- 1Faculty of Natural Sciences, Institute of Laboratory Research on Geomaterials, Comenius University in Bratislava, Bratislava, Slovakia
- 2Department of Environmental Engineering, Faculty of Mining and Geology, VŠB–Technical University of Ostrava, Ostrava, Czechia
- 3Radioactive Waste Repository Authority (SÚRAO), Praha, Czechia
- 4Department of Applied Chemistry, Faculty of Agriculture and Technology, University of South Bohemia, České Budějovice, Czechia
Stable iodine isotopes are essential for humans as they are necessary for producing thyroid gland hormones. However, there are hazardous radioactive iodine isotopes that are emitted into the environment through radioactive waste generated by nuclear power plants, nuclear weapon tests, and medical practice. Due to the biophilic character of iodine radionuclides and their enormous biomagnification potential, their elimination from contaminated environments is essential to prevent the spread of radioactive pollution in ecosystems. Since microorganisms play a vital role in controlling iodine cycling and fate in the environment, they also can be efficiently utilized in solving the issue of contamination spread. Thus, this paper summarizes all known on microbial processes that are involved in iodine transformation to highlight their prospects in remediation of the sites contaminated with radioactive iodine isotopes.
1 Introduction
Iodine is a vital trace element essential for both humans and animals. Iodine deficiency disorders often occur in regions where the geochemical environment has low iodine levels. Iodine plays a crucial role in the synthesis of thyroid hormones, specifically T4 (tetraiodo-L-thyronine) and T3 (triiodo-L-thyronine) (Sorrenti et al., 2021). These hormones are responsible for regulating metabolism, growth, and development (Andersson and Braegger, 2021). They control basal metabolic rate, protein synthesis, long bone growth, and neuronal maturation (Mégier et al., 2023). Apart from its role in hormone synthesis, iodine deficiency is also a risk factor for thyroid cancer (Fan et al., 2021). Adequate iodine supply is crucial for preventing diseases of the mammary gland, as iodine lactones may protect against oxidative damage and inhibit cell division (Kupper and Gartner, 2007). Besides its biological importance iodine salts are widely used in pharmaceuticals (Nobukuni, 2009) and antiseptic agents (Grzybowski et al., 2018), radiocontrast agents (Pasternak and Williamson, 2012), light bulbs (Bregnhøj et al., 2019), solar cells (Sun et al., 2015), and LCD displays (Ma et al., 2011).
There are 36 known radioactive isotopes of iodine, with particular attention given to 129I and 131I (Petrov et al., 2022). Following the Fukushima power plant accident in 2011, the concentration of 129I in precipitation increased by four orders of magnitude (Xu et al., 2013), resulting in 100 times higher thyroid radioiodine concentrations of exposed cattle from the affected area in comparison to the control group (Horikami et al., 2022).
In offshore surface waters near Fukushima, 129I concentrations ranged from 0.002 to 0.133 pg. L-1 (Hou et al., 2013; Suzuki et al., 2013). Following the Fukushima nuclear power plant accident, 129I levels in the upper 100 m layer of seawater increased on average to 0.02 pg. L-1, with a maximum of 0.13–0.19 pg. L-1 (Suzuki et al., 2013; Xu et al., 2013). While the dominant iodine specie in seawater is iodate, Hou et al. (2013) observed that the primary form of 129I is iodide.
In the surface layers of Belarusian soil, 129I concentrations rose dramatically after the Chernobyl accident, increasing by three orders of magnitude, from 14.7 pg.kg−1 to 7.7 ng.kg−1 (Mironov et al., 2002).
The median value of yearly release of short-lived iodine isotopes from nuclear power plants in the United States is estimated to range from 10–7 to 10–5 GBq.GW-1. h-1, depending on the type of reactor (Ekidin et al., 2022).
