- 1Department of Vascular Surgery, The Second Hospital of Jilin University, Changchun, Jilin, China
- 2Department of Spine Surgery, The Second Hospital of Jilin University, Changchun, Jilin, China
The intricate healing process of skin wounds includes a variety of cellular and molecular events. Wound healing heavily relies on reactive oxygen species (ROS), which are essential for controlling various processes, including inflammation, cell growth, angiogenesis, granulation, and the formation of extracellular matrix. Nevertheless, an overabundance of reactive oxygen species (ROS) caused by extended oxidative pressure may result in the postponement or failure of wound healing. It is crucial to comprehend the function of reactive oxygen species (ROS) and create biomaterials that efficiently eliminate ROS to enhance the healing process of skin wounds. In this study, a thorough examination is presented on the role of reactive oxygen species (ROS) in the process of wound healing, along with an exploration of the existing knowledge regarding biomaterials employed for ROS elimination. In addition, the article covers different techniques and substances used in the management of skin wound. The future prospects and clinical applications of enhanced biomaterials are also emphasized, highlighting the potential of biomaterials that scavenge active oxygen to promote skin repair. This article seeks to enhance the understanding of the complex processes of ROS in the healing of wounds and the application of ROS-scavenging materials. Its objective is to create novel strategies for effective treatment skin wounds.
1 Introduction
Skin wound, caused by physical or heat-related causes and other possible factors, can cause the skin defect and hinder regular bodily functions (Shi et al., 2019). The healing of skin wound is a meticulous and intricate process that involves immune cells, non-immune cells, cytokines, growth factors, and extracellular component (Heher et al., 2018; Liang et al., 2023). Any disturbance in these factors can potentially result in chronic wound healing. The challenging problem of wound healing comes mainly from chronic wounds, especially those skin wounds caused by underlying physiological factors such as diabetes. The process of wound healing involves ongoing formation of new blood vessels, multiplication of cells, and restructuring of the wound tissue. In recent years, growing evidence indicates that reactive oxygen species (ROS) play a significant role in the physiological and pathological aspects of wound healing. ROS is involved in several skin tissue regeneration processes such as the regulation of inflammation, cell proliferation, angiogenesis, granulation, and the formation of extracellular matrix (Xu et al., 2020).
ROS consist of extremely active and oxidizing compounds, which encompass the superoxide anion (O2–), hydrogen peroxide (H2O2), and hydroxyl radical (−OH). Under normal physiological conditions, moderate levels of ROS participate in cellular signaling and immune responses. Nevertheless, under the influence of external factors such as injury, inflammation, or exposure to radiation, the generation of ROS escalates considerably, resulting in oxidative stress within cells and subsequent harm to skin tissues (Khorsandi et al., 2022). The overproduction and buildup of ROS beyond the cellular ability to counteract them obstructs the wound tissue shift from the inflammatory stage to the proliferative stage (Deng et al., 2019; Malone-Povolny et al., 2019). Consequently, wound area is chronically inflamed, resulting in delayed wound healing. Therefore, maintaining the homeostasis of REDOX in cells can prevent abnormal cell growth and immune dysregulation. Multiple research studies have shown that antioxidants have the potential to expedite the process of wound healing, especially when dealing with chronic wounds (Fitzmaurice et al., 2011; Yang et al., 2024). Therefore, the introduction of antioxidant component has emerged as an effective approach to expedite the healing of chronic wounds.
Due to the comprehensive understanding of the relationship between ROS and skin injuries, the use of ROS removal materials to repair skin wounds has attracted great attention. ROS-scavenging materials have the capability to eliminate surplus ROS, relieving cellular and tissue harm caused by oxidative stress and enhancing the recovery of skin wounds. These substances have the ability to trap, counteract, or hinder the production of ROS, thereby restoring internal redox equilibrium, diminishing inflammatory reactions, and promoting cellular growth and rejuvenation. In this review, recent progress in using ROS-scavenging materials for repair skin wounds is presented. The discussion primarily focuses on the correlation between skin wound healing and ROS. Subsequently, the characteristics and applications of different ROS-scavenging materials in skin wound repair are highlighted. In conclusion, this paper discusses the obstacles encountered and the prospect of future applications in the use of ROS-scavenging materials, with the goal of offering useful insights and recommendations for future studies of ROS-scavenging materials.
2 Factors that influence wound healing
The skin is the body’s largest organ, comprising the epidermis, dermis, and subcutaneous tissue. The outer layer of the skin acts as a shield, safeguarding against dehydration and external irritations. The dermis provides flexibility, support, and houses blood vessels, nerve fibres, and lymphatic systems. The subcutaneous layer contains adipose tissue and rich vascularization, contributing to temperature regulation (Xu et al., 2020). The process of wound healing is a dynamic and organized process that can be classified into acute and chronic wounds according to their characteristics (Table 1).
The process of skin wound healing consists of four separate stages: a) stopping bleeding, b) triggering inflammation, c) promoting cell growth, and d) restructuring (as shown in Figure 1). During the hemostasis stage, the activation of coagulation factors occurs, resulting in the creation of a fibrin clot that offers structural assistance to the damaged tissue. In the phase of inflammation, different types of immune cells, such as inflammation cells, lymphocytes, monocytes, and macrophages, collaborate to eliminate waste from the site of injury. At this stage, neutrophils in the injured area release ROS, antimicrobial peptides, proteolytic enzymes and other substances, and carry out phagocytosis to remove dead cell tissues, foreign bodies and microorganisms in the injured area (Sorg et al., 2017; Raziyeva et al., 2021). At the same time, neutrophils can continuously release pro-inflammatory factors, forming a positive feedback mechanism, prompting more neutrophils to accumulate to the injury site (Hassanshahi et al., 2022). After necrotic tissue and pathogens are removed, neutrophil proliferation is reduced and forced out of the wound. At the same time, the phenotype of macrophages also gradually changed from M1 type, which promoted the release of inflammatory factors, to M2 type, which promoted angiogenesis and tissue regeneration, and wound repair also entered the proliferative stage (Chen C. et al., 2023; Ead and Armstrong, 2023). During the proliferation stage, epithelial cells and fibroblasts undergo active proliferation and migration, gradually filling the exposed wound by forming granulation tissue. During this stage, the combination and placement of components in the extracellular matrix occur, along with the creation of fresh blood vessels. Ultimately, during the maturation or remodelling stage, the granulation tissue is progressively substituted with collagen fibres, leading to the creation of connective tissue (Werner and Grose, 2003; Bainbridge, 2013; Mascharak et al., 2020). It is worth mentioning that these stages do not necessarily occur in separate time periods but instead partially coincide.
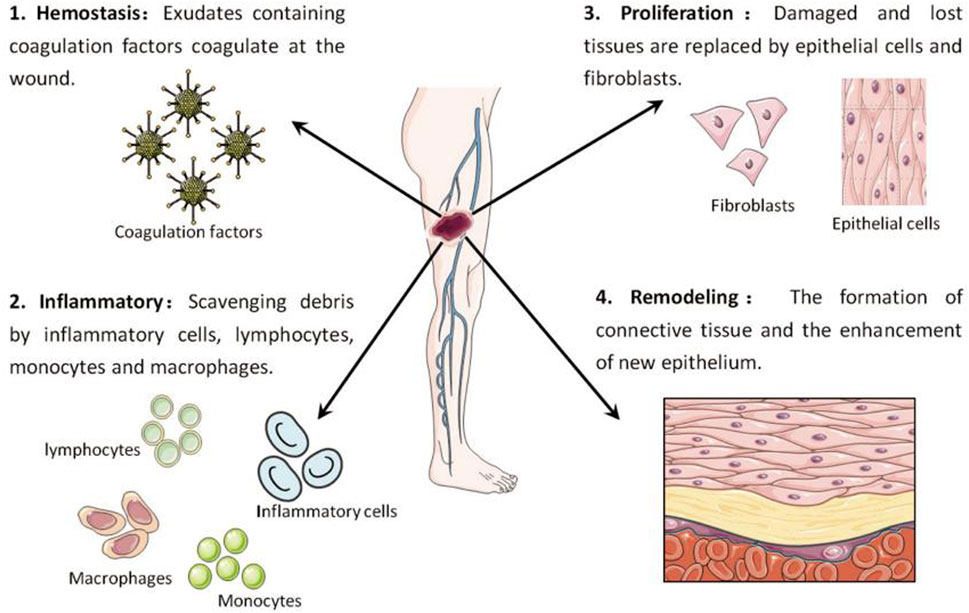
FIGURE 1. Key stages in the wound healing process: Hemostasis (1), inflammation (2), proliferation (3), and remodelling (4) (Xu et al., 2020).
In contrast, chronic wounds are wounds that do not heal correctly for a long time, usually without any inclination to regain functional integrity within a 3-month timeframe (Malone-Povolny et al., 2019). The process of healing is not fully complete, with one or more stages, such as hemostasis, inflammation, proliferation, or remodelling, being extended. Studies have indicated that the liquid present in chronic trauma can impede the development of keratinocytes and fibroblasts (Widgerow, 2013). Cell proliferation and migration are severely hindered due to inadequate release of growth factors. Chronic wounds occur when cellular damage and death surpass the ability of the epidermis to grow and regenerate. Chronic illnesses, vascular problems, diabetes, neuropathy, malnourishment, stress, infections, and edema are among the established elements that impact the skin wound recovery procedure (Zhao et al., 2016; Alam et al., 2021).
3 The significance and influence of reactive oxygen species in skin regeneration
Wound healing is a natural biological process that occurs when skin tissue is damaged. This process involves the constriction of blood vessels and clot formation, followed by inflammation, cell growth, and finally, the remodelling of the wound. Oxygen is essential in nearly every phase of the wound healing process. This is because energy is needed for various processes involved in wound repair, such as biosynthesis, intracellular transport, and cell motility. ATP synthesis, which acts as the main source of energy, is optimized when oxygen is present. With the process of wound healing, there is an increase in the growth of cells and the generation of the extracellular matrix (ECM), leading to a greater need for energy. Consequently, the demand for oxygen also rises to meet the heightened energy requirements during wound healing (Hunt et al., 1969). Oxygen plays a vital role in the process of wound healing, as it not only supplies energy but also acts as an essential necessity for numerous important enzymatic reactions (Dissemond et al., 2015; Lee et al., 2022).
Mitochondria, the endoplasmic reticulum (ER), peroxisomes, various oxidases, and phospholipid metabolism are all potential sources of ROS. In addition, the NADPH oxidase (NOX) family can generate ROS by means of specific enzymes (Bedard and Krause, 2007). Nitrous oxide enzymes are located on cell membranes and can help electrons pass through biofilms, a process that leads to the reduction of oxygen and the production of superoxide (O2–). Subsequently, superoxides are able to chemically react to form various reactive oxygen species, such as hydrogen peroxide (H2O2), peroxyradicals (HO2–), and hydroxyl radical. These ROS play diverse roles in cellular functions such as differentiation, proliferation, apoptosis, migration, and contraction (Soneja et al., 2005; Roy et al., 2006). The ROS group includes oxygen derivatives such as hydroxyl radicals (OH·), peroxides, superoxide anions, and hydrogen peroxide (H2O2). During the non-healing phase of wounds, excessive ROS production occurs, and these ROS molecules oxidize neighboring molecules or cellular components, leading to cellular damage. ROS have been widely regarded as major contributors to cellular damage during the aging process (Beckman and Ames, 1998). Studies have shown that while a moderate amount of reactive oxygen species (ROS) helps maintain intracellular balance, increased ROS levels can have a negative impact on the wound healing process (Zhu et al., 2019). To put it differently, as long as ROS levels remain constant, the integrity and functionality of cells aremaintained. However, ROS also have beneficial effects. The coordinated production of ROS by immune cells is crucial for effective host defense and is essential for cellular signal transduction (Jones et al., 2018; Koo et al., 2019). During the homeostasis phase, ROS produced by NADPH oxidase in blood vessel cells can stimulate chemotactic and adhesion molecule expression, thereby reducing local blood flow (Hoffmann and Griffiths, 2018). During the inflammatory phase, superoxide and H2O2 produced by neutrophils and macrophages play a crucial role in bacterial killing and preventing wound infection. ROS also has the ability to stimulate tumor necrosis factor-α (TNF-α) and platelet-derived growth factor (PDGF) release and support cell migration (Hoffmann and Griffiths, 2018; Xu et al., 2020; He et al., 2022; Khorsandi et al., 2022). REDOX signaling is also required during the proliferation phase. ROS mediates the tissue growth factor-α 1 (TGF-α1) signaling pathway, improving the expression of fibroblast growth factor (FGF), and promoting the proliferation and migration of fibroblasts and the synthesis and migration of collagen and fibronectin. In addition, ROS can stimulate angiogenesis, endothelial cell division and migration by expressing vascular endothelial growth factor (VEGF), and promote blood vessel formation. Therefore, maintaining an optimal level of reactive oxygen species (ROS) is crucial for fighting against microorganisms and ensuring the survival of cells (as shown in Figure 2).
