- Department of Stomatology, The First Hospital of Jilin University, Changchun, China
Bacterial infection refers to the process in which bacteria invade, grow, reproduce, and interact with the body, ultimately causing a series of pathological changes. Nowadays, bacterial infection remains a significant public health issue, posing a huge threat to human health and a serious financial burden. In the post-antibiotic era, traditional antibiotics are prone to inducing bacterial resistance and difficulty in removing bacterial biofilm. In recent years, antibacterial therapy based on nanomaterials has developed rapidly. Compared with traditional antibiotics, nanomaterials effectively remove bacterial biofilms and rarely result in bacterial resistance. However, due to nanomaterials’ strong permeability and effectiveness, they will easily cause cytotoxicity when they are not controlled. In addition, the antibacterial effect of non-responsive nanomaterials cannot be perfectly exerted since the drug release property or other antibacterial effects of these nano-materials are not be positively correlated with the intensity of bacterial infection. Stimuli-responsive antibacterial nanomaterials are a more advanced and intelligent class of nano drugs, which are controlled by exogenous stimuli and microenvironmental stimuli to change the dosage and intensity of treatment. The excellent spatiotemporal controllability enables stimuli-responsive nanomaterials to treat bacterial infections precisely. In this review, we first elaborate on the design principles of various stimuli-responsive antibacterial nanomaterials. Then, we analyze and summarizes the antibacterial properties, advantages and shortcomings of different applied anti-bacterial strategies based on stimuli-responsive nanomaterials. Finally, we propose the challenges of employing stimuli-responsive nanomaterials and corresponding potential solutions.
1 Introduction
Bacterial infection refers to the process of bacteria invading the host body and causing pathological changes (Taylor et al., 2002; Cossart and Sansonetti, 2004). Nowadays, bacterial infection remains a significant security threat to human society and brings a heavy financial burden (Flores-Mireles et al., 2015; Kamaruzzaman et al., 2017). Bacterial infection leads to the occurrence and development of a variety of diseases and bring pain and inconvenience to patients (White et al., 2003; Wang et al., 2004; Marima et al., 2018; Brouwer et al., 2020). For example, there are a large number of bacteria in the oral cavity, and some bacteria will transform into pathogenic bacteria when the host immune response is weak, which causing damage to the teeth, mucous membrane or bone tissue in the oral cavity, and finally seriously affecting the normal life of patients (Jiang et al., 2021). Antibiotic treatment is essential antibacterial therapy. However, antibiotics only target a small number of bacterial targets, making it easy for bacteria to resist antibiotics (Lambert, 2005; Andersson and Hughes, 2010). The emergence of drug-resistant bacteria has increased the difficulty of curing bacterial infection diseases, forcing humans to find new ways to combat bacterial infection. Besides, bacteria usually form bacterial biofilms to counteract immune clearance and antibiotics within the host (Davies, 2003). Therefore, in the post-antibiotic era, scientists need to develop new antibacterial agents that are efficient in killing bacteria, less susceptible to bacterial resistance, and can clear biofilms.
With the rapid development of modern science and technology, nanomaterials have gradually become one of the research hotspots. Nanomaterial means that the size of the material is between 1 and 100 nm, and its surface and volume properties are significantly different from that of ordinary materials (Kreyling et al., 2010). Nanomaterials have the characteristics of small size, large specific surface area, high reactivity and unique optical, electrical and magnetic properties (Khan et al., 2019). Nanomaterials have been widely developed for disease diagnosis, treatment, and prognosis (Yu et al., 2023). Compared with antibiotics, nanomaterials have multiple passive and extensive antibacterial effects, which makes it difficult for bacteria to develop tolerance to nanomaterials (Hu et al., 2021). Nanomaterials also utilize their high permeability to penetrate bacterial biofilms and clear biofilm substrates through various exogenous and chemical properties (Cui et al., 2022). Many antibacterial strategies based on nanomaterials have been applied in the treatment of bacterial infections, such as photothermal therapy (PTT), photodynamic therapy (PDT), sonodynamic therapy (SDT), chemodynamic therapy (CDT), and gas therapy (Quinn et al., 2015; Chen et al., 2020; Roy et al., 2021; Jia et al., 2022; Thomas-Moore et al., 2022).
Despite the powerful antibacterial effects of nanomaterials, non-responsive nanomaterials cause toxicity to tissue cells and insufficient antibacterial properties in complex infectious microenvironments. Specifically, for direct antibacterial nanomaterials, the antibacterial effects of them are generally non-selective, and if these nanomaterials are not controlled for combating bacteria, they will inevitably damage normal tissues and cells. For nano-delivery systems, uncontrolled drug delivery leads to drug release at inappropriate times and locations, which results in toxicity to normal tissue cells and insufficient drug in lesion sites (Huang et al., 2022a). Therefore, it is necessary to install controllable switches for nanomaterials to achieve precise antibacterial therapeutics. Stimuli-responsive antibacterial nanomaterials can be selectively activated by exogenous or microenvironment stimuli (Canaparo et al., 2019). Stimuli-responsive nanomaterials for antibacterial directly would be activated by exogenous or microenvironment stimuli, generating reactive oxygen species (ROS) and heat or releasing antibacterial agents to eliminate bacteria (Zhang et al., 2023). Once nanomaterials achieve desired antibacterial effects, the operators immediately switch off the stimuli sources to prevent the nanomaterial from continuing to exert functions to damage normal tissues and cells. Stimuli-responsive nano-delivery systems intelligent release antibacterial agents according to disease progression and changes in infectious microenvironment.
Hence, this review aims to enhance the understanding of stimuli-responsive antibacterial nanomaterials. First, we briefly introduce antibacterial nanomaterials based on stimuli-responsive strategies. Then, we summarize the design principles of nanomaterials on the basis of different stimuli-responsive mechanisms. Afterwards, we provide a detailed introduction to the applications of stimuli-responsive antibacterial nanomaterials. Finally, we propose perspectives and suggestions on the future development of antibacterial nanomaterials based on stimuli-responsive strategies. We hope scientists better understand and develop stimuli-responsive nanomaterials for sterilization via this review.
2 Design of stimuli-responsive nanomaterials
Exogenous stimuli-responsive nanomaterials mainly include light-responsive, US-responsive, and magnetic-responsive nanomaterials (Karimi et al., 2016). The working mechanism of these nanomaterials is to convert the energy of external stimulation into ROS, heat and other changes of form or state to combat bacteria (Lee et al., 2020; Li et al., 2021a; Um et al., 2021). Although stimuli-responsive antibacterial therapy is based on the unique properties of materials, the design of exogenous stimuli-responsive nanomaterials can be further optimized and improved. For example, changes in the size, shape, structure, and aggregation of nanomaterials significantly affect their functions. Thus, nanomaterials need to be designed and synthesized into types that can maximize their effects (Hotze et al., 2010). Besides, the different functions of nanomaterials require synergy. For instance, the solid bactericidal effect of ROS and the clearing biofilm matrix effect of PTT can be combined for synergistic antibacterial treatment (Hu et al., 2022a). In addition, the generation of ROS in PDT and SDT processes relies on sufficient oxygen, but oxygen in the infected microenvironment is not enough (Tan et al., 2021; Dong et al., 2023a). Therefore, nanomaterials based on PDT and SDT need to be designed to generate oxygen in the infected microenvironment or carry oxygen themselves or generate other free radicals that are not dependent on oxygen. Moreover, the antibacterial effects of many nanomaterials are double-edged swords, and these non-targeted effects may cause damage to normal tissues and cells. Therefore, nanomaterials need to be designed with targeted or on-demand drug delivery capabilities to reduce their toxic effects on tissues. Meanwhile, researchers need to explore the safety thresholds that the body can withstand towards various effects from nanomaterials, which is conducive to optimizing the design of nanomaterials from a biomedical perspective.
Microenvironment-responsive nanomaterials are mainly based on the interaction between nanomaterials and stimuli in the infectious microenvironment, such as hypoxia, low pH, redox products, toxins, and enzymes (Hu et al., 2022b). The design of microenvironment-responsive nanomaterials requires the introduction of nanomaterials that are altered in exogenous and chemical characteristics (like structural modification, hydrophobicity, size, surface charge, and chemical bond) by these stimuli. Scientists further utilize these physicochemical changes to improve nanomaterials' targeting, antibacterial, and drug-release abilities. For example, targeting antibacterial nanomaterials can be designed by introducing charge reversible stimuli-response materials, and nano drug loading systems with controlled release ability can be designed by introducing hydrophobicity changeable or chemical bond breakable stimuli-response materials (Chen et al., 2017; Zhang et al., 2020). The design of microenvironment-responsive nanomaterials should fully consider the interaction between various stimuli and materials in the microenvironment; meanwhile, scientists should consider interferences in the microenvironment because the infection microenvironment is very complex.
In a word, these stimuli-responsive nanomaterials should be designed to remain turn off without external exogenous stimuli or microenvironmental stimuli while immediately keeping turn on with external exogenous stimuli or microenvironmental stimuli. Specifically, different states of exogenous stimuli-responsive nanomaterials should be controlled by operators via regulating the sources of light, ultrasound (US), and magnetic field. When the number of bacteria decreases by more than two logarithms or exogenous stimuli exceeds the tolerance of the body, the operator should stop stimulation. Various states of microenvironmental stimuli-responsive nanomaterials should be spontaneously regulated by the concentration of stimuli, which reflect the degree of bacterial infection. The schematic diagram of stimuli-responsive antibacterial nanomaterials is summarized in Graphical abstract.
3 Applications of precise antibacterial therapeutics based on stimuli-responsive nanomaterials
3.1 Exogenous stimuli-responsive strategy
Numerous exogenous stimuli can activate nanomaterials to eliminate bacteria, such as light, US, and magnetic (Allafchian and Hosseini, 2019; Yu et al., 2022; Bag et al., 2023). Under the stimulation of these external stimuli, stimuli-responsive nanomaterials mainly generate heat or ROS, which have broad-spectrum and efficient bactericidal ability (Cheng et al., 2023a). The merit of this strategy is that the operator can adjust the intensity and duration of treatment by controlling the switch of the machine that generates the stimulus.
