- 1Department of Nuclear Medicine, Affiliated Hospital of Jiangnan University, Wuxi, China
- 2Wuxi School of Medicine, Jiangnan University, Wuxi, China
Multimodal imaging are approaches which combines multiple imaging techniques to obtain multi-aspect information of a target through different imaging modalities, thereby greatly improve the accuracy and comprehensiveness of imaging. Superparamagnetic iron oxide nanoparticles (SPIONs) modified with branched polyethyleneimine have revealed good biocompatibility and stability, high drug loading capacity and nucleic acid transfection efficiency. SPIONs have been developed as functionalized platforms which can be further modified to enhance their functionalities. Those further modifications facilitate the application of SPIONs in multimodal imaging. In this review, we discuss the methods, advantages, applications, and prospects of BPEI-modified SPIONs in multimodal imaging.
1 Introduction
Various molecular imaging techniques such as magnetic resonance imaging (MRI), positron emission tomography (PET), single photon emission computed tomography (SPECT), optical imaging (OI) and ultrasound (US) play a crucial role in the individualized diagnosis and treatment of diseases (Weissleder and Pittet, 2008). These molecular imaging techniques have been applied to evaluate specific molecular targets and visualize the internal structure of the human body. They have also been applied to the non-invasive study of biological processes in vivo at the cellular and molecular levels and play a key role in the diagnosis of diseases, patient management, and healthcare. However, among all the current molecular imaging techniques, there is not a single modality that can perfectly provide all the information needed. For example, optical fluorescence imaging is difficult to quantify, and has limited tissue penetration in vivo. MRI has high resolution but low sensitivity, while PET offers very high sensitivity but relatively poor resolution (Table 1) (Massoud and Gambhir, 2003; Gleich and Weizenecker, 2005; Alphandéry, 2019; Lu et al., 2021). Therefore, multimodal contrast agents and probes have been developed to solve this problem. Multimodal imaging is the combination of two or more imaging technologies, combining the advantages of different imaging modalities, while minimizing the disadvantages of those technologies. These contrast agents and probes make it possible to visualize, quantify, and trace the molecular processes. They can also detect abnormalities in the human body, obtain new information about some diseases, and achieving the effect of “1 + 1>2” to optimize diagnosis and treatment of diseases. For example, contrast agent and probes can be used to guide the scalpel during surgery (by fluorescence imaging), ensuring that all cancerous materials have been removed (by MRI), and tracking and identifying tumor cells and physiological processes (by PET or SPECT imaging). However, the synthesis of contrast agent and probes poses a huge challenge (Jennings and Long, 2009; Zhou and Brahme, 2010; Misri et al., 2012). Nanoparticles (NPs) have been extensively used as contrast agents for molecular imaging due to their potential in combining multimodal imaging, drug delivery, and targeted therapy into a single entity (Table 2 summarized the current application of advanced nanomaterials for multimodal imaging) (Yuan et al., 2021).
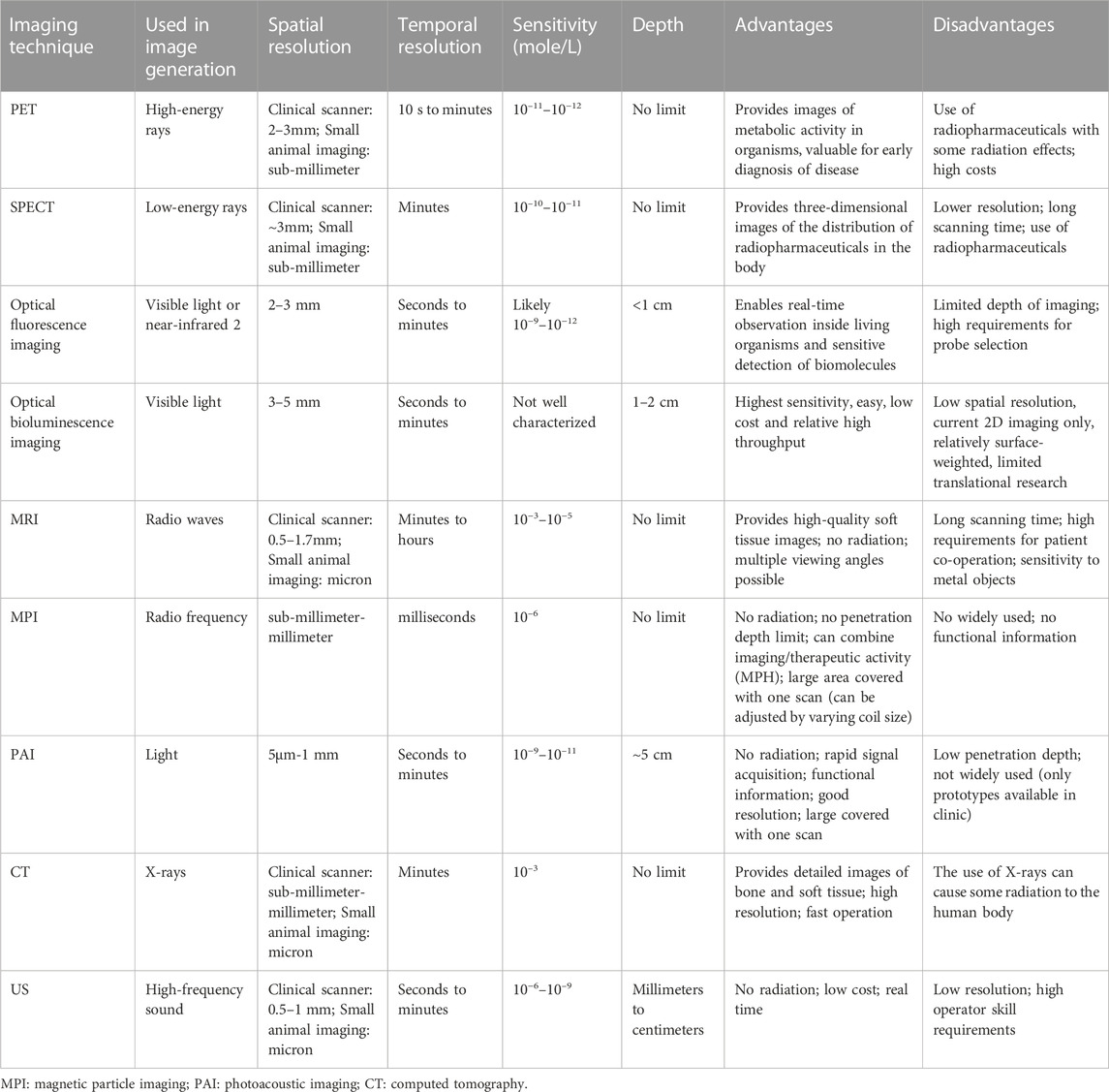
TABLE 1. Common imaging techniques and their sensitivity, spatial resolution, temporal resolution, advantages, and disadvantages.
The application of MRI contrast agents based on magnetic iron oxide nanoparticles began in the 1990s for the clinical diagnosis of liver tumors (Stark et al., 1988). Currently, commercially available products include Feridex (superparamagnetic iron oxide [SPIO]; liver injury imaging), Gastromark (SPIO, gastrointestinal imaging) and Combidex (ultrasmall SPIO [USPIO]; lymphography) (Gao and Yang, 2009). The results of clinical application of these products demonstrate the excellent magnetic imaging performance and in vivo safety of magnetic iron oxide nanoparticles in MRI diagnosis. Thus, magnetic iron oxide nanoparticles as MRI contrast agents are a current domestic and international research hotpot. In recent years, a new generation of SPIO nanoparticles (SPIONs) as MRI contrast agents with complex modified structures and functions haven been developed along with the continuous development of nanoparticle preparation technology (Xing et al., 2014; Ajinkya et al., 2020). However, in vivo targeted imaging applications place high demands on the physicochemical properties of nanoparticles. SPIONs modified by branched polyethyleneimine (BPEI) exhibit good physicochemical properties and can provide functionalization platforms for further chemical modifications for targeting, drug delivering and other functions (Bao et al., 2014; Molaei et al., 2021).
Here, we briefly summarize the application of SPIONs in multimodal imaging, the synthetic approach to BPEI-modified iron oxide nanoparticles, and the prospects and challenges for their application use in multimodal imaging by searching in the PubMed, Web of Science databases based on the keywords “iron oxide, nanoparticles, polyethyleneimine, multimodal, MRI, etc.,”.
