seq, assay for transposase-accessible chromatin (ATAC)-seq, and whole genome bisulfite sequencing (WGBS) can be used to assess epigenetic off-target effects. In summary, advancements in gene editing tools, including BEs, PEs, and epigenetic editors, aim to enhance specificity and reduce off-target effects. However, careful scrutiny and evolving detection methodologies remain essential to ensuring the safety and precision of genome editing applications.
Controlling CRISPR activity is crucial for developing safe gene-editing therapeutics. CRISPR-inhibitory molecules can serve as a failsafe mechanism to deactivate the CRISPR-Cas complex or enhance precision by decreasing off-target effects. Anti-CRISPR (Acr) proteins, initially discovered in 2013, have evolved in phages to counteract bacterial and archaeal CRISPR systems (Bondy-Denomy et al., 2013). Over 80 naturally occurring Acr proteins have been identified (Jia and Patel, 2021), and one synthetic small molecule inhibitor was discovered through high-throughput screening (Maji et al., 2019). Acr proteins can be co-expressed with Cas9 or fused directly to it, for fine-tuning its activity and improving target specificity (Aschenbrenner et al., 2020). AcrIIA4, for example, reduces off-target effects in human cells by interfering with the DNA recognition ability of Cas9 without compromising on-target gene editing (Shin et al., 2017). Acr proteins have also shown promise in attenuating RNA targeting and editing by Cas13a, thus reducing off-targeting (Lin et al., 2020), and they can suppress base editing, minimizing its off-target effects in mammalian cells (Liang et al., 2020). Innovatively, Acr proteins have been employed to enable conditional optogenetic control of Cas9. AcrIIC3-LOV2 hybrids effectively block Cas9 activity in the absence of light but permit genome editing upon light exposure in human cells (Hoffmann et al., 2021). Finally, inducible hybrids of Acr proteins and 4-hydroxytamoxifen-responsive intein enable post-translational control of CRISPR-mediated genome editing (Song et al., 2022). While these approaches hold promise for precise control of genome editing, they are not without risks, and further research is needed to rule out potential toxic effects or immune responses triggered by Acr proteins in humans.
The delivery format of Cas9, whether as DNA, mRNA, or ribonucleoprotein (RNP), plays a crucial role in determining its expression level and exposure duration, impacting both editing efficiency and off-target activity. Short-lived formats like RNP and mRNA have demonstrated lower off-target activity compared to plasmid DNA (Kim et al., 2014; Ramakrishna et al., 2014), aiming for a transient peak expression of CRISPR-Cas9 followed by rapid turnover to prevent off-target effects associated with prolonged expression (Cameron et al., 2017). In the context of in vivo genome editing, the duration of gene editor expression becomes a pivotal consideration when selecting delivery vectors. Adeno-associated virus (AAV) vectors, known for their capacity to sustain long-term gene expression, are favored for in vivo gene therapy. Self-inactivating Cas9 and AAV delivery vectors have been devised to mitigate prolonged exposure to genome editing tools (Epstein and Schaffer, 2017; Li et al., 2019; Ibraheim et al., 2021). Conversely, lipid nanoparticles (LNPs) are emerging as preferred vectors for in vivo gene editing, efficiently delivering Cas9 mRNA and sgRNA and undergoing rapid in vivo degradation, ensuring transient gene editor expression (Zuris et al., 2015; Wang et al., 2016; Finn et al., 2018). The goal of this strategy is reducing off-target risks and is currently being tested in clinical trials (Gillmore et al., 2021). Recently, it was demonstrated that shuttle peptides can be used for delivering CRISPR RNPs to mouse lung epithelial cells (Kulhankova et al., 2023). This method achieved persistent DNA editing in vivo, while showing fast cargo delivery and rapid peptide turnover. An additional layer of control is provided by regulating CRISPR-Cas expression/activity using split Cas9 (Truong et al., 2015; Zetsche et al., 2015), small chemical molecules (Gonzalez et al., 2014; Davis et al., 2015; Dow et al., 2015), light (Nihongaki et al., 2015), and magnetic nanoparticles (Zhu et al., 2019). These methods offer precise control over the timing and extent of Cas9 expression, enhancing safety and specificity, primarily within ex vivo editing workflows where high-efficiency payload delivery is feasible. Selecting the right delivery method and vector for gene editors is vital to achieve desired editing efficiency while minimizing off-target effects, particularly in therapeutic contexts emphasizing safety and precision. Ongoing research and development efforts continue to refine and enhance the delivery of CRISPR-Cas9 tools for diverse gene editing applications.
In spite of efforts to improve the specificity of Cas nucleases, off-target editing remains a risk and hence must be assessed during the pre-clinical development of CRISPR-based therapies. Given the relatively recent development of CRISPR-based therapeutics, specific regulatory guidelines on the pre-clinical assessment for this class of therapies do not as yet exist. In the United States, all genome editing medicinal products are considered cellular and gene therapy products, and hence the existing guidelines for such products are also applicable to genome editing therapies (FDA-2012-D-1038) (FDA, 2022). In the European Union, the situation is more complex. While most gene editing products would be covered by the Advanced Therapy Medicinal Products (ATMPs) definition, some could be subject to Genetically Modified Organisms (GMO) regulations and requirements, whereas others could be classified as biologicals or even small molecules (EMA/319248/2020) (Mourby and Morrison, 2020; EMA, 2021). The existing guidelines for such products also apply to genomic editing depending on how they are classified (EMA/CAT/GTWP/671639/2008, EMA/CAT/852602/2018, EMA/CAT/80183/2014) (EMA, 2021; EMA, 2019; EMA, 2018). Nevertheless, two recent reports from EMA on gene editing (EMA/319248/2020, EMA/47066/2018) and a recent update to its existing guidelines (EMA/CAT/GTWP/671639/2008 Rev1. Corr.), as well as draft guidelines being developed by the FDA for CAR-T cells (FDA-2021-D-0404) and gene editing products (FDA-2021-D-0398) can provide some insight into the types of pre-clinical off-target assessments that are expected when filing an Investigational New Drug (IND)/Investigational Medicinal Product Dossier (IMPD) application for a gene editing-based medicine (EMA, 2018; EMA, 2021; EU-IN, 2021; FDA, 2022a; FDA, 2022b). Specifically on the topic of off-target editing, regulatory agencies recognize that the existing methods for off-target detection have certain limitations with regards to their sensitivity and specificity. Hence, the use of multiple orthogonal methods, including in silico, in vitro, and cell-based approaches to assess off-targets is encouraged, and the sensitivity and specificity of these methods should be reported. Nonetheless, the use of an unbiased genome-wide method to evaluate off-targets is considered a key element. Once identified, the potential off-targets must be validated. Methods used for the verification of bona fide off-targets should be sensitive enough to detect low frequency events and should be performed in models that are predictable, for example, in the target cell-type. In order to account for natural human genetic variation which can influence off-targeting, such analyses should ideally be performed in cell lines/models from multiple donors. The importance of modelling human genetic variation when assessing off-targeting is discussed in further detail below. Owing to the recognition that Cas9-induced DSBs can generate SVs, methods that can also assess genomic integrity should be included during pre-clinical testing. The biological/physiological consequence of any off-targets identified should also be assessed as feasible, whether in relevant animal models or in vitro models. Until there is a greater standardization of methods for the measurement of off-target editing, developers of such products will have to define the strategy for off-target assessment on a case-by-case basis and to consult with the relevant health authorities at an early stage.