3.1.1 Light-responsive strategy
3.1.1.1 PDT
Photosensitizers (PSs), light, and oxygen are the three most crucial elements of PDT (Rodrigues and Correia, 2022). PSs are divided into organic and inorganic PSs, organic PSs like indocyanine green (ICG) have the advantage of high efficiency in producing ROS, while inorganic PSs like TiO2 have the advantages of good biosafety and stability (Yuan et al., 2020; Liu et al., 2022; Cheng et al., 2023b). Lights separate into ultraviolet, visible light, and near-infrared light according to the increased wavelength of light (Ji et al., 2017). The penetration depth of light deepens with the increase of wavelength; moreover, ultraviolet light harms organisms and even induces skin cancer. Therefore, near-infrared light is more conducive to treating bacterial infection (Qi et al., 2019; Fang et al., 2022). The oxygen content is closely related to ROS production (Teng et al., 2023). However, there is often a state of hypoxia in bacterial infection microenvironments. Thus, how to increase ROS production under low oxygen conditions has attracted scientists' attention (Bjarnsholt et al., 2022). Sun et al. developed an advanced nanocomposite (named F@Ce6-M) that considered the above three crucial elements, which consisting of Fe3O4, MnO2, Chlorin e6 (Ce6), and Coumarin 6 (Figure 1A) (Sun et al., 2021). Ce6 is an efficient organic PSs, but it cannot be stimulated by near-infrared light. Because of the red-shifted absorption induced by increased conjugate structure, the nanoplatform could achieve the PDT effect under near-infrared light, and it is beneficial for killing the biofilm deep in the periodontal pocket. MnO2 could generate oxygen via a high level of H2O2 in the periodontitis environment. The number of bacteria decreased by four logarithms after the therapy of nanoparticles (NPs) (Figures 1B, C), and the biofilm was significantly damaged (Figures 1D, E). Thanks to its excellent antibacterial effect, the number of inflammatory cells and the levels of inflammatory factors in periodontal tissue were significantly reduced. After co-culture of nanocomposites and L929 cells for 24 h, when the concentration of Ce6 in F@Ce6-M was 0–5 μM, the relative survival rate of L929 cells was more than 90%, which suggested that F@Ce6-M had good cytocompatibility. Although PDT has many advantages, it also has shortcomings to be addressed, such as short action radius of ROS (Gao et al., 2021).
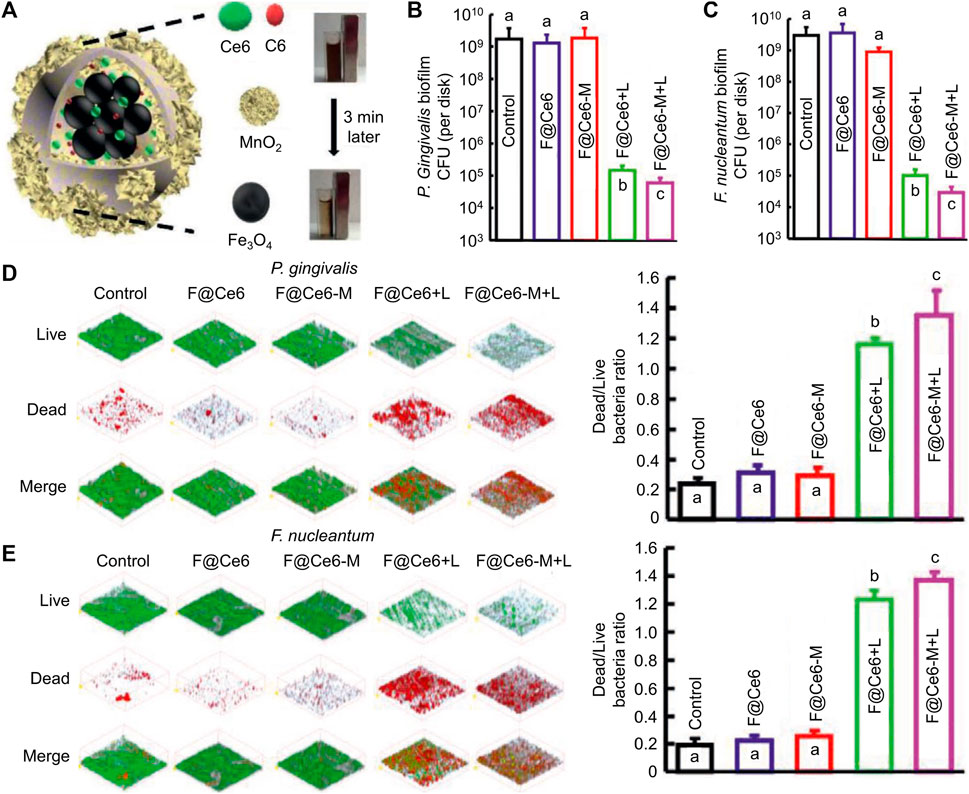
FIGURE 1. (A) schematic diagram of the microstructure and composition of F@Ce6-M. (B–C) Corresponding statistical data of colonies of different biofilms. (D–E) Live/Dead images and corresponding statistical data of colonies of different biofilms (Sun et al., 2021; Sun et al., 2021). Copyright 2021 Wiley-VCH.
3.1.1.2 PTT
Photothermal agents transfer light energy into thermal energy to exert the PTT process (Jung et al., 2018). Compared to PDT with higher sterilization efficiency towards planktonic bacteria, PTT has higher anti-biofilm efficiency. Since the biofilm is rich in extracellular DNA, polysaccharides, proteins, and other biofilm matrices, which can be cleared by a large amount of heat from PTT, when the biofilm matrix is not protected, the planktonic bacteria are more likely to be killed (Buzzo et al., 2021; Qi et al., 2022). Besides, PTT has been proven to promote vascular regeneration, which facilitates infected wound healing (Wang et al., 2021a; Huang et al., 2022b; He et al., 2023). Among inorganic materials, Au nanorod has a high photothermal conversion rate, adjustable absorption wavelength, and low cytotoxicity (He et al., 2022). Among organic materials, polydopamine not only has a high photothermal conversion rate but also has the merits of being easy to synthesize and connect with other functional groups (Hu et al., 2016). To solve the problem of low sterilization rate of pure PTT, researchers always combine PTT and other strategies like PDT and gas therapy. Qi and her co-workers designed a nanocomposite composed of Au rods, PSs (ICG), and nitric oxide donor (S-nitrosothiols) (Qi et al., 2022), PTT effect destroyed biofilm and promoted S-nitrosothiols to generate nitric oxide (Figure 2A). Nitric oxide could disperse biofilm and relieve inflammation via inhibiting NF-kB signaling pathways (Figure 2B). Toxicity tests in vivo by H&E analysis showed the nanocomposites would not result in histological abnormalities or local inflammation of the main organs even after 42 d post-treatment, which proved that the nanocomposites had good histocompatibility. Cheng et al. proved that polydopamine could not only achieve the PTT effect but also transfer light energy into TiO2 to realize the PDT effect (Cheng et al., 2023b). This simple and stable nanoplatform had rich antibacterial functions, and it reduced the number of bacteria in the biofilm by 99%. The wound caused by bacterial infection almost completely healed 10 days after treatment. Although PTT has been widely used in antibacterial therapy, excessive temperature cause damage to tissues and cells. Studies have shown that the temperature of PTT should be controlled below 45 °C (Dong S. et al., 2023).
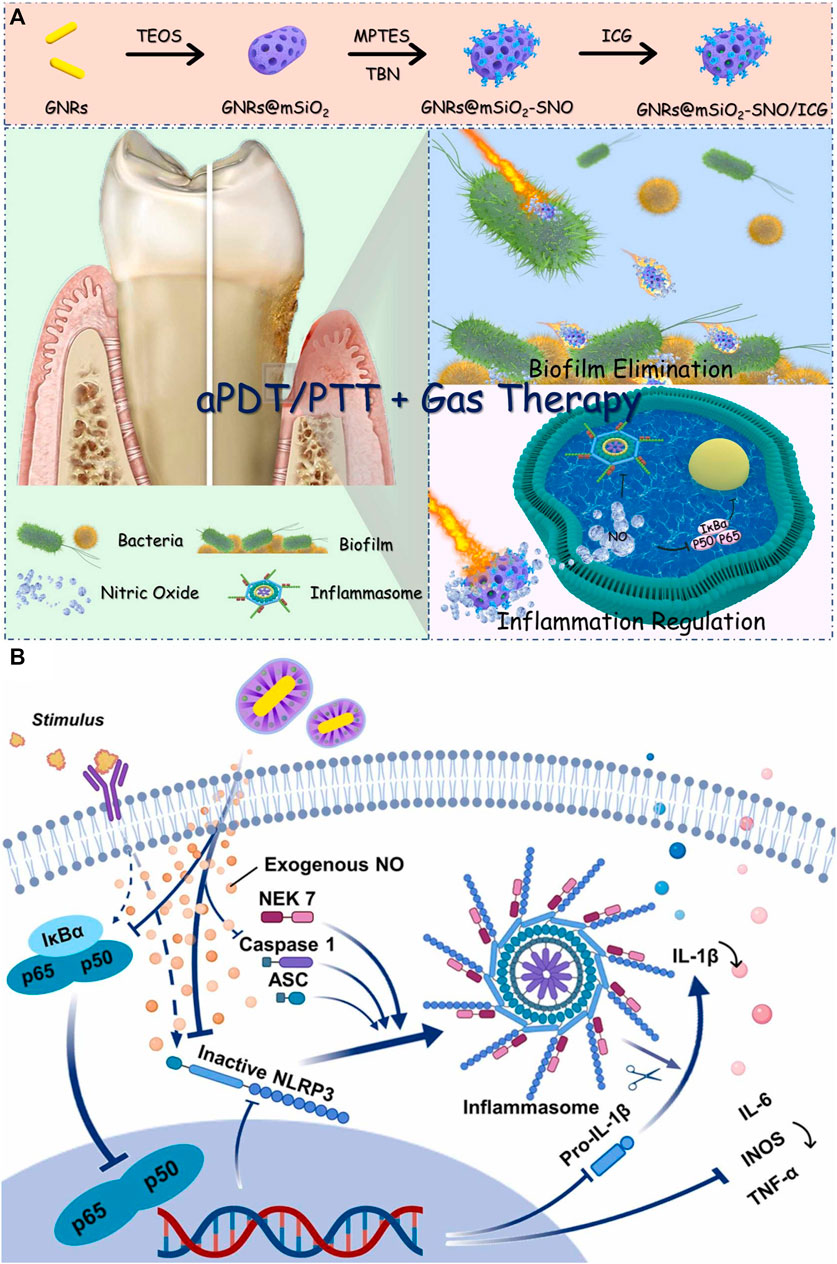
FIGURE 2. (A) Schematic illustration of GNRs@mSiO2-SNO/ICG and its anti-biofilm and anti-inflammatory functions for periodontitis treatment. (B) Schematic diagram of GNRs@mSiO2-SNO/ICG for regulating inflammation (Qi et al., 2022). Copyright 2022 American Chemical Society.
3.1.2 US-responsive strategy
US can activate sonosensitizers to produce ROS via sonochemistry, sonoporation, pyrolysis, and sonoluminescence (He et al., 2019). The penetration distance of US into tissue is more profound than that of near-infrared light, and the US is a non-invasive stimulus. Hence, SDT based on US has received widespread attention recently (Wu et al., 2022). Currently, relatively few materials have been proven to achieve the SDT effect. TiO2 is a fundamental sonosensitizer, but the ROS generation efficiency of TiO2 is insufficient due to its wide bandgap (Ning et al., 2022). To settle this critical problem, researchers have attempted to modify TiO2 with other materials. For example, Yang’s group added Ag into TiO2 to obtain a robust ROS production nanocomposite (Xin et al., 2023). The bandgap of this nanocomposite was obviously reduced after Ag modification, and the ROS yield of this nanocomposite was approximately three times that of pure TiO2 (Figure 3A). Besides, the authors also utilized positively charged chitosan to coat this nanocomposite for targeting bacterial cell walls via the electrostatic attraction effect. This nanocomposite with targeting ability could enter the inside of bacteria and synergistic ROS to eliminate bacteria (Figure 3B). In vivo experiments showed significant inhibition of alveolar bone loss caused by bacterial infection (Figure 3C). Moreover, the nanocomposite showed slightly cytotoxicity toward different cells after co-cultured for 3 and 5 d at the high concentrations of 400 μg/mL. SDT is an emerging antibacterial therapy, but currently, there are very few sonosensitizers available for SDT, and the mechanism of SDT production is also controversial. Future studies had better focus on developing more advanced sonosensitizers or exploring the mechanism of SDT in detail.