2 Application of SPIONs in multimodal imaging
MRI is noninvasive, safe, and radiation-free modality and has a high-spatial resolution. Its applications in molecular and cellular imaging are growing rapidly and plays an essential role in diagnosing and staging of tumors. It is not restricted by the penetration depth of the signal, has no ionizing radiation, and exhibits high soft tissue resolution and wide range of clinical applicability. These advantages have made MRI an important imaging technique of clinical tumors diagnosis (Terreno et al., 2010). Since the approval of the first clinical magnetic resonance (MR) contrast agent, namely, Magnevist (Gd-DTPA), by the U.S. Food Surveillance Administration in 1998 up to the present (Boros et al., 2015), the most widely used agent clinically remains to be chelates based on the metal gadolinium (Gd3+). Particularly, the demand for gadolinium-based MR contrast agents has been increasing in recent decades, with growing concerns about their safety (Shen et al., 2018). Intravenous administration of gadolinium-based contrast agents (GBCAs) is used due to their ability to reduce T1 and T2 relaxation time. GBCAs are mainly excreted through glomerular filtration, with an excretion half-life of 90 min. Therefore, the potential toxicity of GBCAs is directly related to renal disease. In patients with chronic renal failure, the excretion half-life of GBCAs may be significantly prolonged to 24 h or even longer, which may lead to the retention of GBCAs in the body (Malikova and Holesta, 2017). Studies of the potential clinical implications of gadolinium retention have focused on neurologic and cognitive effects (Habermeyer et al., 2020; Solmaz et al., 2021), as gadolinium can cross the blood-brain barrier and deposit in brain tissue, particularly in the dentate nucleus and basal ganglia (Kanda et al., 2014; McDonald et al., 2015), together with the neurotoxicity of free gadolinium (Rogosnitzky and Branch, 2016). Moreover, intravenous administration of large amounts of GBCAs can result in extensive multiorgan deposition. In a study by Robert J. McDonald et al., healthy rats received 20 intravenous injections of 2.5 mmol gadolinium per kilogram (gadolinium-exposed group) or saline (control group) over a 26-day period. Their results demonstrated that the application of macrocyclic gadolinium chelates instead of linear chelates could reduce the deposition but could not eliminate it (McDonald et al., 2017). Therefore, the development of safe and efficient new contrast agents is of great significance and value. Inorganic nanomaterials, especially magnetic nanoparticles have been extensively studied and applied in the biomedical field (Lutz et al., 2006; Moradi Khaniabadi et al., 2017; Nosrati et al., 2019).
SPIONs are often used as MRI imaging probes in molecular imaging, these particles effectively shorten the T2 of water protons, particularly T2* (Laurent et al., 2008). The mechanism of contrast generation is related to the magnetic properties of nanoparticles, which exert a strong magnetic induction effect on the water protons diffusing around the particles. The relaxation and pharmacological properties of SPIOs are mainly controlled by their size. SPIONs usually consist of a core of magnetite (Fe3O4) and γ-magnetohematite (Fe2O3) crystals. A study by Ajay Kumar Gupta et al. concluded that magnetic nanoparticles of 10–100 nm have the best stability and magnetization strength (Gupta and Gupta, 2005). The cores are coated with suitable materials and have total diameters ranging from approximately 60nm–250 nm. Small particles (diameters ranging from 20–50 nm), defined as USPIOs are characterized by a low r2/r1 ratio. Moreover, micrometer-sized particles (micrometer SPIOs) are useful in cell labeling and vascular targeting (Shapiro et al., 2005; Shapiro et al., 2006). Currently, SPIOs are widely used in varieties biomedical applications, such as cell separation (Liu et al., 2008), drug and gene delivery (Pan et al., 2007; Chen et al., 2009; Chen et al., 2010), multimodal imaging photothermal therapy (Cheng et al., 2011), and MRI (Corti et al., 2008; Zhang et al., 2015). For successful biomedical applications, Fe3O4 NPs are usually required to have good colloidal stability, low nonspecific phagocytosis by the reticuloendothelial system (RES), and active targeting specificity after targeting ligand functionalization. Hence, the surface modifications of Fe3O4 NPs with hydrophilic and biocompatible polymers are effective and important strategies. Various materials and polymers such as albumin (Berry et al., 2003), dextran (Berry et al., 2004), dendrimers (Shi et al., 2007), polyethylene glycol (PEG) (Kohler et al., 2006), and polyethyleneimine (PEI) (Chertok et al., 2010) are coated on the surface of Fe3O4 NPs to improve their stability and reduce the clearance by RES.
However, some studies have shown that SPIONs still have many limitations in molecular imaging, such as biocompatibility. Although SPIONs have been widely used in biomedical fields, their biocompatibility is still a problem that needs to be solved. Some studies have also demonstrated that SPIONs may cause toxic reactions in cells, which may impair the normal functions of the cells. Moreover, the stability of SPIONs in organisms is also another issue that requires attention. Under certain conditions, SPIONs may undergo oxidation, aggregation, or decomposition, which may not only hamper their imaging or drug delivery efficacy but may also cause negative implications on organisms. The metabolism of SPIONs in organisms remains another challenge. If SPIONs cannot been efficiently cleared from the organisms, their accumulation in tissues or organs may result in potential health risks. Finally, the preparation of SPIONs is often complicated. It requires precise control of reaction conditions to obtain the desired particle size and shape. Furthermore, surface modifications are also complex and necessary to improve their biocompatibility and functionality (Mahmoudi et al., 2011; Wu et al., 2015; Zhu et al., 2018).
Therefore, researchers have developed a series of surface engineering strategies to modify and functionalize the SPION surface with organic or inorganic materials, such as polymers, biomacromolecules, silica, and metals (Wu et al., 2008; Laurent and Mahmoudi, 2011; Wahajuddin and Arora, 2012). Table 3 summarizes the organic macromolecules which have been used for the iron oxide NPs functionalization and their advantages. Dendrimers are monodispersed polymers characterized by a dendritic structure consisting of oligomers that are repeated and linearly connected through branching units. Dendrimers form macromolecules with dendritic structures through repeated growth and branching, and the degree of branching expands as the number of polymerization generations increases, eventually forming closed three-dimensional spherical structures with embedded cavity structures, surfaces enriched with reactive functional groups, and controllable physicochemical properties. The application of dendrimers for the modification of iron oxide nanoparticles is a novel nanomaterial preparation strategy widely used in the pharmaceutical industry (Sharma et al., 2017). BPEI is a polymer with good water solubility and thermal stability. The branched chains of BPEI are rich in amino groups and have certain internal hydrophobic cavity structures, which provide the potential of multifunctional modification on the surfaces (Li et al., 2016).
3 Advantages of BPEI modification of SPIONs
BPEI is a cationic polymer with excellent water solubility and abundant imino and amine groups, and has been used in a wide range of applications (Jager et al., 2012); in particular, BPEI has been used as a modifier for the preparation of composites (Liu et al., 2014) and as delivery vehicles for biomedicine, drug delivery and gene transfection (An and Gao, 2007; Bao et al., 2014). BPEI modification can greatly improve the dispersibility of magnetic nanomaterials Fe3O4 superparamagnetic nanoparticles, while the surface amine groups enable their conjugations to ligands, antibodies, and drugs. However, the amine groups of BPEI or BPEI-modified nanoparticles may result in severe cytotoxicity and nonspecific cell membrane binding, which may cause undesirable consequence for biological applications. Hence, surface amine group neutralization has been employed to reduce positive charge on surfaces and overcome the above disadvantages. For example, the primary amine groups of BPEI-modified multicarbon nanotubes can be acetylated or carboxylated, which can significantly improve the biocompatibility of those nanotubes (Wen et al., 2013). Table 4 summarizes the advantages of BPEI-modified iron oxide nanomaterials.
4 Methods and applications of BPEI-modified SPIONs
The synthesis methods of BPEI-modified SPIONs include electrostatic adsorption, Covalent binding, Ligand exchange, Hydrothermal method, Photochemistry synthesis and other methods. We summarized the chemical and physical properties as well as applications of different types of BPEI -modified SPIONs synthesis methods (Table 5).