Pre-clinical off-target assessment data in combination with clinical data from CRISPR-based therapeutics currently can inform on the choice of the most predictive strategies to assess human off-target editing risk. However, since most CRISPR-Cas9-based therapies are still in the early stages of clinical development, pre-clinical data is often not publicly disclosed and their predictive value for patient safety is not fully known (as of the article’s revision, the public assessment reports for the approval of exa-cel by the regulatory agencies in the United States and the United Kingdom were not yet accessible). Nevertheless, a review of the published pre-clinical studies in support of ongoing clinical trials is instructive. Although different methods have been employed to assess the off-targeting potential of the molecules currently in clinical trials, a common theme is to perform the off-target assessment in two phases: a discovery phase and a validation phase. The discovery phase typically consists of in silico or unbiased experimental approaches to identify potential off-targets, whereas the validation phase confirms bona fide off-targets among either a subset or all the off-targets identified in the discovery phase in appropriate models. For example, for the molecule NTLA-2001, an SpCas9 mRNA targeting the transthyretin gene via a single sgRNA for the treatment of transthyretin amyloidosis and being developed by Intellia and Regeneron, the discovery phase consisted of three orthogonal methods—in silico off-target prediction with Cas-OFFinder, cell-based GUIDE-Seq in HEK293 cells, and the in vitro SITE-Seq assay with gDNA from human peripheral blood mononuclear cells (PBMCs) (Gillmore et al., 2021). In the validation phase, all 657 potential off-target sites identified by these three methods were then assessed using rhAMPseq (Dobosy et al., 2011) and amplicon sequencing in primary hepatocytes from two donors at dose levels 27 times the EC90 of the molecule. Of the seven off-target sites that were validated, five were intergenic and two were intronic. Further analysis in a dose-response experiment demonstrated that these off-targets were undetectable at therapeutic doses. Potential SVs introduced by Cas9 cleavage were also assessed using two independent methods—long-range PCR around the TTR locus followed by long-read PacBio sequencing as well as a bespoke SV characterization assay based on short-read sequencing (Gillmore et al., 2021). SVs were identified at low frequencies (<1%) and were considered to be of low risk. A similar approach was employed for assessing off-targeting risk for EDIT-101, a SaCas9 based molecule with 2 sgRNAs that was being developed by Editas Medicine for the treatment of Leber Congenital Amaurosis (Maeder et al., 2019). The discovery phase of the assessment consisted of three orthogonal methods—in silico with Cas-OFFinder, GUIDE-Seq in three different cell lines (U2 OS, ARPE19, and SH-SY5Y) and in primary CD4+ T cells and fibroblasts, and Digenome-seq. All 145 sites identified, most of which were identified only in silico, were then assessed in a validation phase using targeted NGS in U2 OS cells, ARPE-19 cells as well as retinal explants from 2 donors. None of the 145 off-target sites were confirmed in this verification step. Interestingly, GUIDE-seq was additionally used in a preliminary screen in U2 OS cells to identify sgRNAs without any off-targets, which may explain the lack of any validated off-targets. Furthermore, on-target editing efficiency was assessed in human photoreceptors using UdiTas (Maeder et al., 2019).
Such a two-step approach has also been used to assess off-target editing for ex vivo therapies. Indeed, for exa-cel, the discovery phase consisted of a combination of in silico assessment and GUIDE-seq in hematopoietic stem and progenitor cells (HSPCs) from 3 different donors. All 223 potential off-target sites identified by these 2 approaches were then tested by hybrid-capture followed by NGS in HSPCs from four donors, and none of the off-targets were found above the threshold of detection (Frangoul et al., 2021). Interestingly, when using a variant-aware in silico approach to assess the off-targets of the very same gRNA, Cancellieri et al. (2023) were able to identify and validate CPS1 as a bona fide off-target in individuals who were carriers of the alternative allele (C) at single nucleotide variant (SNV) rs114518452. This off-target, although identified in silico by Frangoul et al. (2021), was not confirmed in cellular assays due to the lack of representation of rs114518452-C carriers in the donor cells used for the cell-based confirmation assays. Due to the population frequency of this SNV (∼4.5% in African-American populations, and ∼0.01% in Europeans) and the higher prevalence of sickle cell disease in African-American populations, this finding and its implications for the off-targeting consequences of exa-cel were discussed in detail at a FDA Advisory Committee meeting. Although the committee concluded that the risk-benefit profile of the molecule was favorable in spite of this off-target, these discussions highlight the importance of accounting for human genetic variation when assessing off-targeting of CRISPR-based medicines and may portend closer scrutiny of the algorithms and model systems used to investigate this in the future.
In some cases, alternative strategies have also been used for off-target assessment. For example, Stadtmauer et al. (2020) assessed off-target editing in their CRISPR-engineered CAR T-cells using a single unbiased assay, iGuide, at the end of manufacturing. The authors identified a few low frequency off-target edits associated with the different sgRNAs used to knock-out the endogenous T-cell receptor genes (TRAC and TRBC) and immune checkpoint inhibitor (PD-1), yet most of the off-targets were within genes of unknown function or the disruption of whose function was not thought to impact cellular function. However, they did confirm that these edits did not lead to cellular transformation. Due to the propensity for chromosomal rearrangements in the presence of 3 independent sgRNAs, they also assessed translocations at the targeted cut sites using quantitative PCR (qPCR). Although they did identify translocations, especially between the TRAC and TRBC loci, the frequency of translocations diminished over time, suggesting that they do not confer any growth advantage to the cells (Stadtmauer et al., 2020). While there have been no reports of adverse events linked to off-target effects in ongoing clinical trials, it remains premature to ascertain the predictive value of these methods in assessing clinical risks. Indeed, long-term follow-up of patients enrolled in clinical trials will be necessary to determine the impact if any of CRISPR off-target editing on patient health.