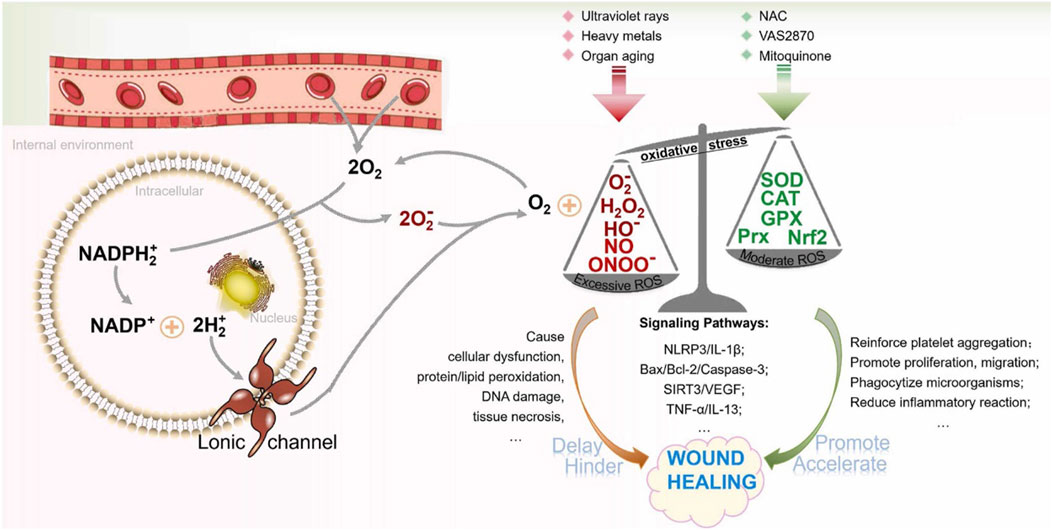
FIGURE 2. In wound healing, the function of ROS is depicted in figure. Limiting ROS levels to the normal range has a beneficial effect on the wound healing process. Too much reactive oxygen species (ROS) can hinder the cell growth process and cause tissue damage, hindering the wound healing (Wang et al., 2023).
An increase in ROS at the wound site may lead to the formation of chronic inflammation. Overactivation of ROS can trigger the functioning of transcription factors, such as activator protein 1 (AP-1), mitogen-activated protein kinases (MAPKs), nuclear factor kappa B (NF-κB), and nuclear factor erythroid 2-like 2 (Nrf2) (An et al., 2018; Asai et al., 2018). Nrf2 plays a vital role in protecting against elevated levels of internal oxidative stress and greatly contributes to the healing of wounds (Koike et al., 2020). On the other hand, the stimulation of NF-κB and AP-1 enhances the amount of matrix metalloproteinases (MMPs) in dermal fibroblasts, leading to the degradation of the extracellular matrix (ECM) and a delay in the healing process of wounds. ROS affect the inflammatory reaction in diabetic models by means of the NLR family pyrin domain-containing 3 (NLRP3)-IL-1β pathway (Cheng et al., 2012). Some mechanism studies have also shown that reactive oxygen species ROS hinder the wound recovery process and increase the degree of tissue damage by counteracting the effects of cytokines such as vascular endothelial growth factor (VEGF) and tumor necrosis factor-α (TNF-α) (Lord et al., 2017; Seiwerth et al., 2018).
At present, there is a notable desire to restore ROS levels to enhance the compromised physiological conditions around injuries and facilitate the process of wound recovery. In regards to the problem of excessive accumulation of reactive oxygen species (ROS) near wounds, we provide an overview of the frequently employed materials for ROS removal. These materials are designed to promote cell growth and movement, thereby accelerating the healing process of wounds by decreasing ROS levels within a specific range.
4 ROS-scavenging materials
We highlight some natural or synthetic materials for ROS removal and describe their properties in detail. The materials commonly used for ROS removal are shown in Table 2.
4.1 Carbon matrix materials
After the discovery of fullerenes, a variety of biomaterials made of carbon, such as carbon nanotubes (CNTs), carbon particles, carbon nanoclusters, graphene, graphene quantum dots (GQDs), and carbon quantum dots (CQDs), have been extensively studied and researched (Nash and Ahmed, 2015). Nanomaterials composed of carbon matrices, serving as a novel category of nanomases utilized in biochemical reactions, exhibit enhanced operational durability and reduced expenses compared to natural enzymes. In the meantime, they are easily prepared and exhibit strong resistance to demanding conditions. Notably, fullerenes and their derivatives have demonstrated effective elimination of hydroxyl radicals and superoxide anions. The special physical and chemical properties of carbon-based biomaterials, such as their large specific surface area, good hydrophilicity, and ability to be modified by oxygen-containing functional groups, have attracted great interest and have been widely used in biomedical applications related to ROS.
Fullerenes, also known as buckyballs, are carbon-based molecules that have a spherical shape and possess strong antioxidant properties (Chen X. et al., 2023). These unique structures have the ability to effectively eliminate various ROS, including hydroxyl radicals and superoxide anions. Nanoparticles composed of fullerene have the ability to hinder cytotoxic effects and prevent damage to mitochondria and lipid peroxidation (Yin et al., 2009). Yin et al. (2009) conducted a study where they prepared and assessed three water-soluble variations of fullerenes to determine their effectiveness in eliminating ROS. The three types of fullerenes, specifically C60[C(COOH)2]2, C60(OH)22, and Gd@C82(OH)22, exhibited the capacity to safeguard cells against oxidative harm caused by H2O2, maintain the potential of the mitochondrial membrane, and hinder the production of intracellular ROS. Furthermore, the clearance efficiency of ROS was assessed for the three substances, where Gd@C82(OH)22 demonstrated the most superior effectiveness, trailed by C60(OH)22 and C60[C(COOH)2]2. In vitro, these compounds were also discovered to efficiently eliminate stable DPPH (2,2-diphenyl-1-picrylhydrazyl) radicals and ROS and hinder lipid peroxidation. The results indicated that fullerene derivatives have the potential to be used as protectants and treatments for cells in living organisms.
There are also other carbon matrix materials, such as carbon nanotubes (CNTs). CNTs are hollow tubular structures formed from carbon atoms that have excellent electrical conductivity and mechanical strength. Some types of CNTs show good antioxidant activity, clearing ROS and protecting cells from oxidative stress (He et al., 2021). Graphene is a carbon-based substance consisting of a solitary layer of carbon atoms, possessing exceptional electrical conductivity and chemical stability. Graphene and its derivatives exhibit remarkable capability in scavenging ROS and can effectively neutralize various types of ROS (Tang et al., 2019). CQDs are tiny carbon substances that exhibit strong fluorescence and are compatible with living organisms. Some specific CQDs possess antioxidant characteristics and are capable of eliminating ROS and diminishing oxidative stress (Li et al., 2023). Black phosphorus is a carbon material at the nanoscale that possesses a significant surface area and strong adsorption capability. As an antioxidant, it has the ability to absorb and counteract ROS (Huang et al., 2022). Various Carbon matrix materials have different mechanisms and characteristics in eliminating ROS. In the biomedical domain, these materials are extensively researched and employed for the purpose of controlling oxidative stress and safeguarding cells against oxidative harm.
4.2 Metal nanoparticles
The research on the elimination of reactive oxygen species in metal-containing biomaterials has made substantial progress because of the discovery of gold nanoparticles with the function of removing ROS, as well as Fe3O4 nanoparticles with peroxidase activity (Ju et al., 2023; Xi et al., 2023). Metal-based materials are a class of inorganic antioxidants with strong ROS scavenging ability. The biomaterials made of metal have catalytic characteristics that resemble peroxidase (POD), catalyst (CAT), superoxide dismutase (SOD), glucose oxidase (GOx), and glutathione peroxidase (GPx), allowing them to effectively eliminate ROS.
CeO2 NPs, known as cerium nanoparticles, possess significant photolytic and antioxidant characteristics, which make them a potential therapeutic option for a range of ailments, including wound healing and other medical conditions (Wang et al., 2020; Xu et al., 2023). Earlier research on CeO2 has discovered its ability to enhance the growth and migration of cells while also balancing the levels of ROS in chronic ulcer, consequently accelerating the process of wound healing (Xu et al., 2023). Bhang et al. prepared a Cu-deposited cerium oxide nanoparticle (CuCe NPs), which showed strong antioxidant effect, significantly promoted the anti-inflammatory effect and M2 polarization of macrophages, and alleviated tissue damage (Im et al., 2023). Furthermore, CeO2 nanoparticles have the capability to imitate the antioxidant function of superoxide dismutase (SOD) and catalase (CAT), potentially expediting the process of wound healing (Rather et al., 2018). The mixed valence states of Ce3 and Ce4 are responsible for the antioxidant characteristics of cerium oxide nanoparticles. Cerium oxide nanoparticles have become widely utilized inorganic nanomaterials due to their exceptional antioxidant characteristics and capacity for automatic regeneration (Huang et al., 2021) (Figure 3).
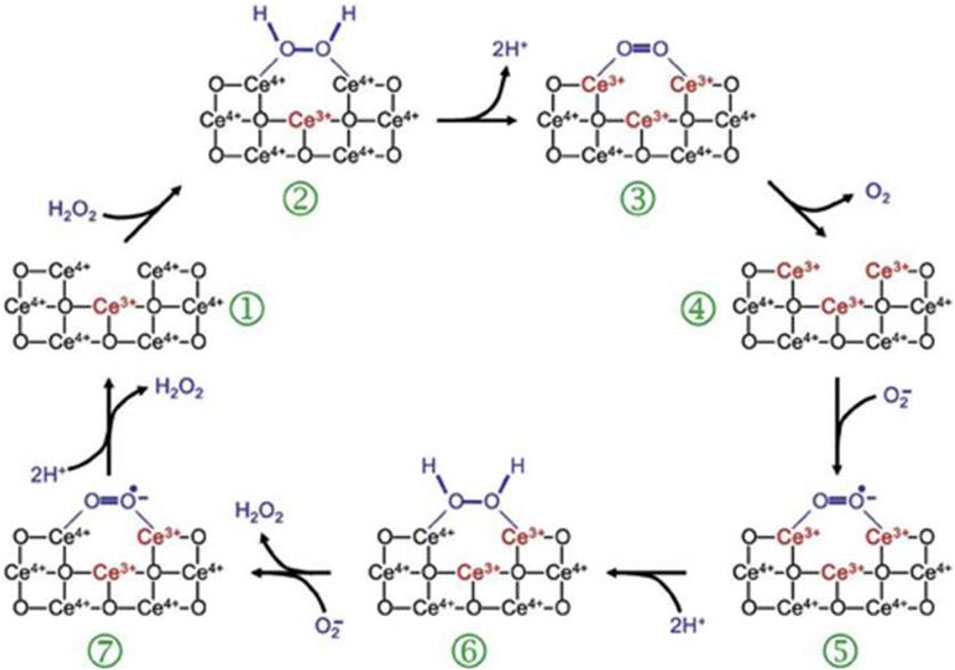
FIGURE 3. ROS-scavenging mechanism of CeO2 NPs (Celardo et al., 2011).