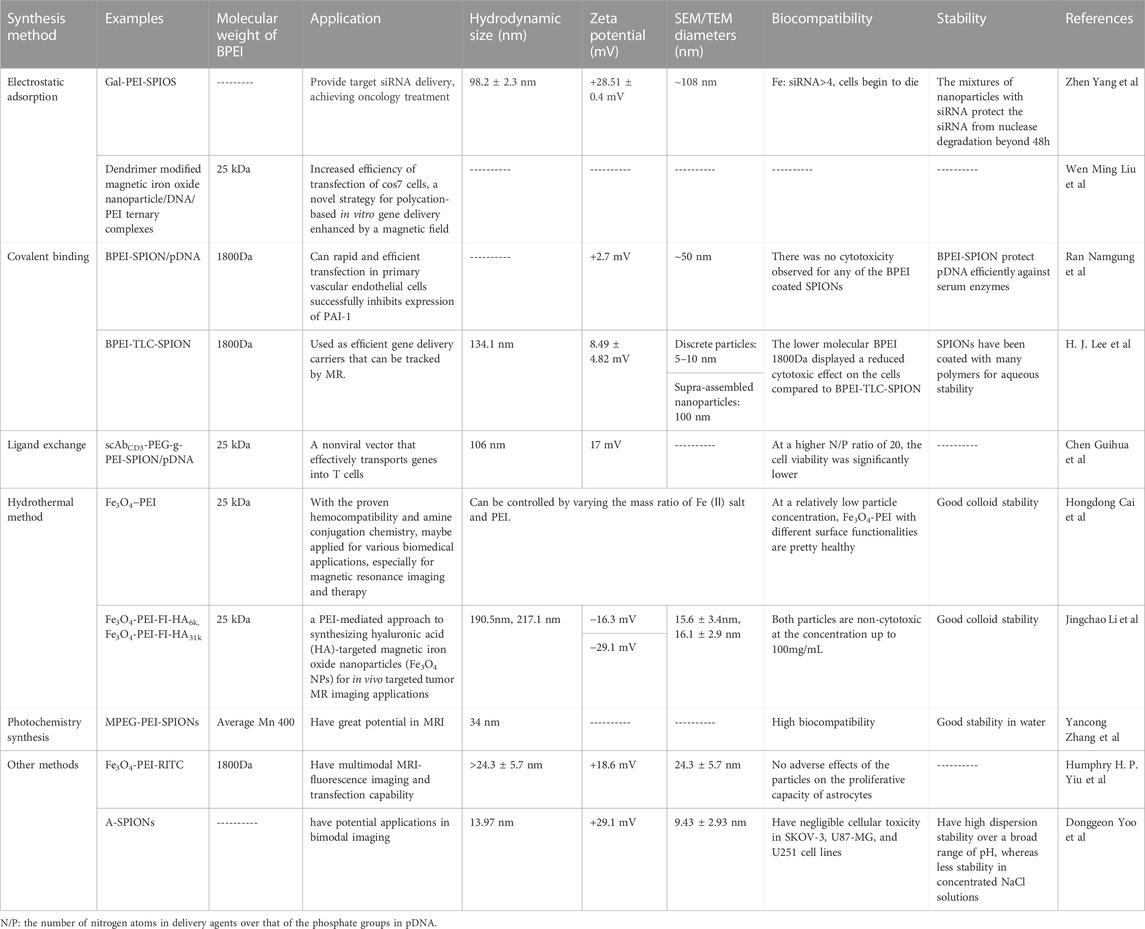
TABLE 5. The chemical and physical properties as well as applications of different types of BPEI -modified SPIONs synthesis methods.
4.1 Electrostatic adsorption
SPIO-based nanoparticles are promising platforms for the in vivo delivery of siRNA in tumor therapies. The development of novel nanoparticles composed of SPIO provides new options for tumor therapy (Steitz et al., 2007). Through electrostatic interactions, positively charged PEI-coated quantum dots are anchored on the surface of magnetic mandrel, which combine magnetization and efficient fluorescence in tandem for biosensors and clinical diagnostic imaging (Chen et al., 2016). Zhen Yang et al. introduced a novel nanoparticle with a core of iron oxide and modified by galactose (Gal) and PEI, the particle was loaded with siRNA and provided targeted delivery of therapeutic siRNA to liver cancer. The carboxyl-capped Fe3O4 was initially synthesized using a modified oxidative coprecipitation method, and PEI was further attached to the Fe3O4-COOH surface by electrostatic adsorption. Finally, Gal-PEG-NH2 was added to the mixed solution to react, and Gal-PEI-SPIOs were purified by removing free PEI and Gal using magnets to precipitate the complex. Gal-PEI-SPIOs, obtained by purification, could tightly bind the siRNA. Gal-PEI-SPIOs could protect siRNA from serum degradation by nuclease in the system, prolong the half-life of siRNA, and deliver the loaded siRNA into tumor cells. Gal-PEI-SPIOs significantly enhance the siRNA accumulation in tumor tissues and inhibited the tumor growth. Gal-PEI-SPIOs provide us with a promising strategy for hepatocellular carcinoma treatment has great prospects in tumor gene therapy (Figure 1) (Yang et al., 2018). Wen Ming Liu et al. reported the use of dendrimer-modified magnetic iron oxide nanoparticle/DNA/PEI ternary complexes for the magnetic infection of mammalian cells. The dendrimer-modified SPION was mixed with plasmid DNA, then cationic polymer PEI was condensed to form ternary complexes with positive surface charges. The results showed that magnetic field significantly increased the transfection efficiency of COS7 cells with the ternary magnetoplexes, particularly in the presence of 10% serum (Liu et al., 2011). Chuanxu Yang et al. also developed a theranostic nanoparticle NP/PEI/siCOX-2 for multimodal imaging and siRNA delivery, which was formed by encapsulation of SPIONs and indocyanine green in a poly (lactic-co-glycolic acid) matrix to serve as a multimodal probe for near-infrared and MRI (Yang et al., 2017).
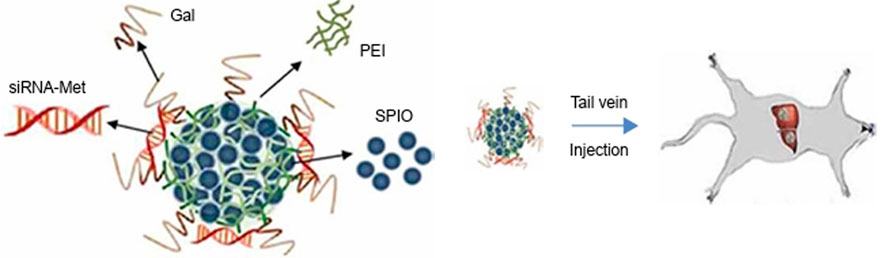
FIGURE 1. Schematic illustration of Gal-PEI-SPIO nanoencapsulated with siRNA and injected into the mouse (Yang et al., 2018).
4.2 Covalent binding
Most studies on magnetic nanoparticle-mediated transfection have been performed by coating magnetic nanoparticles with cationic polymers, such as BPEI and diethylaminoethyl-dextran (DEAE-dextran). However, the transfection efficiencies of such coated magnetic nanoparticles were not satisfactory. Magnetic nanoparticles attached with PEG molecules and BPEI exhibit excellent magnetic transfection efficiencies even in serum-conditioned media, which enable rapid and efficient transfection of primary vascular cells. PAI-1 plays an important role in various vascular dysfunctions, including vascular inflammation and atherosclerosis. Ran Namgung et al. successfully downregulated PAI-1 expression in primary HUVECs using BPEI-SPION/gWIZ-IL-10, demonstrating the potential of BPEI-SPION as magnetic nanoparticle-mediated targeted gene delivery system (Figure 2) (Namgung et al., 2010). H. J. Lee et al. proposed a strategy of using SPIONs to deliver tumor suppressor genes for tumor therapy, in this study BPEI conjugated thermally cross-linked SPIONs (TCL-SPIONs) were served as a p53 plasmid DNA delivery vehicle. Their results demonstrated that BPEI-TCL-SPIONs successfully delivered p53 plasmid DNA into tumor cells and increased p53 tumor suppressor gene expression. MRI result revealed that the negative contrast enhancement increased in a dose-dependent manner with the increase in the BPEI-TCL-SPIONs concentration in the treated cells. These results indicated that BPEI-TCL-SPIONs could be used as efficient gene delivery carriers and tracked by MRI (Lee et al., 2012). The simple surface functionalization with PEI through glutaraldehyde linker activation gave the complex of PEI-coated Fe3O4, which loaded isothiocyanate or green fluorescent protein can be visualized and had high transfection efficiency for siRNA and gene delivery (Nguyen et al., 2018).
4.3 Ligand exchange
Nonviral vector-mediated gene therapy has a great advantage over traditional drug therapies in inducing immunosuppression after organ transplantation. Chen Guihua et al. developed a nonviral T cell targeted gene vector by conjugating the T cell specific ligand CD3 single-chain antibody (scAbCD3) with poly (ethylene glycol)-grafted PEI (scAbCD3-PEG-g-PEI). Then scAbCD3-PEG-g-PEI polymer was complexed with SPIONs and plasmid DNA was condensed into nanoparticles to form the delivery agent (scAbCD3-PEG-g-PEI-SPION/pDNA). Results demonstrated that scAbCD3-PEG-g-PEI-SPION/pDNA exhibited not only high gene deliver efficacy but also low cytotoxicity in rat T-lymphocyte line HB8521 cells. Moreover, the targeting effect of scAbCD3-PEG-g-PEI-SPION was successfully detected by MRI. This study has proven that scAbCD3-PEG-g-PEI-SPION has great potential to be used as a MRI-traceable and T-lymphocyte-targeting gene carrier for immunotherapy (Figure 3) (Chen et al., 2009).