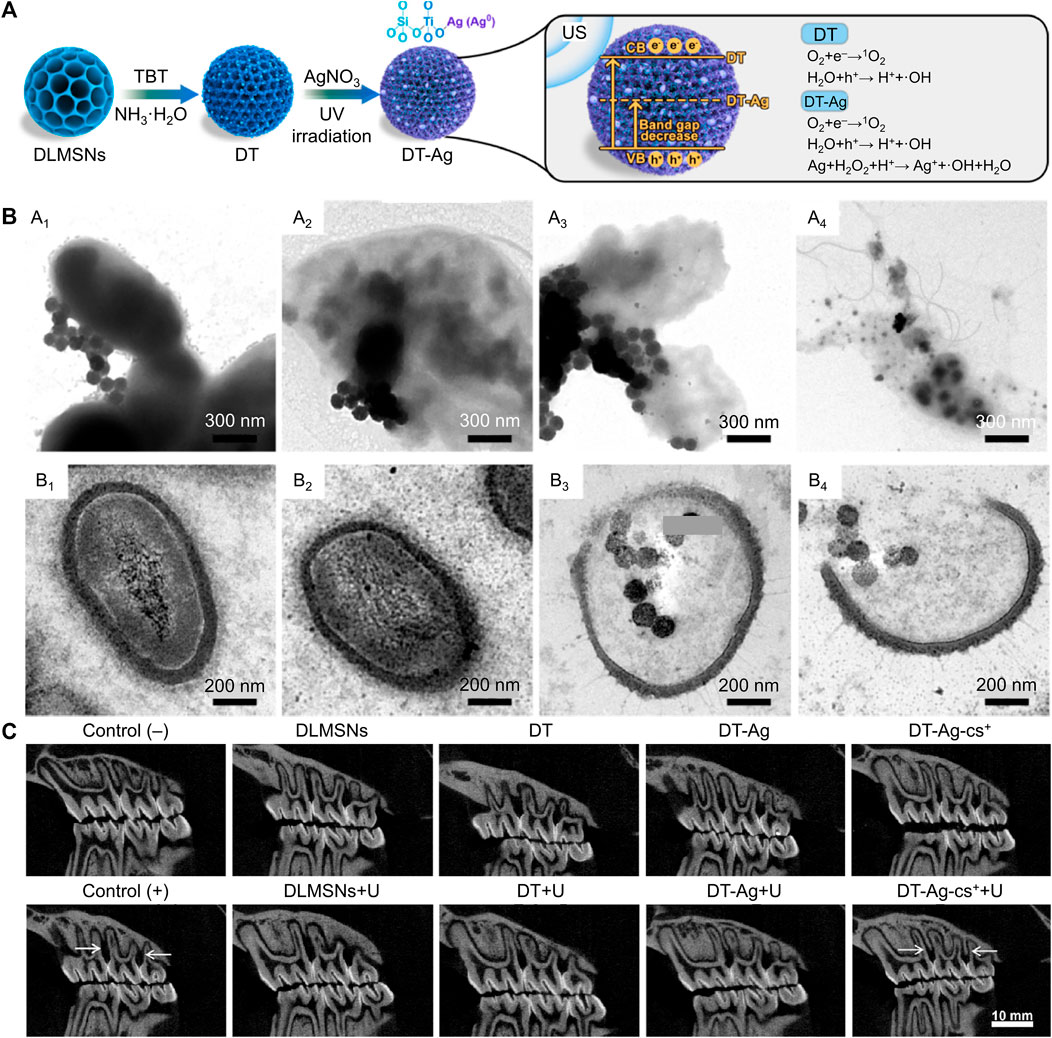
FIGURE 3. (A) Schematic illustration for the synthetic path and SDT mechanism of the nanocomposite. (B) TEM images of bacteria after treated by the nanocomposite. (C) In vivo anti-periodontitis properties of this nanocomposite (Xin et al., 2023; Xin et al., 2023). Copyright 2023 Elsevier.
3.1.3 Magnetic-responsive strategy
3.1.3.1 Magnetophysical therapy
Under a magnetic field, some metal materials will undergo changes in morphology or motion, which can be used to break the structure of bacterial cell membranes and biofilms. For example, Zhou’s group designed glucose oxidase (GOx)-modified Fe3O4 magnetic NPs (named GMNPs) to ameliorate bacterial biofilm-induced persistent endodontic infections (Ji et al., 2021). The bacterial biofilm matrix was obviously destroyed via the movement of Fe3O4 magnetic NPs induced by the magnetic field, the generation of ROS, and nutrient starvation induced by GOx. The biomass and average thickness of Escherichia faecalis and C. albicans biofilms were both decreased after culturing with GMNPs for 48 h, and surface volume ratio of biofilms was increased. In another case, Elbourne et al. found that Ga-Fe metal droplets could be actuated by low-intensity rotating magnetic fields and formed sharp edges to combat bacteria and biofilm (Figure 4A) (Elbourne et al., 2020). Schematic diagram, SEM images and TEM images indicated that the magnetic field changed Ga-Fe droplets into spheroids, nanorods, and nanostars (Figures 4B–E). After the treatment mediated by Ga-Fe droplets with a magnetic field, the structure of biofilm was obviously damaged, and the membrane of bacteria was also penetrated and destroyed (Figures 4F, G). About 99% of live bacteria area found dead after the coincubation of bacteria with NPs and exposure to a magnetic field for 90 min. Besides, red blood cell (RBC), white blood celllysis testing, hemolysis percentage and platelet aggregation tests indicated the Ga-Fe droplets did not significantly change the concentration of WBCs and white blood cell or induce hemolysis, or lead significant platelet aggregation. Magnetophysical strategy is a novel therapy method; however, its effective sterilization takes too long, which limits its potential clinical application.
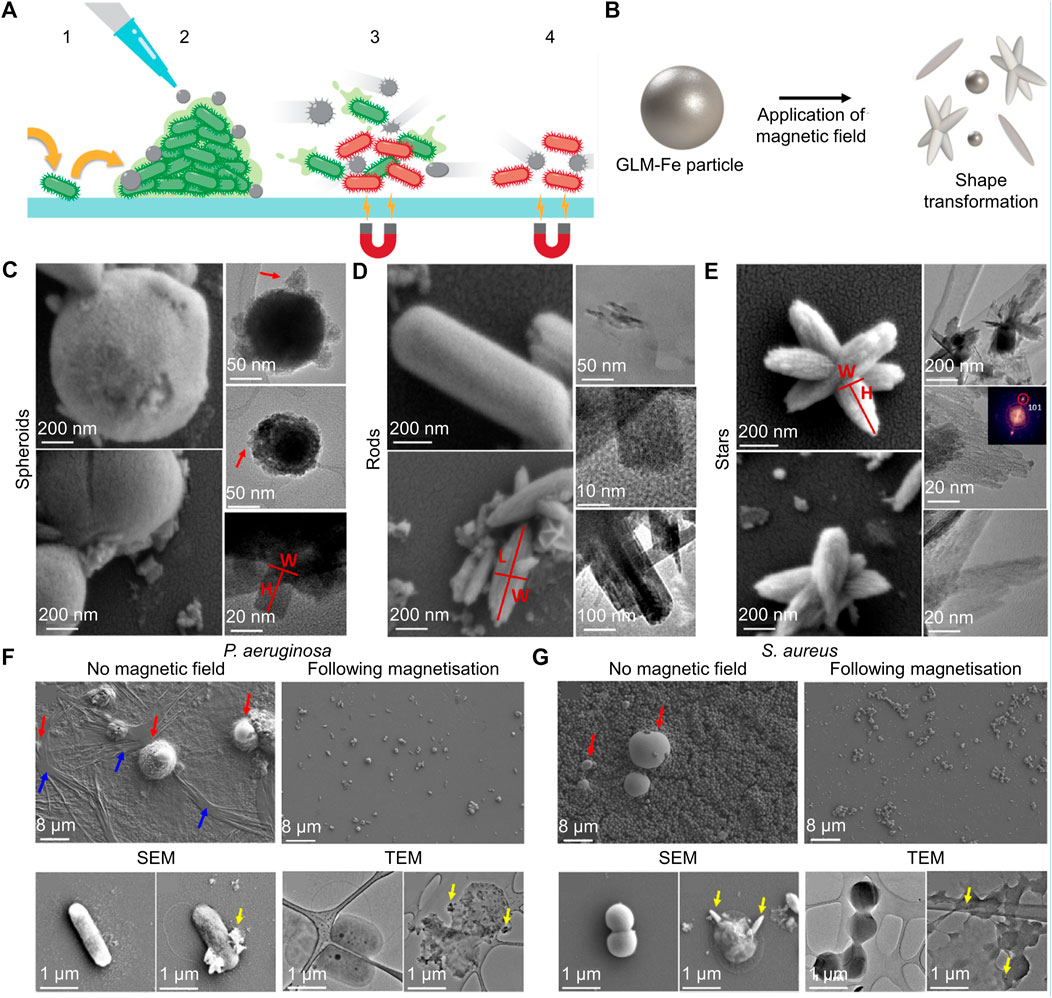
FIGURE 4. (A) Schematic illustration of the antibiofilm properties of the Ga-Fe metal droplets particles. (B) Schematic diagram of Ga-Fe metal droplets particles undergoing deformation in a magnetic field. (C–E) Ga-Fe droplets transform into spheroids, nanorods, and nanostars in a magnetic field. (F–G) SEM and TEM images of various biofilm and bacteria cells after the therapy of Ga-Fe droplets (Elbourne et al., 2020). Copyright 2020 American Chemical Society.
3.1.3.2 Magnetothermal therapy
In addition to magnetophysical therapy, some metal materials like Fe and Co will align directionally when they are exposed to a magnetic field, and hysteresis loss/relaxation loss will generate heat energy, this process is called the magnetothermal effect, Fe and Co based nanomaterials have been applied for bacterial infection via the magnetothermal effect. Hatamie et al. synthesized graphene/Co/poly(ethylene glycol) (PEG) nanocomposites to kill bacteria (Singh et al., 2015; Hatamie et al., 2020). Under alternative current magnetic field, heat generated by Co and the wind edge of graphene (rGO) had synergistic germicidal effect, the rGO/Co/PEG nanocomposites showed antibacterial activity toward E. coli bacteria of about 100% within 15 min. Bigham and his co-workers constructed a magnetic Mg2SiO4-CuFe2O4 nanocomposite (Bigham et al., 2019). Thanks to the magnetothermal and bactericidal effect of Cu ions, these disks showed inhibitory effects against both S. aureus and E. coli (inhibition zones against bacteria around disks are 8.2 ± 0.25 mm and 30.8 ± 0.2 mm, respectively). Besides, this multifunctional ceramic also had the effect of promoting bone regeneration. At present, there are limited materials with magnetothermal effects. Developing more materials with magnetothermal effects may be helpful for the development of this research direction.