Recently, the most promising materials for iodine remediation from nuclear waste include metal oxides (Muhire et al., 2022), bentonite (Yang et al., 2022) and zeolites (Zhang et al., 2022), bismuth-based mineral phases (Levitskaia et al., 2022), silica aerogel (Chang et al., 2022), organopolymeric structures (Kusumkar et al., 2021; Mohan et al., 2022) and various biomass-based sorbents, such as Bi-impregnated spent coffee ground biochar (Kwak et al., 2022). The application of microorganisms was also proposed (Shin et al., 2022).
Microorganisms play an important role in iodine cycling either by active production of its volatile species to the atmosphere or by changing environmental conditions in terrestrial system, thus affecting their retention. In aerobic environments, partially sorbed IO3− species to sediments may be released back into the environment as a consequence of microbially generated reducing conditions (Guido-Garcia et al., 2015). On the other hand, extracellular oxidases of bacterial origin act as a catalyst for iodine retention in soil (Grandbois et al., 2023). Microbial influences on iodine speciation and its environmental fate are complex, governed by both extracellular and intracellular mechanisms, as depicted in Figure 1. Since this review focuses on the biochemical transformations of iodine and their potential in remediation strategies, for comprehensive information on the global iodine environmental cycle refer to our previous work (Duborská et al., 2021a; Duborská et al., 2021b).
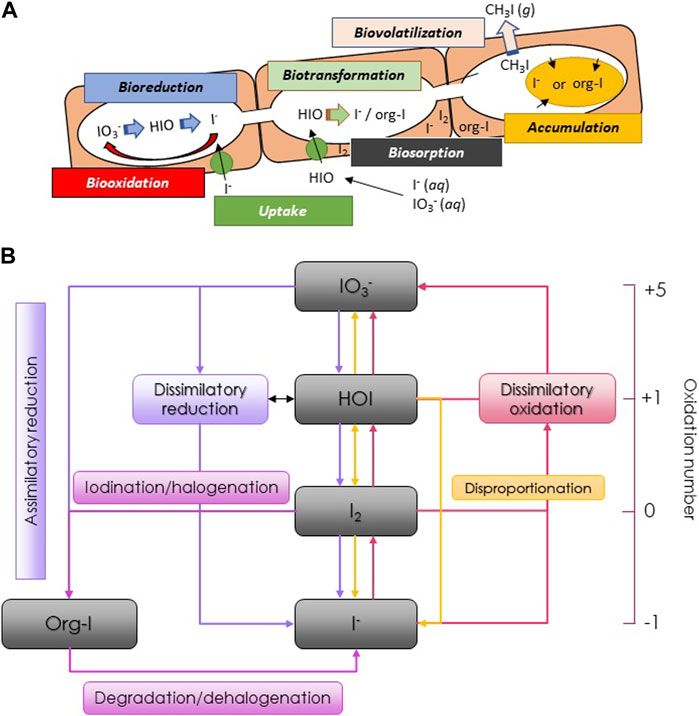
FIGURE 1. (A) Processes of microbially mediated iodine transformations; (B) Biochemical cycle of iodine.
2 Microbial reduction of iodate
Determining iodine species in surface waters poses a significant challenge; iodate (IO3−) is noted as the prevailing species, which can undergo microbially mediated reduction (Zaruba et al., 2017; Wadley et al., 2020). The exact mechanism of iodate reduction is still unclear; however, available data suggest that it is mediated by microorganisms via enzymatic transformations and interactions with extracellular metabolites. It was widely assumed that iodate can be reduced aerobically in surface waters to iodide by microorganisms having a nitrate-reducing activity, such as Escherichia coli (Tsunogai and Sase, 1969). It was hypothesized that iodate electron transport pathways are terminated with the reduction of iodate by nitrate reductase, which serves as an alternative electron acceptor (Toporek et al., 2019). Later, however, Mok et al. (2018) demonstrated that reduction of IO3− by Shewanella oneidensis does not involve nitrate reductase and it is independent of nitrate uptake. On the other hand, Kengen et al. (1999) isolated a chlorate reductase resembling nitrate reductase, which possessed nitrate, iodate and bromate reducing activity. Lee et al. (2018) isolated a bacterium from the groundwaters of radioiodine contaminated Hanford site, which is closely related to Agrobacterium sp. and was capable of the simultaneous reduction of nitrate and iodate. Furthermore, iodate reduction was not observed when nitrate was absent from the growth medium.