4.4 Hydrothermal method
Under high-pressure conditions, polymer shrinkage occurs simultaneously with the encapsulation of inorganic nanoparticles in the BPEI branches, and polymer shrinkage increases with pressure. Hongdong Cai et al. reported a simple hydrothermal synthesis and surface functionalization method of BPEI-coated iron oxide nanoparticles (Fe3O4-PEI NPs). The results demonstrated that the size of Fe3O4-PEI NPs can be controlled by varying the mass ratio of Fe(II) salts to BPEI. Furthermore, the functionalized Fe3O4-PEI NPs displayed good aqueous dispersibility, colloidal stability and relatively high R2 relaxivity. The surface PEGylation and acylation endowed the Fe3O4-PEI NPs with good biocompatibility (Cai et al., 2013). Jingchao Li et al. also reported a BPEI-mediated method of synthesizing hyaluronic acid (HA) targeting magnetic iron oxide nanoparticles for the in vivo targeted tumor MRI imaging. HA is an attractive targeting ligand that binds CD44 receptors, which are overexpressed in many kinds of tumor cells. In this work, PEI-Fe3O4 NPs via a one-pot hydrothermal method. The formed PEO-stabilized Fe3O4 NPs were modified with fluorescein isothiocyanate (FI) and HA with different molecular weight, and finally two kinds of Fe3O4 NPs were obtained. The researchers demonstrated that HA targeted Fe3O4 NPs were capable of endocytosis by tumor cells expressing CD44 receptors and serving as targeted MRI probes of cancer cells in vitro and xenografts in vivo (Figure 4) (Li et al., 2014). More hydrothermal synthesis of nanostructured blends based on SPIONs and branched BPEI polymers have been widely reported (Lv et al., 2014; Popescu et al., 2015).
4.5 Photochemistry synthesis
Novel method for synthesis of SPIONs coated with PEI and modified with poly (ethylene glycol) methyl ether (MPEG), MPEG-PEI-SPIONs, was reported by Yancong Zhang et al.. Firstly, Fe3O4 were prepared by co-precipitation method. Then PEI-SPIONs were successfully prepared in aqueous system using photochemical methods and their surfaces were modified with MPEG. T2 relaxation measurements showed that the magnetic resonance signals were significantly enhanced with the increase of the concentration of nanoparticles in water. Therefore, MPEG-PEI-SPIONs have great potential for application in MRI (Zhang et al., 2015).
4.6 Other methods
Increasingly methods of BPEI-modified SPIONs are being developed for imaging and therapy (Arsianti et al., 2010; Lentijo Mozo et al., 2017; Mulens-Arias et al., 2019; Zou et al., 2020). Humphrey H. P. Yiu et al. developed Fe3O4-PEI-RITC magnetic nanoparticles with multimodal MRI- fluorescence imaging and transfection capability, for use in neural cell replacement therapies. The Fe3O4-PEI-RITC NPs were synthesized by a multi-step chemical grafting procedure: silanisation of NPs with 3-iodopropyltrimethoxysilane; BPEI coupling with iodopropyl groups on the surface and rhodamine isothiocyanate (RITC) binding onto the BPEI coating (Figure 5). The Fe3O4-PEI-RITC NPs combine MRI and fluorescence imaging capabilities with additional potential for transfection applications, and they can further development for non-invasive cell tracking and gene transfer to neural transplant populations (Yiu et al., 2012). Donggeon Yoo et al. reported the preparation of water dispersible angular-shaped amine-functionalized superparamagnetic iron oxide nanoparticles (A-SPIONs), which synthesized by heating iron (Ⅲ) acetylacetonate in a mixture of solvents containing PEG and BPEI under vigorous stirring. A-SPIONs exhibit high relaxivity for MRI and cyanine 5.5 dye-functionalized A-SPIONs were conducted to investigate their fluorescence imaging applications, which resulted that A-SPIONs have potential applications in multimodal imaging (Yoo et al., 2017).
5 Prospects and challenges
Multimodal imaging is a method that combines multiple imaging techniques to provide much more comprehensive and accurate information than single modality. BPEI-modified SPIONs have been used as highly efficient MRI contrast agents, which significantly improve the imaging resolution and contrast. BPEI-modified SPIONs are capable of accommodating different imaging modalities, such as MRI, fluorescence imaging, and photoacoustic imaging, by adjusting their surface properties and structure. While those kinds of modalities have a wide range of prospects for multimodal imaging applications. Apart from the applications in imaging, BPEI-modified SPIONs also demonstrated great potentials of targeted delivery and imaging of specific lesions by conjugating to specific targeting molecules, and these applications greatly facilitate the identification and localization of lesion areas. BPEI modification significantly improves the stability, biocompatibility and biosafety of SPIONs, and reduce the aggregation and clearance in vivo. SPIONs exhibit great prospects for applications in medical diagnosis and therapy.
However, challenges and limitations also exist with BPEI-modified SPIONs in multimodal imaging, such as imaging effectiveness, targeting and specificity, stability, and cost-effectiveness. In particular, the design and synthesis of molecular probes with excellent imaging performance are essential challenges. Moreover, the targeting and specificity of nanoparticles in multimodal imaging are vital for precise imaging. Studies have been conducted to achieve localized imaging of specific tissues and cells by introducing specific targeting molecules on the surface of BPEI-modified SPIONs.
Furthermore, the stability of nanomaterials is crucial for long-time imaging and storage. Various strategies have been proposed to improve the stability of BPEI-modified SPIONs, such as the synthesis of stable core-shell structures and the introduction of cross-linking agents. Finally, the cost of nanoparticles’ synthesis and application is also an important concern. Efforts have been conducted to improve the cost-effectiveness of BPEI-modified SPIONs through improving the synthesis methods.
In conclusion, BPEI-modified SPIONs have demonstrated promising applications in multimodal imaging, but further research and improvements are still needed to overcome the existing challenges and limitations, improve the imaging efficacy, targeting, biocompatibility and stability of the nanoparticles through continuously optimization of synthesis methods and surface modification strategies. Through persistent efforts, accurate and reliable multimodal imaging using BPEI-modified SPIONs can be achieved in the future.
Author contributions
QS: Conceptualization, Data curation, Investigation, Methodology, Software, Writing–original draft. CY: Formal Analysis, Funding acquisition, Project administration, Resources, Supervision, Writing–review and editing.
Funding
The author(s) declare financial support was received for the research, authorship, and/or publication of this article. This work was supported by the Subject Construction Fund from Wuxi Medicine School of Jiangnan University, the Subject Development Fund (FZXK2021011) from Wuxi Health Select Committee.
Conflict of interest
The authors declare that the research was conducted in the absence of any commercial or financial relationships that could be construed as a potential conflict of interest.
Publisher’s note
All claims expressed in this article are solely those of the authors and do not necessarily represent those of their affiliated organizations, or those of the publisher, the editors and the reviewers. Any product that may be evaluated in this article, or claim that may be made by its manufacturer, is not guaranteed or endorsed by the publisher.