Evaluating unintended gene modifications is a pivotal aspect of the pre-clinical development of CRISPR-Cas9-based therapies due to recognized risks tied to such events. Due to the relative novelty of this technology, the ideal screening paradigm to assess off-target editing is still undefined; however, it is likely to employ a range of complementary methods as each method is limited by its inherent sensitivity, as evidenced by certain off-target sites that were missed by other methods (Tsai et al., 2015; Kim et al., 2016; Tsai et al., 2017; Yan et al., 2017; Wienert et al., 2019). A strong collaboration between academia, industry and health authorities is vital to create and harmonize regulations that ensure the safe development of CRISPR-Cas9-based therapies. While ongoing clinical trials have not reported any adverse events linked to CRISPR off-target effects, it’s vital to acknowledge that the long-term impact of these effects on patient health may not manifest immediately. Consequently, continuous monitoring and prolonged patient follow-up are essential to comprehensively grasp the clinical implications of off-target gene editing and to refine safety assessment strategies for forthcoming genome editing-based therapies.
RL: Writing–original draft, Writing–review and editing. MP: Writing–original draft, Writing–review and editing.
The author(s) declare that no financial support was received for the research, authorship, and/or publication of this article.
The authors would like to thank Aurelia Arry for discussions around the regulatory framework, and Esther C. H. Uijttewaal and Marc Sultan for critical reading of the manuscript.
Authors RL and MKP are employed by F Hoffmann-La Roche Ltd.
All claims expressed in this article are solely those of the authors and do not necessarily represent those of their affiliated organizations, or those of the publisher, the editors and the reviewers. Any product that may be evaluated in this article, or claim that may be made by its manufacturer, is not guaranteed or endorsed by the publisher.
Akcakaya, P., Bobbin, M. L., Guo, J. A., Malagon-Lopez, J., Clement, K., Garcia, S. P., et al. (2018). In vivo CRISPR editing with no detectable genome-wide off-target mutations. Nature 561, 416–419. doi:10.1038/s41586-018-0500-9
Altae-Tran, H., Kannan, S., Demircioglu, F. E., Oshiro, R., Nety, S. P., Mckay, L. J., et al. (2021). The widespread IS200/IS605 transposon family encodes diverse programmable RNA-guided endonucleases. Science 374, 57–65. doi:10.1126/science.abj6856
Anzalone, A. V., Randolph, P. B., Davis, J. R., Sousa, A. A., Koblan, L. W., Levy, J. M., et al. (2019). Search-and-replace genome editing without double-strand breaks or donor DNA. Nature 576, 149–157. doi:10.1038/s41586-019-1711-4
Aschenbrenner, S., Kallenberger, S. M., Hoffmann, M. D., Huck, A., Eils, R., and Niopek, D. (2020). Coupling Cas9 to artificial inhibitory domains enhances CRISPR-Cas9 target specificity. Sci. Adv. 6, eaay0187. doi:10.1126/sciadv.aay0187
Atkins, A., Chung, C. H., Allen, A. G., Dampier, W., Gurrola, T. E., Sariyer, I. K., et al. (2021). Off-target analysis in gene editing and applications for clinical translation of CRISPR/Cas9 in HIV-1 therapy. Front. Genome Ed. 3, 673022. doi:10.3389/fgeed.2021.673022
Bae, S., Park, J., and Kim, J. S. (2014). Cas-OFFinder: a fast and versatile algorithm that searches for potential off-target sites of Cas9 RNA-guided endonucleases. Bioinformatics 30, 1473–1475. doi:10.1093/bioinformatics/btu048
Bao, X. R., Pan, Y., Lee, C. M., Davis, T. H., and Bao, G. (2021). Tools for experimental and computational analyses of off-target editing by programmable nucleases. Nat. Protoc. 16, 10–26. doi:10.1038/s41596-020-00431-y
Bohm, S., Splith, V., Riedmayr, L. M., Rotzer, R. D., Gasparoni, G., Nordstrom, K. J. V., et al. (2020). A gene therapy for inherited blindness using dCas9-VPR-mediated transcriptional activation. Sci. Adv. 6, eaba5614. doi:10.1126/sciadv.aba5614
Bondy-Denomy, J., Pawluk, A., Maxwell, K. L., and Davidson, A. R. (2013). Bacteriophage genes that inactivate the CRISPR/Cas bacterial immune system. Nature 493, 429–432. doi:10.1038/nature11723
Bravo, J. P. K., Liu, M. S., Hibshman, G. N., Dangerfield, T. L., Jung, K., Mccool, R. S., et al. (2022). Structural basis for mismatch surveillance by CRISPR-Cas9. Nature 603, 343–347. doi:10.1038/s41586-022-04470-1
Brinkman, E. K., Chen, T., Amendola, M., and Van Steensel, B. (2014). Easy quantitative assessment of genome editing by sequence trace decomposition. Nucleic Acids Res. 42, e168. doi:10.1093/nar/gku936
Brinkman, E. K., Kousholt, A. N., Harmsen, T., Leemans, C., Chen, T., Jonkers, J., et al. (2018). Easy quantification of template-directed CRISPR/Cas9 editing. Nucleic Acids Res. 46, e58. doi:10.1093/nar/gky164
Cameron, P., Fuller, C. K., Donohoue, P. D., Jones, B. N., Thompson, M. S., Carter, M. M., et al. (2017). Mapping the genomic landscape of CRISPR-Cas9 cleavage. Nat. Methods 14, 600–606. doi:10.1038/nmeth.4284
Cancellieri, S., Zeng, J., Lin, L. Y., Tognon, M., Nguyen, M. A., Lin, J., et al. (2023). Human genetic diversity alters off-target outcomes of therapeutic gene editing. Nat. Genet. 55, 34–43. doi:10.1038/s41588-022-01257-y