3.2 Microenvironment stimuli-responsive strategy
The formation of an infectious microenvironment is complicated, its unique features include hypoxia, low pH, enriched redox products, generous toxins, and abundant enzymes (Hu et al., 2022b). Many nanomaterials change these characteristics, and scientists have utilized the interaction between these nanomaterials and infectious microenvironments to construct many intelligent nanomedicines with high spatiotemporal controllability.
3.2.1 Hypoxia-responsive strategy
Due to the continuous oxygen consumption by aerobic bacteria and facultative anaerobic and the low permeability of the infected microenvironment, the oxygen content in the infected microenvironment is insufficient (Wang et al., 2022a). Hypoxic microenvironment induces the overexpression of many reductases, such as azoreductase and nitroreductase, so researchers can design corresponding enzymes-responsive nanomaterials to target the hypoxic microenvironment (Ma et al., 2019; Lin et al., 2022). For example, Ding et al. customized lactose-modified azocalix[4]arene (named LacAC4A) loaded with ciprofloxacin. Lactose provided the nanocomposite with targeting ability towards the bacterial surface, and azocalix could control the release of ciprofloxacin under the hypoxia microenvironment (Li et al., 2022). In another work, Wang’s group cleverly linked PDT and the expression of nitroreductase, rapid sterilization and long-term antibacterial effects (Xiu et al., 2022). Specifically, PDT mediated by Ce6 could rapidly produce ROS to achieve rapid sterilization, and PDT also exacerbated oxygen consumption, which promoted the expression of nitroreductase and the activation of metronidazole to combat bacteria for a long time (Figure 5A). After the treatment by nanocomposite, the bacterial biofilm was significantly damaged, and the corresponding number of bacteria decreased by about six logarithms. As an important feature of the infectious microenvironment, hypoxia brings many physiological changes, and scientists can utilize these changes to design more sophisticated nanomedicines.
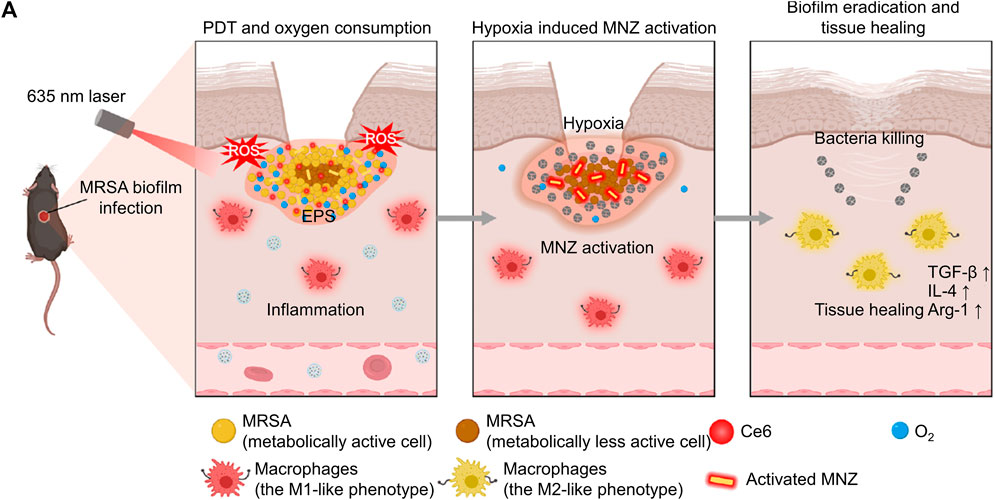
FIGURE 5. (A) The schematic diagram shows the process of the nanosystem exerting PDT effect, the nanosystem releasing antibiotics for long-term sterilization in hypoxic environments, and the nanosystem promoting tissue healing (Xiu et al., 2022; Xiu et al., 2022). Copyright 2022 Springer nature.
3.2.2 pH-responsive strategy
Bacteria produce acidic metabolites such as lactic acid, which lower the pH value of the infected microenvironment (Ma et al., 2020). There are two main types of pH-responsive nanomaterials: materials that can break chemical bonds in acidic environments and materials with ionizable functional groups. For instance, Wan and her co-workers created a novel Mn-doped zeolitic imidazolate framework-8 (named Mn-ZIF-8) (Wan et al., 2021). ZIF-8 was easily disintegrated under acidic conditions (Figure 6A), and subsequently released Zn2+, Mn2+ and Mn4+ from inside (Wan et al., 2021). As 3D Live/Dead (Figure 6B) and SEM (Figure 6C) images are shown, Zn2+ induced bacterial death and biofilm destruction via generating ROS. Mn2+ and Mn4+ could exert the effects of various nanoenzymes and promote the polarization of macrophages from pro-inflammatory M1 type to anti-inflammatory and repair-promoting M2 type (Figure 6D). Benefiting from the comprehensive antibacterial and anti-inflammatory effects of Mn-ZIF-8, bacterial infected wounds can heal within 10 d (Figures 6E, F). All biocompatibility tests indicated that Mn-ZIF-8 had good cytocompatibility, hemocompatibility and histocompatibility, which showed its promising potential for clinical translation. Unlike the above study, Xu et al. (2022) modified Fe3O4 layer by layer with polydopamine, Ag, polydopamine, and glycol chitosan separately (named FePAgPG). In a neutral environment, FePAgPG did not have targeting ability, but in an acidic environment, glycol chitosan could be protonated and bring positive the charge to the nanoplatform, which provided FePAgPG with targeting ability towards the bacterial membrane. The polydopamine located in the second layer prevented the premature release of Ag+ from causing damage to normal tissues and cells, and the great PTT effect of polydopamine could also combat biofilm effectively. Moreover, it’s practical that because this nanocomposite contained Fe3O4, it could be easily attracted and removed by a magnet after being applied to dental caries, which provided convenience for the clinical operations of dentists.
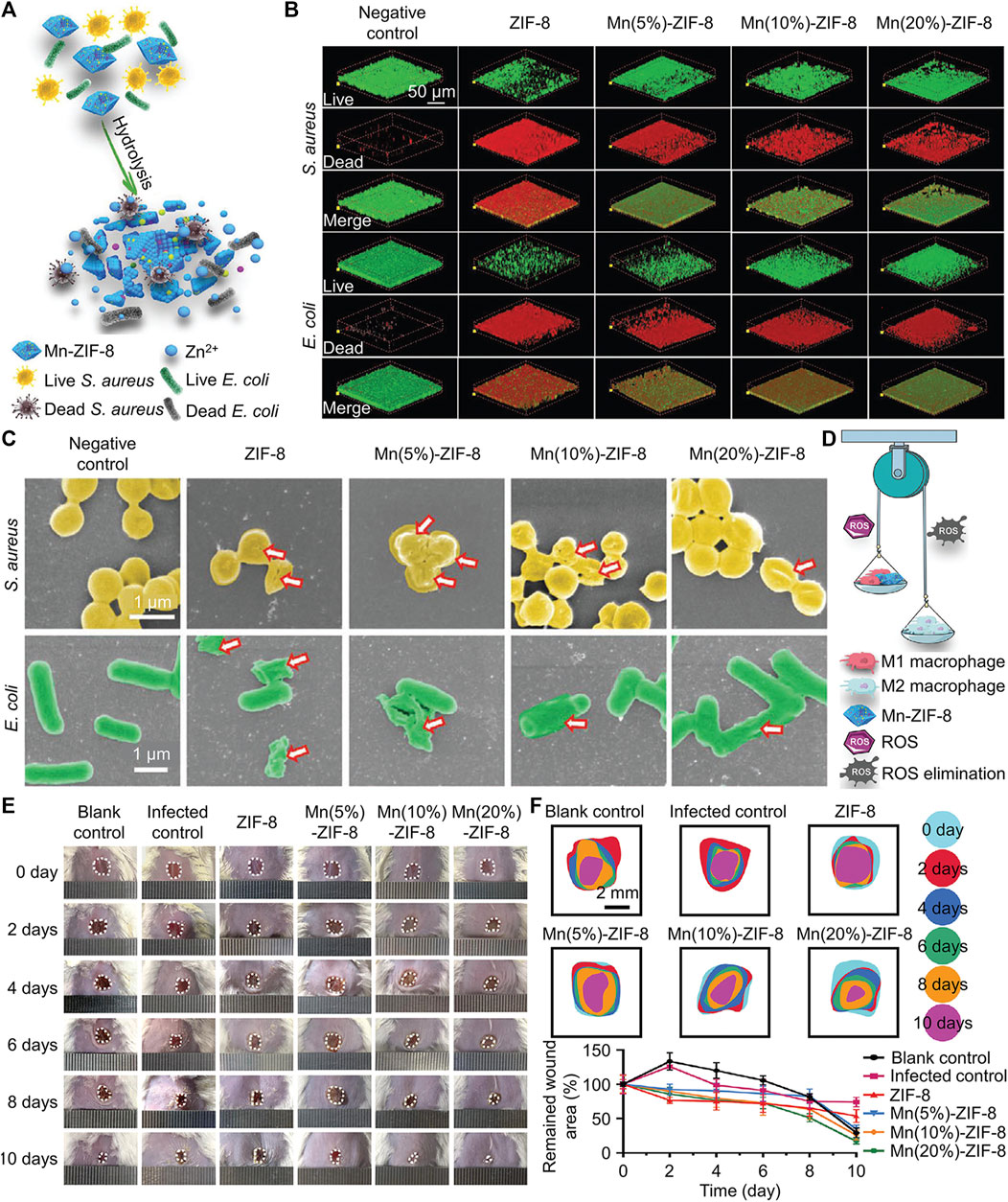
FIGURE 6. (A) Schematic diagram of the antibacterial mechanism of Mn-ZIF-8. (B) Live/Dead images of different biofilm after treated by Mn-ZIF-8. (C) SEM images of different biofilm after treated by Mn-ZIF-8. (D) Schematic diagram of the antiinflammation mechanism of Mn-ZIF-8. (E) Images of skin wounds under different treatments. (F) Changes in the sizes of wound areas in different groups (Wan et al., 2021). Copyright 2021 Wiley-VCH.
3.2.3 Redox products-responsive strategy
3.2.3.1 ROS-responsive strategy
Unlike the normal tissue microenvironment, there is a large amount of ROS in the bacterial infection microenvironment (Hicks et al., 2019). This is because bacteria will secrete lipopolysaccharide, DNA, peptidoglycan, flagellin and other proinflammatory substances to promote the recruitment of inflammatory cells, which will produce ROS (Suzuki et al., 1996; Graves, 2008; Woo et al., 2022). Scientists have developed various polymer nanomaterials for ROS-responsive antibacterial strategy, on the one hand, this strategy achieves precise and on-demand treatment of drugs, on the other hand, this strategy consuming ROS to prevent its damage to the host. Mesoporous silica nanoparticles (MSNs) have a high specific surface area, high porosity, and a radially ordered porous structure, makes them widely applied in drug delivery therapy, but MSNs-mediated drug delivery strategy lacks the ability for controllable delivery (Mizoshita and Tanaka, 2017). To solve this problem, Li et al. (2022) adopted thioketal grafted methoxy PEG (mPEG-TK) as the intelligent door control system of MSNs to carry vancomycin. TK could degrade polymers under ROS stimulation and release vancomycin by opening the door of MSNs. The experimental results showed that with the increase of ROS, the release of vancomycin significantly accelerated, and this process lasted for 36 h. The bacterial infection was completely eliminated after 14 d of treatment with this new antibiotic carrier.
