- 1Shandong Key Laboratory of Rheumatic Disease and Translational Medicine, Department of Oncology, Shandong Lung Cancer Institute, The First Affiliated Hospital of Shandong First Medical University and Shandong Provincial Qianfoshan Hospital, Jinan, China
- 2School of Clinical Medicine, Jining Medical University, Jining, China
Cancer therapies, such as chemotherapy and radiotherapy, are often unsatisfactory due to several limitations, including drug resistance, inability to cross biological barriers, and toxic side effects on the body. These drawbacks underscore the need for alternative treatments that can overcome these challenges and provide more effective and safer options for cancer patients. In recent years, the use of live bacteria, engineered bacteria, or bacterial derivatives to deliver antitumor drugs to specific tumor sites for controlled release has emerged as a promising therapeutic tool. This approach offers several advantages over traditional cancer therapies, including targeted drug delivery and reduced toxicity to healthy tissues. Ongoing research in this field holds great potential for further developing more efficient and personalized cancer therapies, such as E. coli, Salmonella, Listeria, and bacterial derivatives like outer membrane vesicles (OMVs), which can serve as vehicles for drugs, therapeutic proteins, or antigens. In this review, we describe the advances, challenges, and future directions of research on using live bacteria or OMVs as carriers or components derived from bacteria of delivery systems for cancer therapy.
1 Introduction
Based on the “Global Cancer Statistics 2020” released by the American Cancer Society, cancer is a significant human health threat in developed and developing countries. It is increasingly becoming a leading cause of death, with over half of all cases occurring in Asia (Sung et al., 2021). Existing cancer treatments include chemotherapy, radiation therapy, surgery, and biologic therapies (Lou et al., 2021). Chemotherapy is a systemic treatment that can kill tumor cells but may also harm normal cells, leading to side effects and a high risk of drug resistance (Liang et al., 2019). Radiotherapy is a localized treatment, but it may not reach certain parts of the tumor, and some tumors are not sensitive to radiation therapy, resulting in treatment failure. Immunotherapy, which includes immune checkpoint inhibitors, tumor vaccines, CAR-T cell therapy, and others (Waldman et al., 2020), typically has fewer toxic side effects than traditional antitumor therapies. However, the therapeutic effect of single immunotherapy is often limited, and it is usually combined with radiotherapy and chemotherapy to enhance tumor treatment effectiveness (Cao and Liu, 2020). Therefore, there is an urgent need to investigate new therapeutic approaches for tumor control.
Bacteria are organisms that have existed for millions of years and are essential for human health due to their unique functions, such as motility, microenvironment sensing, nutrient competition, intra-tumor targeting, the presence of extracellular enzymes, and immune stimulation (Dróżdż et al., 2020). Additionally, some anaerobic bacteria can survive and multiply under hypoxic or micro-hypoxic conditions. Facultative anaerobes, including Salmonella, are highly adaptable, can colonize aerobic and anoxic environments, produce ATP aerobically or anaerobically and promote the migration (Dróżdż et al., 2020). This ability enables the effective control of antitumor loads at the tumor site and precise timing of drug transport while reducing systemic toxicity to the host by regulating the expression of bacterial genes (Yang et al., 2021). Based on this, certain facultative anaerobes, such as Escherichia coli (Jiang et al., 2010), Salmonella (Liang et al., 2019), and Listeria monocytogene (Yang et al., 2014), have been exploited or genetically modified to become tumor-targeting bacteria for delivering cytokines, immunomodulators (Chowdhury et al., 2019), cytotoxic agents (Li et al., 2019), therapeutic peptides/proteins (Chiang and Huang, 2021), prodrug-converting enzymes (Aganja et al., 2022), and small interfering RNA to the tumor site (Yang et al., 2021), moreover, bacteria’s capacity to produce outer membrane vesicles (OMVs) has been utilized for tumor immunity, tumor-engineered vaccines, and drug delivery, making it a novel tool with great potential for antitumor therapy and biological applications (Figure 1).
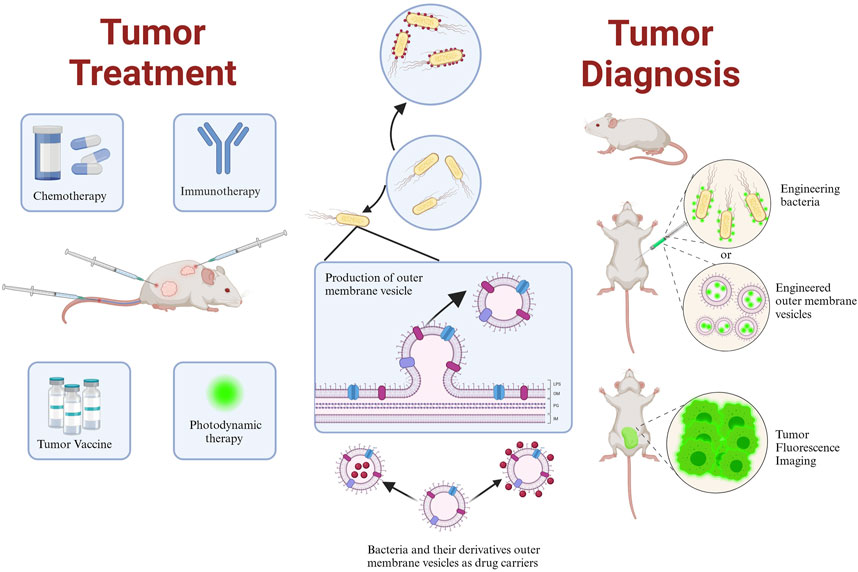
FIGURE 1. Schematic representation of the application of bacteria and their derivative outer membrane vesicles in tumor therapy and diagnosis. Created with BioRender.com.
In recent years, with the advancements in molecular biology and nanotechnology, there have been significant developments in the design of advantageous components derived from bganxieninde1acteria. These advancements include the development of bacterial membrane-encapsulated nano-drug delivery systems and bacterial-nanoparticle hybridization systems. These innovations not only promote the application of bacteria but also offer new opportunities for optimizing protocols in tumor treatment (Cacicedo et al., 2018; Hou et al., 2021; Li et al., 2022; Wang et al., 2022; Xie et al., 2022). This article aimed to summarize the genetic editing or engineering techniques used in several facultative anaerobes and other bacteria for antitumor therapy and to discuss the potential future directions in drug delivery vehicles.
2 Facultative anaerobes-based drug delivery systems
Genetically engineered bacteria can be utilized as vectors for tumor treatment or immune system activation (Figure 2) (Fu et al., 2023). Their ability to colonize tumors as drug-delivery vehicles depends on the conditions and composition of the tumor microenvironment (TME). The TME is a complex multicellular environment that includes soluble factors, transformed extracellular matrix, epigenetically modified and reprogrammed fibroblasts, as well as immunosuppressive cells (Deepak et al., 2020). The TME is characterized by hypoxia and low pH values, primarily caused by abnormal capillary formation leading to hypoxia and nutrient deficiencies in specific areas. Cellular metabolism also produces significant amounts of lactic acid (Zhang et al., 2022). Both the high-lactate and low-nutrient properties of the TME are compatible with the growth conditions of facultative anaerobes. Additionally, hypoxia provides the most common mechanism for bacterial targeting of tumors (Holay et al., 2021). Consequently, the colonization of anaerobic bacteria can be limited to the hypoxic TME without affecting healthy tissues (Zhou et al., 2018; Holay et al., 2021). As drug delivery agents, facultative anaerobes can increase the local drug concentration in the TME, reduce drug extravasation to surrounding healthy tissues, and mitigate drug side effects. This section introduced some classical facultative anaerobes and other bacteria as drug carriers (Table 1).
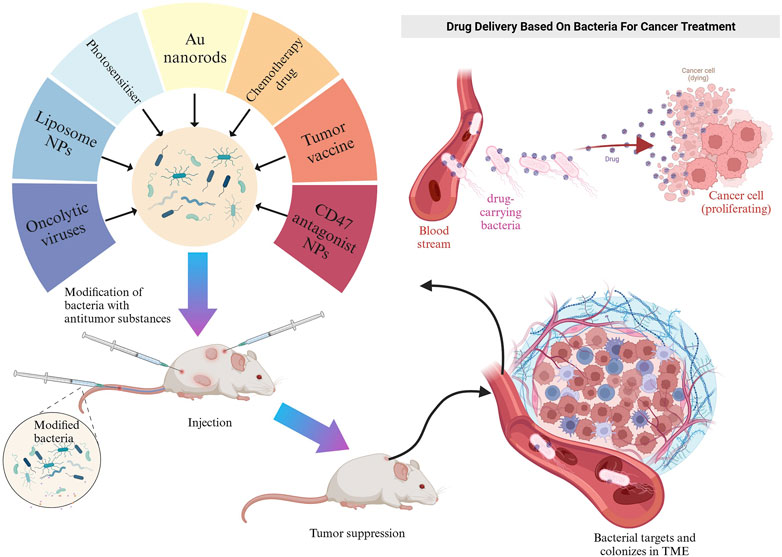
FIGURE 2. Schematic representation of engineered bacteria target TME in mice. Created with BioRender.com.
2.1 E. coli as drug delivery vehicles
E. coli is a facultative anaerobe that can colonize and proliferate in hypoxic or necrotic regions of tumors (Xie et al., 2022). Based on this property, genetically modifying them to carry drugs, cytokines, etc., can lead to antitumor ability, targeting, or production of beneficial substances for tumor therapy (Zhang et al., 2021; Howell and Forbes, 2022). He et al. modified Bovine serum albumin on the surface of attenuated E. coli. They demonstrated that modified E. coli enhanced adherence to and targeted cancer cells, with more vital adherence and targeting of bladder cancer cells with high expression of secreted acidic cysteine-rich proteins (He et al., 2023). Oncolytic viruses can regulate the TME and optimize the effects of immune-mediated tumor eradication (Harrington et al., 2019). However, as a monotherapy, their efficacy is more limited (Fukuhara et al., 2016). Sun et al. encapsulated a layer of biocompatible and biopreservation liposomes on the surface of the oncolytic viruses and then constructed “E. coli liposome-OA.” Thanks to the high homing ability of E. coli, the enrichment rate of lysosomal viruses in the tumor was significantly increased, and the inflammatory response of E. coli in the tumor region was utilized to enhance the infiltration of immune cells in the tumor region (Figure 3) (Sun et al., 2022). Zhang et al. used electroshock to transfer prepared paclitaxel liposomes into E. coli or Lactobacillus casei, and found that the E. coli-containing preparation had a more potent anticancer effect than the L. casei-containing preparation (Zhang et al., 2020). CD47 is a transmembrane protein expressed in phagocytes and abundantly expressed in various malignant cells. CD47 blocks the drive for T cell-mediated immune tumor eradication. The antitumor activity of anti-CD47 therapies is mediated by blocking antiphagocytic signaling (Liu et al., 2015). Sreyan et al. designed E. coli strains containing synchronous lysis circuits to colonize tumors with CD47-releasing nano-antibody antagonists, increasing the activation of tumor-infiltrating T cells and inducing persistent and systemic anti-tumor immunity (Chowdhury et al., 2019). Notably, the ability of E. coli itself to act as a foreign substance to stimulate the immune system and enhance anti-tumor immunity is also a significant advantage as a drug delivery vehicle (Sun et al., 2022).