Chavez, A., Scheiman, J., Vora, S., Pruitt, B. W., Tuttle, M., Lin, S., et al. (2015). Highly efficient Cas9-mediated transcriptional programming. Nat. Methods 12, 326–328. doi:10.1038/nmeth.3312
Chen, J. S., Dagdas, Y. S., Kleinstiver, B. P., Welch, M. M., Sousa, A. A., Harrington, L. B., et al. (2017). Enhanced proofreading governs CRISPR-Cas9 targeting accuracy. Nature 550, 407–410. doi:10.1038/nature24268
Chen, P. J., Hussmann, J. A., Yan, J., Knipping, F., Ravisankar, P., Chen, P. F., et al. (2021). Enhanced prime editing systems by manipulating cellular determinants of editing outcomes. Cell 184, 5635–5652. doi:10.1016/j.cell.2021.09.018
Cho, S. W., Kim, S., Kim, Y., Kweon, J., Kim, H. S., Bae, S., et al. (2014). Analysis of off-target effects of CRISPR/Cas-derived RNA-guided endonucleases and nickases. Genome Res. 24, 132–141. doi:10.1101/gr.162339.113
Chuai, G., Ma, H., Yan, J., Chen, M., Hong, N., Xue, D., et al. (2018). DeepCRISPR: optimized CRISPR guide RNA design by deep learning. Genome Biol. 19, 80. doi:10.1186/s13059-018-1459-4
Cromwell, C. R., Sung, K., Park, J., Krysler, A. R., Jovel, J., Kim, S. K., et al. (2018). Incorporation of bridged nucleic acids into CRISPR RNAs improves Cas9 endonuclease specificity. Nat. Commun. 9, 1448. doi:10.1038/s41467-018-03927-0
Crosetto, N., Mitra, A., Silva, M. J., Bienko, M., Dojer, N., Wang, Q., et al. (2013). Nucleotide-resolution DNA double-strand break mapping by next-generation sequencing. Nat. Methods 10, 361–365. doi:10.1038/nmeth.2408
Davis, K. M., Pattanayak, V., Thompson, D. B., Zuris, J. A., and Liu, D. R. (2015). Small molecule-triggered Cas9 protein with improved genome-editing specificity. Nat. Chem. Biol. 11, 316–318. doi:10.1038/nchembio.1793
Dobosy, J. R., Rose, S. D., Beltz, K. R., Rupp, S. M., Powers, K. M., Behlke, M. A., et al. (2011). RNase H-dependent PCR (rhPCR): improved specificity and single nucleotide polymorphism detection using blocked cleavable primers. BMC Biotechnol. 11, 80. doi:10.1186/1472-6750-11-80
Doench, J. G., Fusi, N., Sullender, M., Hegde, M., Vaimberg, E. W., Donovan, K. F., et al. (2016). Optimized sgRNA design to maximize activity and minimize off-target effects of CRISPR-Cas9. Nat. Biotechnol. 34, 184–191. doi:10.1038/nbt.3437
Doman, J. L., Pandey, S., Neugebauer, M. E., An, M., Davis, J. R., Randolph, P. B., et al. (2023). Phage-assisted evolution and protein engineering yield compact, efficient prime editors. Cell 186, 3983–4002. doi:10.1016/j.cell.2023.07.039
Dow, L. E., Fisher, J., O'rourke, K. P., Muley, A., Kastenhuber, E. R., Livshits, G., et al. (2015). Inducible in vivo genome editing with CRISPR-Cas9. Nat. Biotechnol. 33, 390–394. doi:10.1038/nbt.3155
EMA (European Medicines Agency) (2018). Report of the EMA expert meeting on genome editing technologies used in medicinal product development. Available at: https://www.ema.europa.eu/en/documents/scientific-guideline/guideline-quality-non-clinical-and-clinical-aspects-medicinal-products-containing-genetically-modified-cells-revision-1_en.pdf.
EMA (European Medicines Agency) (2019), Guideline on the quality, non-clinical and clinical aspects of gene therapy medicinal products in clinical trials. Published 2019 (Draft). Available at: https://www.ema.europa.eu/en/documents/scientific-guideline/draft-guideline-quality-non-clinical-and-clinical-requirements-investigational-advanced-therapy-medicinal-products-clinical-trials_en.pdf.
EMA (European Medicines Agency (2021). Guideline on quality, non-clinical and clinical aspects of medicinal products containing genetically modified cells. Available at: https://www.ema.europa.eu/en/documents/scientific-guideline/guideline-quality-non-clinical-and-clinical-aspects-medicinal-products-containing-genetically-modified-cells-revision-1_en.pdf.
EMA (2021). Genome editing EU-IN horizon scanning report. Available at: https://www.ema.europa.eu/en/documents/report/genome-editing-eu-horizon-scanning-report_en.pdf
Epstein, B. E., and Schaffer, D. V. (2017). Combining engineered nucleases with adeno-associated viral vectors for therapeutic gene editing. Adv. Exp. Med. Biol. 1016, 29–42. doi:10.1007/978-3-319-63904-8_2
European Medicines Agency (2018). Guideline on quality, non-clinical and clinical requirements for investigational advanced therapy medicinal products in clinical trials. Available at: https://www.ema.europa.eu/en/documents/scientific-guideline/guideline-quality-non-clinical-and-clinical-aspects-gene-therapy-medicinal-products_en.pdf.
FDA (2022). Draft Guidance for industry, Human Gene Therapy Products Incorporating Human Genome Editing. Published 2022. Available at: https://www.fda.gov/media/156894/download.
FDA (U.S. Food and Drug Administration) (2022a). Draft Guidance for industry, Considerations for the development of chimeric antigen receptor (CAR) T cell products. Available at: https://www.fda.gov/media/156896/download
FDA (U.S. Food and Drug Administration) (2022b). Draft Guidance for industry, Human gene therapy products incorporating human genome editing. Available at: https://www.fda.gov/media/156894/download.