3.2.3.2 GSH-responsive strategy
As the infection microenvironment presents hypoxia, bacteria need to carry out glycolysis, which will produce a large amount of GSH (Liu et al., 2021). In addition, as mentioned earlier, there is a high level of ROS in the infected microenvironment. Bacteria will produce GSH to consume ROS to resist the damage caused by ROS (Wang et al., 2021b). However, there are relatively few materials that can respond to GSH. Until recent years, scientists attempted to construct GSH-responsive nanomaterials via unstable disulfide bonds. Li et al. (2021b) developed mesoporous organosilica NPs to deliver Ag and gentamicin. In order to prevent the uncontrolled release of antibiotics and Ag from causing toxicity to normal tissues, the authors introduced disulfide bonds to modify this nanocomposite. The controlled release mediated by disulfide bonds could achieve the efficient utilization of Ag by inhibiting its aggregation, while the on-demand release of gentamicin promoted the entry of Ag into bacteria. The synergistic effect between Ag and gentamicin was significantly greater than their respective effects on bacteria. More importantly, this new nano-drug exhibited strong killing effects on multiple drug-resistant bacteria. GSH-responsive disulfide bonds have been widely used in other antibacterial gas and drug therapies, and we will not elaborate on these here (Peng et al., 2022; Su et al., 2022). However, disulfide bond is a well-known unstable chemical bond that can be broken by ROS, nitric oxide and other stimuli, which may lead to the low sensitivity of disulfide bond-mediated controlled release system because it is easily affected by other factors in the complex infected microenvironment (Wang et al., 2021c; Yang et al., 2021).
3.2.4 Toxin-responsive strategy
During bacterial colonization and proliferation, bacteria secrete various toxins, and the amount of toxins is often positively correlated with bacterial vitality and the infection process. For example, bacteria secrete toxins and cause damage to the host cell membrane, which inspired scientists to design many toxin-responsive nanomedicines (Pornpattananangkul et al., 2011). Chen’s group designed a bacterial toxin-responsive nanoplatform to achieve on-demand and efficient PDT against bacterial infection (Zhuge et al., 2022). Specifically, PDT often causes damage to host cells, and PDT relies heavily on oxygen content, but the bacterial infection microenvironment exhibits characteristics of hypoxia. To solve these two scientific problems, the authors employed RBC membranes to co-encapsulate perfluorocarbon nanoemulsion and PSs. The pore-forming toxin secreted by methicillin-resistant S. aureus, group A Streptococcus, and Listeria monocytogenes (LM) caused damage to the RBC membrane, which led to the release of oxygen and PSs in perfluorocarbon nanoemulsion (Figure 7A). The on-demand release of PSs combined with sufficient oxygen had achieved a good antibacterial effect in the vicinity of bacterial infections. Similarly, Wu and his co-workers utilized liposomes to coat CaO2 and antibiotics (Wu et al., 2019). Under the action of bacterial toxin, the outer shell of the liposome ruptured, and then water molecules entered the interior of the liposome through the perforation and reacted with CaO2 to produce oxygen. The large amount of oxygen facilitated the rapid release of antibiotics, which exerted an antibacterial effect (Figure 7B). Toxin-responsive nanomaterials are almost based on the interaction between toxins and lipids. In the future, researchers can design more intelligent nanomaterials based on the interactions between other toxins and materials.
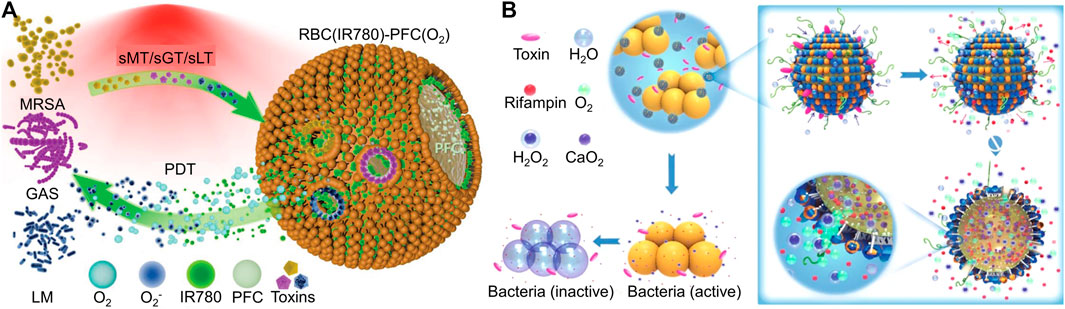
FIGURE 7. (A) Schematic diagram of biomimetic nanobubbles for the on-demand PDT towards bacterial infections (Zhuge et al., 2022). Copyright 2022 Wiely-VCH. (B) Schematic illustration of stimulus-powered antibiotic release from nanoreactors for bacterial infection therapy (Wu et al., 2019). Copyright 2019 Springer nature.
3.2.5 Enzyme-responsive strategy
Bacteria secrete multiple overexpressed enzymes in the bacterial infection microenvironment, such as matrix metalloproteinases (MMPs), lipase and gelatinase (Zhou et al., 2022). These enzymes cause the decomposition of certain materials, and researchers can use these materials degraded by these enzymes to construct enzyme-responsive NPs.
The wound healing is inhibited by bacterial infections and bacterial secretion of MMPs (Eming et al., 2008). To treat bacterial biofilm-induced corneal blindness, Han et al. MMP-responsive supramolecular nanoplatform, which was composed of Ce6, β-cyclodextrin (β-CD) and MMP-9-sensitive peptides (Han et al., 2020). In the microenvironment without MMP-9, the surface of NPs carried negative charges, which could prevent NPs from adhering to normal eye cells and thus prolong their retention time in tears. Under the stimulation of MMP-9, the negatively charged peptide shell was destroyed, and positively charged components within NPs were exposed and targeted negatively charged bacterial biofilms via electrostatic attraction. NPs with the ability to target biofilms effectively enhanced the antibacterial effect of Ce6, which prevented further damage to the cornea by bacteria and effectively alleviated corneal inflammation.
Many Gram-positive and Gram-negative bacteria secrete lipase such as Bacillus, Pseudomonas, and Burkholderia (Bender and Flieger, 2010). Vasilev’s group utilized lipase-responsive polycaprolactone to load ultra-small Ag NPs (1.6 ± 0.2 nm) for selective combating bacteria (Uroro et al., 2023). Polycaprolactone is a biocompatible material approved by the Food and Drug Administration (FDA) for application in the medical field. Under the action of lipase secreted by bacteria, Polycaprolactone could decompose and release antibacterial Ag+. In addition, this nanoplatform eliminated bacteria that secreted lipase and did not exert an inhibitory effect on bacteria that did not secrete lipase, such as Escherichia coli, the characteristic of this nanoplatform is conducive to the efficient removal of target pathogenic bacteria without disrupting the balance of bacterial communities. Wang et al. (2022b) constructed a nanodrug with a core loaded with alpha-lipoic acid and a poly amidoamine dendrimer shell loaded with minocycline hydrochloride. Under the action of an acidic microenvironment and lipase, NPs released minocycline hydrochloride and alpha-lipoic to combat bacteria and resist the oxidation of ROS for comprehensively treating periodontitis.
Xia’s group developed an anti-infection microneedle patch based on the characteristic of Staphylococcus aureus secretes gelatinase (Lei et al., 2022). The tip of this microneedle was composed of degradable materials polyethylene pyrrole and Type III collagen protein, which could achieve exogenous delivery of gelatin nanocomposite. Then, the gelatin nanocomposite would release antibacterial photothermal peptide AMP-Cypate under the stimulation of gelatinase. Experimental data showed that the microneedle patch could degrade and release gelatin nanocomposite within 20 min, and the efficiency of gelatin nanocomposite releasing antibacterial photothermal peptide increased with the rise of bacterial concentration. This microneedle patch cleverly combined non-invasive microneedle therapy, PTT and on-demand drug delivery strategy. In another study, Liu’s group used RBC membrane and gelatin to deliver Ru-Se NPs (Lin et al., 2019). RBC membrane prevented NPs from being eliminated by the body and neutralize exotoxin produced by bacteria. The release of Ru-Se NPs from gelatin could effectively damage the bacterial cell membrane, leading to leakage of its contents. More importantly, Ru-Se NPs had fluorescence imaging functions, which could help scientists monitor the treatment process in real time.
Although researchers have developed many enzyme-responsive antibacterial nanomaterials in recent years, an issue that cannot be ignored is that other components generated in nanomaterials may inactivate enzymes (such as ROS and heat). Therefore, how to construct enzyme-responsive antibacterial NPs without affecting enzyme activity is a future scientific problem.
Advances in precise antibacterial therapeutics based on stimuli-responsive nanomaterials are listed in Table 1.
4 Challenges and future perspectives
In the post-antibiotic era, nanomaterials are regarded as potential substitutes for antibiotics due to their high bactericidal efficiency, rich functionality, and ease of modification. Especially stimuli-responsive nanomaterials based on the physical and chemical properties of the nanomaterials themselves and the characteristics of the infectious microenvironment, the antibacterial effects or release of antibacterial agents of these nanomaterials can be effectively controlled to achieve precise antibacterial treatment. Intelligent stimuli-responsive nanomaterials reduce toxicity to tissues and improve antibacterial efficiency, and they have been widely developed and achieved satisfactory results in recent years. However, there are still many challenges to be addressed in applying stimuli-responsive nanomaterials in antibacterial therapy. (1) Nanomaterials utilize various physical effects, chemical effects and antibacterial agents to kill bacteria, but these ways inevitably cause damage to normal tissue cells, which exacerbates inflammatory reactions and inhibits the healing of infected tissues (Bai et al., 2023). More advanced stimuli-responsive nanomaterials should have the function of regulating inflammatory reactions or promoting tissue healing. For example, scientists can focus on cells that regulate inflammation and promote tissue repair, such as macrophages and regulatory T cells. (2) Although the existing stimuli-responsive nanomaterials have rich functions, their synthesis steps must be simplified, making it difficult for others to replicate experimental results and conduct clinical transformation (Lu et al., 2016). Therefore, scientists need to design stimuli-responsive nanomaterials with simple synthesis steps that do not require strict experimental conditions. (3) The existing stimuli-responsive nanomaterials have high costs and strict storage conditions (Gu et al., 2016). Therefore, cheaper, stable, and easily obtainable materials should be more widely used in constructing stimuli-responsive nanomaterials. (4) Life activities have the characteristics of complexity and variability, the metabolic patterns of nanomaterials in the body are still unclear, and the diseases' fate also needs to be explored (Couvreur and Vauthier, 2006). Therefore, even though nanomaterials have been approved for clinical treatment, they still need some help. We should obtain more data from animal experiments and preclinical studies about stimuli-responsive nanomaterials. (5) Reduction in size of nanomaterials makes them deposit in the main organs, which affects the biocompatibility of nanomaterials and causes cytotoxicity. In addition, more complex nanomedicines in recent years will also impact on their safety. However, current preclinical toxicity experimental data cannot represent the real situation of the human body. Microfluidics technologies provide cytotoxicity tests with more realistic real microenvironments, they can improve the reliability of cytotoxicity tests (Beckwith et al., 2018). (6) The further commercial prospects of these strategies are of great significance for their sustainable development (Rao et al., 2018). However, there are many challenges currently hampering their commercial prospects, including scalable manufacturing, biocompatibility, regulation and socioeconomic acceptance. The solution to this problem demands collaboration among materials scientists, pharmacists, clinicians, funding bodies, governments and big companies.