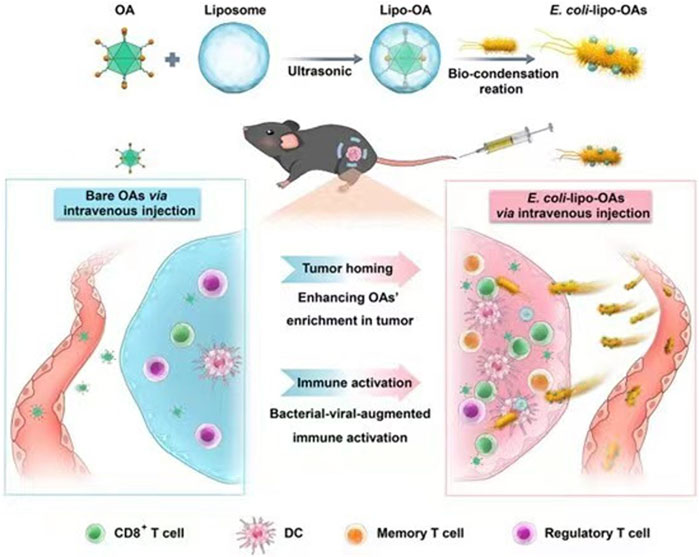
FIGURE 3. Schematic representation of self-propelled bacterium-vessels carrying OAs that can home to the tumor lesion and enhance the antitumor responses through bacterial–viral-augmented immune activation. Reproduced with permission from (Sun et al., 2022). Copyright © 2022 American Chemical Society.
As a highly proliferative tissue, tumor tissue requires neovascularization to transport oxygen, nutrients and excrete metabolic waste (Eulberg et al., 2022). Therefore, tumor progression necessitates the initiation of tumor angiogenesis (Lugano et al., 2020). Conversely, inhibiting tumor angiogenesis or destroying existing vessels can slow tumor progression. Heparan sulfatase 1 (HSulf-1) suppresses tumorigenesis by inhibiting angiogenesis in vivo (Narita et al., 2006). Yang et al. demonstrated that the modification of E. coli to express HSulf-1 (HSEc) in vivo resulted in the overexpression of HSulf-1. HSEc can actively target and colonize tumor sites to hinder angiogenesis and metastasis. Moreover, the surface-attached adriamycin glycogen nanoparticles (NPs) can penetrate tumor cells, leading to intracellular DNA damage (PDOX@). The combined biological and chemotherapeutic treatment with HSEc shows significant inhibition of melanoma and reduced side effects in mouse models (Yang et al., 2023).
E. coli, when genetically modified, can be combined with other therapeutic tools to achieve antitumor effects (Lou et al., 2021). For instance, Sun, Ye et al. modified E. coli to overexpress glucose dehydrogenase. Subsequently, doxorubicin (DOX) was coupled to the surface of the modified E. coli, reducing the toxic effect of DOX through the self-driven tumor colonization property of E. coli, resulting in its selective accumulation in tumors. Meanwhile, DOX activated NADPH oxidase and catalyzed the conversion of NADPH and O2 to NADP+ and reactive oxygen species (ROS), enhancing the efficacy of DOX-mediated immunotherapy and chemotherapy. In a mice tumor model, the modified bacteria inhibited the growth of primary and distant tumors and synergistically enhanced the efficacy of immunotherapy (Ushio-Fukai and Nakamura, 2008; Kan et al., 2014; Skonieczna et al., 2017; Sun et al., 2021). Deng et al. genetically modified non-invasive Escherichia coli to obtain E. coli overexpressing human peroxidase coli(p). Under near-infrared (NIR) light irradiation, the generated O2 was converted to cytotoxic 1O₂. PDA/Ce6 was obtained by encapsulating polydopamine (pDA) with chlorin e6 (ce6), and then pDA/Ce6 was encapsulated with E. coli(p) to obtain E. coli(p)/pDA/Ce6. Coli(p)/pDA/Ce6 was replicated and accumulated in tumors, and NIR light was introduced to achieve photothermal and O2-enhanced photodynamic therapy (PDT) (Deng et al., 2021). In another study conducted by Dai et al., various charges of zinc phthalocyanine (ZnPc) photosensitizers were prepared and investigated for their electrostatic adherence characteristics to E. coli. The researchers found that ZnPc conjugates with four positive charges (designated as ZnPc-IR710) demonstrated the most extraordinary capacity for loading and the best imaging properties of fluorescence to E. coli. With the support of E. coli, E. coli @ ZnPc - IR710 significantly inhibited tumor growth in a mouse subcutaneous 4T1 breast cancer model (Dai et al., 2020). Wang et al. genetically engineered E. coli to produce gas vesicles, known as GVs-E. coli, which carried an acoustic reporter gene (ARG) encoding the formation of gas vesicles (GVs). They performed ultrasound imaging and co-focused ultrasound ablation surgery (FUAS) for hypoxic tumor regions and environments in mice. The researchers enriched multifunctional NPs in tumor target areas and co-delivered multifunctional cationic lipid NPs (including IR780, perfluorohexane, and banoxantrone dihydrochloride (AQ4N)) to tumors, enabling focused multimodal imaging and enhancing the efficacy of FUAS. The hypoxic environment of the tumors stimulated AQ4N to kill tumor cells in conjunction with the FUAS procedure (Wang et al., 2022). Similarly, experiments by Yang and Jiang et al. demonstrated that genetically modified E. coli, carrying an ARG encoding the formation of GVs, could be visualized using ultrasound. Intravenous injection of the modified E. coli for targeted breast cancer therapy specifically targeted tumor areas and persistently localized into the TME, resulting in significant suppression of tumor growth (Yang et al., 2021).
2.1.1 E. coli nissle 1917
The well-studied E. coli Nissle 1917 (Ec N) is a non-pathogenic probiotic that selectively colonizes the intermediate zone between solid tumors’ live and necrotic zones (Li et al., 2019). It is difficult for chemotherapeutic agents to cross the biological barrier to enter solid tumors’ necrotic and hypoxic zones, whereas Ec N can selectively proliferate in these zones. This property makes it a high-quality vehicle for drug delivery (Chen et al., 2023). At the same time, it was found that Ec N also has a significant advantage over conventional nanomedicines in tumor accumulation and overall biodistribution, and the average amount of Ec N detected in tumors is remarkably higher than the number of injections due to the fixation and multiplication of Ec N (Liu et al., 2021). However, using NPs and other decorative drug surfaces can increase the stability of the drug in vivo and prolong the residence time of the drug in the circulatory system. Therefore, nanomaterials can be combined with Ec N to overcome the problem of small cumulative doses of nanomedicine in the tumor region and achieve tumor-targeted therapy. Yao et al. utilized Ec N and synthesized TeNRs intracellularly to obtain Te@EcN, an integrated therapeutic system with a high loading rate and efficient photothermal conversion, which can damage and induce tumor cell death after irradiation with NIR. Meanwhile, Ec N could act as an immune adjuvant to promote dendritic cells (DCs) maturation and further enhance the initiation of cytotoxic T cells. Te@EcN reprogrammed tumor-associated macrophages (TAMs) and remodeled the immune-suppressive TME, which inhibited tumor recurrence and metastasis (Yao et al., 2022).
Ec N can also be modified to produce or carry desired anticancer substances, including butyrate, a short-chain fatty acid produced by colon intestinal bacteria fermenting dietary fiber, known for its ability to inhibit the proliferation of cancer cells (Hajjar et al., 2021; Richter et al., 2021). Deng et al. genetically modified Ec N to synthesize bio butyrate under hypoxic conditions, eliminating the need for butyrate purification and delivering butyrate in situ, thereby mitigating side effects and improving bioavailability. Moreover, the bio butyrate-producing E. coli can also deliver other anticancer drugs or therapeutic adjuvants, further enhancing the therapeutic efficacy of the delivery system in vivo (Chiang and Hong, 2021). It was reported that under the control of the ara BAD promoter (PBAD), which enables Ec N to contain the small cytotoxic protein Hly E, the expression of HlyE was efficiently regulated in a time-controlled manner, resulting in specific colonization of tumors with a tumor-organ ratio of 106:1 and causing tumor cell regression in murine colorectal cancer (Chiang and Huang, 2021). In another study, He et al. utilized Ec N as a carrier to transport the anticancer protein p53 and the antiangiogenic factor Tum-5 to the tumor hypoxic zone in cancer treatment, which yielded excellent tumor treatment results (He et al., 2019). Additionally, Xie et al. attached DOX to Ec N using an acid-soluble cis-aconitic anhydride linker (Ec N-ca-Dox) to achieve targeted accumulation of bacteria in tumors and acid-responsive release of anticancer drugs. In an experiment where Ec N-ca-Dox was intravenously injected into mice for 3 hours, the accumulation of DOX in tumors was 12.9% per Gram of tissue injected dose, which decreased to 6.4% after 3 days. This approach proved to be far superior to nanocarriers, significantly improving the antitumor efficacy of intravenous Ec N-ca-Dox treatment by inhibiting tumor growth, prolonging animal survival, and inducing apoptosis of tumor cells (Xie et al., 2017).
Tumor treatments using Ec N as a drug carrier can also be combined with other treatments, such as chemotherapy, immunotherapy, and photothermal effects, to improve therapeutic outcomes and minimize side effects (Li et al., 2019; Wang et al., 2022; Yao et al., 2022; Zhu et al., 2023). For instance, Xie et al. specifically developed Ec NZ/F@Au by incorporating fluorouracil (5-FU) and macrophage phenotypic modulators zoledronic acids (ZOLs) into Ec N through electroporation and modifying gold nanorods on its surface. When exposed to NIR irradiation, the photothermal effects of the gold nanorods increased the local temperature and facilitated the in-situ conversion of active Ec N into bacterial ghosts (BGs). This conversion led to a gradual release of the drug from BGs through transmembrane channels, while intermittent NIR light triggered a stepwise increase in BGs formation and drug release, enabling controlled drug release. The combined action of 5-FU and ZOLs effectively inhibited tumor growth (Xie et al., 2021). Photosensitizers are crucial in PDT. One example is 5-Aminolevulinic acid (5-ALA), an intelligent photosensitizer that can be converted into protoporphyrin IX (Pp IX), a potent photosensitizer for PDT (Kim et al., 2013; Chen et al., 2021a). Based on this, Chen et al. employed Ec N as a carrier for 5-ALA delivery to colorectal cancer (CRC) cells, which induced the aggregation of anti-tumor protoporphyrin X (PpⅨ) under 630 nm laser irradiation. The results demonstrated the effectiveness of this administration method without causing harm to normal tissue cells (Chen et al., 2021a). O2 is a crucial component of PDT (Agostinis et al., 2011). Ding et al. genetically modified Ec N to express peroxidase and created an engineered E. coli/BPQDs (EB) system by attaching black phosphorus quantum dots (BPQDs) to the bacterial layer through electrostatic attraction. This system was capable of targeting hypoxic tissues after intravenous injection and, upon irradiation with a 660 nm laser, generating reactive oxygen species (ROS) and disrupting cell membranes. Consequently, peroxidase was released, degrading hydrogen peroxide to produce O2 and enhancing PDT (Ding et al., 2021). It is not difficult to find that when antitumor therapy using Ec N as a drug carrier is combined with other antitumor therapies, it can produce synergistic and surprising therapeutic effects.