Besides bacteria, several species of phytoplankton are also producing iodide, as reported by Bluhm et al. (2010), who assumed that due to increased cell permeability during the senescent phase of the algae, the exuded sulfidic species reduced the iodate. The reduced sulfidic species or glutathione were also found to promote iodate reduction during cell apoptosis (Taurog et al., 1966). However, later, it was suggested that the presence of iodide may be the result of the release of I− from C-I bonds during the decomposition of organic matter instead of IO3− reduction by sulfide (Farrenkopf et al., 1997).
The idea of the existence of an independent iodate reductase was already proposed by early studies, however, such enzyme has not been yet completely characterized. Iodate-reducing bacterium Pseudomonas sp., isolated from marine sediments by Amachi et al. (2007), reduces iodate anaerobically via a specific iodate reductase. Recent studies also indicate that iodate reduction by S. oneidensis involves a yet unidentified iodate reductase associated with the outer membrane MtrAB module, which enables the extracellular reduction of iodate (Toporek et al., 2019). The same bacterial specie was also studied by Shin et al. (2022), who suggested that an extracellular EEC-associated dimethyl sulfoxide (DMSO) reductase with a molybdenum enzyme center is responsible for formate-dependent iodate reduction. A recent study by Reyes-Umana et al. (2022) showed that the dissimilatory iodate reduction by estuarine bacterium Denitromonas sp. is mediated by a molybdenum-dependent iodate reductase. Such molybdoenzyme, a dimethylsulfoxide reductase, was also isolated from Pseudomonas sp. (Yamazaki et al., 2020). This specie uses iodate as a terminal electron acceptor for anaerobic respiration. Based on their results with hypobromous acid, Müller et al. (2021) suggested that the reaction of dimethyl sulfide (DMS) with hypoiodous acid might also be a possible mechanism of assimilatory iodate reduction.
3 Microbial oxidation
Shimada et al. (2022) presented, that 90% of 129I in drain water of the Fukushima nuclear power plant was in the form of iodide. Still, it can be oxidized by microorganisms. An iodine-oxidizing bacterium, Pseudomonas iodooxidans, was first identified by Gozlan and Margalith (1974). This specie possesses iodide oxidation abilities due to the production of a specific heme-peroxidase. Such enzymatic activity requires oxygen, but not hydrogen peroxide, as an electron acceptor. This was proposed by Amachi et al. (2005b) after isolating iodide oxidizing bacteria from natural gas brine waters, which were phylogenetically most closely related to Roseovarius tolerans and Rhodothalassium salexigens. This enzyme was later identified as a multicopper oxidase by Suzuki et al. (2012), who isolated it from an Alphaproteobacteria strain.
The molecular iodine produced by catalytic oxidation of iodide by Roseovarius sp. inhibited the growth of other sensitive bacteria in the environment, such as E. coli, as demonstrated by Zhao et al. (2013). Later, Yuliana et al. (2015) suggested that this iodide oxidizing enzyme could be utilized as a novel antimicrobial enzymatic system.
A newly discovered aerobic iodide oxidizing bacteria Iodidimonas gelatinilytica isolated by Iino et al. (2021) from brine and surface seawater was capable of oxidizing iodide by a putative multicopper oxidase, which is phylogenetically distinct from other bacterial multicopper oxidases (Amachi and Iino, 2022).