Finn, J. D., Smith, A. R., Patel, M. C., Shaw, L., Youniss, M. R., Van Heteren, J., et al. (2018). A single administration of CRISPR/Cas9 lipid nanoparticles achieves robust and persistent in vivo genome editing. Cell Rep. 22, 2227–2235. doi:10.1016/j.celrep.2018.02.014
Frangoul, H., Altshuler, D., Cappellini, M. D., Chen, Y. S., Domm, J., Eustace, B. K., et al. (2021). CRISPR-Cas9 gene editing for sickle cell disease and β-thalassemia. N. Engl. J. Med. 384, 252–260. doi:10.1056/nejmoa2031054
Frock, R. L., Hu, J., Meyers, R. M., Ho, Y. J., Kii, E., and Alt, F. W. (2015). Genome-wide detection of DNA double-stranded breaks induced by engineered nucleases. Nat. Biotechnol. 33, 179–186. doi:10.1038/nbt.3101
Fu, Y., Sander, J. D., Reyon, D., Cascio, V. M., and Joung, J. K. (2014). Improving CRISPR-Cas nuclease specificity using truncated guide RNAs. Nat. Biotechnol. 32, 279–284. doi:10.1038/nbt.2808
Gabriel, R., Lombardo, A., Arens, A., Miller, J. C., Genovese, P., Kaeppel, C., et al. (2011). An unbiased genome-wide analysis of zinc-finger nuclease specificity. Nat. Biotechnol. 29, 816–823. doi:10.1038/nbt.1948
Gaudelli, N. M., Komor, A. C., Rees, H. A., Packer, M. S., Badran, A. H., Bryson, D. I., et al. (2017). Programmable base editing of A*T to G*C in genomic DNA without DNA cleavage. Nature 551, 464–471. doi:10.1038/nature24644
Giannoukos, G., Ciulla, D. M., Marco, E., Abdulkerim, H. S., Barrera, L. A., Bothmer, A., et al. (2018). UDiTaS, a genome editing detection method for indels and genome rearrangements. BMC Genomics 19, 212. doi:10.1186/s12864-018-4561-9
Gillmore, J. D., Gane, E., Taubel, J., Kao, J., Fontana, M., Maitland, M. L., et al. (2021). CRISPR-Cas9 in vivo gene editing for transthyretin amyloidosis. N. Engl. J. Med. 385, 493–502. doi:10.1056/nejmoa2107454
Gonzalez, F., Zhu, Z., Shi, Z. D., Lelli, K., Verma, N., Li, Q. V., et al. (2014). An iCRISPR platform for rapid, multiplexable, and inducible genome editing in human pluripotent stem cells. Cell Stem Cell 15, 215–226. doi:10.1016/j.stem.2014.05.018
Grunewald, J., Zhou, R., Iyer, S., Lareau, C. A., Garcia, S. P., Aryee, M. J., et al. (2019). CRISPR DNA base editors with reduced RNA off-target and self-editing activities. Nat. Biotechnol. 37, 1041–1048. doi:10.1038/s41587-019-0236-6
Guo, C., Ma, X., Gao, F., and Guo, Y. (2023). Off-target effects in CRISPR/Cas9 gene editing. Front. Bioeng. Biotechnol. 11, 1143157. doi:10.3389/fbioe.2023.1143157
Guschin, D. Y., Waite, A. J., Katibah, G. E., Miller, J. C., Holmes, M. C., and Rebar, E. J. (2010). A rapid and general assay for monitoring endogenous gene modification. Methods Mol. Biol. 649, 247–256. doi:10.1007/978-1-60761-753-2_15
Haeussler, M., Schonig, K., Eckert, H., Eschstruth, A., Mianne, J., Renaud, J. B., et al. (2016). Evaluation of off-target and on-target scoring algorithms and integration into the guide RNA selection tool CRISPOR. Genome Biol. 17, 148. doi:10.1186/s13059-016-1012-2
Hilton, I. B., D'ippolito, A. M., Vockley, C. M., Thakore, P. I., Crawford, G. E., Reddy, T. E., et al. (2015). Epigenome editing by a CRISPR-Cas9-based acetyltransferase activates genes from promoters and enhancers. Nat. Biotechnol. 33, 510–517. doi:10.1038/nbt.3199
Hoffmann, M. D., Mathony, J., Upmeier Zu Belzen, J., Harteveld, Z., Aschenbrenner, S., Stengl, C., et al. (2021). Optogenetic control of Neisseria meningitidis Cas9 genome editing using an engineered, light-switchable anti-CRISPR protein. Nucleic Acids Res. 49, e29. doi:10.1093/nar/gkaa1198
Hsu, P. D., Lander, E. S., and Zhang, F. (2014). Development and applications of CRISPR-Cas9 for genome engineering. Cell 157, 1262–1278. doi:10.1016/j.cell.2014.05.010
Hsu, P. D., Scott, D. A., Weinstein, J. A., Ran, F. A., Konermann, S., Agarwala, V., et al. (2013). DNA targeting specificity of RNA-guided Cas9 nucleases. Nat. Biotechnol. 31, 827–832. doi:10.1038/nbt.2647
Ibraheim, R., Tai, P. W. L., Mir, A., Javeed, N., Wang, J., Rodriguez, T. C., et al. (2021). Self-inactivating, all-in-one AAV vectors for precision Cas9 genome editing via homology-directed repair in vivo. Nat. Commun. 12, 6267. doi:10.1038/s41467-021-26518-y
Iyer, V., Shen, B., Zhang, W., Hodgkins, A., Keane, T., Huang, X., et al. (2015). Off-target mutations are rare in Cas9-modified mice. Nat. Methods 12, 479. doi:10.1038/nmeth.3408
Jacquin, A. L. S., Odom, D. T., and Lukk, M. (2019). Crisflash: open-source software to generate CRISPR guide RNAs against genomes annotated with individual variation. Bioinformatics 35, 3146–3147. doi:10.1093/bioinformatics/btz019
Jia, N., and Patel, D. J. (2021). Structure-based functional mechanisms and biotechnology applications of anti-CRISPR proteins. Nat. Rev. Mol. Cell Biol. 22, 563–579. doi:10.1038/s41580-021-00371-9
Jin, S., Zong, Y., Gao, Q., Zhu, Z., Wang, Y., Qin, P., et al. (2019). Cytosine, but not adenine, base editors induce genome-wide off-target mutations in rice. Science 364, 292–295. doi:10.1126/science.aaw7166
Jinek, M., Chylinski, K., Fonfara, I., Hauer, M., Doudna, J. A., and Charpentier, E. (2012). A programmable dual-RNA-guided DNA endonuclease in adaptive bacterial immunity. Science 337, 816–821. doi:10.1126/science.1225829