2.1.2 E. coli MG1655
The nonpathogenic MG1655 naturally reduces nontoxic nitrate to nitrous oxide with antitumor activity through nitrate/nitrite reductase (Gusarov et al., 2009; Zheng et al., 2018). It is frequently utilized in antitumor therapy as an engineered bacterium, combined with other therapeutic approaches such as immunotherapy and photothermal therapy (PTT). Chen et al. engineered MG1655 to express tumor necrosis factor and bind it to black phosphorus NPs. This binding facilitates the transfer of photoelectrons generated by laser irradiation of black phosphorus NPs, triggering the metabolism of nitrate reduction to nitric oxide for in-situ release at the tumor site. This process promotes therapeutic efficacy and the polarization of M2-type macrophages to M1-type macrophages. Simultaneously, the immune system enhances the immune effect by i) promoting apoptosis of tumor cells, ii) activating the action of T lymphocytes, and iii) promoting the release of pro-inflammatory factors (Chen et al., 2023). Wang et al. biomineralized gold NPs in MG1655 and constructed a thermally activated biohybrid system (TAB@Au) by exploiting the bacteria’s ability to express the therapeutic protein cytolysin A (Cly A) (Wai et al., 2003; Wang et al., 2021). After laser irradiation of the gold NPs, the collected photons are converted into heat, and the inserted promoter is used to induce the expression of Cly A, thereby enhancing the PTT and inhibiting the proliferation of tumor cells (Wai et al., 2003; Wang et al., 2021). Wei et al. prepared Riquimod (R848) and DOX NPs, which attached E. coli to R848 through electrostatic action to form R848-loaded E. coli. This system targets the hypoxic environment of tumors, and the E. coli is phagocytosed by M2-type macrophages and releases R848, polarizing the M2-type macrophages to M1-type macrophages. This process enhances the secretion of cytokines such as IL-6, promotes anti-tumor immune response, remodels the immunosuppressed TME, and kills tumor cells. DOX NPs can trigger immunogenic cell death, weakening the immunosuppression of the TME and promoting immunotherapy (Wei et al., 2021).
E. coli MG1655, an engineered bacterium, can be utilized for precise diagnosis and prediction of treatment response. According to Yun et al., optoacoustic images and tyrosinase-expressing E. coli were employed to enhance the visualization of cancer-targeting bacteria, enabling accurate diagnosis and prediction of treatment response in colon cancer cases (Yun et al., 2021).
2.2 Salmonella as drug delivery vehicles
Salmonella typhimurium, a facultative anaerobe, is capable of surviving in both aerobic and anaerobic environments. It has the ability to target and colonize both non-hypoxic and hypoxic tumors (Nguyen and Min, 2017). It replicates preferentially in tumor areas, with a ratio of up to 1000:1 or even 10,000:1 compared to normal tissue (Eisenstark, 2018). Based on these properties, S. typhimurium has been designed and engineered as a targeted treatment for cancer. It can be combined with other therapies, such as chemotherapy and radiotherapy, to enhance their effects, synergistically modulating the TME and offering significant diagnostic and therapeutic advantages (Mi et al., 2019). S. typhimurium can be modified to transport antitumor substances and induce tumor regression (Zhao et al., 2005; Zhao et al., 2006). This section focused on the experimental progress of Salmonella typhimurium as a vector for tumor treatment and diagnosis.
The VNP20009 gene modification strain (VNP) can be enriched in tumors due to its extremely tumor-targeting properties and stable heritability (Liu et al., 2023). Tumor necrosis factor α (TNF-α) is a tumor necrosis factor that can cause tumor necrosis with high doses and repeated local injections (Balkwill, 2009). However, the significant toxicity to humans limits the use of TNF-α. Liu et al. constructed a new VNP delivery system that expresses anti-TNF-α nanoantibodies, which can dramatically increase delivery efficiency by continuously releasing nanoantibodies in a hypoxic tumor environment. They combined the delivery system with cherry to visualize tumor-expressed TNF-α nb. It was demonstrated that the system showed sound therapeutic effects in melanoma mice, which may be related to the fact that VNP α TNF-α inhibited tumor progression by reducing tumor vascular density, inducing more apoptosis in tumor tissues, and activating the immune system (Liu et al., 2023). Tan et al. engineered S. typhimurium to express Cly A by genetic modification for treating mouse pancreatic cancer. It was demonstrated that after intravenous injection, the engineered bacteria could colonize the TME, significantly reduce the expression of tumor mesenchymal cell markers, increase immune cells, and destroy tumor stromal cells and cancer cells, thereby significantly promoting the secretion of inflammatory cytokines, such as interleukin (IL-1b) and TNF-α, and inhibiting tumor growth (Tan et al., 2022). Chen et al. developed indocyanine green (ICG)-loaded NPs (INPs) as nano photosensitizers for fluorescence-guided PTT of tumors, followed by attaching INPs to S. typhimurium YB1 and delivering them to the oxygen-deficient tumor cores to kill tumor cells with the photothermal effect of the INPs (Figure 4) (Chen et al., 2019). INPs are highly biocompatible, have high spatiotemporal selectivity, and excellent photothermal conversion efficiency (Zheng et al., 2013; Chen et al., 2016; Chen et al., 2019). Under NIR laser irradiation, the temperature of the tumor region can reach up to 63 °C, killing both the tumor cells and the YB1 strain colonized within the tumor (Chen et al., 2019).
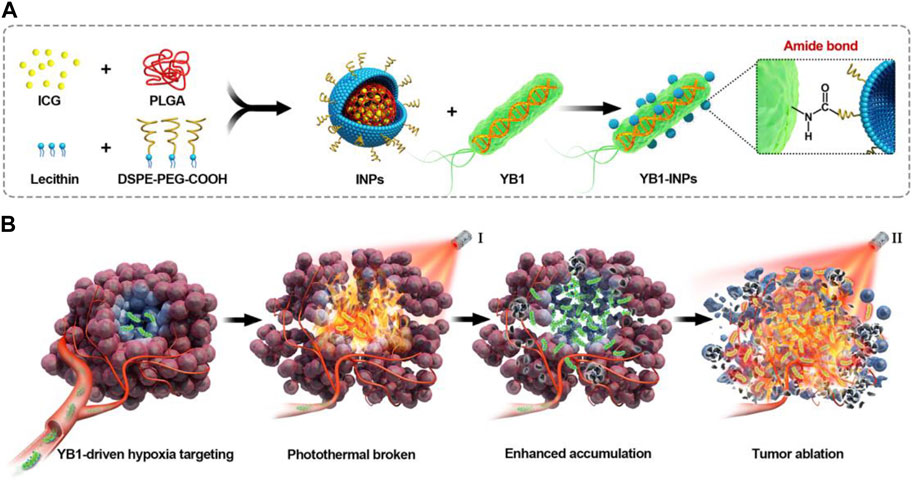
FIGURE 4. Schematic illustration of nanophotosensitizers (INPs)-attached YB1 as a hypoxia-targeting delivery system for large solid tumors photothermal therapy. (A) Preparation procedure of YB1-INPs. Synthesized INPs with single-step sonication were attached to YB1 through amide bonds. (B) YB1-INPs with hypoxia-targeting and photothermal-assisted bioaccumulation for tumor penetrative therapy. After migrating into tumor hypoxic cores and subsequently irradiating with NIR laser, the loosening of tumor tissue and tumor lysis generate bacteria-attracting nutrients, which further enhances the accumulation and coverage of YB1-INPs in large solid tumors. Ultimately, the enriched YB1-INPs under NIR laser irradiation completely ablated the large solid tumor without relapse. Reproduced with permission from (Chen et al., 2019). © 2019 Elsevier Ltd. All rights reserved.
Attenuated Salmonella can also be utilized for delivering antitumor antigens. In the experiments conducted by Chen et al., they constructed a plasmid (pcDNA3.1-HPV16-L1) and a co-expression plasmid carried by Salmonella attenuata (pcDNA3.1-HPV16-L1-siE6). The Salmonella carrying pcDNA3.1-HPV16-L1 can generate HPV16-L1 antibodies INF-γ and IL-2 in the serum of mice, which have a preventive effect against HPV. Furthermore, pCG-siE6 suppressed the expression of E7 and E6 genes, leading to the inhibition of cervical cancer growth both in vitro and vivo (Chen et al., 2021b). Maria V. Stegantseva et al. examined the delivery method of DNA vaccines for neuroblastoma and discovered that trans-Salmonella delivery of DNA vaccines elicited a stronger immune response (Stegantseva et al., 2020).
Resistance to chemotherapeutic agents often leads to poor tumor treatment or tumor recurrence (Zahan et al., 2020). One resistance mechanism that is strongly linked to modifying genes and proteins regulating the apoptotic mitochondrial pathway is members in the Bcl-2 family (Stavrovskaya, 2000; Johnstone et al., 2002; Mateos-Chávez et al., 2019). The BH3 structural domain peptide from the proapoptotic protein Bax has the potential to oppose the anti-apoptotic activities of proteins in the Bcl-2 family, thereby recovering apoptosis and inducing chemo-sensitivity in tumor cells (Moreau et al., 2003; Mateos-Chávez et al., 2019). In a study by Alfredo Mateos-Chávez et al., the Bax BH3 polypeptide was expressed and released using the Mis L self-transport system on the surface of S. typhimurium SL326183 (Mateos-Chávez et al., 2019). The results showed that Salmonella L-STXP significantly reduced the viability and induced apoptosis in the human B-type non-Hodgkin’s lymphomas (NHL) cell line Ramos cells. These findings have positive implications for the remission of non-Hodgkin’s lymphoma and the reduction of drug resistance (Mateos-Chávez et al., 2019).
2.3 Listeria monocytogenes as drug delivery vehicles
Unlike E. coli and Salmonella, Listeria monocytogenes (LM) is a Gram-positive bacterium with a unique life cycle (Oladejo et al., 2021). A portion of these LM escapes from primary phagocytic vesicles, grows and divides macrophages in the cytoplasm, provides cell-penetration drive via host cell actin and filamentous pseudopod protrusions, and repeats the cycle of growth, actin recruitment, and cell-to-cell spread (Goldfine and Wadsworth, 2002). Infected LM and its progeny can bypass an organism’s humoral immune system (Brunt et al., 1990). The cell-mediated immunity of LM is associated with CD4+ and CD8+ T-cell responses, and it is this feature that makes LM ideally suited to serve as a platform for antigen delivery (Goldfine and Wadsworth, 2002; Oladejo et al., 2021). This section presented recent experimental advances in LM as an antitumor delivery platform.