References
Ajinkya, N., Yu, X., Kaithal, P., Luo, H., Somani, P., and Ramakrishna, S. (2020). Magnetic iron oxide nanoparticle (IONP) synthesis to applications: present and future. Mater. (Basel) 13 (20), 4644. doi:10.3390/ma13204644
Alphandéry, E. (2019). Iron oxide nanoparticles as multimodal imaging tools. RSC Adv. 9 (69), 40577–40587. doi:10.1039/c9ra08612a
An, F., and Gao, B. (2007). Chelating adsorption properties of PEI/SiO(2) for plumbum ion. J. Hazard Mater 145 (3), 495–500. doi:10.1016/j.jhazmat.2006.11.051
Arbab, A. S., Bashaw, L. A., Miller, B. R., Jordan, E. K., Lewis, B. K., Kalish, H., et al. (2003). Characterization of biophysical and metabolic properties of cells labeled with superparamagnetic iron oxide nanoparticles and transfection agent for cellular MR imaging. Radiology 229 (3), 838–846. doi:10.1148/radiol.2293021215
Arsianti, M., Lim, M., Marquis, C. P., and Amal, R. (2010). Assembly of polyethylenimine-based magnetic iron oxide vectors: insights into gene delivery. Langmuir 26 (10), 7314–7326. doi:10.1021/la9041919
Bai, J., Wang, J. T., Rubio, N., Protti, A., Heidari, H., Elgogary, R., et al. (2016). Triple-Modal imaging of magnetically-targeted nanocapsules in solid tumours in vivo. Theranostics 6 (3), 342–356. doi:10.7150/thno.11918
Bao, X., Wang, W., Wang, C., Wang, Y., Zhou, J., Ding, Y., et al. (2014). A chitosan-graft-PEI-candesartan conjugate for targeted co-delivery of drug and gene in anti-angiogenesis cancer therapy. Biomaterials 35 (29), 8450–8466. doi:10.1016/j.biomaterials.2014.06.025
Berry, C. C., Wells, S., Charles, S., Aitchison, G., and Curtis, A. S. (2004). Cell response to dextran-derivatised iron oxide nanoparticles post internalisation. Biomaterials 25 (23), 5405–5413. doi:10.1016/j.biomaterials.2003.12.046
Berry, C. C., Wells, S., Charles, S., and Curtis, A. S. (2003). Dextran and albumin derivatised iron oxide nanoparticles: influence on fibroblasts in vitro. Biomaterials 24 (25), 4551–4557. doi:10.1016/s0142-9612(03)00237-0
Boros, E., Gale, E. M., and Caravan, P. (2015). MR imaging probes: design and applications. Dalton Trans. 44 (11), 4804–4818. doi:10.1039/c4dt02958e
Cai, H., An, X., Cui, J., Li, J., Wen, S., Li, K., et al. (2013). Facile hydrothermal synthesis and surface functionalization of polyethyleneimine-coated iron oxide nanoparticles for biomedical applications. ACS Appl. Mater Interfaces 5 (5), 1722–1731. doi:10.1021/am302883m
Chang, Y., Meng, X., Zhao, Y., Li, K., Zhao, B., Zhu, M., et al. (2011). Novel water-soluble and pH-responsive anticancer drug nanocarriers: doxorubicin-PAMAM dendrimer conjugates attached to superparamagnetic iron oxide nanoparticles (IONPs). J. Colloid Interface Sci. 363 (1), 403–409. doi:10.1016/j.jcis.2011.06.086
Chastellain, M., Petri, A., and Hofmann, H. (2004). Particle size investigations of a multistep synthesis of PVA coated superparamagnetic nanoparticles. J. Colloid Interface Sci. 278 (2), 353–360. doi:10.1016/j.jcis.2004.06.025
Chen, G., Chen, W., Wu, Z., Yuan, R., Li, H., Gao, J., et al. (2009). MRI-visible polymeric vector bearing CD3 single chain antibody for gene delivery to T cells for immunosuppression. Biomaterials 30 (10), 1962–1970. doi:10.1016/j.biomaterials.2008.12.043
Chen, S., Zhang, J., Song, S., Feng, R., Ju, Y., Xiong, C., et al. (2016). Hydrophilic magnetofluorescent nanobowls: rapid magnetic response and efficient photoluminescence. Langmuir 32 (2), 611–618. doi:10.1021/acs.langmuir.5b03978
Chen, Y., Chen, H., Zeng, D., Tian, Y., Chen, F., Feng, J., et al. (2010). Core/shell structured hollow mesoporous nanocapsules: a potential platform for simultaneous cell imaging and anticancer drug delivery. ACS Nano 4 (10), 6001–6013. doi:10.1021/nn1015117
Chen, Y. S., Zhao, Y., Beinat, C., Zlitni, A., Hsu, E. C., Chen, D. H., et al. (2021). Ultra-high-frequency radio-frequency acoustic molecular imaging with saline nanodroplets in living subjects. Nat. Nanotechnol. 16 (6), 717–724. doi:10.1038/s41565-021-00869-5
Chen, Z., Mu, X., Han, Z., Yang, S., Zhang, C., Guo, Z., et al. (2019). An optical/photoacoustic dual-modality probe: ratiometric in/ex vivo imaging for stimulated H(2)S upregulation in mice. J. Am. Chem. Soc. 141 (45), 17973–17977. doi:10.1021/jacs.9b09181
Chena, F., Gaoa, Q., Hong, G., and Ni, J. (2008). Synthesis of magnetite core-shell nanoparticles by surface-initiated ring-opening polymerization of L-lactide. J. Magn. Magn. Mater 320 (13), 1921–1927. doi:10.1016/j.jmmm.2008.02.132
Cheng, L., Yang, K., Li, Y., Chen, J., Wang, C., Shao, M., et al. (2011). Facile preparation of multifunctional upconversion nanoprobes for multimodal imaging and dual-targeted photothermal therapy. Angew. Chem. Int. Ed. Engl. 50 (32), 7385–7390. doi:10.1002/anie.201101447
Chertok, B., David, A. E., and Yang, V. C. (2010). Polyethyleneimine-modified iron oxide nanoparticles for brain tumor drug delivery using magnetic targeting and intra-carotid administration. Biomaterials 31 (24), 6317–6324. doi:10.1016/j.biomaterials.2010.04.043
Corti, M., Lascialfari, A., Marinone, M., Masotti, A., Micotti, E., Orsini, F., et al. (2008). Magnetic and relaxometric properties of polyethylenimine-coated superparamagnetic MRI contrast agents. J. Magn. Magn. Mater 320 (14), E316–E319. doi:10.1016/j.jmmm.2008.02.115
D’Souza, A. J., Schowen, R. L., and Topp, E. M. (2004). Polyvinylpyrrolidone-drug conjugate: synthesis and release mechanism. J. Control Release 94 (1), 91–100. doi:10.1016/j.jconrel.2003.09.014
Far, H. S., Hasanzadeh, M., Nashtaei, M. S., Rabbani, M., Haji, A., and Hadavi Moghadam, B. (2020). PPI-Dendrimer-Functionalized magnetic metal-organic framework (Fe(3)O(4)@MOF@PPI) with high adsorption capacity for sustainable wastewater treatment. ACS Appl. Mater Interfaces 12 (22), 25294–25303. doi:10.1021/acsami.0c04953
Gaihre, B., Aryal, S., Khil, M. S., and Kim, H. Y. (2008). Encapsulation of Fe3O4 in gelatin nanoparticles: effect of different parameters on size and stability of the colloidal dispersion. J. Microencapsul. 25 (1), 21–30. doi:10.1080/02652040701737697
Gao, R. Q. C. Y. M., and Yang, C. (2009). Superparamagnetic iron oxide nanoparticles: from preparations to in vivo MRI applications. J. Mater. Chem. 19 (35), 6274–6293. doi:10.1039/b902394a
Gleich, B., and Weizenecker, J. (2005). Tomographic imaging using the nonlinear response of magnetic particles. Nature 435 (7046), 1214–1217. doi:10.1038/nature03808
Gomez-Lopera, S. A., Arias, J. L., Gallardo, V., and Delgado, A. V. (2006). Colloidal stability of magnetite/poly(lactic acid) core/shell nanoparticles. Langmuir 22 (6), 2816–2821. doi:10.1021/la0530079
Gupta, A. K., and Gupta, M. (2005). Synthesis and surface engineering of iron oxide nanoparticles for biomedical applications. Biomaterials 26 (18), 3995–4021. doi:10.1016/j.biomaterials.2004.10.012
Habermeyer, J., Boyken, J., Harrer, J., Canneva, F., Ratz, V., Moceri, S., et al. (2020). Comprehensive phenotyping revealed transient startle response reduction and histopathological gadolinium localization to perineuronal nets after gadodiamide administration in rats. Sci. Rep. 10 (1), 22385. doi:10.1038/s41598-020-79374-z
Jager, M., Schubert, S., Ochrimenko, S., Fischer, D., and Schubert, U. S. (2012). Branched and linear poly(ethylene imine)-based conjugates: synthetic modification, characterization, and application. Chem. Soc. Rev. 41 (13), 4755–4767. doi:10.1039/c2cs35146c
Jennings, L. E., and Long, N. J. (2009). Two is better than one'--probes for dual-modality molecular imaging. Chem. Commun. (Camb) 24, 3511–3524. doi:10.1039/b821903f
Jie, L., Lang, D., Kang, X., Yang, Z., Du, Y., and Ying, X. (2019). Superparamagnetic iron oxide nanoparticles/doxorubicin-loaded starch-octanoic micelles for targeted tumor therapy. J. Nanosci. Nanotechnol. 19 (9), 5456–5462. doi:10.1166/jnn.2019.16548
Kanda, T., Ishii, K., Kawaguchi, H., Kitajima, K., and Takenaka, D. (2014). High signal intensity in the dentate nucleus and globus pallidus on unenhanced T1-weighted MR images: relationship with increasing cumulative dose of a gadolinium-based contrast material. Radiology 270 (3), 834–841. doi:10.1148/radiol.13131669
Kang, N. Y., Lee, J. Y., Lee, S. H., Song, I. H., Hwang, Y. H., Kim, M. J., et al. (2020). Multimodal imaging probe development for pancreatic β cells: from fluorescence to PET. J. Am. Chem. Soc. 142 (7), 3430–3439. doi:10.1021/jacs.9b11173
Khodadust, R., Unsoy, G., Yalcın, S., Gunduz, G., and Gunduz, U. (2013). PAMAM dendrimer-coated iron oxide nanoparticles:synthesis and characterization of different generations. J. Nanopart Res. 15 (3), 1488. doi:10.1007/s11051-013-1488-6
Kohler, N., Sun, C., Fichtenholtz, A., Gunn, J., Fang, C., and Zhang, M. (2006). Methotrexate-immobilized poly(ethylene glycol) magnetic nanoparticles for MR imaging and drug delivery. Small 2 (6), 785–792. doi:10.1002/smll.200600009
Krasitskaya, V. V., Kudryavtsev, A. N., Yaroslavtsev, R. N., Velikanov, D. A., Bayukov, O. A., Gerasimova, Y. V., et al. (2022). Starch-coated magnetic iron oxide nanoparticles for affinity purification of recombinant proteins. Int. J. Mol. Sci. 23 (10), 5410. doi:10.3390/ijms23105410
Laurent, S., Forge, D., Port, M., Roch, A., Robic, C., Vander Elst, L., et al. (2008). Magnetic iron oxide nanoparticles: synthesis, stabilization, vectorization, physicochemical characterizations, and biological applications. Chem. Rev. 108 (6), 2064–2110. doi:10.1021/cr068445e
Laurent, S., and Mahmoudi, M. (2011). Superparamagnetic iron oxide nanoparticles: promises for diagnosis and treatment of cancer. Int. J. Mol. Epidemiol. Genet. 2 (4), 367–390.