Kemaladewi, D. U., Bassi, P. S., Erwood, S., Al-Basha, D., Gawlik, K. I., Lindsay, K., et al. (2019). A mutation-independent approach for muscular dystrophy via upregulation of a modifier gene. Nature 572, 125–130. doi:10.1038/s41586-019-1430-x
Kim, D., Bae, S., Park, J., Kim, E., Kim, S., Yu, H. R., et al. (2015). Digenome-seq: genome-wide profiling of CRISPR-Cas9 off-target effects in human cells. Nat. Methods 12, 237–243. doi:10.1038/nmeth.3284
Kim, D., and Kim, J. S. (2018). DIG-seq: a genome-wide CRISPR off-target profiling method using chromatin DNA. Genome Res. 28, 1894–1900. doi:10.1101/gr.236620.118
Kim, D., Kim, S., Kim, S., Park, J., and Kim, J. S. (2016). Genome-wide target specificities of CRISPR-Cas9 nucleases revealed by multiplex Digenome-seq. Genome Res. 26, 406–415. doi:10.1101/gr.199588.115
Kim, D., Luk, K., Wolfe, S. A., and Kim, J. S. (2019). Evaluating and enhancing target specificity of gene-editing nucleases and deaminases. Annu. Rev. Biochem. 88, 191–220. doi:10.1146/annurev-biochem-013118-111730
Kim, D. Y., Moon, S. B., Ko, J. H., Kim, Y. S., and Kim, D. (2020). Unbiased investigation of specificities of prime editing systems in human cells. Nucleic Acids Res. 48, 10576–10589. doi:10.1093/nar/gkaa764
Kim, S., Kim, D., Cho, S. W., Kim, J., and Kim, J. S. (2014). Highly efficient RNA-guided genome editing in human cells via delivery of purified Cas9 ribonucleoproteins. Genome Res. 24, 1012–1019. doi:10.1101/gr.171322.113
Kleinstiver, B. P., Pattanayak, V., Prew, M. S., Tsai, S. Q., Nguyen, N. T., Zheng, Z., et al. (2016). High-fidelity CRISPR-Cas9 nucleases with no detectable genome-wide off-target effects. Nature 529, 490–495. doi:10.1038/nature16526
Komor, A. C., Kim, Y. B., Packer, M. S., Zuris, J. A., and Liu, D. R. (2016). Programmable editing of a target base in genomic DNA without double-stranded DNA cleavage. Nature 533, 420–424. doi:10.1038/nature17946
Kulcsar, P. I., Talas, A., Huszar, K., Ligeti, Z., Toth, E., Weinhardt, N., et al. (2017). Crossing enhanced and high fidelity SpCas9 nucleases to optimize specificity and cleavage. Genome Biol. 18, 190. doi:10.1186/s13059-017-1318-8
Kulcsar, P. I., Talas, A., Ligeti, Z., Krausz, S. L., and Welker, E. (2022). SuperFi-Cas9 exhibits remarkable fidelity but severely reduced activity yet works effectively with ABE8e. Nat. Commun. 13, 6858. doi:10.1038/s41467-022-34527-8
Kulhankova, K., Traore, S., Cheng, X., Benk-Fortin, H., Hallee, S., Harvey, M., et al. (2023). Shuttle peptide delivers base editor RNPs to rhesus monkey airway epithelial cells in vivo. Nat. Commun. 14, 8051. doi:10.1038/s41467-023-43904-w
Kurt, I. C., Zhou, R., Iyer, S., Garcia, S. P., Miller, B. R., Langner, L. M., et al. (2021). CRISPR C-to-G base editors for inducing targeted DNA transversions in human cells. Nat. Biotechnol. 39, 41–46. doi:10.1038/s41587-020-0609-x
Kuscu, C., Arslan, S., Singh, R., Thorpe, J., and Adli, M. (2014). Genome-wide analysis reveals characteristics of off-target sites bound by the Cas9 endonuclease. Nat. Biotechnol. 32, 677–683. doi:10.1038/nbt.2916
Kwon, J., Kim, M., Hwang, W., Jo, A., Hwang, G. H., Jung, M., et al. (2023). Extru-seq: a method for predicting genome-wide Cas9 off-target sites with advantages of both cell-based and in vitro approaches. Genome Biol. 24, 4. doi:10.1186/s13059-022-02842-4
Lazzarotto, C. R., Malinin, N. L., Li, Y., Zhang, R., Yang, Y., Lee, G., et al. (2020). CHANGE-seq reveals genetic and epigenetic effects on CRISPR-Cas9 genome-wide activity. Nat. Biotechnol. 38, 1317–1327. doi:10.1038/s41587-020-0555-7
Lei, Z., Meng, H., Lv, Z., Liu, M., Zhao, H., Wu, H., et al. (2021). Detect-seq reveals out-of-protospacer editing and target-strand editing by cytosine base editors. Nat. Methods 18, 643–651. doi:10.1038/s41592-021-01172-w
Li, A., Lee, C. M., Hurley, A. E., Jarrett, K. E., De Giorgi, M., Lu, W., et al. (2019). A self-deleting AAV-CRISPR system for in vivo genome editing. Mol. Ther. Methods Clin. Dev. 12, 111–122. doi:10.1016/j.omtm.2018.11.009
Liang, M., Sui, T., Liu, Z., Chen, M., Liu, H., Shan, H., et al. (2020). AcrIIA5 suppresses base editors and reduces their off-target effects. Cells 9, 1786. doi:10.3390/cells9081786
Liang, P., Xie, X., Zhi, S., Sun, H., Zhang, X., Chen, Y., et al. (2019). Genome-wide profiling of adenine base editor specificity by EndoV-seq. Nat. Commun. 10, 67. doi:10.1038/s41467-018-07988-z
Liang, S. Q., Liu, P., Ponnienselvan, K., Suresh, S., Chen, Z., Kramme, C., et al. (2023). Genome-wide profiling of prime editor off-target sites in vitro and in vivo using PE-tag. Nat. Methods 20, 898–907. doi:10.1038/s41592-023-01859-2
Liang, S. Q., Liu, P., Smith, J. L., Mintzer, E., Maitland, S., Dong, X., et al. (2022). Genome-wide detection of CRISPR editing in vivo using GUIDE-tag. Nat. Commun. 13, 437. doi:10.1038/s41467-022-28135-9
Liao, H. K., Hatanaka, F., Araoka, T., Reddy, P., Wu, M. Z., Sui, Y., et al. (2017). In Vivo target gene activation via CRISPR/Cas9-Mediated trans-epigenetic modulation. Cell 171, 1495–1507. doi:10.1016/j.cell.2017.10.025
Lin, P., Qin, S., Pu, Q., Wang, Z., Wu, Q., Gao, P., et al. (2020). CRISPR-Cas13 inhibitors block RNA editing in bacteria and mammalian cells. Mol. Cell 78, 850–861.e5. doi:10.1016/j.molcel.2020.03.033