Platinum (Pt) nanoparticles are frequently employed as catalysts in synthetic chemistry for hydrogenation and oxidation reactions. Additionally, Pt is an inherent element of cisplatin and other chemotherapy drugs. Research has indicated that Pt has the ability to facilitate the transformation of O2– into H2O2, as well as the transformation of H2O2 into H2O and O2 (Umeda et al., 2011; Yan et al., 2023). Consequently, it is regarded as a promising SOD/CAT simulator for managing oxidative stress. Kim et al. (2010) connected Pt with the HIV-1 TAT fusion protein and found that Pt can improves cell endocytosis, produces antioxidant effects, and has higher bioavailability and lower toxicity. Moreover, studies have shown that TAT-Pt nanoparticles (TAT-Pt NPs) can increase the nematode survival rate under acute oxidative stress induced by paraquat, as well as in the presence of chronic endogenous ROS. These results suggest that TAT-Pt nanoparticles have the potential to be an effective drug for the treatment of chronic inflammation.
Copper nanoparticles, found in superoxide dismutase and glutathione, boost the effectiveness of SOD and other enzymes, enhancing the body’s capacity to eliminate harmful free radicals. Copper impacts distinct signaling pathways in living organisms, such as the nuclear factor E2-associated factor (Nrf) signaling pathway and the mitochondrial signaling pathway mediated by CDK4. By means of this phenomenon, the body can maintain homeostasis and avert inflammatory ailments (Iskra and Majewski, 2000; Wang et al., 2015).
Moreover, various inorganic nanoparticles, such as gold, selenium, and vanadium, demonstrate impressive abilities to scavenge ROS. The metal nanoparticles exhibit the capacity to efficiently combat environments with oxidative stress and contribute to safeguarding cells (Vernekar et al., 2014; Rosenkrans et al., 2020). However, the use of metal-based biomaterials for ROS removal in the clinic is limited due to poor blood solubility, lack of stability, and the risk of unknown toxicity in vivo over a long period of time.
4.3 Small antioxidant molecules
Natural organic small molecule ROS scavengers can be extracted from natural sources such as fruits, vegetables, herbs, tea leaves, and dietary supplements. The FDA has approved certain natural compounds for the treatment of diseases associated with oxidative stress. These compounds include catechins, baicalin, vitamins A, C, and E, coenzyme Q10 (CoQ10), and N-acetylcysteine (NAC) (Firuzi et al., 2011; Mut-Salud et al., 2016). In addition, many artificial organic small molecule reactive oxygen scavengers have also been applied to remove reactive oxygen species. For example, butylated hydroxyanisole (BHA), butylated hydroxytoluene (BHT), and their analogues are used as synthetic ROS scavengers (Dassarma et al., 2018). Nevertheless, the utilization of these artificial substances is restricted because of the potential dangers they may present, such as liver toxicity, kidney toxicity, and carcinogenic risks.
TEMPO is a widely recognized reactive oxygen species (ROS) scavenger consisting of oxygen and nitro radicals such as OH and O2 (Fink et al., 2007; Nag et al., 2022). TEMPO seizes unmatched electrons from other unbound radicals via nitrogen oxide’s single-electron transfer, causing the redox reaction to alternate between the oxidized form of nitrogen oxide, oxammonium cation, and hydroxylamine (Soule et al., 2007). Hence, scientists have been committed to creating polymer nanomaterials with antioxidant properties by incorporating TEMPO or its derivatives into the polymer structure and utilizing them for the management of diverse ailments (Yamada et al., 2003; Marciniak et al., 2016).
4.4 Antioxidant enzymes
Several natural enzymes, such as superoxide dismutase (SOD), catalase (CAT), and glutathione peroxidase (GPx), have the capacity to remove ROS. These enzymes can facilitate the breakdown of ROS, thereby reducing oxidative damage. Superoxide dismutase (SOD) is a vital enzyme with antioxidant properties that are essential for combating oxidative stress. SOD, as an intrinsic factor, has the ability to clear free radicals, and contributes to the differentiation of superoxide free radicals, converting them into hydrogen peroxide (Zhang W. Q. et al., 2021). Glutathione peroxidase (GPx) enzymatically reduces H2O2 and organic peroxides, relying on glutathione as an electron donor. Humans possess a total of eight GPx genes, namely, GPX1-8. Among these, GPX1-4 are selenoproteins that include selenocysteine (SeCys) residues within their catalytic core, as stated in reference (Brigelius-Flohé and Maiorino, 2013). Wound injuries frequently show reduced levels of glutathione and GPx activity because GPx relies on glutathione as an electron provider. Despite the reduced levels of GPX1 protein in damaged skin, GPX1 mRNA expression is increased in wound injuries, and both protein and GPx levels are decreased in normal rat wounds (Steiling et al., 1999; Iuchi et al., 2010).
4.5 Natural materials
Bilirubin is a metabolite naturally present in the human body, which is both an intrinsic antioxidant and a molecule involved in regulation. Under conditions of oxidative stress, insoluble bilirubin undergoes oxidation to form soluble biliverdin. Bilirubin can effectively inhibits the production of ROS through the NOX pathway and inhibits lipopolysaccharide (LPS) -mediated inflammation by targeting the REDOX sensitive transcription factor hypoxia-Inducible Factor-1α (HIF-1α) (Idelman et al., 2015). To enhance the antioxidant properties of bilirubin, Jon et al. utilized covalent bonding and self-assembly methods to create nanoparticles consisting of bilirubin conjugated with polyethylene glycol (PEG-BR NPs) (Kim et al., 2017). PEG-BR nanoparticles not only maintain the antioxidant capacity of natural bilirubin, but also show greater stability and durability in living organisms. Moreover, they promote the polarization of M1 macrophages towards the M2 phenotype through the inhibition of inflammatory cytokine secretion, thereby alleviating inflammation. In summary, because of its beneficial properties as an antioxidant and anti-inflammatory agent, bilirubin presents a promising approach for treating inflammatory conditions.
Polydopamine (PDA) is a melanin-like substance that has antioxidant properties. At present, researchers have found that PDA may have the effect of scavenging free radicals due to the REDOX chemistry of its polyphenol structure, as well as endogenous free radical tolerance and effective energy transfer properties (Hu et al., 2020). The catechol component has the ability to neutralize free radicals by providing hydrogen atoms to the phenol hydroxyl group and promoting a reduction reaction by electron transfer. The interaction between the produced phenoxyl radicals and secondary quenching radicals results in the creation of enduring quinone structures. PDA is often utilized to scavenge ROS in the treatment of conditions resulting from oxidative stress. PDA NPs are renowned for their ability to scavenge free radicals and can be employed to counteract different forms of ROS. PDA provide a convenient and efficient method for creating coatings on various material surfaces. These coatings possess a wide range of impressive biological characteristics, such as anti-inflammatory, anticancer, and antioxidant properties (Dai et al., 2019). When using natural materials as ROS scavengers in vivo, the biocompatibility and biodegradability of the materials should be considered. It is very important to take inspiration from natural materials that have an innate ability to remove ROS, as this will greatly facilitate the creation and development of ROS-scavenging materials.
5 Application of ROS-scavenging materials in wound healing
ROS scavenging materials have shown the ability to effectively regulate ROS levels during injury, manage oxidative stress, and promote wound healing. Over the past few years, many scientists have studied different substances with antioxidant properties to promote the process of wound healing. These materials possess diverse characteristics and functions, enabling them to promote wound healing through various mechanisms, such as enhancing cell proliferation and migration, modulating inflammatory responses, facilitating angiogenesis, and promoting collagen synthesis. In this chapter, we will discuss the application of these materials in skin wound repair (Figure 4).
5.1 Utilizing nanomaterials for the purpose of promoting wound healing
In the context of skin wound healing, the application of nanomaterials has shown great potential in promoting wound healing through multiple mechanisms. Nanomaterials possess a large surface area-to-volume ratio and highly tunable physical properties, which can enhance cell adhesion and migration, thereby promoting epithelial cell healing and regeneration at the wound site. In this subsection, we will primarily focus on discussing the applications of nanomaterials in facilitating skin wound healing. We will introduce different types of nanomaterials, including metallic nanoparticles and nonmetallic nanoparticles, and provide detailed descriptions of their mechanisms in enhancing cell adhesion and migration, modulating inflammatory responses, promoting angiogenesis, and facilitating collagen synthesis.
According to Rather et al. (2018), CeNPs actively combat cellular oxidative harm by efficiently eliminating ROS. In some studies, polycaprolactone (PCL)-gelatin nanofibers (PGNPNF) were prepared by electrospinning technology, and their antioxidant capacity was tested. The XRD analysis indicated a reduction in crystallinity of approximately 2.6 times when compared to the original PCL, which facilitated the quick degradation of nanofibers and the subsequent release of CeNPs. PGNPNF meshes exhibited superoxide dismutase (SOD)-mimetic activity. The proliferation of 3T3-L1 cells on PGNPNF meshes was confirmed to have increased by approximately 48% according to SEM analysis. Ma et al. employed electrostatic interactions to develop a biocompatible nanocomposite called MoS2−CeO2, formed by combining PEG-MoS2 and CeO2 (Ma et al., 2021). Upon histological examination of tissue samples from the nanocomposite and near-infrared laser-treated groups, it was observed that inflammation was decreased, fibroblast migration was enhanced, and collagen fibres were densely arranged in a parallel manner. This was in stark contrast to the control group, where collagen deposition appeared uneven. The control group showed lower collagen synthesis than all treatment groups.
In summary, MOS2-CEO2 nanocomposites combined with near-infrared 808 nm laser therapy exhibit significant antibacterial, antioxidant, anti-inflammatory and regenerative properties, ultimately promoting rapid healing of chronic skin injuries. Sun and his colleagues synthesized graphene quantum dots (GQDs) materials and found that GQDs exhibit peroxisase-like properties because they are able to help break down H2O2 and have strong antibacterial effects (Sun et al., 2014). In all in vitro studies, GQDs showed strong inhibitory effects against both gram-negative bacteria (E. coli) and Gram-positive bacteria (Staphylococcus aureus). By utilizing these characteristics, band-aids based on GQDs were created, showcasing remarkable antibacterial efficacy in living organisms (Figure 5). Using a similar strategy, hybrid nanozymes consisting of Au-g-C3N4 were synthesized for antibacterial applications (Wang et al., 2017). The hybrid nanozymes displayed enhanced peroxidase-like activity due to the synergistic effect of combining Au nanoparticles with C3N4 sheets. Moreover, this collaborative impact enabled a decrease in the necessary amount of H2O2 for antibacterial uses. In vitro, the research displayed impressive bactericidal effects on both gram-negative and gram-positive bacteria. In addition, it effectively degrades existing bacterial biofilms and hinders the formation of new ones.
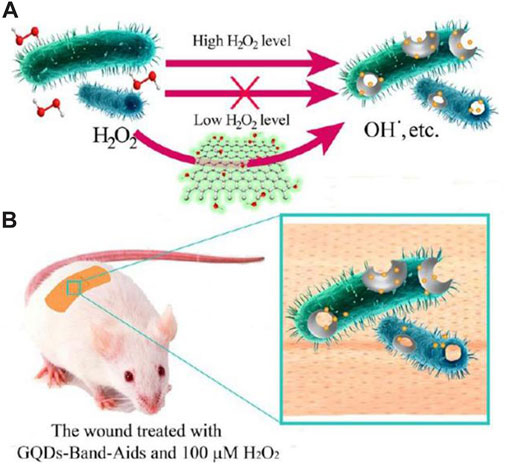
FIGURE 5. (A) The designed system based on GQDs and low level of H2O2 for the antibacterial application. (B) The GQD-Band-Aids used in wound disinfection in vivo (Sun et al., 2014).
Li et al. (2021) prepared a hybrid hydrogel (β-GO/RB/PVA HD) consisting of oxidized graphene, rose bengal, and polyvinyl alcohol. A comprehensive assessment was conducted on the mechanical characteristics and antibacterial effects activated by light from the hydrogel. The β-GO inorganic mesh intertwined with the porous framework of PVA, leading to a notable enhancement in the hydrogel’s mechanical characteristics. Both in vitro and in vivo experiments have shown that the heat generated by β-GO under 808 nm light irradiation and the ROS generated by RB under 550 nm light irradiation can produce significant antibacterial effects in as little as 10 min. In addition, the mixed hydrogels significantly accelerated the healing of infected wounds.