Pancreatic tumors are located in a relatively hidden manner, and early symptoms are not easily detectable. However, these tumors have a high metastasis rate and often result in a poor prognosis. Therefore, there is an urgent need to develop methods for effective preventive control. In a study by Benso et al., immunogenic tetanus toxoid proteins (TT856-1313) were selectively delivered into pancreatic ductal tumor cells using attenuated LM. This led to the accumulation of tetanus toxoid proteins within the tumor cells, introduction of tetanus toxoid protein CD4 T cells into the TME, and production of anti-tumor substances. As a result, tumor load and metastatic foci were effectively reduced, and survival rates in mice increased (Selvanesan et al., 2022). Another study by Victoria M. Kim et al. focused on LM immunotherapy based on ANXA2 expression (Lm-ANXA2). It was discovered that Lm-ANXA2 significantly prolonged survival in a mouse model of PDACs. Furthermore, sequential treatment with Lm-ANXA2 and anti-PD-1 increased interferon-γ (INFγ) expression in the TME and promoted ANXA2 epitope formation. These findings suggest that LM has the potential to serve as a vector in the treatment of pancreatic tumors (Kim et al., 2019). In addition to pancreatic tumors, some researchers have identified a potential role for immunotherapy in the treatment of cervical cancer. Su et al. genetically modified LM by deleting the actA and plcB genes or recombining shuffled HPV-16 E6E7 protein expression. They then delivered these modified strains to the spleen and liver through intravenous injection, inducing antigen-specific cellular immunity. The study experimentally demonstrated the effectiveness of both genetically modified strains against cervical cancer. It was found that combined immunotherapy was more effective than single-strain immunotherapy (Su et al., 2021).
2.4 Bifidobacterium bifidum as drug delivery vehicles
B. bifidum is a Gram-positive bacterium that is well-known to humans and has been used as a probiotic in the treatment of various diseases (Levit et al., 2022; Cao et al., 2023). Similar to the aforementioned bacteria, Bifidobacteria also have the ability to specifically target tumors and colonize and replicate within them, highlighting their potential as a delivery platform for antitumor therapy (Lu et al., 2022). Furthermore, engineered bifidobacteria have distinct advantages in aiding tumor therapy diagnostics. For instance, Sheethal Reghu et al. utilized bifidobacteria encapsulated with indocyanine green (ICG) nanoparticles to develop light-inducing functional bacteria for cancer photothermal therapy (Reghu and Miyako, 2022). In another study, Li et al. incorporated Ce6 nanoparticles into B. bifidum and attached an anti-death receptor five antibody to the bacterium’s surface, resulting in significant inhibition of tumor growth through laser and ultrasound irradiation (Figure 5) (Li et al., 2022). Due to its probiotic properties, B. bifidum exhibits good biocompatibility and significantly reduces side effects when used as a delivery platform in vivo, particularly for assisting photothermal and laser therapies. Apart from the synergistic effects of photothermal and laser therapies, engineered bifidobacteria can also be combined with immunotherapy. He et al. targeted the hypoxic environment of tumors by covalently attaching DOX-loaded CaP/SiO2 nanoparticles (DNPs) to bifidobacteria, leading to DOX chemotherapy and simultaneous induction of immunogenic cell death (ICD), which effectively suppressed metastasis of murine melanomas while controlling the primary tumors (He et al., 2023).
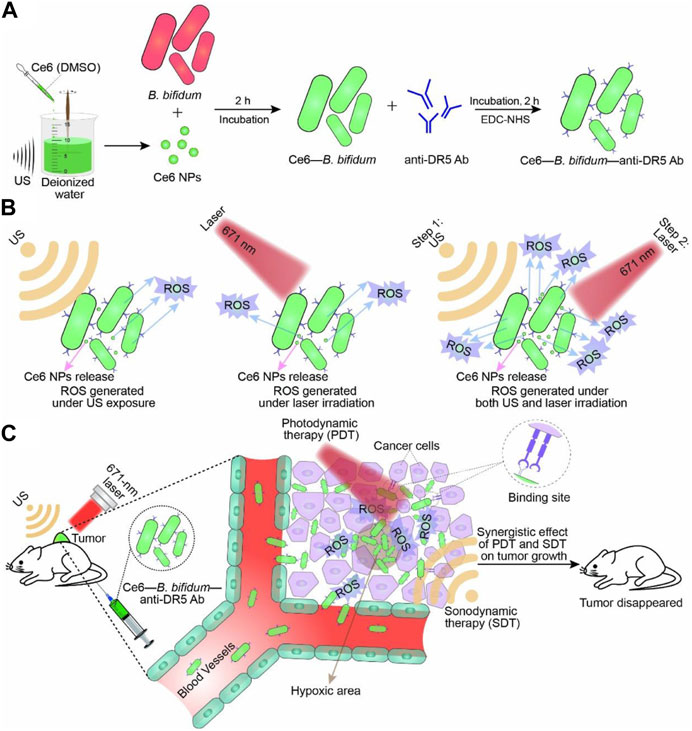
FIGURE 5. Schematic illustration of Ce6–B. bifidum–anti-DR5 Ab for tumor-targeted PDT and SDT. (A) Preparation of Ce6 NPs and Ce6–B. bifidum–anti-DR5 Ab. (B) Combination of laser and US exposure exerted a synergistic effect on ROS generation of Ce6–B. bifidum–anti-DR5 Ab. (C) Combined 671 nm laser and US targeted therapy. Reproduced with permission from (Li et al., 2022). © 2022 Acta Materialia Inc. Published by Elsevier Ltd. All rights reserved.
2.5 Akkermansia muciniphila as drug delivery vehicles
A. muciniphila is a typical human gut bacterium. A. muciniphila is negatively associated with obesity, diabetes, cardiovascular disease, and low-grade inflammation (Plovier et al., 2017; Depommier et al., 2019; Yang et al., 2019; Yoon et al., 2021). In recent years, it has been discovered that various bacteria are associated with the development and treatment of CRC (Zheng et al., 2019; Jiang et al., 2023; Kang et al., 2023). A. muciniphila is considered one of the most promising bacteria for suppressing tumors in CRC due to its multiple mechanisms of colorectal cancer inhibition. It inhibits colorectal tumorigenesis through three main mechanisms: i) induction of TLR2/NLRP3-mediated M1-like TAMs, ii) reprogramming of TME, and iii) purification of membrane protein-regulated CD8 T cells (Shi et al., 2020; Fan et al., 2021; Luo et al., 2021; Jiang et al., 2023). Moreover, changes in the abundance of A. muciniphila in the intestinal tract can help predict clinical responses to immune checkpoint inhibitors in non-small cell lung cancer and advanced melanoma (Derosa et al., 2022; Lee et al., 2022). In conclusion, A. muciniphila is an excellent bacterium with anti-tumor properties.
3 OMVs based drug delivery system
OMVs are active substances produced by Gram-negative bacteria in response to external stimuli (Toyofuku et al., 2019; Dell’Annunziata et al., 2021). These vesicles are mainly rich in lipopolysaccharides (LPS), phospholipids, outer membrane, and periplasmic proteins, which play a crucial role in bacterial survival and invasion (Kaparakis-Liaskos and Ferrero, 2015; Kim et al., 2015; Gerritzen et al., 2017; Wang et al., 2022). The formation of OMVs can be regulated by increasing the accumulation of relevant components on the outer membrane, altering the fluidity of the bacterial membrane, decreasing the cross-linking of peptidoglycan, and other destabilizing factors of the outer membrane (Figure 6) (Xie et al., 2022; Long et al., 2022). OMVs, when modified by tumor antigens, have been shown to induce antigenic specific humoral and cellular immunity in mice, exerting a strong inhibitory effect on tumors. Additionally, encapsulated OMVs contain virulence factors that can be delivered to host cells, stimulating bacterial-host cell interactions with intrinsic antitumor activity. This is possible due to their powerful pathogen-associated molecular patterns (PAMPs), including LPS and lipoproteins, which can be efficiently recognized and internalized by immune cells (Chen et al., 2022). Bacterial outer membrane vesicles hold promise as lipid-like bilayer nanocarriers due to their low toxicity, drug-carrying capacity, and ease of modification and industrialization (Sartorio et al., 2021). In this section, we focused on experimental advances using OMVs as a vehicle to aid in tumor therapy and diagnosis.
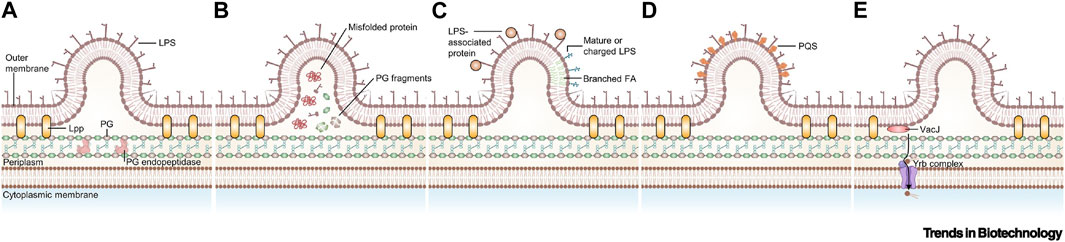
FIGURE 6. Currently proposed models for the biogenesis of outer membrane vesicles (OMVs). (A) Disruption of peptidoglycan–lipoprotein crosslinks (Toyofuku et al., 2017). (B) Accumulation of envelope component (Schwechheimer et al., 2014). (C) Enrichment of specific LPS in some area (Chowdhury and Jagannadham, 2013). (D) Insertion of the Pseudomonas quinolone signal (Tashiro et al., 2010). (E) Downregulation of VacJ/Yrb ABC transporter (Zingl et al., 2020). Reproduced with permission from (Xie et al., 2022). © 2022 Published by Elsevier Ltd. Abbreviations: FA, fatty acid; Lpp, lipoprotein; PG, peptidoglycan; PQS, Pseudomonas quinolone signal.
3.1 OMVs as delivery platform combined with antitumor therapy
OMVs, which are spherical NPs derived from bacteria, are highly biocompatible and ideally suited in morphology and size for carrying antitumor drug delivery in synergistic tumor diagnostics and therapy (Feng et al., 2022). Liu et al. utilized OMVs derived from E. coli to construct functionalized Fe3O4-MnO2 (FMO) nanoplatforms, enabling neutrophil-mediated targeted transport and photothermal enhancement of cancer immunotherapy, thereby enhancing the accumulation of FMO NPs in tumor tissues. FMO NPs undergo reactive breakdown at the tumor site to produce Mn and iron ions, induce ICD and O2, and regulate the hypoxic environment of the tumor. Additionally, PTT and Fe3O4 induced by MnO can directly destroy tumor cells, stimulate systemic immune responses, and promote nanoparticle enrichment of neutrophil-prepared NPs by augmenting the inflammation response at the tumor site, thus enabling immunotherapy of tumors (Liu et al., 2023). Li, Wu et al. introduced Ce6 and adriamycin together into OMVs extracted using E. coli. Ce6 acted as a photosensitizer to potentiate PDT, DOX served as a chemotherapeutic agent to kill tumor cells, and OMVs enhanced anti-tumor immunity. Together, these three components were used to eradicate triple-negative breast cancer in mice without side effects and prevent its metastasis to the lung (Li et al., 2023). Shi et al. loaded 5-FU into OMVs. They utilized OMVs modified nanocarriers mesoporous silica, combining the advantages of intestinal absorption of OMVs and the high drug loading capacity of MSNs to release 5-FU after adsorption of OMVs on the intestinal surface. This targeted delivery of 5-FU reduced the side effects of liver and spleen damage associated with conventional drug administration (Shi et al., 2020). Li et al. prepared genetically engineered OMVs inserted into the extracellular domain of programmed death protein 1 (PD1). These OMVs bind to PD-L1 on the surface of tumor cells and protect T-cells from immunosuppression caused by PD1/PD-L1, thereby enhancing the immune response (Li et al., 2020).