5 Conclusion
In conclusion, stimuli-responsive nanomaterials and their antibacterial strategies have been widely proven in sterilization fields. Next, further application of stimuli-responsive nanomaterials to large animal models is necessary. Afterwards, human trials and FDA approval are needed for further application of stimuli-responsive nanomaterials. Finally, stimuli-responsive nanomaterials enter the stage of commercial exploration and mass production. Although stimuli-responsive nanomaterials face many challenges in their clinical application, they are still great substitutes for antibiotics. With the advancement of technology, in-depth research exploration, and the improvement of relevant laws and regulations, the application of stimuli-responsive nanomaterials in bacterial infection will inevitably have a bright prospect.
Author contributions
ZW: Writing–original draft. RN: Writing–review and editing. YW: Investigation, Software, Writing–original draft. QW: Data curation, Formal Analysis, Writing–original draft. XL: Formal Analysis, Methodology, Project administration, Writing–review and editing. YL: Investigation, Resources, Supervision, Writing–original draft, Writing–review and editing.
Conflict of interest
The authors declare that the research was conducted in the absence of any commercial or financial relationships that could be construed as a potential conflict of interest.
Publisher’s note
All claims expressed in this article are solely those of the authors and do not necessarily represent those of their affiliated organizations, or those of the publisher, the editors and the reviewers. Any product that may be evaluated in this article, or claim that may be made by its manufacturer, is not guaranteed or endorsed by the publisher.
References
Allafchian, A., and Hosseini, S. (2019). Antibacterial magnetic nanoparticles for therapeutics: a review. IET nanobiotechnology 13 (8), 786–799. doi:10.1049/iet-nbt.2019.0146
Andersson, D., and Hughes, D. (2010). Antibiotic resistance and its cost: is it possible to reverse resistance? Nat. Rev. Microbiol. 8 (4), 260–271. doi:10.1038/nrmicro2319
Bag, N., Bardhan, S., Roy, S., Roy, J., Mondal, D., Guo, B., et al. (2023). Nanoparticle-mediated stimulus-responsive antibacterial therapy. Biomaterials Sci. 11 (6), 1994–2019. doi:10.1039/d2bm01941h
Bai, X., Yang, Y., Zheng, W., Huang, Y., Xu, F., and Bao, Z. (2023). Synergistic photothermal antibacterial therapy enabled by multifunctional nanomaterials: progress and perspectives. Mater. Chem. Front. 7 (3), 355–380. doi:10.1039/d2qm01141g
Beckwith, A. L., Borenstein, J. T., and Velásquez-García, L. F. (2018). Monolithic, 3D-printed microfluidic platform for recapitulation of dynamic tumor microenvironments. J. Microelectromechanical Syst. 27 (6), 1009–1022. doi:10.1109/jmems.2018.2869327
Bender, J., and Flieger, A. (2010). “Lipases as pathogenicity factors of bacterial pathogens of humans,” in Handbook of hydrocarbon and lipid microbiology, 3241–3258.
Bigham, A., Aghajanian, A. H., Allahdaneh, S., and Hassanzadeh-Tabrizi, S. A. (2019). Multifunctional mesoporous magnetic Mg2SiO4–CuFe2O4 core-shell nanocomposite for simultaneous bone cancer therapy and regeneration. Ceram. Int. 45 (15), 19481–19488. doi:10.1016/j.ceramint.2019.06.205
Bjarnsholt, T., Whiteley, M., Rumbaugh, K., Stewart, P., Jensen, P., and Frimodt-Møller, N. (2022). The importance of understanding the infectious microenvironment. Lancet. Infect. Dis. 22 (3), e88–e92. doi:10.1016/s1473-3099(21)00122-5
Brouwer, S., Barnett, T., Ly, D., Kasper, K., De Oliveira, D., Rivera-Hernandez, T., et al. (2020). Prophage exotoxins enhance colonization fitness in epidemic scarlet fever-causing Streptococcus pyogenes. Nat. Commun. 11 (1), 5018–5029. doi:10.1038/s41467-020-18700-5
Buzzo, J., Devaraj, A., Gloag, E., Jurcisek, J., Robledo-Avila, F., Kesler, T., et al. (2021). Z-form extracellular DNA is a structural component of the bacterial biofilm matrix. Cell. 184 (23), 5740–5758.e17. doi:10.1016/j.cell.2021.10.010
Canaparo, R., Foglietta, F., Giuntini, F., Della Pepa, C., Dosio, F., and Serpe, L. (2019). Recent developments in antibacterial therapy: focus on stimuli-responsive drug-delivery systems and therapeutic nanoparticles. Mol. (Basel, Switz. 24 (10), 1991–2014. doi:10.3390/molecules24101991
Chen, B., Dai, W., He, B., Zhang, H., Wang, X., Wang, Y., et al. (2017). Current multistage drug delivery systems based on the tumor microenvironment. Theranostics 7 (3), 538–558. doi:10.7150/thno.16684
Chen, Y., Gao, Y., Chen, Y., Liu, L., Mo, A., and Peng, Q. (2020). Nanomaterials-based photothermal therapy and its potentials in antibacterial treatment. J. Control. release 328, 251–262. official journal of the Controlled Release Society. doi:10.1016/j.jconrel.2020.08.055
Cheng, S., Wang, Q., Qi, M., Sun, W., Wang, K., Li, W., et al. (2023a). Nanomaterials-mediated on-demand and precise antibacterial therapies. Mater. Des. 230, 111982. doi:10.1016/j.matdes.2023.111982
Cheng, S., Qi, M., Li, W., Sun, W., Li, M., Lin, J., et al. (2023b). Dual-responsive nanocomposites for synergistic antibacterial therapies facilitating bacteria-infected wound healing. Adv. Healthc. Mater. 12 (6), e2202652. doi:10.1002/adhm.202202652
Cossart, P., and Sansonetti, P. (2004). Bacterial invasion: the paradigms of enteroinvasive pathogens. Sci. (New York, N.Y.) 304 (5668), 242–248. doi:10.1126/science.1090124
Couvreur, P., and Vauthier, C. (2006). Nanotechnology: intelligent design to treat complex disease. Pharm. Res. 23, 1417–1450. doi:10.1007/s11095-006-0284-8
Cui, F., Li, T., Wang, D., Yi, S., Li, J., and Li, X. (2022). Recent advances in carbon-based nanomaterials for combating bacterial biofilm-associated infections. J. Hazard. Mater. 431, 128597. doi:10.1016/j.jhazmat.2022.128597
Davies, D. (2003). Understanding biofilm resistance to antibacterial agents. Nat. Rev. Drug Discov. 2 (2), 114–122. doi:10.1038/nrd1008
Dong, H., Xiu, W., Wan, L., Li, Q., Zhang, Y., Ding, M., et al. (2023a). Biofilm microenvironment response nanoplatform synergistically degrades biofilm structure and relieves hypoxia for efficient sonodynamic therapy. Chem. Eng. J. 453, 139839. doi:10.1016/j.cej.2022.139839
Dong, S., Dong, Y., Zhao, Z., Liu, J., Liu, S., Feng, L., et al. (2023b). “Electron transport chain interference” strategy of amplified mild-photothermal therapy and defect-engineered multi-enzymatic activities for synergistic tumor-personalized suppression. J. Am. Chem. Soc. 145 (17), 9488–9507. doi:10.1021/jacs.2c09608
Elbourne, A., Cheeseman, S., Atkin, P., Truong, N. P., Syed, N., Zavabeti, A., et al. (2020). Antibacterial liquid metals: biofilm treatment via magnetic activation. ACS Nano 14 (1), 802–817. doi:10.1021/acsnano.9b07861
Eming, S., Smola, H., Hartmann, B., Malchau, G., Wegner, R., Krieg, T., et al. (2008). The inhibition of matrix metalloproteinase activity in chronic wounds by a polyacrylate superabsorber. Biomaterials 29 (19), 2932–2940. doi:10.1016/j.biomaterials.2008.03.029
Fang, J., Wan, Y., Sun, Y., Sun, X., Qi, M., Cheng, S., et al. (2022). Near-infrared-activated nanohybrid coating with black phosphorus/zinc oxide for efficient biofilm eradication against implant-associated infections. Chem. Eng. J. 435, 134935. doi:10.1016/j.cej.2022.134935
Flores-Mireles, A., Walker, J., Caparon, M., and Hultgren, S. (2015). Urinary tract infections: epidemiology, mechanisms of infection and treatment options. Nat. Rev. Microbiol. 13 (5), 269–284. doi:10.1038/nrmicro3432
Gao, F., Shao, T., Yu, Y., Xiong, Y., and Yang, L. (2021). Surface-bound reactive oxygen species generating nanozymes for selective antibacterial action. Nat. Commun. 12 (1), 745–762. doi:10.1038/s41467-021-20965-3
Graves, D. (2008). Cytokines that promote periodontal tissue destruction. J. periodontology 79, 1585–1591. doi:10.1902/jop.2008.080183
Gu, M., Zhang, Q., and Lamon, S. (2016). Nanomaterials for optical data storage. Nat. Rev. Mater. 1 (12), 16070–16083. doi:10.1038/natrevmats.2016.70
Han, H., Gao, Y., Chai, M., Zhang, X., Liu, S., Huang, Y., et al. (2020). Biofilm microenvironment activated supramolecular nanoparticles for enhanced photodynamic therapy of bacterial keratitis. J. Control. Release 327, 676–687. doi:10.1016/j.jconrel.2020.09.014
Hatamie, S., Shih, P.-J., Soufi Zomorod, M., Heravi, P., Ahadian, M. M., and Hatami, N. (2020). Hyperthermia response of PEGylated magnetic graphene nanocomposites for heating applications and accelerate antibacterial activity using magnetic fluid hyperthermia. Appl. Phys. A 126 (4), 276–285. doi:10.1007/s00339-020-3454-3
He, Y., Wan, J., Yang, Y., Yuan, P., Yang, C., Wang, Z., et al. (2019). Multifunctional polypyrrole-coated mesoporous TiO2 nanocomposites for photothermal, sonodynamic, and chemotherapeutic treatments and dual-modal ultrasound/photoacoustic imaging of tumors. Adv. Healthc. Mater. 8 (9), e1801254. doi:10.1002/adhm.201801254
He, J., Zhang, H., Zhu, J., Zhang, X., Liu, X., Ramachandraiah, K., et al. (2022). Layer-by-layer synthesis of Au nanorods@metal-organic framework core-shell nanohybrids for magnetic resonance imaging guided photothermal therapy. Mater. Today Commun. 33, 104560. doi:10.1016/j.mtcomm.2022.104560