In the same way, substances such as platinum, copper and Prussian blue are also highly antioxidant due to their ability to mimic natural antioxidant enzymes such as catalase and superoxide dismutase (SOD). The utilization of these carriers can be employed to investigate wound healing activities. In addition, antioxidant nanomaterials can also serve as metal-organic frameworks (MOFs), where the highly permeable arrangement of MOFs allows unpaired electrons to interact with active sites within the material, enhancing the catalytic performance of these unpaired electrons (Cheng et al., 2017).
5.2 Skin wound therapeutic application of enzymes and peptides with antioxidant properties
As stated in the preceding section, superoxide dismutase (SOD) is an antioxidant enzyme that plays a role in combating oxidative stress. In the process of chronic wound healing, insufficient synthesis of superoxide dismutase (SOD) will aggravate oxidative stress and hinder effective wound healing. Research has shown that the use of hydrogels containing SOD significantly improves the recovery of diabetic ulcers (Zhang et al., 2018). Similarly, in a mouse burn model, plasma thiobarbituric acid reactivity (TBAR) metabolite levels were significantly reduced by intravenous administration of 10 mg/kg Cu/Zn-SOD[poly (butyl ester) -coupled SOD] (Fitzmaurice et al., 2011). Compared to the control rats, the animals treated with SOD exhibited a 7-day improvement in survival.
Antioxidants and antimicrobial peptides are also commonly used in the treatment of skin wounds, and their effects include fighting oxidative substances, regulating the production of cytokines, aiding cell movement and growth, and promoting the formation of new blood vessels (Steinstraesser et al., 2008). Cai et al. (2021) improved cell sensitivity by constructing Gj-CATH3 peptide analogues. Gj-CATH3 analogues showed high antioxidant activity. However, experiments on cytotoxicity and hemolysis demonstrated a notable decrease in toxicity. Additional in vitro investigations conducted on HaCaT cells revealed a noteworthy enhancement in cellular growth. Preclinical experiments additionally demonstrated impressive effectiveness in healing wounds, along with a significant increase in the activity of superoxide dismutase (SOD) (Figure 6). Calycosin-7-glucoside (CG) is an isoflavone component found in Astragalus membranaceus (AR), commonly known as Huangqi. Chen J. et al. (2023) investigated the mechanism of CG in treating diabetic wounds by using a diabetic wound model and an LPS- and IFN-γ-induced inflammation model in RAW264.7 cells. The study results showed that CG accelerated wound healing and promoted granulation tissue regeneration in diabetic mice. Protein chip technology revealed that CG increased the production of M-CSF, G-CSF, GM-CSF, IL-10, IL-13, and IL-4 but did not increase MCP-1, IL-1β, IL-1α, TNF-α, and TNF-RII. Furthermore, CG increased the proportion of anti-inflammatory Ly6CLo/- monocytes in peripheral blood and M2 macrophages in the wound. ELISA and flow cytometry analysis showed that CG enhanced the expression levels of IL-10, VEGF, CD206, and Arg-1 while significantly reducing the levels of IL-1, IL-6, IL-12, TNF-α, CD86, and iNOS. CG promoted the recruitment of anti-inflammatory monocytes and reduced the mitochondrial glycolysis rate, thus inducing M2 macrophage polarization through the ROS/AMPK/STAT6 pathway. These findings suggest that CG may be a promising therapeutic agent for diabetic wound treatment. According to Cao et al. (2018), cathelicidin-OA1 from Odorrana andersonii, which is a cathelicidin known for its strong antioxidant properties, was discovered. Cell proliferation in HaCaT and fibroblasts was observed in vitro, and it was found to be dependent on both the dose and time. The histopathological findings on live tissues during the initial phases of wound healing revealed improved tissue re-epithelialization and the formation of granulation. The experimental rats showed greater wound-healing ability than the other rats. In addition, elevated levels of TNF-α and TGF-β were observed in the experimental group, which can cause growth factors and cells to be attracted to the injury site, ultimately enhancing the wound healing rate.
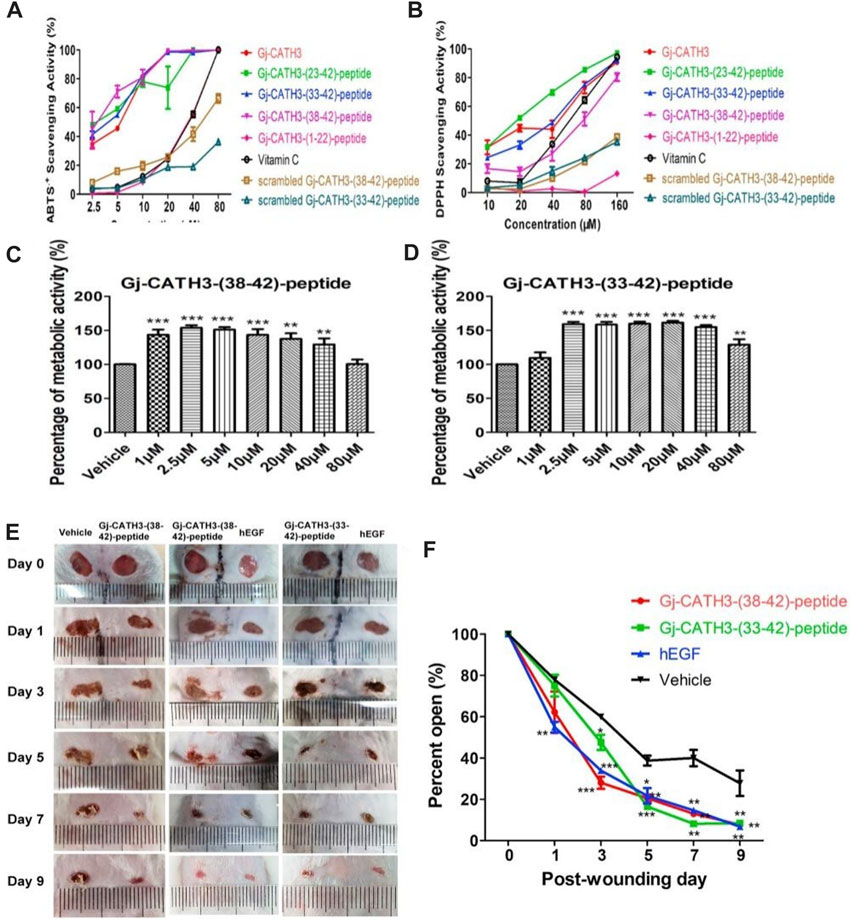
FIGURE 6. Biocompatibility and antioxidant activity of Gj-CATH3 peptide analogs in vivo and in vitro. (A) ABTS + free radical and (B) DPPH free radical scavenging activity of Gj-CATH3 peptide analogs. (C,D) Effect of Gj-CATH3 peptide analogs on the proliferation of HaCaT cells. In vivo assessment of the Gj-CATH3 peptide analogs for wound healing (E) Photographic snapshots of temporal development of healing wounds for the different Gj-CATH3 peptide analogs in 0–9 days. (F) Wound closure rate of different Gj-CATH3 peptide analogs at different healing times (Cai et al., 2021).
Hussain et al. (2021) created a dopamine-modified multidomain peptide (DAP) that possesses numerous advantageous characteristics for the process of wound healing. The attributes encompass the ability to combat microbes, the capacity to scavenge reactive oxygen species (ROS), and the ability to adhere. Cell proliferation was significantly increased by treating 3T3 fibroblasts with DAP. In the mouse full-thickness model, the immunohistochemical analysis revealed a notable decrease in pro-inflammatory indicators such as IL-6 and TNF-α among the rats treated with DAP. Rats treated with DAP exhibited significant growth of blood vessels and accumulation of collagen. In the study conducted by Zhang J. et al. (2021), a new antioxidant peptide was obtained from blackfish. Among the four tested peptides, SDGSNIHFPN and PGLMLGGSPPGLLGGSPP exhibited the most pronounced antioxidant properties. The studies on cell viability showed that they have the ability to shield cells from damage caused by H2O2. Additionally, the investigation revealed that these peptides not only functioned as powerful antioxidants through the activation of the KEAP1-Nrf2 signaling pathway but also displayed promise as a therapeutic approach to enhance the speed of wound healing.
5.3 Utilize free radical capture treatment for the healing of wounds
Free radical capture therapy effectively reduces ROS levels by introducing materials with antioxidant properties, thereby reducing oxidative stress and the inflammatory response and promoting the wound healing process. These ROS-scavenging materials can work through a variety of mechanisms. First, they can react directly with ROS, neutralizing harmful free radicals and thereby reducing tissue damage and inflammation. Second, they can also regulate the balance of ROS production, prevent excessive ROS production, maintain redox balance, and facilitate normal cell function and wound healing. In this chapter, we will focus on the use of free radical capture therapy to promote skin wound healing. Various natural enzymes, including glutathione (GSH) peroxidase, superoxide dismutase (SOD), and catalase, have been discovered to possess the capability of diminishing levels of free radicals. Free radical scavengers interrupt the peroxide chain by interacting with free radicals. Nevertheless, in the presence of a substantial quantity of unbound radicals (e.g., O2–), this mechanism will be impeded. Therefore, as the disease progresses, the body experiences the accumulation of free radicals that hinder the action of these enzymes. Therefore, the solution to this dilemma must use antioxidants.
Due to their identical enzymatic catalytic activity to SOD, PDA NPs exhibit enhanced stability and durability. It is extensively utilized in the management of ailments resulting from oxidative stress. Jing et al. (2020) developed 2D nanosheets (NSs) composed of polydopamine (PDA), referred to as PDA NSs. PDA NSs has the ability to scavenge free radicals such as O2–, ABTS and DPPH. In addition, PDA NSs showed significant antioxidant activity against O2 free radicals. Furthermore, PDA NSs exhibited anti-inflammatory characteristics. In vivo experiment results showed that on the 14th day of administration of 60 μg/mL experimental animals, the wound was completely healed without obvious scar formation. The histological assessment of the treatment group revealed a notable reduction in inflammatory cells and an increased level of collagen accumulation at the site of the wound in comparison to the control group. Fu Y. et al. (2021) developed a plan to improve the antioxidant capability of polydopamine nanoparticles (PDA NPs) through the creation of reduced PDA NPs. These reduced PDA NPs exhibit potent antioxidant properties and are incorporated into a hydrogel dressing to prevent cellular stimulation caused by external oxidative stress. As a result, this approach promotes wound healing rate.
To meet the requirements of wound healing, N-acetylcysteine (NAC) acts as an antioxidant by neutralizing excessive production of ROS and promoting both angiogenesis and epidermal maturation. Hou et al. (2019) utilized a molding method to create a sandwiched framework scaffold, known as the PCL-COL/NAC scaffold. This scaffold comprised a core of PCL nanofibers surrounded by collagen loaded with NAC on both sides (Figure 7). The performance of the PCL-Col/NAC scaffold is outstanding, and it consistently delivers drugs over time. The in vitro experiments demonstrated that PCL-Col/NAC scaffolds exhibited superior attributes in terms of cell migration and proliferation compared to the control group. After 21 days, the assessment of the complete skin defect wound model demonstrated a positive treatment result and enhanced development of the outer layer of the skin when compared to the control group. CD31 staining in immunohistological analysis revealed a comparatively greater abundance of recently developed blood vessels in the PCL-Col/NAC group than in the control group.