Liu, X. S., Wu, H., Ji, X., Stelzer, Y., Wu, X., Czauderna, S., et al. (2016). Editing DNA methylation in the mammalian genome. Cell 167, 233–247.e17. doi:10.1016/j.cell.2016.08.056
Maeder, M. L., Stefanidakis, M., Wilson, C. J., Baral, R., Barrera, L. A., Bounoutas, G. S., et al. (2019). Development of a gene-editing approach to restore vision loss in Leber congenital amaurosis type 10. Nat. Med. 25, 229–233. doi:10.1038/s41591-018-0327-9
Maji, B., Gangopadhyay, S. A., Lee, M., Shi, M., Wu, P., Heler, R., et al. (2019). A high-throughput platform to identify small-molecule inhibitors of CRISPR-cas9. Cell 177, 1067–1079. doi:10.1016/j.cell.2019.04.009
Mali, P., Yang, L., Esvelt, K. M., Aach, J., Guell, M., Dicarlo, J. E., et al. (2013). RNA-guided human genome engineering via Cas9. Science 339, 823–826. doi:10.1126/science.1232033
Mashal, R. D., Koontz, J., and Sklar, J. (1995). Detection of mutations by cleavage of DNA heteroduplexes with bacteriophage resolvases. Nat. Genet. 9, 177–183. doi:10.1038/ng0295-177
Matharu, N., Rattanasopha, S., Tamura, S., Maliskova, L., Wang, Y., Bernard, A., et al. (2019). CRISPR-mediated activation of a promoter or enhancer rescues obesity caused by haploinsufficiency. Science 363, eaau0629. doi:10.1126/science.aau0629
Mckenna, A., and Shendure, J. (2018). FlashFry: a fast and flexible tool for large-scale CRISPR target design. BMC Biol. 16, 74. doi:10.1186/s12915-018-0545-0
Mourby, M., and Morrison, M. (2020). Gene therapy regulation: could in-body editing fall through the net? Eur. J. Hum. Genet. 28, 979–981. doi:10.1038/s41431-020-0607-y
Nihongaki, Y., Kawano, F., Nakajima, T., and Sato, M. (2015). Photoactivatable CRISPR-Cas9 for optogenetic genome editing. Nat. Biotechnol. 33, 755–760. doi:10.1038/nbt.3245
Nobles, C. L., Reddy, S., Salas-Mckee, J., Liu, X., June, C. H., Melenhorst, J. J., et al. (2019). iGUIDE: an improved pipeline for analyzing CRISPR cleavage specificity. Genome Biol. 20, 14. doi:10.1186/s13059-019-1625-3
Pan, X., Qu, K., Yuan, H., Xiang, X., Anthon, C., Pashkova, L., et al. (2022). Massively targeted evaluation of therapeutic CRISPR off-targets in cells. Nat. Commun. 13, 4049. doi:10.1038/s41467-022-31543-6
Qi, L. S., Larson, M. H., Gilbert, L. A., Doudna, J. A., Weissman, J. S., Arkin, A. P., et al. (2013). Repurposing CRISPR as an RNA-guided platform for sequence-specific control of gene expression. Cell 152, 1173–1183. doi:10.1016/j.cell.2013.02.022
Ramakrishna, S., Kwaku Dad, A. B., Beloor, J., Gopalappa, R., Lee, S. K., and Kim, H. (2014). Gene disruption by cell-penetrating peptide-mediated delivery of Cas9 protein and guide RNA. Genome Res. 24, 1020–1027. doi:10.1101/gr.171264.113
Ran, F. A., Hsu, P. D., Lin, C. Y., Gootenberg, J. S., Konermann, S., Trevino, A. E., et al. (2013). Double nicking by RNA-guided CRISPR Cas9 for enhanced genome editing specificity. Cell 154, 1380–1389. doi:10.1016/j.cell.2013.08.021
Ryan, D. E., Taussig, D., Steinfeld, I., Phadnis, S. M., Lunstad, B. D., Singh, M., et al. (2018). Improving CRISPR-Cas specificity with chemical modifications in single-guide RNAs. Nucleic Acids Res. 46, 792–803. doi:10.1093/nar/gkx1199
Saito, M., Xu, P., Faure, G., Maguire, S., Kannan, S., Altae-Tran, H., et al. (2023). Fanzor is a eukaryotic programmable RNA-guided endonuclease. Nature 620, 660–668. doi:10.1038/s41586-023-06356-2
Schmid-Burgk, J. L., Gao, L., Li, D., Gardner, Z., Strecker, J., Lash, B., et al. (2020). Highly parallel profiling of Cas9 variant specificity. Mol. Cell 78, 794–800.e8. doi:10.1016/j.molcel.2020.02.023
Shalem, O., Sanjana, N. E., Hartenian, E., Shi, X., Scott, D. A., Mikkelson, T., et al. (2014). Genome-scale CRISPR-Cas9 knockout screening in human cells. Science 343, 84–87. doi:10.1126/science.1247005
Shin, J., Jiang, F., Liu, J. J., Bray, N. L., Rauch, B. J., Baik, S. H., et al. (2017). Disabling Cas9 by an anti-CRISPR DNA mimic. Sci. Adv. 3, e1701620. doi:10.1126/sciadv.1701620
Singh, R., Kuscu, C., Quinlan, A., Qi, Y., and Adli, M. (2015). Cas9-chromatin binding information enables more accurate CRISPR off-target prediction. Nucleic Acids Res. 43, e118. doi:10.1093/nar/gkv575
Slaymaker, I. M., Gao, L., Zetsche, B., Scott, D. A., Yan, W. X., and Zhang, F. (2016). Rationally engineered Cas9 nucleases with improved specificity. Science 351, 84–88. doi:10.1126/science.aad5227
Smith, C., Gore, A., Yan, W., Abalde-Atristain, L., Li, Z., He, C., et al. (2014). Whole-genome sequencing analysis reveals high specificity of CRISPR/Cas9 and TALEN-based genome editing in human iPSCs. Cell Stem Cell 15, 12–13. doi:10.1016/j.stem.2014.06.011
Song, G., Zhang, F., Tian, C., Gao, X., Zhu, X., Fan, D., et al. (2022). Discovery of potent and versatile CRISPR-Cas9 inhibitors engineered for chemically controllable genome editing. Nucleic Acids Res. 50, 2836–2853. doi:10.1093/nar/gkac099
Stadtmauer, E. A., Fraietta, J. A., Davis, M. M., Cohen, A. D., Weber, K. L., Lancaster, E., et al. (2020). CRISPR-engineered T cells in patients with refractory cancer. Science 367, eaba7365. doi:10.1126/science.aba7365
Thakore, P. I., D'ippolito, A. M., Song, L., Safi, A., Shivakumar, N. K., Kabadi, A. M., et al. (2015). Highly specific epigenome editing by CRISPR-Cas9 repressors for silencing of distal regulatory elements. Nat. Methods 12, 1143–1149. doi:10.1038/nmeth.3630