Lee, H. J., Nguyen, Y. T., Muthiah, M., Vu-Quang, H., Namgung, R., Kim, W. J., et al. (2012). MR traceable delivery of p53 tumor suppressor gene by PEI-functionalized superparamagnetic iron oxide nanoparticles. J. Biomed. Nanotechnol. 8 (3), 361–371. doi:10.1166/jbn.2012.1407
Lentijo Mozo, S., Zuddas, E., Casu, A., and Falqui, A. (2017). Synthesizing iron oxide nanostructures: the polyethylenenemine (PEI) role. Crystals 7 (1), 22. doi:10.3390/cryst7010022
Li, A., Zhou, B., Alves, C. S., Xu, B., Guo, R., Shi, X., et al. (2016). Mechanistic studies of enhanced PCR using PEGylated PEI-entrapped gold nanoparticles. ACS Appl. Mater Interfaces 8 (39), 25808–25817. doi:10.1021/acsami.6b09310
Li, G.-y., Huang, K.-l., Jiang, Y.-r., Ding, P., and Yang, D.-l. (2008a). Preparation and characterization of carboxyl functionalization of chitosan derivative magnetic nanoparticles. Biochem. Eng. J. 40 (3), 408–414. doi:10.1016/j.bej.2008.01.018
Li, G.-Y., Jiang, Y.-R., Huang, K.-L., Ding, P., and Yao, L.-L. (2008b). Kinetics of adsorption of Saccharomyces cerevisiae mandelated dehydrogenase on magnetic Fe3O4–chitosan nanoparticles. Colloids Surfaces A Physicochem. Eng. Aspects 320 (1-3), 11–18. doi:10.1016/j.colsurfa.2008.01.017
Li, H., Fu, C., Miao, X., Li, Q., Zhang, J., Yang, H., et al. (2017). Multifunctional magnetic co-delivery system coated with polymer mPEG-PLL-FA for nasopharyngeal cancer targeted therapy and MR imaging. J. Biomater. Appl. 31 (8), 1169–1181. doi:10.1177/0885328217692964
Li, J., He, Y., Sun, W., Luo, Y., Cai, H., Pan, Y., et al. (2014). Hyaluronic acid-modified hydrothermally synthesized iron oxide nanoparticles for targeted tumor MR imaging. Biomaterials 35 (11), 3666–3677. doi:10.1016/j.biomaterials.2014.01.011
Liang, M., Tan, H., Zhou, J., Wang, T., Duan, D., Fan, K., et al. (2018). Bioengineered H-ferritin nanocages for quantitative imaging of vulnerable plaques in atherosclerosis. ACS Nano 12 (9), 9300–9308. doi:10.1021/acsnano.8b04158
Ling, Y., Tang, X., Wang, F., Zhou, X., Wang, R., Deng, L., et al. (2017). Highly efficient magnetic hyperthermia ablation of tumors using injectable polymethylmethacrylate–Fe3O4. RSC Adv. 7 (5), 2913–2918. doi:10.1039/c6ra20860f
Liu, H. L., Sonn, C. H., Wu, J. H., Lee, K. M., and Kim, Y. K. (2008). Synthesis of streptavidin-FITC-conjugated core-shell Fe3O4-Au nanocrystals and their application for the purification of CD4+ lymphocytes. Biomaterials 29 (29), 4003–4011. doi:10.1016/j.biomaterials.2008.06.031
Liu, L., Yuan, Y., Yang, Y., McMahon, M. T., Chen, S., and Zhou, X. (2019). A fluorinated aza-BODIPY derivative for NIR fluorescence/PA/(19)F MR tri-modality in vivo imaging. Chem. Commun. (Camb) 55 (42), 5851–5854. doi:10.1039/c9cc01253b
Liu, W. M., Xue, Y. N., He, W. T., Zhuo, R. X., and Huang, S. W. (2011). Dendrimer modified magnetic iron oxide nanoparticle/DNA/PEI ternary complexes: a novel strategy for magnetofection. J. Control Release 152 (Suppl. 1), e159–e160. doi:10.1016/j.jconrel.2011.08.061
Liu, Z., Wang, Y., Zu, Y., Fu, Y., Li, N., Guo, N., et al. (2014). Synthesis of polyethylenimine (PEI) functionalized silver nanoparticles by a hydrothermal method and their antibacterial activity study. Mater Sci. Eng. C Mater Biol. Appl. 42, 31–37. doi:10.1016/j.msec.2014.05.007
Lu, C., Han, L., Wang, J., Wan, J., Song, G., and Rao, J. (2021). Engineering of magnetic nanoparticles as magnetic particle imaging tracers. Chem. Soc. Rev. 50 (14), 8102–8146. doi:10.1039/d0cs00260g
Lutz, J. F., Stiller, S., Hoth, A., Kaufner, L., Pison, U., and Cartier, R. (2006). One-pot synthesis of pegylated ultrasmall iron-oxide nanoparticles and their in vivo evaluation as magnetic resonance imaging contrast agents. Biomacromolecules 7 (11), 3132–3138. doi:10.1021/bm0607527
Lv, Z., Li, D., Tang, X., Pulli, B., Cheng, J., Zhao, P., et al. (2014). Theranostic nanoparticles based on bioreducible polyethylenimine-coated iron oxide for reduction-responsive gene delivery and magnetic resonance imaging. Int. J. Nanomedicine 9, 3347–3361. doi:10.2147/ijn.s61463
Ma, H. L., Xu, Y. F., Qi, X. R., Maitani, Y., and Nagai, T. (2008). Superparamagnetic iron oxide nanoparticles stabilized by alginate: pharmacokinetics, tissue distribution, and applications in detecting liver cancers. Int. J. Pharm. 354 (1-2), 217–226. doi:10.1016/j.ijpharm.2007.11.036
Mahmoudi, M., Sant, S., Wang, B., Laurent, S., and Sen, T. (2011). Superparamagnetic iron oxide nanoparticles (SPIONs): development, surface modification and applications in chemotherapy. Adv. Drug Deliv. Rev. 63 (1-2), 24–46. doi:10.1016/j.addr.2010.05.006
Malikova, H., and Holesta, M. (2017). Gadolinium contrast agents - are they really safe? J. Vasc. Access 18 (Suppl. 2), 1–7. doi:10.5301/jva.5000713
Massoud, T. F., and Gambhir, S. S. (2003). Molecular imaging in living subjects: seeing fundamental biological processes in a new light. Genes Dev. 17 (5), 545–580. doi:10.1101/gad.1047403
McDonald, R. J., McDonald, J. S., Dai, D., Schroeder, D., Jentoft, M. E., Murray, D. L., et al. (2017). Comparison of gadolinium concentrations within multiple rat organs after intravenous administration of linear versus macrocyclic gadolinium chelates. Radiology 285 (2), 536–545. doi:10.1148/radiol.2017161594
McDonald, R. J., McDonald, J. S., Kallmes, D. F., Jentoft, M. E., Murray, D. L., Thielen, K. R., et al. (2015). Intracranial gadolinium deposition after contrast-enhanced MR imaging. Radiology 275 (3), 772–782. doi:10.1148/radiol.15150025
Mikhaylova, M., Kim, D. K., Bobrysheva, N., Osmolowsky, M., Semenov, V., Tsakalakos, T., et al. (2004). Superparamagnetism of magnetite nanoparticles: dependence on surface modification. Langmuir 20 (6), 2472–2477. doi:10.1021/la035648e
Misri, R., Saatchi, K., and Hafeli, U. O. (2012). Nanoprobes for hybrid SPECT/MR molecular imaging. Nanomedicine (Lond) 7 (5), 719–733. doi:10.2217/nnm.12.32
Molaei, H., Zaaeri, F., Sharifi, S., Ali, R., Saeed, S., Abdolmohammadi, J., et al. (2021). Polyethylenimine-graft-poly (maleic anhydride-alt-1-octadecene) coated Fe3O4 magnetic nanoparticles: promising targeted pH-sensitive system for curcumin delivery and MR imaging. Int. J. Polym. Mater. Polym. BIOMATERIALS 70 (18), 1344–1353. doi:10.1080/00914037.2020.1798435