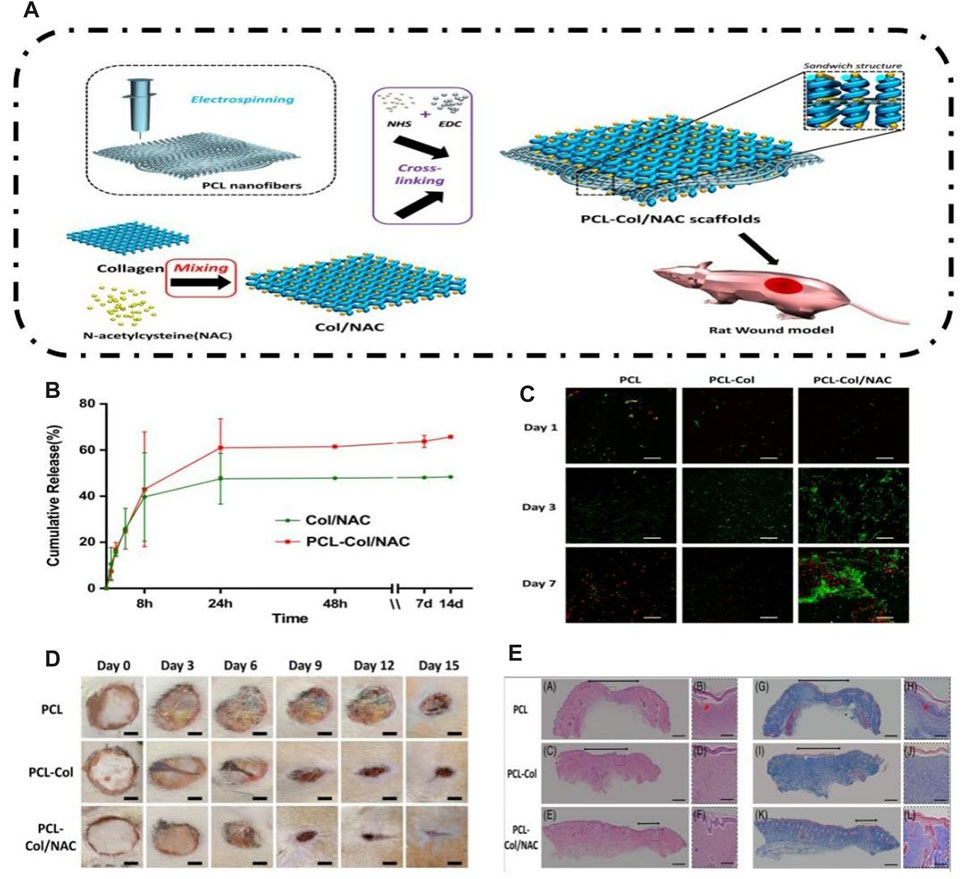
FIGURE 7. Preparation of the PCL-COL/NAC scaffold and its effect on skin tissue regeneration. (A) Schematic of experimental procedures for fabricating the PCL-COL/NAC scaffold. (B) Time-dependent cumulative release profiles of NAC by Col/NAC and PCL-COL/NAC. (C) Confocal fluorescence images for FDA/PI staining of NIH 3T3 fibroblasts cultured on the scaffolds for 1, 3, and 7 days. (D) Photographic snapshots of the temporal development of healing wounds for the different scaffolds at 0 and -15 days. (E) H&E staining and Masson staining images of the wound section on the 15th day for each group (Hou et al., 2019).
5.4 Using cells and cell products for the purpose of wound healing
Stem cells, exosomes, and cytokines have emerged as promising therapeutic strategies for promoting skin wound healing. Stem cells are a category of cells with self-renewal and multilineage differentiation capabilities (Fu X. et al., 2021). They can differentiate into various cell types, including epithelial cells, endothelial cells, and fibroblasts, thereby facilitating wound healing at different stages. Exosomes, on the other hand, are small vesicles secreted by cells and are rich in bioactive molecules such as proteins, nucleic acids, and growth factors (Yang et al., 2023). They possess regulatory functions similar to their parent cells and can promote wound healing by delivering these bioactive molecules. Additionally, cytokines play a crucial role in wound healing (Behm et al., 2012). They are signaling proteins produced by cells that can regulate various aspects of wound healing, including cell proliferation, migration, and differentiation, as well as inflammation modulation and angiogenesis. Although some studies have reported the application of stem cells, exosomes, and cytokines in promoting skin wound healing, their specific mechanisms and effects require further investigation. This chapter aims to provide an overview of the applications of stem cells, exosomes, and cytokines in promoting skin wound healing and explore their role in ROS clearance. We will review relevant research findings, including the impact of these therapeutic strategies on wound healing speed, epithelial regeneration, angiogenesis, and inflammation modulation. Furthermore, we discuss the mechanisms by which stem cells, exosomes, and cytokines clear ROS, as well as their potential clinical applications in promoting skin wound healing.
MSCs promote the movement and growth of important cells by combining fibronectin and collagen proteins while also controlling proteins associated with the extracellular matrix (Chen et al., 2012). There has been an increasing fascination in the potential of MSCs derived from bone marrow in the realm of wound healing therapy. Mohanty and Pradhan created a biomaterial bandage called EGF-curcumin and incorporated it into MSCs, resulting in the formation of MSCs-EGF-Cur B (Mohanty and Pradhan, 2020). The application of EGF-Cur B to MSCs led to a significant increase in the expression of MSC transcription factors, exceeding the levels observed in MSCs cultured using conventional methods. EGF-Cur B demonstrated superior effectiveness in enhancing cell proliferation compared to curcumin B alone. In rats, the administration of MSCs-EGF-Cur B greatly improved the healing of wounds, the production of collagen proteins, the formation of granulation tissue, and the growth of new blood vessels. Wu et al. (2007) and de Mayo et al. (2017) investigated the effects of mesenchymal stem cells (MSCs) on skin wound healing using bone marrow-derived MSCs (BM-MSCs). Luo et al. (2010) examined the effects of umbilical cord blood-derived MSCs (UCB-MSCs). These studies yielded positive results both in vitro and in vivo, demonstrating that stem cell therapy can accelerate wound healing through direct promotion of skin regeneration by expressing keratin and forming glandular structures, promoting the formation of blood vessel-like structures by endothelial cells, reducing inflammation, and decreasing collagen deposition. Researchers have also investigated whether adipose tissue-derived MSCs from patients with chronic kidney disease (CKD) have the same functionality as cells from normal sources in wound healing (Khanh et al., 2017). It was found that uremic toxins induced increased expression of ROS in na-MSCs, leading to decreased expression of hypoxia-inducible factor-1α (HIF-1α) under hypoxic conditions. CKD-AT-MSCs isolated from early-stage CKD patients exhibited a significant imbalance in redox state and high expression of ROS. In a mouse skin flap model, nAT-MSCs promoted inflammatory cell recruitment and ischemic recovery, while CKD-AT-MSCs showed functional defects and delayed wound healing processes. Importantly, the expression of prolyl hydroxylase domain 2 (PHD2) was selectively increased in CKD-AT-MSCs, and its inhibition restored HIF-1α expression and the wound healing function of CKD-AT-MSCs. These findings suggest that more research is needed on the functionality of bone marrow-derived mesenchymal stem cells in CKD patients before their clinical application.
The biological effects of MSC-derived extracellular vesicles (EVs) are recognized to be similar to those of MSCs in terms of tissue regeneration, maintenance of homeostasis, and wound healing (Ding et al., 2023). Shiekh et al. (2020a) developed a specialized wound dressing called OxOBand, which incorporates extracellular vesicles (EVs) loaded with oxygen-releasing antioxidants. This innovative dressing was specifically designed to assist in the closure of chronic wounds and promote skin regeneration. According to the research, the EVs utilized in OxOB promoted the migration of human fibroblasts and keratinocytes while also enabling fibroblasts and keratinocytes to have an extended lifespan in situations of elevated glucose levels. Following a 2-week duration, a significant reduction in oxidative stress was noted in diabetic wounds that were subjected to the OxOB and dressing, in contrast to diabetic control wounds. Moreover, the utilization of the OxOBand dressing promoted the proliferation of epithelial cells and hair follicles.
Ma et al. (2022) proposed a novel core-shell hyaluronic acid (HA) MN patch that encapsulates iron-mesenchymal stem cell-derived artificial nanovesicles (Fe-MSC-NVs) and polydopamine nanoparticles (PDA NPs) for wound healing (Figure 8). The Fe-MSC-NVs, containing multifunctional therapeutic cytokines, were encapsulated within the HA core at the tip of the MN to accelerate angiogenesis. PDA NPs were encapsulated within the shell of methacrylic acid-modified hyaluronic acid (HAMA) to overcome the detrimental effects of ROS-derived oxidative stress. The results demonstrated that Fe-MSC-NVs significantly enhanced the migration, proliferation, and tube formation of human umbilical vein endothelial cells (HUVECs). Furthermore, the combination of PDA NPs and Fe-MSC-NVs further promoted M2 macrophage polarization, thereby suppressing wound inflammation. In in vivo experiments, the Fe-MSC-NVs/PDA MN patch showed excellent efficacy in diabetic wound healing.
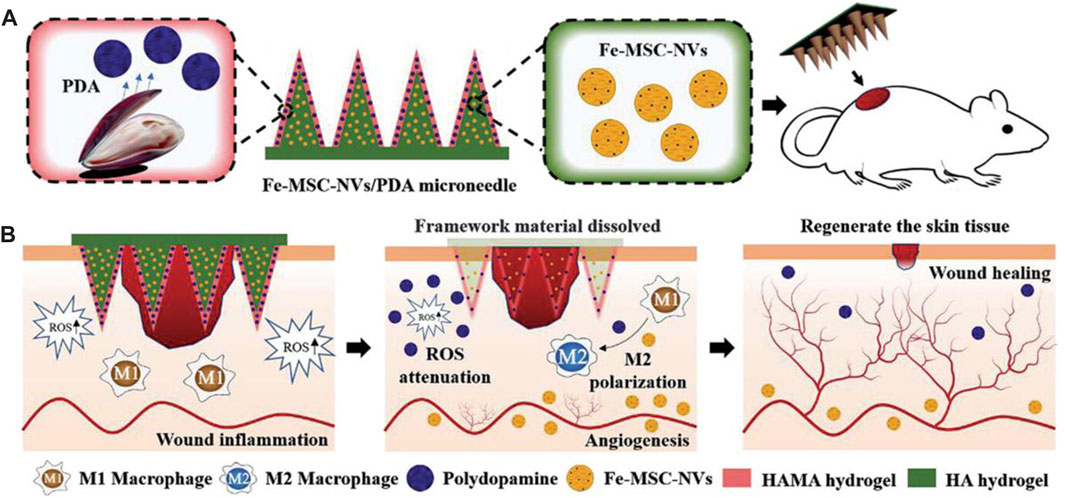
FIGURE 8. Schematic illustrations of the Fe-MSC-NVs/PDA MN patch for diabetic wound healing: (A) schematic of the Fe-MSC-NVs/PDA MN patch; (B) schematic of the wound closure process (Ma et al., 2022).
These findings emphasize the potential of engineered extracellular nanovesicles as a promising approach for skin wound repair in regenerative medicine. Therefore, a mixed system containing growth factors, MSCs, EVs, and other antioxidant chemical substances can effectively treat non-healing wounds through various pathways. We have summarized the above studies in Table 3.
Reactive oxygen species play an important role in many biological functions. Excessive ROS and oxidative stress can induce a strong inflammatory response, interfere with tissue repair, disrupt tissue repair, and disrupt the immune system. Implantation of wound dressings after skin wound can also increase oxidative stress as well as increase inflammatory cell types. To date, numerous ROS scavengers have been proposed, including systemically delivered nanoparticles, therapeutic drugs, and biomaterials for inflammation regulation and tissue repair. Currently, biomaterials with the inherent ability to regulate ROS by removing ROS have been designed, including free radical scavenging polymers, therapeutic drugs, antioxidants, enzymes, and nanomaterials, and have been used for skin tissue regeneration and cell therapy. This chapter details the practical application of these ROS-scavenging biomaterials in skin wound. These materials can release biosignaling molecules, growth factors and therapeutic drugs through damage microenvironment and cell-mediated ways, which help regulate ROS, reduce oxidative stress, and regulate tissue repair. These biological materials that regulate ROS require further attention from the scientific community.