3.2 OMVs converted to a tumor vaccine
OMVs contain PAMPs that strongly stimulate the natural immune system and are often used in the preparation of tumor vaccines as immune adjuvants. In a study by Yue et al., tumor antigens were loaded along with mouse IgG fragments into the Cly A of OMVs. Subsequently, an arabinose-inducible promoter was introduced to control the expression of the fusion protein. The controlled manufacture of OMVs containing tumor antigens was achieved by orally administering modified bacteria and an expression elicitor, arabinose. It was demonstrated that this method caused significant tumor suppression in various mouse tumor models (Yue et al., 2022). In another study by Li et al., OMVs were utilized as a platform for mRNA transfer. This was achieved by modifying the RNA-binding protein L7Ae and the lysosomal escape protein Listeriolysin O (OMV-LL). The OMV-LL was then used to adsorb C/D sequence-labeled mRNA antigen (OMV-LL-mRNA), which was subsequently delivered into DCs. The mRNA antigen was presented by Listeria lysozyme O-mediated escape in vivo. The experimental results showed that OMV-LL-mRNA could induce long-term immune memory and protect mice from tumors after 2 months. Moreover, it caused 37.5% complete regression in a colon cancer model and significantly inhibited melanoma development (Li et al., 2022). In a study by Cheng et al., an OMV-based vaccine platform was constructed by fusing antigens with Cly A proteins to the surface of OMVs. This simplified the antigen presentation process through a “plug-and-play” approach. Multiple protein capture agents were used to modify OMVs, enabling the display of multiple different tumor antigens. This induced a synergistic anti-tumor immune response. The OMVs obtained in this manner not only eliminated melanoma lung metastases but also inhibited the growth of subcutaneous colorectal cancer (Cheng et al., 2021).
4 Conclusions and future prospects
The above findings suggest that E. coli, Salmonella, LM, B. bifidum, and A. muciniphila specifically target hypoxic tumors. Moreover, their derivative OMV significantly enhances innate immunity, serving as a potential means for drug delivery and improving the effectiveness of other therapies. Furthermore, they can be utilized as delivery platforms for tumor vaccines. Thus, adjuvant bacteria and their derivatives are highly regarded as excellent carrier platforms for antitumor drug delivery and synergistic antitumor therapy. They have become an indispensable weapon in the fight against tumors. Nevertheless, there are several issues that require attention. Firstly, it is important to consider the toxicity of drug-carrying bacteria, particularly as patients undergoing radiotherapy often have a weakened immune system. If the toxicity of the bacteria is excessive, it could overwhelm the immune system, increasing the risk of bacterial infection. To mitigate this risk and prevent adverse events, it is crucial to minimize the toxicity of the bacteria used as carriers. Secondly, to enhance the bacterial loading rate of the drug, smaller doses of bacteria can be administered to deliver a greater quantity of drugs to the targeted area, thereby reducing the dependence on high bacterial doses. Lastly, the yield of OMV is currently limited, and various issues need to be addressed to increase its production and enhance its purification. However, with the continuous advancement of modern molecular biotechnology, these challenges will be tackled, bringing renewed hope for antitumor therapy.
Author contributions
DS: Writing–original draft, Writing–review and editing. XY: Writing–original draft, Writing–review and editing. YC: Writing–original draft, Writing–review and editing. PH: Writing–review and editing. YiZ: Writing–review and editing. YaZ: Writing–review and editing. NL: Writing–review and editing. JX: Writing–review and editing. LQ: Writing–review and editing. GD: Writing–review and editing. FC: Conceptualization, Data curation, Formal Analysis, Funding acquisition, Investigation, Methodology, Resources, Supervision, Validation, Visualization, Writing–original draft, Writing–review & editing. JZ: Conceptualization, Data curation, Formal Analysis, Funding acquisition, Investigation, Methodology, Resources, Supervision, Validation, Visualization, Writing–original draft, Writing–review & editing.
Funding
The author(s) declare financial support was received for the research, authorship, and of this article. This work was financially supported by the Shandong Provincial Natural Science Foundation (ZR2022QH248, ZR2021LSW023), the Cultivating Fund of National Natural Science Foundation From Shandong First Medical University (202201-074).
Conflict of interest
The authors declare that the research was conducted in the absence of any commercial or financial relationships that could be construed as a potential conflict of interest.
Publisher’s note
All claims expressed in this article are solely those of the authors and do not necessarily represent those of their affiliated organizations, or those of the publisher, the editors and the reviewers. Any product that may be evaluated in this article, or claim that may be made by its manufacturer, is not guaranteed or endorsed by the publisher.
References
Aganja, R. P., Sivasankar, C., Senevirathne, A., and Lee, J. H. (2022). Salmonella as a promising curative tool against cancer. Pharmaceutics 14, 2100. doi:10.3390/pharmaceutics14102100
Agostinis, P., Berg, K., Cengel, K. A., Foster, T. H., Girotti, A. W., Gollnick, S. O., et al. (2011). Photodynamic therapy of cancer: an update. CA. Cancer J. Clin. 61, 250–281. doi:10.3322/caac.20114
Balkwill, F. (2009). Tumour necrosis factor and cancer. Nat. Rev. Cancer 9, 361–371. doi:10.1038/nrc2628
Brunt, L. M., Portnoy, D. A., and Unanue, E. R. (1990). Presentation of Listeria monocytogenes to CD8+ T cells requires secretion of hemolysin and intracellular bacterial growth. J. Immunol. 145, 3540–3546. doi:10.4049/jimmunol.145.11.3540
Cacicedo, M. L., Islan, G. A., León, I. E., Álvarez, V. A., Chourpa, I., Allard-Vannier, E., et al. (2018). Bacterial cellulose hydrogel loaded with lipid nanoparticles for localized cancer treatment. Colloids Surf. B Biointerfaces 170, 596–608. doi:10.1016/j.colsurfb.2018.06.056
Cao, F., Jin, L., Gao, Y., Ding, Y., Wen, H., Qian, Z., et al. (2023). Artificial-enzymes-armed Bifidobacterium longum probiotics for alleviating intestinal inflammation and microbiota dysbiosis. Nat. Nanotechnol. 18, 617–627. doi:10.1038/s41565-023-01346-x
Cao, Z., and Liu, J. (2020). Bacteria and bacterial derivatives as drug carriers for cancer therapy. J. Control. Release 326, 396–407. doi:10.1016/j.jconrel.2020.07.009
Chen, B., Zhang, X., Cheng, L., Chen, X., Tang, J., Zhang, P., et al. (2023a). Surface programmed bacteria as photo-controlled NO generator for tumor immunological and gas therapy. J. Control Release 353, 889–902. doi:10.1016/j.jconrel.2022.12.030
Chen, F., Zang, Z., Chen, Z., Cui, L., Chang, Z., Ma, A., et al. (2019). Nanophotosensitizer-engineered Salmonella bacteria with hypoxia targeting and photothermal-assisted mutual bioaccumulation for solid tumor therapy. Biomaterials 214, 119226. doi:10.1016/j.biomaterials.2019.119226
Chen, H., Lei, P., Ji, H., Yang, Q., Peng, B., Ma, J., et al. (2023b). Advances in Escherichia coli Nissle 1917 as a customizable drug delivery system for disease treatment and diagnosis strategies. Mater Today Bio 18, 100543. doi:10.1016/j.mtbio.2023.100543
Chen, H., Zhou, M., Zeng, Y., Lv, Z., Wang, P., and Han, L. (2022). Recent advances in biomedical applications of bacterial outer membrane vesicles. J. Mater Chem. B 10, 7384–7396. doi:10.1039/d2tb00683a
Chen, J., Li, X., Liu, Y., Su, T., Lin, C., Shao, L., et al. (2021a). Engineering a probiotic strain of Escherichia coli to induce the regression of colorectal cancer through production of 5-aminolevulinic acid. Microb. Biotechnol. 14, 2130–2139. doi:10.1111/1751-7915.13894
Chen, J., Zhao, S., Tan, W., Wang, T., Wu, S., Wang, C., et al. (2021b). Attenuated Salmonella carrying plasmid co-expressing HPV16 L1 and siRNA-E6 for cervical cancer therapy. Sci. Rep. 11, 20083. doi:10.1038/s41598-021-99425-3
Chen, Z., Zhao, P., Luo, Z., Zheng, M., Tian, H., Gong, P., et al. (2016). Cancer cell membrane-biomimetic nanoparticles for homologous-targeting dual-modal imaging and photothermal therapy. ACS Nano 10, 10049–10057. doi:10.1021/acsnano.6b04695