He, L., Di, D., Chu, X., Liu, X., Wang, Z., Lu, J., et al. (2023). Photothermal antibacterial materials to promote wound healing. J. Control. Release 363, 180–200. doi:10.1016/j.jconrel.2023.09.035
Hicks, J., Halkerston, R., Silman, N., Jackson, S., Aylott, J., and Rawson, F. (2019). Real-time bacterial detection with an intracellular ROS sensing platform. Biosens. Bioelectron. 141, 111430. doi:10.1016/j.bios.2019.111430
Hotze, E., Phenrat, T., and Lowry, G. (2010). Nanoparticle aggregation: challenges to understanding transport and reactivity in the environment. J. Environ. Qual. 39 (6), 1909–1924. doi:10.2134/jeq2009.0462
Hu, D., Zhang, J., Gao, G., Sheng, Z., Cui, H., and Cai, L. (2016). Indocyanine green-loaded polydopamine-reduced graphene oxide nanocomposites with amplifying photoacoustic and photothermal effects for cancer theranostics. Theranostics 6 (7), 1043–1052. doi:10.7150/thno.14566
Hu, X., Shang, Y., Yan, K., Sedgwick, A., Gan, H., Chen, G., et al. (2021). Low-dimensional nanomaterials for antibacterial applications. J. Mater. Chem. B 9 (17), 3640–3661. doi:10.1039/d1tb00033k
Hu, X., Zhang, H., Wang, Y., Shiu, B.-C., Lin, J.-H., Zhang, S., et al. (2022a). Synergistic antibacterial strategy based on photodynamic therapy: progress and perspectives. Chem. Eng. J. 450, 138129. doi:10.1016/j.cej.2022.138129
Hu, Y., Ruan, X., Lv, X., Xu, Y., Wang, W., Cai, Y., et al. (2022b). Biofilm microenvironment-responsive nanoparticles for the treatment of bacterial infection. Nano Today 46, 101602. doi:10.1016/j.nantod.2022.101602
Huang, Y., Zou, L., Wang, J., Jin, Q., and Ji, J. (2022a). Stimuli-responsive nanoplatforms for antibacterial applications. Wiley Interdiscip. Rev. Nanomedicine nanobiotechnology 14 (3), e1775. doi:10.1002/wnan.1775
Huang, S., Xu, S., Hu, Y., Zhao, X., Chang, L., Chen, Z., et al. (2022b). Preparation of NIR-responsive, ROS-generating and antibacterial black phosphorus quantum dots for promoting the MRSA-infected wound healing in diabetic rats. Acta biomater. 137, 199–217. doi:10.1016/j.actbio.2021.10.008
Ji, Y., Yang, M., Dong, H., Hou, T., Wang, L., and Li, Y. (2017). Two-dimensional germanium monochalcogenide photocatalyst for water splitting under ultraviolet, visible to near-infrared light. Nanoscale 9 (25), 8608–8615. doi:10.1039/c7nr00688h
Ji, Y., Han, Z., Ding, H., Xu, X., Wang, D., Zhu, Y., et al. (2021). Enhanced eradication of bacterial/fungi biofilms by glucose oxidase-modified magnetic nanoparticles as a potential treatment for persistent endodontic infections. ACS Appl. Mater. interfaces 13 (15), 17289–17299. doi:10.1021/acsami.1c01748
Jia, C., Guo, Y., and Wu, F. (2022). Chemodynamic therapy via fenton and fenton-like nanomaterials: strategies and recent advances. Small (Weinheim der Bergstrasse, Ger. 18 (6), e2103868. doi:10.1002/smll.202103868
Jiang, Y., Song, B., Brandt, B., Cheng, L., Zhou, X., Exterkate, R., et al. (2021). Comparison of red-complex bacteria between saliva and subgingival plaque of periodontitis patients: a systematic review and meta-analysis. Front. Cell. Infect. Microbiol. 11, 727732. doi:10.3389/fcimb.2021.727732
Jung, H., Verwilst, P., Sharma, A., Shin, J., Sessler, J., and Kim, J. (2018). Organic molecule-based photothermal agents: an expanding photothermal therapy universe. Chem. Soc. Rev. 47 (7), 2280–2297. doi:10.1039/c7cs00522a
Kamaruzzaman, N. F., Kendall, S., and Good, L. (2017). Targeting the hard to reach: challenges and novel strategies in the treatment of intracellular bacterial infections. Br. J. Pharmacol. 174 (14), 2225–2236. doi:10.1111/bph.13664
Karimi, M., Ghasemi, A., Sahandi Zangabad, P., Rahighi, R., Moosavi Basri, S., Mirshekari, H., et al. (2016). Smart micro/nanoparticles in stimulus-responsive drug/gene delivery systems. Chem. Soc. Rev. 45 (5), 1457–1501. doi:10.1039/c5cs00798d
Khan, I., Saeed, K., and Khan, I. (2019). Nanoparticles: properties, applications and toxicities. Arabian J. Chem. 12 (7), 908–931. doi:10.1016/j.arabjc.2017.05.011
Kreyling, W. G., Semmler-Behnke, M., and Chaudhry, Q. (2010). A complementary definition of nanomaterial. Nano today 5 (3), 165–168. doi:10.1016/j.nantod.2010.03.004
Lambert, P. (2005). Bacterial resistance to antibiotics: modified target sites. Adv. drug Deliv. Rev. 57 (10), 1471–1485. doi:10.1016/j.addr.2005.04.003
Lee, H., and Gaharwar, A. (2020). Light-responsive inorganic biomaterials for biomedical applications. Adv. Sci. (Weinheim, Baden-Wurttemberg, Ger. 7 (17), 2000863. doi:10.1002/advs.202000863
Lei, X., Li, M., Wang, C., Cui, P., Qiu, L., Zhou, S., et al. (2022). Degradable microneedle patches loaded with antibacterial gelatin nanoparticles to treat staphylococcal infection-induced chronic wounds. Int. J. Biol. Macromol. 217, 55–65. doi:10.1016/j.ijbiomac.2022.07.021
Li, J., Ding, Z., Li, Y., Miao, J., Wang, W., Nundlall, K., et al. (2020). Reactive oxygen species-sensitive thioketal-linked mesoporous silica nanoparticles as drug carrier for effective antibacterial activity. Mater. Des. 195, 109021. doi:10.1016/j.matdes.2020.109021
Li, Z., Li, Y., Chen, C., and Cheng, Y. (2021a). Magnetic-responsive hydrogels: from strategic design to biomedical applications. J. Control. Release 335, 541–556. doi:10.1016/j.jconrel.2021.06.003
Li, H., Li, D., Chen, F., Yang, C., Li, X., Zhang, Y., et al. (2021b). Nanosilver-decorated biodegradable mesoporous organosilica nanoparticles for GSH-responsive gentamicin release and synergistic treatment of antibiotic-resistant bacteria. Int. J. nanomedicine 16, 4631–4642. doi:10.2147/ijn.s315067
Li, J., Hu, Y., Hu, B., Wang, W., Xu, H., Hu, X., et al. (2022). Lactose azocalixarene drug delivery system for the treatment of multidrug-resistant pseudomonas aeruginosa infected diabetic ulcer. Nat. Commun. 13 (1), 6279–6290. doi:10.1038/s41467-022-33920-7
Lin, A., Liu, Y., Zhu, X., Chen, X., Liu, J., Zhou, Y., et al. (2019). Bacteria-responsive biomimetic selenium nanosystem for multidrug-resistant bacterial infection detection and inhibition. ACS Nano 13 (12), 13965–13984. doi:10.1021/acsnano.9b05766
Lin, Q., Li, C., Wang, L., Cai, H., Tang, L., and Gu, Y. (2022). Ultrasensitive near-infrared fluorescence probe activated by nitroreductase for in vivo hypoxia detection. Sensors Actuators B Chem. 371, 132521. doi:10.1016/j.snb.2022.132521
Liu, X., Li, Y., Wang, K., Chen, Y., Shi, M., Zhang, X., et al. (2021). GSH-responsive nanoprodrug to inhibit glycolysis and alleviate immunosuppression for cancer therapy. Nano Lett. 21 (18), 7862–7869. doi:10.1021/acs.nanolett.1c03089
Liu, S., Chen, X., Yu, M., Li, J., Liu, J., Xie, Z., et al. (2022). Applications of titanium dioxide nanostructure in stomatology. Mol. (Basel, Switz. 27 (12), 3881–3898. doi:10.3390/molecules27123881
Lu, M., Ozcelik, A., Grigsby, C. L., Zhao, Y., Guo, F., Leong, K. W., et al. (2016). Microfluidic hydrodynamic focusing for synthesis of nanomaterials. Nano Today 11 (6), 778–792. doi:10.1016/j.nantod.2016.10.006
Ma, D., Huang, C., Zheng, J., Zhou, W., Tang, J., Chen, W., et al. (2019). Azoreductase-responsive nanoprobe for hypoxia-induced mitophagy imaging. Anal. Chem. 91 (2), 1360–1367. doi:10.1021/acs.analchem.8b03492
Ma, Z., Li, J., Bai, Y., Zhang, Y., Sun, H., and Zhang, X. (2020). A bacterial infection-microenvironment activated nanoplatform based on spiropyran-conjugated glycoclusters for imaging and eliminating of the biofilm. Chem. Eng. J. 399, 125787. doi:10.1016/j.cej.2020.125787
Marimani, M., Ahmad, A., and Duse, A. (2018). The role of epigenetics, bacterial and host factors in progression of Mycobacterium tuberculosis infection. Tuberc. Edinb. Scotl. 113, 200–214. doi:10.1016/j.tube.2018.10.009
Mizoshita, N., and Tanaka, H. (2017). Interface-assisted synthesis of mesoporous silica nanoparticles using neat tetraalkoxysilanes. Microporous Mesoporous Mater. 239, 1–8. doi:10.1016/j.micromeso.2016.09.025
Ning, S., Dai, X., Tang, W., Guo, Q., Lyu, M., Zhu, D., et al. (2022). Cancer cell membrane-coated C-TiO2 hollow nanoshells for combined sonodynamic and hypoxia-activated chemotherapy. Acta Biomater. 152, 562–574. doi:10.1016/j.actbio.2022.08.067
Peng, H., Xie, B., Cen, X., Dai, J., Dai, Y., Yang, X., et al. (2022). Glutathione-responsive multifunctional nanoparticles based on mannose-modified pillar[5]arene for targeted antibiotic delivery against intracellular methicillin-resistant S. aureus. Mater. Chem. Front. 6 (3), 360–367. doi:10.1039/d1qm01459e
Pornpattananangkul, D., Zhang, L., Olson, S., Aryal, S., Obonyo, M., Vecchio, K., et al. (2011). Bacterial toxin-triggered drug release from gold nanoparticle-stabilized liposomes for the treatment of bacterial infection. J. Am. Chem. Soc. 133 (11), 4132–4139. doi:10.1021/ja111110e
Qi, M., Li, X., Sun, X., Li, C., Tay, F., Weir, M., et al. (2019). Novel nanotechnology and near-infrared photodynamic therapy to kill periodontitis-related biofilm pathogens and protect the periodontium. Dent. Mater. official Publ. Acad. Dent. Mater. 35 (11), 1665–1681. doi:10.1016/j.dental.2019.08.115
Qi, M., Ren, X., Li, W., Sun, Y., Sun, X., Li, C., et al. (2022). NIR responsive nitric oxide nanogenerator for enhanced biofilm eradication and inflammation immunotherapy against periodontal diseases. Nano Today 43, 101447. doi:10.1016/j.nantod.2022.101447