6 Future and prospects
In the field of skin repair, the application of ROS removal materials shows great potential and provides many interesting directions for future research and clinical applications. First, the development of new ROS removal materials will be the focus of attention. In recent years, advances in nanotechnology, materials science, and bioengineering have opened up new opportunities for the design and preparation of highly efficient ROS-removing materials. For example, by manipulating the surface structure and chemical functional groups of materials, higher ROS removal efficiency and selectivity can be achieved. In addition, the development of functionalized biomaterials that can not only clear ROS but also provide functions such as growth factors and cell adhesion matrix to facilitate the skin repair process will also become a promising research direction. Second, combination treatment strategies will play an important role in skin repair. Combining ROS removal materials with other therapeutic strategies allows for a more comprehensive and synergistic therapeutic effect. For example, combined with technologies such as phototherapy, gene therapy or drug delivery, more precise treatments can be achieved, providing customized treatment options for different types of skin injuries and diseases. This combined treatment strategy is expected to improve treatment outcomes and accelerate the skin repair process. Third, translating ROS scavenging materials from in vitro studies to in vivo experiments and clinical applications is an important goal for the future. Although some progress has been made, there are still some challenges to successfully applying ROS removal materials to clinical treatment. For example, issues such as material stability, biocompatibility and drug delivery need to be addressed. Future research should focus on addressing these questions and validating the safety and efficacy of ROS-clearing materials through clinical trials and clinical trials.
In addition, an in-depth understanding of the mechanism of ROS in different skin pathological processes is also an important research direction. By studying the mechanisms of ROS production and signaling and their influence on cell function in different diseases, ROS clearance materials can be better designed and optimized to achieve more precise treatments. Personalized treatment strategies are also a prospective research direction in the future by targeting the characteristics of specific diseases and individuals to design and apply ROS clearance materials to achieve better therapeutic outcomes.
In summary, ROS removal materials have broad prospects and potential in the field of skin repair. Future research will focus on the development of novel materials, the application of combination therapy strategies, the challenges of clinical translation, mechanistic research, and personalized therapy. With continued innovation and progress, ROS removal materials are expected to become an important tool for skin repair, providing patients with better treatment options and efficacy.
Author contributions
YD: Writing–original draft. ZW: Writing–review and editing.
Funding
The authors declare that no financial support was received for the research, authorship, and/or publication of this article.
Conflict of interest
The authors declare that the research was conducted in the absence of any commercial or financial relationships that could be construed as a potential conflict of interest.
Publisher’s note
All claims expressed in this article are solely those of the authors and do not necessarily represent those of their affiliated organizations, or those of the publisher, the editors and the reviewers. Any product that may be evaluated in this article, or claim that may be made by its manufacturer, is not guaranteed or endorsed by the publisher.
References
Alam, W., Hasson, J., and Reed, M. (2021). Clinical approach to chronic wound management in older adults. J. Am. Geriatrics Soc. 69 (8), 2327–2334. doi:10.1111/jgs.17177
An, Y., Liu, W. J., Xue, P., Ma, Y., Zhang, L. Q., Zhu, B., et al. (2018). Autophagy promotes MSC-mediated vascularization in cutaneous wound healing via regulation of VEGF secretion. Cell Death Dis. 9, 58. doi:10.1038/s41419-017-0082-8
Asai, E., Yamamoto, M., Ueda, K., and Waguri, S. (2018). Spatiotemporal alterations of autophagy marker LC3 in rat skin fibroblasts during wound healing process. Fukushima J. Med. Sci. 64 (1), 15–22. doi:10.5387/fms.2016-13
Bainbridge, P. (2013). Wound healing and the role of fibroblasts. J. Wound Care 22 (8), 407–408. doi:10.12968/jowc.2013.22.8.407
Bay, C., Chizmar, Z., Reece, E. M., Yu, J. Z., Winocour, J., Vorstenbosch, J., et al. (2021). Comparison of skin substitutes for acute and chronic wound management. Seminars Plastic Surg. 35 (03), 171–180. doi:10.1055/s-0041-1731463
Beckman, K. B., and Ames, B. N. (1998). The free radical theory of aging matures. Physiol. Rev. 78 (2), 547–581. doi:10.1152/physrev.1998.78.2.547
Bedard, K., and Krause, K.-H. (2007). The NOX family of ROS-generating NADPH oxidases: physiology and pathophysiology. Physiol. Rev. 87 (1), 245–313. doi:10.1152/physrev.00044.2005
Behm, B., Babilas, P., Landthaler, M., and Schreml, S. (2012). Cytokines, chemokines and growth factors in wound healing. J. Eur. Acad. Dermatology Venereol. 26 (7), 812–820. doi:10.1111/j.1468-3083.2011.04415.x
Brigelius-Flohé, R., and Maiorino, M. (2013). Glutathione peroxidases. Biochimica Biophysica Acta-General Subj. 1830 (5), 3289–3303. doi:10.1016/j.bbagen.2012.11.020
Cai, S., Lu, C., Liu, Z., and Wang, W. (2021). Derivatives of gecko cathelicidin-related antioxidant peptide facilitate skin wound healing. Eur. J. Pharmacol., 890. doi:10.1016/j.ejphar.2020.173649
Cao, X. Q., Wang, Y., Wu, C., Li, X., Fu, Z., Yang, M., et al. (2018). Cathelicidin-OA1, a novel antioxidant peptide identified from an amphibian, accelerates skin wound healing. Sci. Rep. 8, 943. doi:10.1038/s41598-018-19486-9
Celardo, I., Pedersen, J. Z., Traversa, E., and Ghibelli, L. (2011). Pharmacological potential of cerium oxide nanoparticles. Nanoscale 3 (4), 1411–1420. doi:10.1039/c0nr00875c
Chen, C., Liu, T., Tang, Y., Luo, G., Liang, G., and He, W. (2023a). Epigenetic regulation of macrophage polarization in wound healing. Burns Trauma 11, 11. doi:10.1093/burnst/tkac057
Chen, J., Ma, H., and Meng, Y. (2023c). Analysis of the mechanism underlying diabetic wound healing acceleration by Calycosin-7-glycoside using network pharmacology and molecular docking. Phytomedicine, 114. doi:10.1016/j.phymed.2023.154773
Chen, J. S., Wong, V. W., and Gurtner, G. C. (2012). Therapeutic potential of bone marrow-derived mesenchymal stem cells for cutaneous wound healing. Front. Immunol. 3, 192. doi:10.3389/fimmu.2012.00192
Chen, X., Zhang, Y., Yu, W., Zhang, W., Tang, H., and Yuan, W. E. (2023b). In situ forming ROS-scavenging hybrid hydrogel loaded with polydopamine-modified fullerene nanocomposites for promoting skin wound healing. J. Nanobiotechnology 21 (1), 129. doi:10.1186/s12951-023-01879-2
Cheng, C. F., Fan, J. H., Fedesco, M., Guan, S. X., Li, Y., Bandyopadhyay, B., et al. (2012). Transforming growth factor α (TGFα)-Stimulated secretion of HSP90α: using the receptor LRP-1/CD91 to promote human skin cell migration against a tgfα-rich environment during wound healing (vol 28, pg 3344, 2008). Mol. Cell. Biol. 32 (1), 240. doi:10.1128/MCB.06491-11
Cheng, H. J., Liu, Y., Hu, Y., Ding, Y., Lin, S., Cao, W., et al. (2017). Monitoring of heparin activity in live rats using metal-organic framework nanosheets as peroxidase mimics. Anal. Chem. 89 (21), 11552–11559. doi:10.1021/acs.analchem.7b02895
Dai, Q., Geng, H., Yu, Q., Hao, J., and Cui, J. (2019). Polyphenol-based particles for theranostics. Theranostics 9 (11), 3170–3190. doi:10.7150/thno.31847
Dassarma, B., Nandi, D. K., Gangopadhyay, S., and Samanta, S. (2018). Hepatoprotective effect of food preservatives (butylated hydroxyanisole, butylated hydroxytoluene) on carbon tetrachloride-induced hepatotoxicity in rat. Toxicol. Rep. 5, 31–37. doi:10.1016/j.toxrep.2017.12.009
de Mayo, T., Conget, P., Becerra-Bayona, S., Sossa, C. L., Galvis, V., and Arango-Rodríguez, M. L. (2017). The role of bone marrow mesenchymal stromal cell derivatives in skin wound healing in diabetic mice. Plos One 12 (6), e0177533. doi:10.1371/journal.pone.0177533
Deng, Z., Shi, F., Zhou, Z., Sun, F., Sun, M. H., Sun, Q., et al. (2019). M1 macrophage mediated increased reactive oxygen species (ROS) influence wound healing via the MAPK signaling in vitro and in vivo. Toxicol. Appl. Pharmacol. 366, 83–95. doi:10.1016/j.taap.2019.01.022
Ding, J. Y., Chen, M. J., Wu, L. F., Shu, G. F., Fang, S. J., Li, Z. Y., et al. (2023). Mesenchymal stem cell-derived extracellular vesicles in skin wound healing: roles, opportunities and challenges. Mil. Med. Res. 10 (1), 36. doi:10.1186/s40779-023-00472-w
Dissemond, J., Kröger, K., Storck, M., Risse, A., and Engels, P. (2015). Topical oxygen wound therapies for chronic wounds: a review. J. Wound Care 24 (2), 53–63. doi:10.12968/jowc.2015.24.2.53
Ead, J. K., and Armstrong, D. G. (2023). Granulocyte-macrophage colony-stimulating factor: conductor of the wound healing orchestra? Int. Wound J. 20 (4), 1229–1234. doi:10.1111/iwj.13919
Fink, M. P., Macias, C. A., Xiao, J., Tyurina, Y. Y., Jiang, J., Belikova, N., et al. (2007). Hemigramicidin-TEMPO conjugates: novel mitochondria-targeted anti-oxidants. Biochem. Pharmacol. 74 (6), 801–809. doi:10.1016/j.bcp.2007.05.019
Firuzi, O., Miri, R., Tavakkoli, M., and Saso, L. (2011). Antioxidant therapy: current status and future prospects. Curr. Med. Chem. 18 (25), 3871–3888. doi:10.2174/092986711803414368
Fitzmaurice, S. D., Sivamani, R. K., and Isseroff, R. R. (2011). Antioxidant therapies for wound healing: a clinical guide to currently commercially available products. Skin Pharmacol. Physiology 24 (3), 113–126. doi:10.1159/000322643