Mondini, S., Cenedese, S., Marinoni, G., Molteni, G., Santo, N., Bianchi, C. L., et al. (2008). One-step synthesis and functionalization of hydroxyl-decorated magnetite nanoparticles. J. Colloid Interface Sci. 322 (1), 173–179. doi:10.1016/j.jcis.2008.03.008
Moradi Khaniabadi, P., Shahbazi-Gahrouei, D., Malik Shah Abdul Majid, A., Suhaimi Jaafar, M., Moradi Khaniabadi, B., and Shahbazi-Gahrouei, S. (2017). In vitro study of SPIONs-C595 as molecular imaging probe for specific breast cancer (MCF-7) cells detection. Iran. Biomed. J. 21 (6), 360–368. doi:10.18869/acadpub.ibj.21.6.360
Morales, M. A., Finotelli, P. V., Coaquira, J. A. H., Rocha-Leão, M. H. M., Diaz-Aguila, C., Baggio-Saitovitch, E. M., et al. (2008). In situ synthesis and magnetic studies of iron oxide nanoparticles in calcium-alginate matrix for biomedical applications. Mater. Sci. Eng. C 28 (2), 253–257. doi:10.1016/j.msec.2006.12.016
Mulens-Arias, V., Rojas, J. M., Sanz-Ortega, L., Portilla, Y., Pérez-Yagüe, S., and Barber, D. F. (2019). Polyethylenimine-coated superparamagnetic iron oxide nanoparticles impair in vitro and in vivo angiogenesis. Nanomedicine Nanotechnol. Biol. Med. 21, 102063. doi:10.1016/j.nano.2019.102063
Murugan, E., J, N. J., Ariraman, M., Rajendran, S., Kathirvel, J., Akshata, C. R., et al. (2018). Core-shell nanostructured Fe(3)O(4)-poly(styrene-co-vinylbenzyl chloride) grafted PPI dendrimers stabilized with AuNPs/PdNPs for efficient nuclease activity. ACS Omega 3 (10), 13685–13693. doi:10.1021/acsomega.8b01326
Namgung, R., Singha, K., Yu, M. K., Jon, S., Kim, Y. S., Ahn, Y., et al. (2010). Hybrid superparamagnetic iron oxide nanoparticle-branched polyethylenimine magnetoplexes for gene transfection of vascular endothelial cells. Biomaterials 31 (14), 4204–4213. doi:10.1016/j.biomaterials.2010.01.123
Nguyen, A. H., Abdelrasoul, G. N., Lin, D., Maadi, H., Tong, J., Chen, G., et al. (2018). Polyethylenimine-coated iron oxide magnetic nanoparticles for high efficient gene delivery. Appl. Nanosci. 8 (4), 811–821. doi:10.1007/s13204-018-0775-z
Nosrati, H., Salehiabar, M., Fridoni, M., Abdollahifar, M. A., Kheiri Manjili, H., Davaran, S., et al. (2019). New insight about biocompatibility and biodegradability of iron oxide magnetic nanoparticles: stereological and in vivo MRI monitor. Sci. Rep. 9 (1), 7173. doi:10.1038/s41598-019-43650-4
Olsen, D., Yang, C., Bodo, M., Chang, R., Leigh, S., Baez, J., et al. (2003). Recombinant collagen and gelatin for drug delivery. Adv. Drug Deliv. Rev. 55 (12), 1547–1567. doi:10.1016/j.addr.2003.08.008
Pan, B., Cui, D., Sheng, Y., Ozkan, C., Gao, F., He, R., et al. (2007). Dendrimer-modified magnetic nanoparticles enhance efficiency of gene delivery system. Cancer Res. 67 (17), 8156–8163. doi:10.1158/0008-5472.can-06-4762
Pardoe, H., Chua-anusorn, W., Pierre, T. S., and Dobson, J. (2001). Structural and magnetic properties of nanoscale iron oxide particles synthesized in the presence of dextran or polyvinyl alcohol. J. Magn. Magn. Mater 225 (1-2), 41–436.
Paul, K. G., Frigo, T. B., Groman, J. Y., and Groman, E. V. (2004). Synthesis of ultrasmall superparamagnetic iron oxides using reduced polysaccharides. Bioconjug Chem. 15 (2), 394–401. doi:10.1021/bc034194u
Popescu, L. M., Piticescu, R. M., Petriceanu, M., Ottaviani, M. F., Cangiotti, M., Vasile, E., et al. (2015). Hydrothermal synthesis of nanostructured hybrids based on iron oxide and branched PEI polymers. Influence of high pressure on structure and morphology. Mater. Chem. Phys. 161, 84–95. doi:10.1016/j.matchemphys.2015.05.018
Rogosnitzky, M., and Branch, S. (2016). Gadolinium-based contrast agent toxicity: a review of known and proposed mechanisms. Biometals 29 (3), 365–376. doi:10.1007/s10534-016-9931-7
Shan, G. B., Xing, J. M., Luo, M. F., Liu, H. Z., and Chen, J. Y. (2003). Immobilization of Pseudomonas delafieldii with magnetic polyvinyl alcohol beads and its application in biodesulfurization. Biotechnol. Lett. 25 (23), 1977–1981. doi:10.1023/b:bile.0000004388.15751.8c
Shapiro, E. M., Sharer, K., Skrtic, S., and Koretsky, A. P. (2006). In vivo detection of single cells by MRI. Magn. Reson Med. 55 (2), 242–249. doi:10.1002/mrm.20718
Shapiro, E. M., Skrtic, S., and Koretsky, A. P. (2005). Sizing it up: cellular MRI using micron-sized iron oxide particles. Magn. Reson Med. 53 (2), 329–338. doi:10.1002/mrm.20342
Sharma, A. K., Gothwal, A., Kesharwani, P., Alsaab, H., Iyer, A. K., and Gupta, U. (2017). Dendrimer nanoarchitectures for cancer diagnosis and anticancer drug delivery. Drug Discov. Today 22 (2), 314–326. doi:10.1016/j.drudis.2016.09.013
Shen, Z., Song, J., Zhou, Z., Yung, B. C., Aronova, M. A., Li, Y., et al. (2018). Dotted core-shell nanoparticles for T(1) -weighted MRI of tumors. Adv. Mater 30, e1803163. doi:10.1002/adma.201803163
Shi, X., Thomas, T. P., Myc, L. A., Kotlyar, A., and Baker, J. R. (2007). Synthesis, characterization, and intracellular uptake of carboxyl-terminated poly(amidoamine) dendrimer-stabilized iron oxide nanoparticles. Phys. Chem. Chem. Phys. 9 (42), 5712–5720. doi:10.1039/b709147h
Singh, H., Laibinis, P. E., Hatton, T. A., and Rigid, (2005). Rigid, superparamagnetic chains of permanently linked beads coated with magnetic nanoparticles. Synthesis and rotational dynamics under applied magnetic fields. Langmuir 21 (24), 11500–11509. doi:10.1021/la0517843
Solmaz, V., Köse Özlece, H., Fatih Bozkurt, M., Özkul, B., and Erbaş, O. (2021). Repeated gadoteric acid and gadobutrol exposure causes deterioration of behavior and memory functions in rats: MRI, histopathological and biochemical evidence. Brain Res. 1754, 147256. doi:10.1016/j.brainres.2020.147256
Stark, D. D., Weissleder, R., Elizondo, G., Hahn, P. F., Saini, S., Todd, L. E., et al. (1988). Superparamagnetic iron oxide: clinical application as a contrast agent for MR imaging of the liver. Radiology 168 (2), 297–301. doi:10.1148/radiology.168.2.3393649
Steitz, B., Hofmann, H., Kamau, S. W., Hassa, P. O., Hottiger, M. O., von Rechenberg, B., et al. (2007). Characterization of PEI-coated superparamagnetic iron oxide nanoparticles for transfection: size distribution, colloidal properties and DNA interaction. J. Magn. Magn. Mater 311 (1), 300–305. doi:10.1016/j.jmmm.2006.10.1194
Tajabadi, M., Khosroshahi, M. E., and Bonakdar, S. (2013). Colloids and surfaces A: physicochemical and engineering aspects. Colloids Surfaces A Physicochem. Eng. Aspects 431, 18–26. doi:10.1016/j.colsurfa.2013.04.003