In terrestrial systems, extracellular iodide oxidases of bacterial and fungal origin have an important role in iodine retention potential of surface soils (Seki et al., 2013). In early studies, it was suggested by Raciborski (1905) that Aspergillus niger produces an extracellular iodide-oxidase, which is responsible for the oxidation of iodide to free iodine. Later, Pearce (1940) discovered that this oxidation is catalyzed by hydrogen peroxidase, therefore, the oxidation of iodide is a secondary oxidation reaction by hydrogen peroxide formed as a result of oxidation of glucose catalyzed by glucose oxidase. Similar multicopper oxidase was found to play role in terrestrial bacterium Rhodanobacter denitrificans (Shiroyama et al., 2015).
In soils, at least part of iodide is oxidized by fungal laccase in the presence of redox mediators (Nihei et al., 2018; Lee et al., 2020). Nihei et al. (2018) isolated iodide-oxidizing fungi from soil, including the strains of Trichoderma hamatum and Scytalidium album.
4 Iodine biomethylation
Methylated iodine production is a growth rate-dependent process in most marine heterotrophic bacterial groups (Gómez-Consarnau et al., 2021). Iodide-oxidizing bacteria also possess volatile organic iodine production abilities. Fuse et al. (2003) identified iodine-methylating marine bacterium (phylogenetically close to R. tolerans) that produces CH2I2, CH3I and CH2ClI. The sole production of CH3I by this specie was reported by Amachi et al. (2005b). Methyl iodide production was observed in members of Proteobacteria, Cytophaga-Flexibacter-Bacteroides groups (Amachi et al., 2004), Alteromonas sp., Vibrio sp. (Amachi et al., 2001), and marine cyanobacterium Calothrix parasitica (Okuda et al., 2023). Even brown algae Ectocarpus sp. (Küpper et al., 2018) and Laminaria sp. (Nightingale et al., 1995) produce methylated iodine compounds.
Terrestrial bacteria, e.g., Varivorax sp. (Amachi et al., 2001), and microscopic filamentous fungi, such as Alternaria alternata and Fusarium oxysporum (Ban-nai et al., 2006; Duborská et al., 2017), are also capable of iodine methylation.
Although, the exact mechanism is not yet completely explained, iodine methylation by microorganisms is probably following the pathway of the Challenger mechanism that is typical for As methylation (Challenger, 1951). Alternatively, a vanadium-dependent haloperoxidase enzyme is involved in this unique process (Punitha et al., 2018).
5 Bioaccumulation
Various elements, including radionuclides, can be actively taken up by microorganisms (Urik et al., 2010; Urik et al., 2011) or be fixed to microbial cell walls or extracellular polysaccharides of biofilms (Čerňanský et al., 2007; Littera et al., 2011). Iodide accumulation in bacteria presumably takes place via nucleophilic substitution of functional groups in cellular organic molecules (Li et al., 2011). The iodine uptake ability is relatively high in Cyanobacteria, green algae and Ochrophytes (Fukuda et al., 2014). High accumulation ability was found in bacterial strains of Streptomyces/Kitasatospora spp., Ralstonia/Cupriavidus spp., and Bacillus mycoides that were isolated from aquifers at 129I-contaminated Savannah River site. However, these aerobic bacteria did not accumulate significant amounts of iodine (Li et al., 2011). Fukuda et al. (2014) identified three cyanobacteria (Nostoc commune, Scytonema javanicum, Stigonema ocellatum) and xantophycean algae Ophiocytum sp. with high ability to accumulate radioactive 125I from water. Amachi et al. (2005a) isolated two iodide-accumulating bacteria from marine sediments belonging to the Flavobacteriaceae family and were closely related to Flexibacter aggregans and Arenibacter troitsensis. Iwamoto and Shiraiwa (2012) suggested that microalgae Emiliana huxleyi could be used in industry for extracting iodine from iodine-contaminated waters, since it is able to accumulate ten times more iodine than normally found in seawaters.
According to our best knowledge, only two studies available present iodine accumulation rates of microscopic filamentous fungi. Therefore more attention should be paid to these species since they cover up to 30% of soil microbial biomass (Gschwend et al., 2022) and participate in a whole range of processes (Costa et al., 2018).