Cheng, K., Zhao, R., Li, Y., Qi, Y., Wang, Y., Zhang, Y., et al. (2021). Bioengineered bacteria-derived outer membrane vesicles as a versatile antigen display platform for tumor vaccination via Plug-and-Display technology. Nat. Commun. 12, 2041. doi:10.1038/s41467-021-22308-8
Chiang, C. J., and Hong, Y. H. (2021). In situ delivery of biobutyrate by probiotic Escherichia coli for cancer therapy. Sci. Rep. 11, 18172. doi:10.1038/s41598-021-97457-3
Chiang, C. J., and Huang, P. H. (2021). Metabolic engineering of probiotic Escherichia coli for cytolytic therapy of tumors. Sci. Rep. 11, 5853. doi:10.1038/s41598-021-85372-6
Chowdhury, C., and Jagannadham, M. V. (2013). Virulence factors are released in association with outer membrane vesicles of Pseudomonas syringae pv. tomato T1 during normal growth. Biochimica Biophysica Acta (BBA) - Proteins Proteomics 1834, 231–239. doi:10.1016/j.bbapap.2012.09.015
Chowdhury, S., Castro, S., Coker, C., Hinchliffe, T. E., Arpaia, N., and Danino, T. (2019). Programmable bacteria induce durable tumor regression and systemic antitumor immunity. Nat. Med. 25, 1057–1063. doi:10.1038/s41591-019-0498-z
Dai, T., Ye, F., Hu, P., Pan, X., Chen, J., Huang, Y., et al. (2020). A strategy for enhanced tumor targeting of photodynamic therapy based on Escherichia coli-driven drug delivery system. Sci. China Mater. 64, 232–240. doi:10.1007/s40843-020-1363-2
Deepak, K. G. K., Vempati, R., Nagaraju, G. P., Dasari, V. R., S, N., Rao, D. N., et al. (2020). Tumor microenvironment: challenges and opportunities in targeting metastasis of triple negative breast cancer. Pharmacol. Res. 153, 104683. doi:10.1016/j.phrs.2020.104683
Dell’Annunziata, F., Folliero, V., Giugliano, R., De Filippis, A., Santarcangelo, C., Izzo, V., et al. (2021). Gene transfer potential of outer membrane vesicles of gram-negative bacteria. Int. J. Mol. Sci. 22, 5985. doi:10.3390/ijms22115985
Deng, X., Yang, W., Shao, Z., and Zhao, Y. (2021). Genetically modified bacteria for targeted phototherapy of tumor. Biomaterials 272, 120809. doi:10.1016/j.biomaterials.2021.120809
Depommier, C., Everard, A., Druart, C., Plovier, H., Van Hul, M., Vieira-Silva, S., et al. (2019). Supplementation with akkermansia muciniphila in overweight and obese human volunteers: A proof-of-concept exploratory study. Nat. Med. 25, 1096–1103. doi:10.1038/s41591-019-0495-2
Derosa, L., Routy, B., Thomas, A. M., Iebba, V., Zalcman, G., Friard, S., et al. (2022). Intestinal Akkermansia muciniphila predicts clinical response to PD-1 blockade in patients with advanced non-small-cell lung cancer. Nat. Med. 28, 315–324. doi:10.1038/s41591-021-01655-5
Ding, S., Liu, Z., Huang, C., Zeng, N., Jiang, W., and Li, Q. (2021). Novel engineered bacterium/black phosphorus quantum dot hybrid system for hypoxic tumor targeting and efficient photodynamic therapy. ACS Appl. Mater Interfaces 13, 10564–10573. doi:10.1021/acsami.0c20254
Dróżdż, M., Makuch, S., Cieniuch, G., Woźniak, M., and Ziółkowski, P. (2020). Obligate and facultative anaerobic bacteria in targeted cancer therapy: current strategies and clinical applications. Life Sci. 261, 118296. doi:10.1016/j.lfs.2020.118296
Eisenstark, A. (2018). A geneticist’s view of prostate cancer: prostate cancer treatment considerations. Adv. Exp. Med. Biol. 1095, 125–129. doi:10.1007/978-3-319-95693-0_8
Eulberg, D., Frömming, A., Lapid, K., Mangasarian, A., and Barak, A. (2022). The prospect of tumor microenvironment-modulating therapeutical strategies. Front. Oncol. 12, 1070243. doi:10.3389/fonc.2022.1070243
Fan, L., Xu, C., Ge, Q., Lin, Y., Wong, C. C., Qi, Y., et al. (2021). A. Muciniphila suppresses colorectal tumorigenesis by inducing TLR2/NLRP3-mediated M1-like TAMs. Cancer Immunol. Res. 9, 1111–1124. doi:10.1158/2326-6066.CIR-20-1019
Feng, Q., Ma, X., Cheng, K., Liu, G., Li, Y., Yue, Y., et al. (2022). Engineered bacterial outer membrane vesicles as controllable two-way adaptors to activate macrophage phagocytosis for improved tumor immunotherapy. Adv. Mater 34, e2206200. doi:10.1002/adma.202206200
Fu, S., Zhang, R., Gao, Y., Xiong, J., Li, Y., Pu, L., et al. (2023). Programming the lifestyles of engineered bacteria for cancer therapy. Natl. Sci. Rev. 10, nwad031. doi:10.1093/nsr/nwad031
Fukuhara, H., Ino, Y., and Todo, T. (2016). Oncolytic virus therapy: A new era of cancer treatment at dawn. Cancer Sci. 107, 1373–1379. doi:10.1111/cas.13027
Gerritzen, M. J. H., Martens, D. E., Wijffels, R. H., van der Pol, L., and Stork, M. (2017). Bioengineering bacterial outer membrane vesicles as vaccine platform. Biotechnol. Adv. 35, 565–574. doi:10.1016/j.biotechadv.2017.05.003
Goldfine, H., and Wadsworth, S. J. (2002). Macrophage intracellular signaling induced by Listeria monocytogenes. Microbes Infect. 4, 1335–1343. doi:10.1016/s1286-4579(02)00011-4
Gusarov, I., Shatalin, K., Starodubtseva, M., and Nudler, E. (2009). Endogenous nitric oxide protects bacteria against a wide spectrum of antibiotics. Science 325, 1380–1384. doi:10.1126/science.1175439
Hajjar, R., Richard, C. S., and Santos, M. M. (2021). The role of butyrate in surgical and oncological outcomes in colorectal cancer. Am. J. Physiol. Gastrointest. Liver Physiol. 320, G601–G608. doi:10.1152/ajpgi.00316.2020
Harrington, K., Freeman, D. J., Kelly, B., Harper, J., and Soria, J. C. (2019). Optimizing oncolytic virotherapy in cancer treatment. Nat. Rev. Drug Discov. 18, 689–706. doi:10.1038/s41573-019-0029-0
He, L., Yang, H., Tang, J., Liu, Z., Chen, Y., Lu, B., et al. (2019). Intestinal probiotics E. coli Nissle 1917 as a targeted vehicle for delivery of p53 and Tum-5 to solid tumors for cancer therapy. J. Biol. Eng. 13, 58. doi:10.1186/s13036-019-0189-9
He, T., Wang, L., Gou, S., Lu, L., Liu, G., Wang, K., et al. (2023a). Enhanced immunogenic cell death and antigen presentation via engineered bifidobacterium bifidum to boost chemo-immunotherapy. ACS Nano 17, 9953–9971. doi:10.1021/acsnano.2c11474
He, Z., Chen, K., An, Y., He, J., Zhang, X., Tang, L., et al. (2023b). BSA modification of bacterial surface: A promising anti-cancer therapeutic strategy. BMC Microbiol. 23, 105. doi:10.1186/s12866-023-02830-z
Holay, M., Guo, Z., Pihl, J., Heo, J., Park, J. H., Fang, R. H., et al. (2021). Bacteria-inspired nanomedicine. ACS Appl. Bio Mat. 4, 3830–3848. doi:10.1021/acsabm.0c01072
Hou, W., Li, J., Cao, Z., Lin, S., Pan, C., Pang, Y., et al. (2021). Decorating bacteria with a therapeutic nanocoating for synergistically enhanced biotherapy. Small 17, e2101810. doi:10.1002/smll.202101810
Howell, L. M., and Forbes, N. S. (2022). Bacteria-based immune therapies for cancer treatment. Seminars Cancer Biol. 86, 1163–1178. doi:10.1016/j.semcancer.2021.09.006
Jiang, S. N., Phan, T. X., Nam, T. K., Nguyen, V. H., Kim, H. S., Bom, H. S., et al. (2010). Inhibition of tumor growth and metastasis by a combination of Escherichia coli–mediated cytolytic therapy and radiotherapy. Cell. Ther. 18, 635–642. doi:10.1038/mt.2009.295
Jiang, Y., Xu, Y., Zheng, C., Ye, L., Jiang, P., Malik, S., et al. (2023). Acetyltransferase from Akkermansia muciniphila blunts colorectal tumourigenesis by reprogramming tumour microenvironment. Gut 72, 1308–1318. doi:10.1136/gutjnl-2022-327853
Johnstone, R. W., Ruefli, A. A., and Lowe, S. W. (2002). Apoptosis: A link between cancer genetics and chemotherapy. Cell. 108, 153–164. doi:10.1016/s0092-8674(02)00625-6
Kan, S., Cheung, W. M., Zhou, Y., and Ho, W. S. (2014). Enhancement of doxorubicin cytotoxicity by tanshinone IIA in HepG2 human hepatoma cells. Planta Med. 80, 70–76. doi:10.1055/s-0033-1360126
Kang, X., Ng, S. K., Liu, C., Lin, Y., Zhou, Y., Kwong, T. N. Y., et al. (2023). Altered gut microbiota of obesity subjects promotes colorectal carcinogenesis in mice. EBioMedicine 93, 104670. doi:10.1016/j.ebiom.2023.104670
Kaparakis-Liaskos, M., and Ferrero, R. L. (2015). Immune modulation by bacterial outer membrane vesicles. Nat. Rev. Immunol. 15, 375–387. doi:10.1038/nri3837
Kim, C. H., Chung, C. W., Lee, H. M., Kim, D. H., Kwak, T. W., Jeong, Y. I. L., et al. (2013). Synergistic effects of 5-aminolevulinic acid based photodynamic therapy and celecoxib via oxidative stress in human cholangiocarcinoma cells. Int. J. Nanomedicine 8, 2173–2186. doi:10.2147/IJN.S44394