Franz, M. G., Kuhn, M. A., Wright, T. E., Wachtel, T. L., and Robson, M. C. (2000). Use of the wound healing trajectory as an outcome determinant for acute wound healing. Wound Repair Regen. 8 (6), 511–516. doi:10.1046/j.1524-475x.2000.00511.x
Fu, X., He, Q., Tao, Y., Wang, M., Wang, W., Wang, Y., et al. (2021b). Recent advances in tissue stem cells. Sci. China-Life Sci. 64 (12), 1998–2029. doi:10.1007/s11427-021-2007-8
Fu, Y., Zhang, J., and Wang, Y. (2021a). Reduced polydopamine nanoparticles incorporated oxidized dextran/chitosan hybrid hydrogels with enhanced antioxidative and antibacterial properties for accelerated wound healing. Carbohydr. Polym., 257. doi:10.1016/j.carbpol.2020.117598
Han, G., and Ceilley, R. (2017). Chronic wound healing: a review of current management and treatments. Adv. Ther. 34 (3), 599–610. doi:10.1007/s12325-017-0478-y
Hassanshahi, A., Moradzad, M., Ghalamkari, S., Fadaei, M., Cowin, A. J., and Hassanshahi, M. (2022). Macrophage-mediated inflammation in skin wound healing. Cells 11 (19), 2953. doi:10.3390/cells11192953
He, S. Y., Huang, J., Zhang, Q., Zhao, W., Xu, Z., and Zhang, W. (2021). Bamboo-like nanozyme based on nitrogen-doped carbon nanotubes encapsulating cobalt nanoparticles for wound antibacterial applications. Adv. Funct. Mater. 31 (41). doi:10.1002/adfm.202105198
He, X., Xue, J., Shi, L., Kong, Y., Zhan, Q., Sun, Y., et al. (2022). Recent antioxidative nanomaterials toward wound dressing and disease treatment via ROS scavenging. Mater. Today Nano 17, 100149. doi:10.1016/j.mtnano.2021.100149
Heher, P., Mühleder, S., Mittermayr, R., Redl, H., and Slezak, P. (2018). Fibrin-based delivery strategies for acute and chronic wound healing. Adv. Drug Deliv. Rev. 129, 134–147. doi:10.1016/j.addr.2017.12.007
Hoffmann, M. H., and Griffiths, H. R. (2018). The dual role of Reactive Oxygen Species in autoimmune and inflammatory diseases: evidence from preclinical models. Free Radic. Biol. Med. 125, 62–71. doi:10.1016/j.freeradbiomed.2018.03.016
Hou, J., Chen, L., Liu, Z., Li, J., Yang, J., Zhong, A., et al. (2019). Sustained release of N-acetylcysteine by sandwich structured polycaprolactone/collagen scaffolds for wound healing. J. Biomed. Mater. Res. Part A 107 (7), 1414–1424. doi:10.1002/jbm.a.36656
Hu, J. F., Yang, L., Yang, P., Jiang, S., Liu, X., and Li, Y. (2020). Polydopamine free radical scavengers. Biomaterials Sci. 8 (18), 4940–4950. doi:10.1039/d0bm01070g
Huang, S. C., Xu, S., Hu, Y., Zhao, X., Chang, L., Chen, Z., et al. (2022). Preparation of NIR-responsive, ROS-generating and antibacterial black phosphorus quantum dots for promoting the MRSA-infected wound healing in diabetic rats. Acta Biomater. 137, 199–217. doi:10.1016/j.actbio.2021.10.008
Huang, X., He, D., Pan, Z., Luo, G., and Deng, J. (2021). Reactive-oxygen-species-scavenging nanomaterials for resolving inflammation. Mater. Today Bio 11, 100124. doi:10.1016/j.mtbio.2021.100124
Hunt, T. K., Zederfeldt, B., and Goldstick, T. K. (1969). Oxygen and healing. Am. J. Surg. 118 (4), 521–525. doi:10.1016/0002-9610(69)90174-3
Hurlow, J., and Bowler, P. G. (2022). Acute and chronic wound infections: microbiological, immunological, clinical and therapeutic distinctions. J. Wound Care 31 (5), 436–445. doi:10.12968/jowc.2022.31.5.436
Hussain, M., Suo, H., Xie, Y., Wang, K., Wang, H., Hou, Z., et al. (2021). Dopamine-substituted multidomain peptide hydrogel with inherent antimicrobial activity and antioxidant capability for infected wound healing. Acs Appl. Mater. Interfaces 13 (25), 29380–29391. doi:10.1021/acsami.1c07656
Idelman, G., Smith, D. L. H., and Zucker, S. D. (2015). Bilirubin inhibits the up-regulation of inducible nitric oxide synthase by scavenging reactive oxygen species generated by the toll-like receptor 4-dependent activation of NADPH oxidase. Redox Biol. 5, 398–408. doi:10.1016/j.redox.2015.06.008
Im, G. B., Kim, Y. G., Yoo, T. Y., Kim, Y. H., Kim, K., Hyun, J., et al. (2023). Ceria nanoparticles as copper chaperones that activate SOD1 for synergistic antioxidant therapy to treat ischemic vascular diseases. Adv. Mater. 35 (16), e2208989. doi:10.1002/adma.202208989
Iskra, M., and Majewski, W. (2000). Copper and zinc concentrations and the activities of ceruloplasmin and superoxide dismutase in atherosclerosis obliterans. Biol. Trace Elem. Res. 73 (1), 55–66. doi:10.1385/bter:73:1:55
Iuchi, Y., Roy, D., Okada, F., Kibe, N., Tsunoda, S., Suzuki, S., et al. (2010). Spontaneous skin damage and delayed wound healing in SOD1-deficient mice. Mol. Cell. Biochem. 341 (1-2), 181–194. doi:10.1007/s11010-010-0449-y
Jing, Y., Deng, Z., Yang, X., Li, L., Gao, Y., and Li, W. (2020). Ultrathin two-dimensional polydopamine nanosheets for multiple free radical scavenging and wound healing. Chem. Commun. 56 (74), 10875–10878. doi:10.1039/d0cc02888f
Jones, R. E., Foster, D. S., and Longaker, M. T. (2018). Management of chronic wounds-2018. Jama-Journal Am. Med. Assoc. 320 (14), 1481–1482. doi:10.1001/jama.2018.12426
Ju, J. H., Chen, Y., Liu, Z., Huang, C., Li, Y., Kong, D., et al. (2023). Modification and application of Fe3O4 nanozymes in analytical chemistry: a review. Chin. Chem. Lett. 34 (5), 107820. doi:10.1016/j.cclet.2022.107820
Khanh, V. C., Ohneda, K., Kato, T., Yamashita, T., Sato, F., Tachi, K., et al. (2017). Uremic toxins affect the imbalance of redox state and overexpression of prolyl hydroxylase 2 in human adipose tissue-derived mesenchymal stem cells involved in wound healing. Stem Cells Dev. 26 (13), 948–963. doi:10.1089/scd.2016.0326
Khorsandi, K., Hosseinzadeh, R., Esfahani, H., Zandsalimi, K., Shahidi, F. K., and Abrahamse, H. (2022). Accelerating skin regeneration and wound healing by controlled ROS from photodynamic treatment. Inflamm. Regen. 42 (1), 40. doi:10.1186/s41232-022-00226-6
Kim, D. W., Le, T. M. D., Lee, S. M., Kim, H. J., Ko, Y., Jeong, J. H., et al. (2020). Microporous organic nanoparticles anchoring CeO2 materials: reduced toxicity and efficient reactive oxygen species-scavenging for regenerative wound healing. Chemnanomat 6 (7), 1104–1110. doi:10.1002/cnma.202000067
Kim, J., Shirasawa, T., and Miyamoto, Y. (2010). The effect of TAT conjugated platinum nanoparticles on lifespan in a nematode Caenorhabditis elegans model. Biomaterials 31 (22), 5849–5854. doi:10.1016/j.biomaterials.2010.03.077
Kim, J. Y., Lee, D. Y., Kang, S., Miao, W., Kim, H., Lee, Y., et al. (2017). Bilirubin nanoparticle preconditioning protects against hepatic ischemia-reperfusion injury. Biomaterials 133, 1–10. doi:10.1016/j.biomaterials.2017.04.011
Koike, Y., Yozaki, M., Utani, A., and Murota, H. (2020). Fibroblast growth factor 2 accelerates the epithelial-mesenchymal transition in keratinocytes during wound healing process. Sci. Rep. 10 (1), 18545. doi:10.1038/s41598-020-75584-7
Koo, M.-A., Hee Hong, S., Hee Lee, M., Kwon, B. J., Mi Seon, G., Sung Kim, M., et al. (2019). Effective stacking and transplantation of stem cell sheets using exogenous ROS-producing film for accelerated wound healing. Acta Biomater. 95, 418–426. doi:10.1016/j.actbio.2019.01.019
Lee, G., Ko, Y. G., Bae, K. H., Kurisawa, M., Kwon, O. K., and Kwon, O. H. (2022). Green tea catechin-grafted silk fibroin hydrogels with reactive oxygen species scavenging activity for wound healing applications. Biomaterials Res. 26 (1), 62. doi:10.1186/s40824-022-00304-3
Li, Q., Shen, X., and Xing, D. M. (2023). Carbon quantum dots as ROS-generator and -scavenger: a comprehensive review. Dyes Pigments, 208. doi:10.1016/j.dyepig.2022.110784
Li, Y. N., Wang, J., Yang, Y., et al. (2021). A rose bengal/graphene oxide/PVA hybrid hydrogel with enhanced mechanical properties and light-triggered antibacterial activity for wound treatment. Mater. Sci. Eng. C-Materials Biol. Appl., 118. doi:10.1016/j.msec.2020.111447
Liang, C. Z., He, J., Cao, Y., Liu, G., Zhang, C., Qi, Z., et al. (2023). Advances in the application of Mxene nanoparticles in wound healing. J. Biol. Eng. 17 (1), 39. doi:10.1186/s13036-023-00355-7
Lord, M. S., Ellis, A. L., Farrugia, B. L., Whitelock, J. M., Grenett, H., Li, C., et al. (2017). Perlecan and vascular endothelial growth factor-encoding DNA-loaded chitosan scaffolds promote angiogenesis and wound healing. J. Control. Release 250, 48–61. doi:10.1016/j.jconrel.2017.02.009
Luo, G. X., Cheng, W., He, W., Wang, X., Tan, J., Fitzgerald, M., et al. (2010). Promotion of cutaneous wound healing by local application of mesenchymal stem cells derived from human umbilical cord blood. Wound Repair Regen. 18 (5), 506–513. doi:10.1111/j.1524-475x.2010.00616.x
Ma, T. F., Zhai, X., Huang, Y., Zhang, M., Zhao, X., Du, Y., et al. (2021). A smart nanoplatform with photothermal antibacterial capability and antioxidant activity for chronic wound healing. Adv. Healthc. Mater. 10 (13), e2100033. doi:10.1002/adhm.202100033
Ma, W. J., Zhang, X., Liu, Y., Fan, L., Gan, J., Liu, W., et al. (2022). Polydopamine decorated microneedles with Fe-MSC-Derived nanovesicles encapsulation for wound healing. Adv. Sci. 9 (13), e2103317. doi:10.1002/advs.202103317
Malone-Povolny, M. J., Maloney, S. E., and Schoenfisch, M. H. (2019). Nitric oxide therapy for diabetic wound healing. Adv. Healthc. Mater. 8 (12), e1801210. doi:10.1002/adhm.201801210
Marciniak, A., Walczyna, B., Rajtar, G., Marciniak, S., Wojtak, A., and Lasiecka, K. (2016). Tempol, a membrane-permeable radical scavenger, exhibits anti-inflammatory and cardioprotective effects in the cerulein-induced pancreatitis rat model. Oxidative Med. Cell. Longev. 2016, 1–7. doi:10.1155/2016/4139851
Mascharak, S., desJardins-Park, H. E., and Longaker, M. T. (2020). Fibroblast heterogeneity in wound healing: hurdles to clinical translation. Trends Mol. Med. 26 (12), 1101–1106. doi:10.1016/j.molmed.2020.07.008
Mohanty, C., and Pradhan, J. (2020). A human epidermal growth factor-curcumin bandage bioconjugate loaded with mesenchymal stem cell for in vivo diabetic wound healing. Mater. Sci. Eng. C-Materials Biol. Appl., 111.