Terreno, E., Castelli, D. D., Viale, A., and Aime, S. (2010). Challenges for molecular magnetic resonance imaging. Chem. Rev. 110 (5), 3019–3042. doi:10.1021/cr100025t
Torres Martin de Rosales, R., Tavaré, R., Glaria, A., Varma, G., Protti, A., and Blower, P. J. (2011). 99mTc-Bisphosphonate-Iron oxide nanoparticle conjugates for dual-modality biomedical imaging. Bioconjugate Chem. 22 (3), 455–465. doi:10.1021/bc100483k
Wahajuddin, S. A., and Arora, S. (2012). Superparamagnetic iron oxide nanoparticles: magnetic nanoplatforms as drug carriers. Int. J. Nanomedicine 7, 3445–3471. doi:10.2147/ijn.s30320
Wang, L., Chen, S., Zhu, Y., Zhang, M., Tang, S., Li, J., et al. (2018). Triple-Modal imaging-guided chemo-photothermal synergistic therapy for breast cancer with magnetically targeted phase-shifted nanoparticles. ACS Appl. Mater Interfaces 10 (49), 42102–42114. doi:10.1021/acsami.8b16323
Weissleder, R., and Pittet, M. J. (2008). Imaging in the era of molecular oncology. Nature 452 (7187), 580–589. doi:10.1038/nature06917
Wen, S. H., Zheng, F. Y., Shen, M. W., and Shi, X. Y. (2013). Surface modification and PEGylation of branched polyethyleneimine for improved biocompatibility. J. Appl. Polym. Sci. 128 (6), 3807–3813. doi:10.1002/app.38444
Wu, W., He, Q., and Jiang, C. (2008). Magnetic iron oxide nanoparticles: synthesis and surface functionalization strategies. Nanoscale Res. Lett. 3 (11), 397–415. doi:10.1007/s11671-008-9174-9
Wu, W., Wu, Z., Yu, T., Jiang, C., and Kim, W. S. (2015). Recent progress on magnetic iron oxide nanoparticles: synthesis, surface functional strategies and biomedical applications. Sci. Technol. Adv. Mater 16 (2), 023501. doi:10.1088/1468-6996/16/2/023501
Xiang, J. J., Tang, J. Q., Zhu, S. G., Nie, X. M., Lu, H. B., Shen, S. R., et al. (2003). IONP-PLL: a novel non-viral vector for efficient gene delivery. J. Gene Med. 5 (9), 803–817. doi:10.1002/jgm.419
Xiao, Y. T., Zhou, C., Ye, J. C., Yang, X. C., Li, Z. J., Zheng, X. B., et al. (2019). Integrin α6-targeted positron emission tomography imaging of colorectal cancer. ACS Omega 4 (13), 15560–15566. doi:10.1021/acsomega.9b01920
Xing, R., Liu, G., Zhu, J., Hou, Y., and Chen, X. (2014). Functional magnetic nanoparticles for non-viral gene delivery and MR imaging. Pharm. Res. 31 (6), 1377–1389. doi:10.1007/s11095-013-1205-2
Xu, C., Wang, Y., Zhang, C., Jia, Y., Luo, Y., and Gao, X. (2017). AuGd integrated nanoprobes for optical/MRI/CT triple-modal in vivo tumor imaging. Nanoscale 9 (13), 4620–4628. doi:10.1039/c7nr01064h
Yan, R., Hu, Y., Liu, F., Wei, S., Fang, D., Shuhendler, A. J., et al. (2019). Activatable NIR fluorescence/MRI bimodal probes for in vivo imaging by enzyme-mediated fluorogenic reaction and self-assembly. J. Am. Chem. Soc. 141 (26), 10331–10341. doi:10.1021/jacs.9b03649
Yang, C., Vu-Quang, H., Husum, D. M. U., Tingskov, S. J., Vinding, M. S., Nielsen, T., et al. (2017). Theranostic poly(lactic-co-glycolic acid) nanoparticle for magnetic resonance/infrared fluorescence bimodal imaging and efficient siRNA delivery to macrophages and its evaluation in a kidney injury model. Nanomedicine 13 (8), 2451–2462. doi:10.1016/j.nano.2017.08.007
Yang, Z., Duan, J., Wang, J., Liu, Q., Shang, R., Yang, X., et al. (2018). Superparamagnetic iron oxide nanoparticles modified with polyethylenimine and galactose for siRNA targeted delivery in hepatocellular carcinoma therapy. Int. J. Nanomedicine 13, 1851–1865. doi:10.2147/ijn.s155537
Yiu, H. H., Pickard, M. R., Olariu, C. I., Williams, S. R., Chari, D. M., and Rosseinsky, M. J. (2012). Fe3O4-PEI-RITC magnetic nanoparticles with imaging and gene transfer capability: development of a tool for neural cell transplantation therapies. Pharm. Res. 29 (5), 1328–1343. doi:10.1007/s11095-011-0632-1
Yoo, D., Lee, C., Seo, B., and Piao, Y. (2017). One pot synthesis of amine-functionalized and angular-shaped superparamagnetic iron oxide nanoparticles for MR/fluorescence bimodal imaging application. RSC Adv. 7 (21), 12876–12885. doi:10.1039/c6ra28495g
Yuan, H., Liang, H., Hou, P., and Li, J. (2021). Advanced nanomaterials for multimodal molecular imaging. Chem. Res. Chin. Univ. 37 (4), 840–845. doi:10.1007/s40242-021-1196-1
Zhang, W., Ding, X., Cheng, H., Yin, C., Yan, J., Mou, Z., et al. (2019). Dual-targeted gold nanoprism for recognition of early apoptosis, dual-model imaging and precise cancer photothermal therapy. Theranostics 9 (19), 5610–5625. doi:10.7150/thno.34755
Zhang, Y., Zhang, L., Song, X., Gu, X., Sun, H., Fu, C., et al. (2015). Synthesis of superparamagnetic iron oxide nanoparticles modified with MPEG-PEI via Photochemistry as new MRI contrast agent. J. Nanomater 2015, 1–6. doi:10.1155/2015/417389
Zhou, S. A., and Brahme, A. (2010). Development of high-resolution molecular phase-contrast stereoscopic X-ray imaging for accurate cancer diagnostics. Radiat. Prot. Dosim. 139 (1-3), 334–338. doi:10.1093/rpd/ncq012
Zhu, N., Ji, H., Yu, P., Niu, J., Farooq, M. U., Akram, M. W., et al. (2018). Surface modification of magnetic iron oxide nanoparticles. Nanomater. (Basel) 8 (10), 810. doi:10.3390/nano8100810
Keywords: BPEI modification, SPION, multimodal imaging, MRI, nanoparticles
Citation: Shen Q and Yu C (2024) Advances in superparamagnetic iron oxide nanoparticles modified with branched polyethyleneimine for multimodal imaging. Front. Bioeng. Biotechnol. 11:1323316. doi: 10.3389/fbioe.2023.1323316
Received: 17 October 2023; Accepted: 18 December 2023;
Published: 25 January 2024.
Edited by:
Luca Menichetti, National Research Council (CNR), ItalyReviewed by:
Roberto Francischello, University of Pisa, ItalyRiccardo Di Corato, Italian National Research Council, Italy
Copyright © 2024 Shen and Yu. This is an open-access article distributed under the terms of the Creative Commons Attribution License (CC BY). The use, distribution or reproduction in other forums is permitted, provided the original author(s) and the copyright owner(s) are credited and that the original publication in this journal is cited, in accordance with accepted academic practice. No use, distribution or reproduction is permitted which does not comply with these terms.
*Correspondence: Chunjing Yu, eWNqd3hkMTk3OEBqaWFuZ25hbi5lZHUuY24=