Korobova (2010) reported 1.8–147 mg.kg-1 iodine levels in further not specified soil microorganisms (dry weight) in the central Russian plain but suggested that fungi might have been a great contributors to this number. The highest iodine accumulation rates under laboratory conditions were reported for Alternaria alternata, a soil-borne pathogen, up to 63 μg.g-1 with an equally high capacity for both iodide and iodate species from culture media (Ban-nai et al., 2006; Duborská et al., 2017). Penicillium chrysogenum is able to accumulate up to 10.2% of iodine depending on soil iodine content and the degree of the organisms' tolerance to its high concentrations (Letunova et al., 1986).
6 Potential use of microorganism in bioremediation
Iodine remediation techniques involving microorganisms in contaminated sites have been studied in limited case studies, however, they offer great potential for addressing iodine contamination through various interaction mechanisms. These mechanisms include microbial methylation of iodide to volatile gases and microbial reduction of iodate to iodide (Shin et al., 2022). Understanding and harnessing these microbial processes can significantly contribute to effective iodine remediation strategies. Species which could be potentially useful for iodine remediation are presented in Table 1.
Deinococcus radiodurans, a radiation-resistant bacterium known for its remarkable resilience, has shown promising results as an excellent candidate for on-site bioremediation. Research conducted by Choi et al. (Choi et al., 2016; Choi et al., 2017; Shim et al., 2018) demonstrated that the biomass of D. radiodurans, containing biogenic gold and silver nanoparticles, prepared by bioprecipitation, exhibits more than 99% removal efficiency. This suggests that these complexes hold significant potential for addressing iodine contamination.
In a recent study conducted by Tang et al. (2022), a novel approach for capturing radioactive iodine from polluted atmospheres was presented. The researchers developed a porous sponge-like complex absorbent composed of alginate and fungal mycelium of Actinomucor elegans. This absorbent material offers a high surface area and efficient capture of radioactive iodine, providing a potential solution for addressing airborne contamination.
Nanocomposites made of bacterial cellulose pellicles present another viable approach for iodine capture. These nanocomposites, which exhibit irregularly webbed and highly hydrated structures, offer superior chemical and structural stability. Cellulose-producing bacteria, such as Acetobacter xylinus, are responsible for producing these nanocomposites. Zia et al. (Zia et al., 2022) explored the potential of bacterial cellulose pellicle nanocomposites as effective iodine capture agents from both vapor and aqueous solutions. These nanocomposites’ have high surface area and their stability make them suitable for capturing and removing iodine contaminants.
Au-Duong and Lee (2018) developed a flexible metal-organic framework-bacterial cellulose nanocomposite by immersing bacterial cellulose pellicles in zinc ions and 2-methylimidazole. After photothermal regeneration, this nanocomposite exhibited remarkable performance, maintaining 99% and 87% of its initial iodine uptake capacity during the second and sixth uses, respectively. This finding highlights the potential of metal-organic framework-bacterial cellulose nanocomposites for long-term and sustainable iodine remediation efforts.
Sasamura et al. (2023) proposed Azocarus sp. as a potential bioaugmentation agent for 129I-contaminated waters. This strain demonstrates an effective iodate respiration mechanism, making it a promising candidate for bioremediation in water environments.
In addition to these innovative approaches, iodine biomethylation holds promise as a means for capturing and recovering iodine volatilized by microorganisms. Technologies such as soil vapor extraction can be employed to capture iodine vapors, while activated carbon adsorption provides an effective method for capturing and concentrating iodine from both air and water sources. Strickland et al. (2017) explored the use of activated carbon as a reliable medium for iodine capture, which can subsequently be regenerated or safely disposed of, ensuring the sustainability of the remediation process.