Mondin, G., Wisser, F. M., Leifert, A., Mohamed-Noriega, N., Grothe, J., Dörfler, S., et al. (2013). Metal deposition by electroless plating on polydopamine functionalized micro- and nanoparticles. J. Colloid Interface Sci. 411, 187–193. doi:10.1016/j.jcis.2013.08.028
Mut-Salud, N., Álvarez, P. J., Garrido, J. M., Carrasco, E., Aránega, A., and Rodríguez-Serrano, F. (2016). Antioxidant intake and antitumor therapy: toward nutritional recommendations for optimal results. Oxidative Med. Cell. Longev. 2016, 1–19. doi:10.1155/2016/6719534
Nag, O. K., Naciri, J., Lee, K., Oh, E., Almeida, B., and Delehanty, J. B. (2022). Liquid crystal nanoparticle conjugates for scavenging reactive oxygen species in live cells. Pharmaceuticals 15 (5), 604. doi:10.3390/ph15050604
Nash, K. M., and Ahmed, S. (2015). Nanomedicine in the ROS-mediated pathophysiology: applications and clinical advances. Nanomedicine-Nanotechnology Biol. Med. 11 (8), 2033–2040. doi:10.1016/j.nano.2015.07.003
Nicks, B. A., Ayello, E. A., Woo, K., Nitzki-George, D., and Sibbald, R. G. (2010). Acute wound management: revisiting the approach to assessment, irrigation, and closure considerations. Int. J. Emerg. Med. 3 (4), 399–407. doi:10.1007/s12245-010-0217-5
Rather, H. A., Thakore, R., Singh, R., Jhala, D., Singh, S., and Vasita, R. (2018). Antioxidative study of Cerium Oxide nanoparticle functionalised PCL-Gelatin electrospun fibers for wound healing application. Bioact. Mater. 3 (2), 201–211. doi:10.1016/j.bioactmat.2017.09.006
Raziyeva, K., Kim, Y., Zharkinbekov, Z., Kassymbek, K., Jimi, S., and Saparov, A. (2021). Immunology of acute and chronic wound healing. Biomolecules 11 (5), 700. doi:10.3390/biom11050700
Rosenkrans, Z. T., Sun, T., Jiang, D., Chen, W., Barnhart, T. E., Zhang, Z., et al. (2020). Selenium-doped carbon quantum dots act as broad-spectrum antioxidants for acute kidney injury management. Adv. Sci. 7 (12), 2000420. doi:10.1002/advs.202000420
Roy, S., Khanna, S., Nallu, K., Hunt, T. K., and Sen, C. K. (2006). Dermal wound healing is subject to redox control. Mol. Ther. 13 (1), 211–220. doi:10.1016/j.ymthe.2005.07.684
Seiwerth, S., Rucman, R., Turkovic, B., Sever, M., Klicek, R., Radic, B., et al. (2018). BPC 157 and standard angiogenic growth factors. Gastrointestinal tract healing, lessons from tendon, ligament, muscle and bone healing. Curr. Pharm. Des. 24 (18), 1972–1989. doi:10.2174/1381612824666180712110447
Shi, L., Liu, X., Wang, W., Jiang, L., and Wang, S. (2019). A self-pumping dressing for draining excessive biofluid around wounds. Adv. Mater. 31 (5), e1804187. doi:10.1002/adma.201804187
Shiekh, P. A., Singh, A., and Kumar, A. (2020a). Exosome laden oxygen releasing antioxidant and antibacterial cryogel wound dressing OxOBand alleviate diabetic and infectious wound healing. Biomaterials, 249. doi:10.1016/j.biomaterials.2020.120020
Shiekh, P. A., Singh, A., and Kumar, A. (2020b). Data supporting exosome laden oxygen releasing antioxidant and antibacterial cryogel wound dressing OxOBand alleviate diabetic and infectious wound healing. Data Brief, 31. doi:10.1016/j.dib.2020.105671
Soneja, A., Drews, M., and Malinski, T. (2005). Role of nitric oxide, nitroxidative and oxidative stress in wound healing. Pharmacol. Rep. 57, 108–119.
Sorg, H., Tilkorn, D. J., Hager, S., Hauser, J., and Mirastschijski, U. (2017). Skin wound healing: an update on the current knowledge and concepts. Eur. Surg. Res. 58 (1-2), 81–94. doi:10.1159/000454919
Soule, B. P., Hyodo, F., Matsumoto, K., Simone, N., Cook, J., Krishna, M., et al. (2007). The chemistry and biology of nitroxide compounds. Free Radic. Biol. Med. 42 (11), 1632–1650. doi:10.1016/j.freeradbiomed.2007.02.030
Steiling, H., Munz, B., Werner, S., and Brauchle, M. (1999). Different types of ROS-scavenging enzymes are expressed during cutaneous wound repair. Exp. Cell Res. 247 (2), 484–494. doi:10.1006/excr.1998.4366
Steinstraesser, L., Koehler, T., Jacobsen, F., Daigeler, A., Goertz, O., Langer, S., et al. (2008). Host defense peptides in wound healing. Mol. Med. 14 (7-8), 528–537. doi:10.2119/2008-00002.steinstraesser
Sun, H. J., Gao, N., Dong, K., Ren, J., and Qu, X. (2014). Graphene quantum dots-band-aids used for wound disinfection. Acs Nano 8 (6), 6202–6210. doi:10.1021/nn501640q
Tang, P. F., Han, L., Li, P. F., Jia, Z. R., Wang, K., Zhang, H. P., et al. (2019). Mussel-inspired electroactive and antioxidative scaffolds with incorporation of polydopaminereduced graphene oxide for enhancing skin wound healing. Acs Appl. Mater. Interfaces 11 (8), 7703–7714. doi:10.1021/acsami.8b18931
Umeda, M., Inoue, M., and Shironita, S. (2011). Direct evaluation of O2-reduction-based H2O2 generation at Pt/C-containing powder microelectrode using scanning electrochemical microscopy. Fuel Cell30 (1), 53–63. doi:10.1149/1.3562459
Vecin, N. M., and Kirsner, R. S. (2023). Skin substitutes as treatment for chronic wounds: current and future directions. Front. Med. 10, 1154567. doi:10.3389/fmed.2023.1154567
Vernekar, A. A., Sinha, D., Srivastava, S., Paramasivam, P. U., D’Silva, P., and Mugesh, G. (2014). An antioxidant nanozyme that uncovers the cytoprotective potential of vanadia nanowires. Nat. Commun. 5, 5301. doi:10.1038/ncomms6301
Vijayakumar, V., Samal, S. K., Mohanty, S., and Nayak, S. K. (2019). Recent advancements in biopolymer and metal nanoparticle-based materials in diabetic wound healing management. Int. J. Biol. Macromol. 122, 137–148. doi:10.1016/j.ijbiomac.2018.10.120
Wallace, L. A., Gwynne, L., and Jenkins, T. (2019). Challenges and opportunities of pH in chronic wounds. Ther. Deliv. 10 (11), 719–735. doi:10.4155/tde-2019-0066
Wang, B., Feng, L., Jiang, W. D., Wu, P., Kuang, S. Y., Jiang, J., et al. (2015). Copper-induced tight junction mRNA expression changes, apoptosis and antioxidant responses via NF-κB, TOR and Nrf2 signaling molecules in the gills of fish: preventive role of arginine. Aquat. Toxicol. 158, 125–137. doi:10.1016/j.aquatox.2014.10.025
Wang, G., Yang, F., Zhou, W., Xiao, N., Luo, M., and Tang, Z. (2023). The initiation of oxidative stress and therapeutic strategies in wound healing. Biomed. Pharmacother. 157, 114004. doi:10.1016/j.biopha.2022.114004
Wang, S. S., Chen, R., Yu, Q., Huang, W., Lai, P., Tang, J., et al. (2020). Near-infrared plasmon-boosted heat/oxygen enrichment for reversing rheumatoid arthritis with metal/semiconductor composites. Acs Appl. Mater. Interfaces 12 (41), 45796–45806. doi:10.1021/acsami.0c13261
Wang, Z. Z., Dong, K., Liu, Z., Zhang, Y., Chen, Z., Sun, H., et al. (2017). Activation of biologically relevant levels of reactive oxygen species by Au/g-C3N4 hybrid nanozyme for bacteria killing and wound disinfection. Biomaterials 113, 145–157. doi:10.1016/j.biomaterials.2016.10.041
Werner, S., and Grose, R. (2003). Regulation of wound healing by growth factors and cytokines. Physiol. Rev. 83 (3), 835–870. doi:10.1152/physrev.2003.83.3.835
Widgerow, A. D. (2013). Chronic wounds - is cellular 'reception' at fault? Examining integrins and intracellular signalling. Int. Wound J. 10 (2), 185–192. doi:10.1111/j.1742-481x.2012.00967.x
Wu, Y. J., Chen, L., Scott, P. G., and Tredget, E. E. (2007). Mesenchymal stem cells enhance wound healing through differentiation and angiogenesis. Stem Cells 25 (10), 2648–2659. doi:10.1634/stemcells.2007-0226
Xi, Y. H., Pan, W., Liu, Y., Liu, J., Xu, G., Su, Y., et al. (2023). α-Lipoic acid loaded hollow gold nanoparticles designed for osteoporosis treatment: preparation, characterization and in vitro evaluation. Artif. Cells Nanomedicine Biotechnol. 51 (1), 131–138. doi:10.1080/21691401.2022.2149542
Xu, X. Q., Wang, R., Li, Y., Wu, R., Yan, W., Zhao, S., et al. (2023). Cerium oxide nanozymes alleviate oxidative stress in tenocytes for Achilles tendinopathy healing. Nano Res. 16 (5), 7364–7372. doi:10.1007/s12274-023-5416-5
Xu, Z., Han, S., Gu, Z., and Wu, J. (2020). Advances and impact of antioxidant hydrogel in chronic wound healing. Adv. Healthc. Mater. 9 (5), e1901502. doi:10.1002/adhm.201901502
Yamada, J., Yoshimura, S., Yamakawa, H., Sawada, M., Nakagawa, M., Hara, S., et al. (2003). Cell permeable ROS scavengers, Tiron and Tempol, rescue PC12 cell death caused by pyrogallol or hypoxia/reoxygenation. Neurosci. Res. 45 (1), 1–8. doi:10.1016/s0168-0102(02)00196-7
Yan, W., Sun, J., Hu, T., Tian, S., Feng, J., and Xiong, Y. (2023). Significant boosting effect of single atom Pt towards the ultrasonic generation of H2O2: a two-way catalytic mechanism. Appl. Catal. B-Environmental, 323. doi:10.1016/j.apcatb.2022.122143
Yang, G., Waheed, S., Wang, C., Shekh, M., Li, Z., and Wu, J. (2023). Exosomes and their bioengineering strategies in the cutaneous wound healing and related complications: current knowledge and future perspectives. Int. J. Biol. Sci. 19 (5), 1430–1454. doi:10.7150/ijbs.80430
Yang, J. Q., Huang, Z., Tan, J., Pan, J., Chen, S., and Wan, W. (2024). Copper ion/gallic acid MOFs-laden adhesive pomelo peel sponge effectively treats biofilm-infected skin wounds and improves healing quality. Bioact. Mater. 32, 260–276. doi:10.1016/j.bioactmat.2023.10.005
Yin, J. J., Lao, F., Fu, P. P., Wamer, W. G., Zhao, Y., Wang, P. C., et al. (2009). The scavenging of reactive oxygen species and the potential for cell protection by functionalized fullerene materials. Biomaterials 30 (4), 611–621. doi:10.1016/j.biomaterials.2008.09.061
Zhang, J., Song, P. K., Zhao, L. Y., Sun, Y., Yu, K., Yin, J., et al. (2021b). Malnutrition in relation with dietary, geographical, and socioeconomic factors among older Chinese. Food Chem. 34, 337–347. doi:10.3967/bes2021.045
Zhang, L., Ma, Y., Pan, X., Chen, S., Zhuang, H., and Wang, S. (2018). A composite hydrogel of chitosan/heparin/poly (γ-glutamic acid) loaded with superoxide dismutase for wound healing. Carbohydr. Polym. 180, 168–174. doi:10.1016/j.carbpol.2017.10.036
Zhang, W. Q., Chen, L., Xiong, Y., Panayi, A. C., Abududilibaier, A., Hu, Y., et al. (2021a). Antioxidant therapy and antioxidant-related bionanomaterials in diabetic wound healing. Front. Bioeng. Biotechnol. 9, 707479. doi:10.3389/fbioe.2021.707479
Zhao, H., Huang, J., and Li, Y. (2020). ROS-scavenging hydrogel to promote healing of bacteria infected diabetic wounds. Biomaterials, 258. doi:10.1016/j.biomaterials.2020.120286
Zhao, R., Liang, H., Clarke, E., Jackson, C., and Xue, M. (2016). Inflammation in chronic wounds. Int. J. Mol. Sci. 17 (12), 2085. doi:10.3390/ijms17122085
Keywords: reactive oxygen species, oxidative stress, wound healing, biomaterials, skin wound
Citation: Dong Y and Wang Z (2023) ROS-scavenging materials for skin wound healing: advancements and applications. Front. Bioeng. Biotechnol. 11:1304835. doi: 10.3389/fbioe.2023.1304835
Received: 30 September 2023; Accepted: 01 December 2023;
Published: 12 December 2023.
Edited by:
Antonella Motta, University of Trento, ItalyReviewed by:
Tam P. Nguyen, University of Texas at Arlington, United StatesGwang-Bum Im, Harvard Medical School, United States
Copyright © 2023 Dong and Wang. This is an open-access article distributed under the terms of the Creative Commons Attribution License (CC BY). The use, distribution or reproduction in other forums is permitted, provided the original author(s) and the copyright owner(s) are credited and that the original publication in this journal is cited, in accordance with accepted academic practice. No use, distribution or reproduction is permitted which does not comply with these terms.
*Correspondence: Zheng Wang, d2FuZ3o5OTlAMTYzLmNvbQ==