Quinn, J., Whittaker, M., and Davis, T. (2015). Delivering nitric oxide with nanoparticles. J. Control. release 205, 190–205. official journal of the Controlled Release Society. doi:10.1016/j.jconrel.2015.02.007
Rao, R., Pint, C. L., Islam, A. E., Weatherup, R. S., Hofmann, S., Meshot, E. R., et al. (2018). Carbon nanotubes and related nanomaterials: critical advances and challenges for synthesis toward mainstream commercial applications. ACS Nano 12 (12), 11756–11784. doi:10.1021/acsnano.8b06511
Rodrigues, J., and Correia, J. (2022). Enhanced photodynamic therapy: a review of combined energy sources. Cells 11 (24), 3995–4012. doi:10.3390/cells11243995
Roy, J., Pandey, V., Gupta, I., and Shekhar, H. (2021). Antibacterial sonodynamic therapy: current status and future perspectives. ACS biomaterials Sci. Eng. 7 (12), 5326–5338. doi:10.1021/acsbiomaterials.1c00587
Singh, S., Barick, K. C., and Bahadur, D. (2015). Inactivation of bacterial pathogens under magnetic hyperthermia using Fe3O4–ZnO nanocomposite. Powder Technol. 269, 513–519. doi:10.1016/j.powtec.2014.09.032
Su, Z., Kong, L., Dai, Y., Tang, J., Mei, J., Qian, Z., et al. (2022). Bioresponsive nano-antibacterials for H2S-sensitized hyperthermia and immunomodulation against refractory implant-related infections. Sci. Adv. 8 (14), eabn1701–1901. doi:10.1126/sciadv.abn1701
Sun, X., Sun, J., Sun, Y., Li, C., Fang, J., Zhang, T., et al. (2021). Oxygen self-sufficient nanoplatform for enhanced and selective antibacterial photodynamic therapy against anaerobe-induced periodontal disease. Adv. Funct. Mater. 31 (20), 2101040. doi:10.1002/adfm.202101040
Suzuki, K., Sato, H., Kikuchi, T., Abe, T., Nakaji, S., Sugawara, K., et al. (1996). Capacity of circulating neutrophils to produce reactive oxygen species after exhaustive exercise. J. Appl. physiology (Bethesda, Md, 1985) 81 (3), 1213–1222. doi:10.1152/jappl.1996.81.3.1213
Tan, H., Tian, Y., Yang, H., Liu, Z., Liang, X., Li, B., et al. (2021). Oxygen-sufficient lipid nanobubbles combined with UTMD for enhanced sonodynamic therapy of Hep-G2 cells. J. Biomed. Mater. Res. Part B, Appl. biomaterials 109 (11), 1796–1806. doi:10.1002/jbm.b.34839
Taylor, P. W., Stapleton, P. D., and Luzio, J. P. (2002). New ways to treat bacterial infections. Drug Discov. today 7 (21), 1086–1091. doi:10.1016/s1359-6446(02)02498-4
Teng, K., Niu, L., and Yang, Q. (2023). Supramolecular photosensitizer enables oxygen-independent generation of hydroxyl radicals for photodynamic therapy. J. Am. Chem. Soc. 145 (7), 4081–4087. doi:10.1021/jacs.2c11868
Thomas-Moore, B., Del Valle, C., Field, R., and Marín, M. (2022). Recent advances in nanoparticle-based targeting tactics for antibacterial photodynamic therapy. Photochem. photobiological Sci. 21 (6), 1111–1131. Official journal of the European Photochemistry Association and the European Society for Photobiology. doi:10.1007/s43630-022-00194-3
Um, W., Lee, J., Pramod Kumar, E. K., Kim, C., You, D., and Park, J. (2021). Recent advances in nanomaterial-based augmented sonodynamic therapy of cancer. Chem. Commun. Camb. Engl. 57 (23), 2854–2866. doi:10.1039/d0cc07750j
Uroro, E. O., Bright, R., Lakshika Dabare, P. R., Quek, J. Y., Goswami, N., and Vasilev, K. (2023). Enzyme-responsive polycationic silver nanocluster-loaded PCL nanocomposites for antibacterial applications. Mater. Today Chem. 28, 101376. doi:10.1016/j.mtchem.2023.101376
Wan, Y., Fang, J., Wang, Y., Sun, J., Sun, Y., Sun, X., et al. (2021). Antibacterial zeolite imidazole frameworks with manganese doping for immunomodulation to accelerate infected wound healing. Adv. Healthc. Mater. 10 (22), e2101515. doi:10.1002/adhm.202101515
Wang, L., Fang, X., and Pan, G. (2004). Bacillary dysentery as a causative factor of irritable bowel syndrome and its pathogenesis. Gut 53 (8), 1096–1101. doi:10.1136/gut.2003.021154
Wang, X., Wang, X., Yue, Q., Xu, H., Zhong, X., Sun, L., et al. (2021a). Liquid exfoliation of TiN nanodots as novel sonosensitizers for photothermal-enhanced sonodynamic therapy against cancer. Nano Today 39, 101170. doi:10.1016/j.nantod.2021.101170
Wang, X., Shi, Q., Zha, Z., Zhu, D., Zheng, L., Shi, L., et al. (2021b). Copper single-atom catalysts with photothermal performance and enhanced nanozyme activity for bacteria-infected wound therapy. Bioact. Mater. 6 (12), 4389–4401. doi:10.1016/j.bioactmat.2021.04.024
Wang, K., Shang, T., Zhang, L., Zhou, L., Liu, C., Fu, Y., et al. (2021c). Application of a reactive oxygen species-responsive drug-eluting coating for surface modification of vascular stents. ACS Appl. Mater. Interfaces 13 (30), 35431–35443. doi:10.1021/acsami.1c08880
Wang, Z., Liu, X., Duan, Y., and Huang, Y. (2022a). Infection microenvironment-related antibacterial nanotherapeutic strategies. Biomaterials 280, 121249. doi:10.1016/j.biomaterials.2021.121249
Wang, L., Li, Y., Ren, M., Wang, X., Li, L., Liu, F., et al. (2022b). pH and lipase-responsive nanocarrier-mediated dual drug delivery system to treat periodontitis in diabetic rats. Bioact. Mater. 18, 254–266. doi:10.1016/j.bioactmat.2022.02.008
White, A., Gompertz, S., Bayley, D., Hill, S., O'Brien, C., Unsal, I., et al. (2003). Resolution of bronchial inflammation is related to bacterial eradication following treatment of exacerbations of chronic bronchitis. Thorax 58 (8), 680–685. doi:10.1136/thorax.58.8.680
Woo, C., Tso, P., and Yiu, J. (2022). Commensal gut microbiota-based strategies for oral delivery of therapeutic proteins. Trends Pharmacol. Sci. 43 (12), 1004–1013. doi:10.1016/j.tips.2022.08.002
Wu, Y., Song, Z., Wang, H., and Han, H. (2019). Endogenous stimulus-powered antibiotic release from nanoreactors for a combination therapy of bacterial infections. Nat. Commun. 10 (1), 4464–4564. doi:10.1038/s41467-019-12233-2
Wu, N., Fan, C.-H., and Yeh, C.-K. (2022). Ultrasound-activated nanomaterials for sonodynamic cancer theranostics. Drug Discov. Today 27 (6), 1590–1603. doi:10.1016/j.drudis.2022.02.025
Xin, Y., Guo, Z., Ma, A., Shi, E., Li, Z., Liang, Z., et al. (2023). A robust ROS generation nanoplatform combating periodontitis via sonodynamic/chemodynamic combination therapy. Chem. Eng. J. 451, 138782. doi:10.1016/j.cej.2022.138782
Xiu, W., Wan, L., Yang, K., Li, X., Yuwen, L., Dong, H., et al. (2022). Potentiating hypoxic microenvironment for antibiotic activation by photodynamic therapy to combat bacterial biofilm infections. Nat. Commun. 13 (1), 3875–3888. doi:10.1038/s41467-022-31479-x
Xu, X., Fan, M., Yu, Z., Zhao, Y., Zhang, H., Wang, J., et al. (2022). A removable photothermal antibacterial “warm paste” target for cariogenic bacteria. Chem. Eng. J. 429, 132491. doi:10.1016/j.cej.2021.132491
Yang, Y., Huang, Z., and Li, L. (2021). Advanced nitric oxide donors: chemical structure of NO drugs, NO nanomedicines and biomedical applications. Nanoscale 13 (2), 444–459. doi:10.1039/d0nr07484e
Yu, H., Xu, X., Xie, Z., Huang, X., Lin, L., Jiao, Y., et al. (2022). High-efficiency near-infrared light responsive antibacterial system for synergistic ablation of bacteria and biofilm. ACS Appl. Mater. interfaces 14 (32), 36947–36956. doi:10.1021/acsami.2c08406
Yu, C., Li, L., Wang, S., Xu, Y., Wang, L., Huang, Y., et al. (2023). Advances in nanomaterials for the diagnosis and treatment of head and neck cancers: a review. Bioact. Mater. 25, 430–444. doi:10.1016/j.bioactmat.2022.08.010
Yuan, Z., Lin, C., He, Y., Tao, B., Chen, M., Zhang, J., et al. (2020). Near-infrared light-triggered nitric-oxide-enhanced photodynamic therapy and low-temperature photothermal therapy for biofilm elimination. ACS Nano 14 (3), 3546–3562. doi:10.1021/acsnano.9b09871
Zhang, H., Fan, T., Chen, W., Li, Y., and Wang, B. (2020). Recent advances of two-dimensional materials in smart drug delivery nano-systems. Bioact. Mater. 5 (4), 1071–1086. doi:10.1016/j.bioactmat.2020.06.012
Zhang, B., Lu, D., and Duan, H. (2023). Recent advances in responsive antibacterial materials: design and application scenarios. Biomaterials Sci. 11 (2), 356–379. doi:10.1039/d2bm01573k
Zhou, Q., Si, Z., Wang, K., Li, K., Hong, W., Zhang, Y., et al. (2022). Enzyme-triggered smart antimicrobial drug release systems against bacterial infections. J. Control. Release 352, 507–526. doi:10.1016/j.jconrel.2022.10.038
Keywords: stimuli-responsive, nanomaterials, antibacterial, exogenous stimuli, microenvironment stimuli
Citation: Wu Z, Nie R, Wang Y, Wang Q, Li X and Liu Y (2023) Precise antibacterial therapeutics based on stimuli-responsive nanomaterials. Front. Bioeng. Biotechnol. 11:1289323. doi: 10.3389/fbioe.2023.1289323
Received: 05 September 2023; Accepted: 09 October 2023;
Published: 18 October 2023.
Edited by:
Chao Yin, Nanjing University of Posts and Telecommunications, ChinaReviewed by:
Li Jie, Nanjing Tech University, ChinaMeng Ding, Nanjing University, China
Xuefeng Yang, Anhui University, China
Copyright © 2023 Wu, Nie, Wang, Wang, Li and Liu. This is an open-access article distributed under the terms of the Creative Commons Attribution License (CC BY). The use, distribution or reproduction in other forums is permitted, provided the original author(s) and the copyright owner(s) are credited and that the original publication in this journal is cited, in accordance with accepted academic practice. No use, distribution or reproduction is permitted which does not comply with these terms.
*Correspondence: Yuguang Liu, bGl1eWcxOTg5QDE2My5jb20=