Kim, J. H., Lee, J., Park, J., and Gho, Y. S. (2015). Gram-negative and Gram-positive bacterial extracellular vesicles. Semin. Cell. Dev. Biol. 40, 97–104. doi:10.1016/j.semcdb.2015.02.006
Kim, V. M., Blair, A. B., Lauer, P., Foley, K., Che, X., Soares, K., et al. (2019). Anti-pancreatic tumor efficacy of a Listeria-based, Annexin A2-targeting immunotherapy in combination with anti-PD-1 antibodies. J. Immunother. cancer 7, 132. doi:10.1186/s40425-019-0601-5
Lee, K. A., Thomas, A. M., Bolte, L. A., Björk, J. R., de Ruijter, L. K., Armanini, F., et al. (2022). Cross-cohort gut microbiome associations with immune checkpoint inhibitor response in advanced melanoma. Nat. Med. 28, 535–544. doi:10.1038/s41591-022-01695-5
Levit, R., Cortes-Perez, N. G., de Moreno de Leblanc, A., Loiseau, J., Aucouturier, A., Langella, P., et al. (2022). Use of genetically modified lactic acid bacteria and bifidobacteria as live delivery vectors for human and animal health. Gut Microbes 14, 2110821. doi:10.1080/19490976.2022.2110821
Li, J., Hou, W., Lin, S., Wang, L., Pan, C., Wu, F., et al. (2022a). Polydopamine nanoparticle-mediated dopaminergic immunoregulation in colitis. Adv. Sci. (Weinh) 9, e2104006. doi:10.1002/advs.202104006
Li, R., Helbig, L., Fu, J., Bian, X., Herrmann, J., Baumann, M., et al. (2019). Expressing cytotoxic compounds in Escherichia coli Nissle 1917 for tumor-targeting therapy. Res. Microbiol. 170, 74–79. doi:10.1016/j.resmic.2018.11.001
Li, W., Zhang, Z., Liu, J., Wang, B., Pu, G., Li, J., et al. (2022b). Nanodrug-loaded Bifidobacterium bifidum conjugated with anti-death receptor antibody for tumor-targeted photodynamic and sonodynamic synergistic therapy. Acta Biomater. 146, 341–356. doi:10.1016/j.actbio.2022.05.016
Li, Y., Ma, X., Yue, Y., Zhang, K., Cheng, K., Feng, Q., et al. (2022c). Rapid surface display of mRNA antigens by bacteria-derived outer membrane vesicles for a personalized tumor vaccine. Adv. Mater 34, e2109984. doi:10.1002/adma.202109984
Li, Y., Wu, J., Qiu, X., Dong, S., He, J., Liu, J., et al. (2023). Bacterial outer membrane vesicles-based therapeutic platform eradicates triple-negative breast tumor by combinational photodynamic/chemo-/immunotherapy. Bioact. Mater 20, 548–560. doi:10.1016/j.bioactmat.2022.05.037
Li, Y., Zhao, R., Cheng, K., Zhang, K., Wang, Y., Zhang, Y., et al. (2020). Bacterial outer membrane vesicles presenting programmed death 1 for improved cancer immunotherapy via immune activation and checkpoint inhibition. ACS Nano 14, 16698–16711. doi:10.1021/acsnano.0c03776
Liang, K., Liu, Q., Li, P., Luo, H., Wang, H., and Kong, Q. (2019). Genetically engineered Salmonella typhimurium: recent advances in cancer therapy. Cancer Lett. 448, 168–181. doi:10.1016/j.canlet.2019.01.037
Liu, L., Liu, X., Xin, W., Zhou, L., Huang, B., Han, C., et al. (2023a). A bacteria-based system expressing anti-TNF-α nanobody for enhanced cancer immunotherapy. Signal Transduct. Target Ther. 8, 134. doi:10.1038/s41392-023-01364-0
Liu, Q., Gai, Y., Chen, Y., Lan, X., and Jiang, D. (2021). Escherichiacoli Nissle 1917 as a novel microrobot for tumor-targeted imaging and therapy. Pharmaceutics 13, 1226. doi:10.3390/pharmaceutics13081226
Liu, X., Pu, Y., Cron, K., Deng, L., Kline, J., Frazier, W. A., et al. (2015). CD47 blockade triggers T cell-mediated destruction of immunogenic tumors. Nat. Med. 21, 1209–1215. doi:10.1038/nm.3931
Liu, X. Z., Wen, Z. J., Li, Y. M., Sun, W. R., Hu, X. Q., Zhu, J. Z., et al. (2023b). Bioengineered bacterial membrane vesicles with multifunctional nanoparticles as a versatile platform for cancer immunotherapy. ACS Appl. Mater Interfaces 15, 3744–3759. doi:10.1021/acsami.2c18244
Long, Q., Zheng, P., Zheng, X., Li, W., Hua, L., Yang, Z., et al. (2022). Engineered bacterial membrane vesicles are promising carriers for vaccine design and tumor immunotherapy. Adv. Drug Deliv. Rev. 186, 114321. doi:10.1016/j.addr.2022.114321
Lou, X., Chen, Z., He, Z., Sun, M., and Sun, J. (2021). Bacteria-mediated synergistic cancer therapy: small microbiome has a big hope. Nano-Micro Lett. 13, 37. doi:10.1007/s40820-020-00560-9
Lu, D., Wang, L., Wang, L., An, L., Huo, M., Xu, H., et al. (2022). Probiotic engineering and targeted sonoimmuno-therapy augmented by STING agonist. Adv. Sci. (Weinh) 9, e2201711. doi:10.1002/advs.202201711
Lugano, R., Ramachandran, M., and Dimberg, A. (2020). Tumor angiogenesis: causes, consequences, challenges and opportunities. Cell. Mol. Life Sci. 77, 1745–1770. doi:10.1007/s00018-019-03351-7
Luo, Z. W., Xia, K., Liu, Y. W., Liu, J. H., Rao, S. S., Hu, X. K., et al. (2021). Extracellular vesicles from akkermansia muciniphila elicit antitumor immunity against prostate cancer via modulation of CD8+ T cells and macrophages. Int. J. Nanomedicine 16, 2949–2963. doi:10.2147/IJN.S304515
Mateos-Chávez, A. A., Muñoz-López, P., Becerra-Báez, E. I., Flores-Martínez, L. F., Prada-Gracia, D., Moreno-Vargas, L. M., et al. (2019). Live attenuated Salmonella enterica expressing and releasing cell-permeable Bax BH3 peptide through the MisL autotransporter system elicits antitumor activity in a murine xenograft model of human B non-hodgkin’s lymphoma. Front. Immunol. 10, 2562. doi:10.3389/fimmu.2019.02562
Mi, Z., Feng, Z. C., Li, C., Yang, X., Ma, M. T., and Rong, P. F. (2019). Salmonella-mediated cancer therapy: an innovative therapeutic strategy. J. Cancer 10, 4765–4776. doi:10.7150/jca.32650
Moreau, C., Cartron, P. F., Hunt, A., Meflah, K., Green, D. R., Evan, G., et al. (2003). Minimal BH3 peptides promote cell death by antagonizing anti-apoptotic proteins. J. Biol. Chem. 278, 19426–19435. doi:10.1074/jbc.M209472200
Narita, K., Staub, J., Chien, J., Meyer, K., Bauer, M., Friedl, A., et al. (2006). HSulf-1 inhibits angiogenesis and tumorigenesis in vivo. Cancer Res. 66, 6025–6032. doi:10.1158/0008-5472.CAN-05-3582
Nguyen, V. H., and Min, J. J. (2017). Salmonella-mediated cancer therapy: roles and potential. Nucl. Med. Mol. Imaging 51, 118–126. doi:10.1007/s13139-016-0415-z
Oladejo, M., Paterson, Y., and Wood, L. M. (2021). Clinical experience and recent advances in the development of listeria-based tumor immunotherapies. Front. Immunol. 12, 642316. doi:10.3389/fimmu.2021.642316
Plovier, H., Everard, A., Druart, C., Depommier, C., Van Hul, M., Geurts, L., et al. (2017). A purified membrane protein from Akkermansia muciniphila or the pasteurized bacterium improves metabolism in obese and diabetic mice. Nat. Med. 23, 107–113. doi:10.1038/nm.4236
Reghu, S., and Miyako, E. (2022). Nanoengineered bifidobacterium bifidum with optical activity for photothermal cancer immunotheranostics. Nano Lett. 22, 1880–1888. doi:10.1021/acs.nanolett.1c04037
Richter, M., Vader, P., and Fuhrmann, G. (2021). Approaches to surface engineering of extracellular vesicles. Adv. Drug Deliv. Rev. 173, 416–426. doi:10.1016/j.addr.2021.03.020
Sartorio, M. G., Pardue, E. J., Feldman, M. F., and Haurat, M. F. (2021). Bacterial outer membrane vesicles: from discovery to applications. Annu. Rev. Microbiol. 75, 609–630. doi:10.1146/annurev-micro-052821-031444
Schwechheimer, C., Kulp, A., and Kuehn, M. J. (2014). Modulation of bacterial outer membrane vesicle production by envelope structure and content. BMC Microbiol. 14, 324. doi:10.1186/s12866-014-0324-1
Selvanesan, B. C., Chandra, D., Quispe-Tintaya, W., Jahangir, A., Patel, A., Meena, K., et al. (2022). Listeria delivers tetanus toxoid protein to pancreatic tumors and induces cancer cell death in mice. Sci. Transl. Med. 14, eabc1600. doi:10.1126/scitranslmed.abc1600
Shi, J., Ma, Z., Pan, H., Liu, Y., Chu, Y., Wang, J., et al. (2020a). Biofilm-encapsulated nano drug delivery system for the treatment of colon cancer. J. Microencapsul. 37, 481–491. doi:10.1080/02652048.2020.1797914
Shi, L., Sheng, J., Chen, G., Zhu, P., Shi, C., Li, B., et al. (2020b). Combining IL-2-based immunotherapy with commensal probiotics produces enhanced antitumor immune response and tumor clearance. J. Immunother. Cancer 8, e000973. doi:10.1136/jitc-2020-000973
Skonieczna, M., Hejmo, T., Poterala-Hejmo, A., Cieslar-Pobuda, A., and Buldak, R. J. (2017). NADPH oxidases: insights into selected functions and mechanisms of action in cancer and stem cells. Oxid. Med. Cell. Longev. 2017, 1–15. doi:10.1155/2017/9420539
Stavrovskaya, A. A. (2000). Cellular mechanisms of multidrug resistance of tumor cells. Biochem. (Mosc) 65, 95–106.