Truong, D. J., Kuhner, K., Kuhn, R., Werfel, S., Engelhardt, S., Wurst, W., et al. (2015). Development of an intein-mediated split-Cas9 system for gene therapy. Nucleic Acids Res. 43, 6450–6458. doi:10.1093/nar/gkv601
Tsai, S. Q., Nguyen, N. T., Malagon-Lopez, J., Topkar, V. V., Aryee, M. J., and Joung, J. K. (2017). CIRCLE-seq: a highly sensitive in vitro screen for genome-wide CRISPR-Cas9 nuclease off-targets. Nat. Methods 14, 607–614. doi:10.1038/nmeth.4278
Tsai, S. Q., Zheng, Z., Nguyen, N. T., Liebers, M., Topkar, V. V., Thapar, V., et al. (2015). GUIDE-seq enables genome-wide profiling of off-target cleavage by CRISPR-Cas nucleases. Nat. Biotechnol. 33, 187–197. doi:10.1038/nbt.3117
Turchiano, G., Andrieux, G., Klermund, J., Blattner, G., Pennucci, V., El Gaz, M., et al. (2021). Quantitative evaluation of chromosomal rearrangements in gene-edited human stem cells by CAST-Seq. Cell Stem Cell 28, 1136–1147.e5. doi:10.1016/j.stem.2021.02.002
Vakulskas, C. A., Dever, D. P., Rettig, G. R., Turk, R., Jacobi, A. M., Collingwood, M. A., et al. (2018). A high-fidelity Cas9 mutant delivered as a ribonucleoprotein complex enables efficient gene editing in human hematopoietic stem and progenitor cells. Nat. Med. 24, 1216–1224. doi:10.1038/s41591-018-0137-0
Veres, A., Gosis, B. S., Ding, Q., Collins, R., Ragavendran, A., Brand, H., et al. (2014). Low incidence of off-target mutations in individual CRISPR-Cas9 and TALEN targeted human stem cell clones detected by whole-genome sequencing. Cell Stem Cell 15, 254–330. doi:10.1016/j.stem.2014.07.009
Wang, M., Zuris, J. A., Meng, F., Rees, H., Sun, S., Deng, P., et al. (2016). Efficient delivery of genome-editing proteins using bioreducible lipid nanoparticles. Proc. Natl. Acad. Sci. U. S. A. 113, 2868–2873. doi:10.1073/pnas.1520244113
Wang, T., Wei, J. J., Sabatini, D. M., and Lander, E. S. (2014). Genetic screens in human cells using the CRISPR-Cas9 system. Science 343, 80–84. doi:10.1126/science.1246981
Wang, X., Wang, Y., Wu, X., Wang, J., Wang, Y., Qiu, Z., et al. (2015). Unbiased detection of off-target cleavage by CRISPR-Cas9 and TALENs using integrase-defective lentiviral vectors. Nat. Biotechnol. 33, 175–178. doi:10.1038/nbt.3127
Wienert, B., Wyman, S. K., Richardson, C. D., Yeh, C. D., Akcakaya, P., Porritt, M. J., et al. (2019). Unbiased detection of CRISPR off-targets in vivo using DISCOVER-Seq. Science 364, 286–289. doi:10.1126/science.aav9023
Xiao, A., Cheng, Z., Kong, L., Zhu, Z., Lin, S., Gao, G., et al. (2014). CasOT: a genome-wide Cas9/gRNA off-target searching tool. Bioinformatics 30, 1180–1182. doi:10.1093/bioinformatics/btt764
Yan, W. X., Mirzazadeh, R., Garnerone, S., Scott, D., Schneider, M. W., Kallas, T., et al. (2017). BLISS is a versatile and quantitative method for genome-wide profiling of DNA double-strand breaks. Nat. Commun. 8, 15058. doi:10.1038/ncomms15058
Zetsche, B., Volz, S. E., and Zhang, F. (2015). A split-Cas9 architecture for inducible genome editing and transcription modulation. Nat. Biotechnol. 33, 139–142. doi:10.1038/nbt.3149
Zhu, H., Zhang, L., Tong, S., Lee, C. M., Deshmukh, H., and Bao, G. (2019). Spatial control of in vivo CRISPR-Cas9 genome editing via nanomagnets. Nat. Biomed. Eng. 3, 126–136. doi:10.1038/s41551-018-0318-7
Zuo, E., Sun, Y., Wei, W., Yuan, T., Ying, W., Sun, H., et al. (2019). Cytosine base editor generates substantial off-target single-nucleotide variants in mouse embryos. Science 364, 289–292. doi:10.1126/science.aav9973
Keywords: gene editing, CRISPR-Cas, off-target activity, safety, pre-clinical development, regulatory guideline, health authority
Citation: Lopes R and Prasad MK (2024) Beyond the promise: evaluating and mitigating off-target effects in CRISPR gene editing for safer therapeutics. Front. Bioeng. Biotechnol. 11:1339189. doi: 10.3389/fbioe.2023.1339189
Received: 15 November 2023; Accepted: 29 December 2023;
Published: 18 January 2024.
Edited by:
Joana Caldeira, Universidade do Porto, PortugalReviewed by:
Mario Andrea Marchisio, Tianjin University, ChinaCopyright © 2024 Lopes and Prasad. This is an open-access article distributed under the terms of the Creative Commons Attribution License (CC BY). The use, distribution or reproduction in other forums is permitted, provided the original author(s) and the copyright owner(s) are credited and that the original publication in this journal is cited, in accordance with accepted academic practice. No use, distribution or reproduction is permitted which does not comply with these terms.
*Correspondence: Rui Lopes, cnVpLmxvcGVzQHJvY2hlLmNvbQ==; Megana K. Prasad, bWVnYW5hLnByYXNhZEByb2NoZS5jb20=
†These authors have contributed equally to this work
Disclaimer: All claims expressed in this article are solely those of the authors and do not necessarily represent those of their affiliated organizations, or those of the publisher, the editors and the reviewers. Any product that may be evaluated in this article or claim that may be made by its manufacturer is not guaranteed or endorsed by the publisher.
Research integrity at Frontiers
Learn more about the work of our research integrity team to safeguard the quality of each article we publish.