Stegantseva, M. V., Shinkevich, V. A., Tumar, E. M., and Meleshko, A. N. (2020). Multi-antigen DNA vaccine delivered by polyethylenimine and Salmonella enterica in neuroblastoma mouse model. Cancer Immunol. Immunother. 69, 2613–2622. doi:10.1007/s00262-020-02652-2
Su, L., Zhang, Y., Zhang, X., Liu, T., Liu, S., Li, Y., et al. (2021). Combination immunotherapy with two attenuated Listeria strains carrying shuffled HPV-16 E6E7 protein causes tumor regression in a mouse tumor model. Sci. Rep. 11, 13404. doi:10.1038/s41598-021-92875-9
Sun, M., Yang, S., Huang, H., Gao, P., Pan, S., Cheng, Z., et al. (2022). Boarding oncolytic viruses onto tumor-homing bacterium-vessels for augmented cancer immunotherapy. Nano Lett. 22, 5055–5064. doi:10.1021/acs.nanolett.2c00699
Sun, M., Ye, H., Shi, Q., Xie, J., Yu, X., Ling, H., et al. (2021). Both-in-one hybrid bacteria suppress the tumor metastasis and relapse via tandem-amplifying reactive oxygen species-immunity responses. Adv. Healthc. Mater 10, e2100950. doi:10.1002/adhm.202100950
Sung, H., Ferlay, J., Siegel, R. L., Laversanne, M., Soerjomataram, I., Jemal, A., et al. (2021). Global cancer Statistics 2020: GLOBOCAN estimates of incidence and mortality worldwide for 36 cancers in 185 countries. CA Cancer J. Clin. 71, 209–249. doi:10.3322/caac.21660
Tan, W., Duong, M. T. Q., Zuo, C., Qin, Y., Zhang, Y., Guo, Y., et al. (2022). Targeting of pancreatic cancer cells and stromal cells using engineered oncolytic Salmonella typhimurium. Mol. Ther. 30, 662–671. doi:10.1016/j.ymthe.2021.08.023
Tashiro, Y., Ichikawa, S., Nakajima-Kambe, T., Uchiyama, H., and Nomura, N. (2010). Pseudomonas quinolone signal affects membrane vesicle production in not only gram-negative but also gram-positive bacteria. Microbes Environ. 25, 120–125. doi:10.1264/jsme2.me09182
Toyofuku, M., Cárcamo-Oyarce, G., Yamamoto, T., Eisenstein, F., Hsiao, C. C., Kurosawa, M., et al. (2017). Prophage-triggered membrane vesicle formation through peptidoglycan damage in Bacillus subtilis. Nat. Commun. 8, 481. doi:10.1038/s41467-017-00492-w
Toyofuku, M., Nomura, N., and Eberl, L. (2019). Types and origins of bacterial membrane vesicles. Nat. Rev. Microbiol. 17, 13–24. doi:10.1038/s41579-018-0112-2
Ushio-Fukai, M., and Nakamura, Y. (2008). Reactive oxygen species and angiogenesis: NADPH oxidase as target for cancer therapy. Cancer Lett. 266, 37–52. doi:10.1016/j.canlet.2008.02.044
Wai, S. N., Lindmark, B., Söderblom, T., Takade, A., Westermark, M., Oscarsson, J., et al. (2003). Vesicle-mediated export and assembly of pore-forming oligomers of the enterobacterial ClyA cytotoxin. Cell. 115, 25–35. doi:10.1016/s0092-8674(03)00754-2
Waldman, A. D., Fritz, J. M., and Lenardo, M. J. (2020). A guide to cancer immunotherapy: from T cell basic science to clinical practice. Nat. Rev. Immunol. 20, 651–668. doi:10.1038/s41577-020-0306-5
Wang, J. W., Chen, Q. W., Luo, G. F., Ji, P., Han, Z. Y., Song, W. F., et al. (2022b). Interference of glucose bioavailability of tumor by engineered biohybrids for potentiating targeting and uptake of antitumor nanodrugs. Nano Lett. 22, 8735–8743. doi:10.1021/acs.nanolett.2c03608
Wang, J., Guo, N., Hou, W., and Qin, H. (2022a). Coating bacteria for anti-tumor therapy. Front. Bioeng. Biotechnol. 10, 1020020. doi:10.3389/fbioe.2022.1020020
Wang, L., Qin, W., Xu, W., Huang, F., Xie, X., Wang, F., et al. (2021). Bacteria-mediated tumor therapy via photothermally-programmed cytolysin A expression. Small 17, e2102932. doi:10.1002/smll.202102932
Wang, S., Guo, J., Bai, Y., Sun, C., Wu, Y., Liu, Z., et al. (2022c). Bacterial outer membrane vesicles as a candidate tumor vaccine platform. Front. Immunol. 13, 987419. doi:10.3389/fimmu.2022.987419
Wang, Y., Tang, Y., Du, Y., Lin, L., Zhang, Z., Ou, X., et al. (2022d). Genetically engineered bacteria-mediated multi-functional nanoparticles for synergistic tumor-targeting therapy. Acta Biomater. 150, 337–352. doi:10.1016/j.actbio.2022.07.056
Wei, B., Pan, J., Yuan, R., Shao, B., Wang, Y., Guo, X., et al. (2021). Polarization of tumor-associated macrophages by nanoparticle-loaded Escherichia coli combined with immunogenic cell death for cancer immunotherapy. Nano Lett. 21, 4231–4240. doi:10.1021/acs.nanolett.1c00209
Xie, J., Li, Q., Haesebrouck, F., Van Hoecke, L., and Vandenbroucke, R. E. (2022a). The tremendous biomedical potential of bacterial extracellular vesicles. Trends Biotechnol. 40, 1173–1194. doi:10.1016/j.tibtech.2022.03.005
Xie, S., Zhang, P., Zhang, Z., Liu, Y., Chen, M., Li, S., et al. (2021). Bacterial navigation for tumor targeting and photothermally-triggered bacterial ghost transformation for spatiotemporal drug release. Acta Biomater. 131, 172–184. doi:10.1016/j.actbio.2021.06.030
Xie, S., Zhao, L., Song, X., Tang, M., Mo, C., and Li, X. (2017). Doxorubicin-conjugated Escherichia coli Nissle 1917 swimmers to achieve tumor targeting and responsive drug release. J. Control Release 268, 390–399. doi:10.1016/j.jconrel.2017.10.041
Xie, Y. J., Huang, M., Li, D., Hou, J. C., Liang, H. H., Nasim, A. A., et al. (2022b). Bacteria-based nanodrug for anticancer therapy. Pharmacol. Res. 182, 106282. doi:10.1016/j.phrs.2022.106282
Yang, H., Jiang, F., Ji, X., Wang, L., Wang, Y., Zhang, L., et al. (2021a). Genetically engineered bacterial protein nanoparticles for targeted cancer therapy. Int. J. Nanomedicine 16, 105–117. doi:10.2147/IJN.S292432
Yang, M., Conceição, M., Chen, W., Yang, F., Zhao, B., Wood, M. J. A., et al. (2023). Engineered bacteria combined with doxorubicin nanoparticles suppress angiogenesis and metastasis in murine melanoma models. Acta Biomater. 158, 734–746. doi:10.1016/j.actbio.2022.12.027
Yang, M., Yang, F., Chen, W., Liu, S., Qiu, L., and Chen, J. (2021b). Bacteria-mediated cancer therapies: opportunities and challenges. Biomater. Sci. 9, 5732–5744. doi:10.1039/D1BM00634G
Yang, Y., Hou, J., Lin, Z., Zhuo, H., Chen, D., Zhang, X., et al. (2014). Attenuated Listeria monocytogenes as a cancer vaccine vector for the delivery of CD24, a biomarker for hepatic cancer stem cells. Cell. Mol. Immunol. 11, 184–196. doi:10.1038/cmi.2013.64
Yang, Y., Zhong, Z., Wang, B., Xia, X., Yao, W., Huang, L., et al. (2019). Early-life high-fat diet-induced obesity programs hippocampal development and cognitive functions via regulation of gut commensal Akkermansia muciniphila. Neuropsychopharmacol 44, 2054–2064. doi:10.1038/s41386-019-0437-1
Yao, Y., Li, J., Li, P., Wang, D., Bao, W., Xiao, Y., et al. (2022). Bacterially synthesized tellurium nanorods for elimination of advanced malignant tumor by photothermal immunotherapy. Small 18, e2105716. doi:10.1002/smll.202105716
Yoon, H. S., Cho, C. H., Yun, M. S., Jang, S. J., You, H. J., Kim, J. H., et al. (2021). Akkermansia muciniphila secretes a glucagon-like peptide-1-inducing protein that improves glucose homeostasis and ameliorates metabolic disease in mice. Nat. Microbiol. 6, 563–573. doi:10.1038/s41564-021-00880-5
Yue, Y., Xu, J., Li, Y., Cheng, K., Feng, Q., Ma, X., et al. (2022). Antigen-bearing outer membrane vesicles as tumour vaccines produced in situ by ingested genetically engineered bacteria. Nat. Biomed. Eng. 6, 898–909. doi:10.1038/s41551-022-00886-2
Yun, M., You, S. H., Nguyen, V. H., Prakash, J., Glasl, S., Gujrati, V., et al. (2021). Reporter gene-based optoacoustic imaging of E. coli targeted colon cancer in vivo. Sci. Rep. 11, 24430. doi:10.1038/s41598-021-04047-4
Zahan, T., Das, P. K., Akter, S. F., Habib, R., Rahman, M. H., Karim, M. R., et al. (2020). Therapy resistance in cancers: phenotypic, metabolic, epigenetic and tumour microenvironmental perspectives. Anticancer Agents Med. Chem. 20, 2190–2206. doi:10.2174/1871520620999200730161829
Zhang, H., Li, Z., Gao, C., Fan, X., Pang, Y., Li, T., et al. (2021). Dual-responsive biohybrid neutrobots for active target delivery. Sci. Robot. 6, eaaz9519. doi:10.1126/scirobotics.aaz9519
Zhang, M., Li, M., Du, L., Zeng, J., Yao, T., and Jin, Y. (2020). Paclitaxel-in-liposome-in-bacteria for inhalation treatment of primary lung cancer. Int. J. Pharm. 578, 119177. doi:10.1016/j.ijpharm.2020.119177
Zhang, Y., Zhai, Z., Duan, J., Wang, X., Zhong, J., Wu, L., et al. (2022). Lactate: the mediator of metabolism and immunosuppression. Front. Endocrinol. (Lausanne) 13, 901495. doi:10.3389/fendo.2022.901495
Zhao, M., Yang, M., Li, X. M., Jiang, P., Baranov, E., Li, S., et al. (2005). Tumor-targeting bacterial therapy with amino acid auxotrophs of GFP-expressing Salmonella typhimurium. Proc. Natl. Acad. Sci. U. S. A. 102, 755–760. doi:10.1073/pnas.0408422102
Zhao, M., Yang, M., Ma, H., Li, X., Tan, X., Li, S., et al. (2006). Targeted therapy with a Salmonella typhimurium leucine-arginine auxotroph cures orthotopic human breast tumors in nude mice. Cancer Res. 66, 7647–7652. doi:10.1158/0008-5472.CAN-06-0716
Zheng, D. W., Chen, Y., Li, Z. H., Xu, L., Li, C. X., Li, B., et al. (2018). Optically-controlled bacterial metabolite for cancer therapy. Nat. Commun. 9, 1680. doi:10.1038/s41467-018-03233-9
Zheng, D. W., Dong, X., Pan, P., Chen, K. W., Fan, J. X., Cheng, S. X., et al. (2019). Phage-guided modulation of the gut microbiota of mouse models of colorectal cancer augments their responses to chemotherapy. Nat. Biomed. Eng. 3, 717–728. doi:10.1038/s41551-019-0423-2
Zheng, M., Yue, C., Ma, Y., Gong, P., Zhao, P., Zheng, C., et al. (2013). Single-step assembly of DOX/ICG loaded lipid-polymer nanoparticles for highly effective chemo-photothermal combination therapy. ACS Nano 7, 2056–2067. doi:10.1021/nn400334y
Zhou, S., Gravekamp, C., Bermudes, D., and Liu, K. (2018). Tumour-targeting bacteria engineered to fight cancer. Nat. Rev. Cancer 18, 727–743. doi:10.1038/s41568-018-0070-z
Zhu, X., Chen, S., Hu, X., Zhao, L., Wang, Y., Huang, J., et al. (2023). Near-infrared nano-optogenetic activation of cancer immunotherapy via engineered bacteria. Adv. Mater 35, e2207198. doi:10.1002/adma.202207198
Keywords: bacteria, bacteria-derived membrane vesicles, drug delivery systems, cancer therapy, outer membranes vesicles
Citation: Song D, Yang X, Chen Y, Hu P, Zhang Y, Zhang Y, Liang N, Xie J, Qiao L, Deng G, Chen F and Zhang J (2023) Advances in anti-tumor based on various anaerobic bacteria and their derivatives as drug vehicles. Front. Bioeng. Biotechnol. 11:1286502. doi: 10.3389/fbioe.2023.1286502
Received: 31 August 2023; Accepted: 21 September 2023;
Published: 03 October 2023.
Edited by:
Zunzhen Ming, Tongji University, ChinaCopyright © 2023 Song, Yang, Chen, Hu, Zhang, Zhang, Liang, Xie, Qiao, Deng, Chen and Zhang. This is an open-access article distributed under the terms of the Creative Commons Attribution License (CC BY). The use, distribution or reproduction in other forums is permitted, provided the original author(s) and the copyright owner(s) are credited and that the original publication in this journal is cited, in accordance with accepted academic practice. No use, distribution or reproduction is permitted which does not comply with these terms.
*Correspondence: Fangjie Chen, aW1jaGVuZmFuZ2ppZUAxNjMuY29t; Jiandong Zhang, emhhbmdqZDE2NUBzaW5hLmNvbQ==