7 Conclusion
The anthropogenic input of radioactive iodine into the atmosphere is a matter of great significance. Currently, there is considerable focus on the development of environmentally friendly adsorbents. Biomass-based sorbents show promise as a cost-effective solution for remediating iodine from water contaminated with atmospheric deposits of radioactive iodine, thereby preventing its incorporation and biomagnification in the food chain. The contamination of seawater and kelp farms with radioiodine poses a particular threat, as these species have been found to accumulate high levels of radioactive 129I. While the study of native soil microbiota should be taken into consideration, the precise role and mechanisms by which they retain and release iodine from soils remain unclear. Nevertheless, soil serves as a significant sink for anthropogenic iodine from the atmosphere, which can subsequently accumulate in plants and forage.
The studies discussed in this paper provide valuable insights into microbially-involved iodine remediation techniques, covering microbial interactions, bioaugmentation, nanocomposites, and biomethylation. By understanding and utilizing these diverse strategies, researchers can contribute to the development of effective and sustainable iodine remediation approaches. Microorganisms play diverse roles in the cycling of iodine and offer promising potential for bioremediation strategies. Further research is necessary to fully comprehend the underlying mechanisms and explore practical applications of microbial processes in addressing iodine contamination. These findings open up new possibilities for the development of effective and sustainable approaches to mitigate iodine pollution in various environments.
Author contributions
ED: Writing–original draft, Writing–review and editing. HV: Writing–original draft. MM: Writing–original draft. MS: Writing–original draft. PM: Writing–original draft.
Funding
The author(s) declare financial support was received for the research, authorship, and/or publication of this article. This work has been financially supported by the Scientific Grant Agency of the Ministry of Education of the Slovak Republic and the Slovak Academy of Sciences under the contracts VEGA No. 1/0139/22 and Project for Specific University Research (SGS) No. SP2023/4 by the Faculty of Mining and Geology of VŠB—Technical University of Ostrava.
Conflict of interest
The authors declare that the research was conducted in the absence of any commercial or financial relationships that could be construed as a potential conflict of interest.
Publisher’s note
All claims expressed in this article are solely those of the authors and do not necessarily represent those of their affiliated organizations, or those of the publisher, the editors and the reviewers. Any product that may be evaluated in this article, or claim that may be made by its manufacturer, is not guaranteed or endorsed by the publisher.
References
Amachi, S., and Iino, T. (2022). The genus Iodidimonas: from its discovery to potential applications. Microorganisms 10, 1661. doi:10.3390/microorganisms10081661
Amachi, S., Kamagata, Y., Kanagawa, T., and Muramatsu, Y. (2001). Bacteria mediate methylation of iodine in marine and terrestrial environments. Appl. Environ. Microbiol. 67, 2718–2722. doi:10.1128/aem.67.6.2718-2722.2001
Amachi, S., Kasahara, M., Fujii, T., Shinoyama, H., Hanada, S., Kamagata, Y., et al. (2004). Radiotracer experiments on biological volatilization of organic iodine from coastal seawaters. Geomicrobiol. J. 21, 481–488. doi:10.1080/01490450490506201
Amachi, S., Kawaguchi, N., Muramatsu, Y., Tsuchiya, S., Watanabe, Y., Shinoyama, H., et al. (2007). Dissimilatory iodate reduction by marine Pseudomonas sp. Strain SCT 73, 5725–5730. doi:10.1128/aem.00241-07
Amachi, S., Mishima, Y., Shinoyama, H., Muramatsu, Y., and Fujii, T. (2005a). Active transport and accumulation of iodide by newly isolated marine bacteria. Appl. Environ. Microbiol. 71, 741–745. doi:10.1128/aem.71.2.741-745.2005
Amachi, S., Muramatsu, Y., Akiyama, Y., Miyazaki, K., Yoshiki, S., Hanada, S., et al. (2005b). Isolation of iodide-oxidizing bacteria from iodide-rich natural gas brines and seawaters. Microb. Ecol. 49, 547–557. doi:10.1007/s00248-004-0056-0
Andersson, M., and Braegger, C. P. (2021). The role of iodine for thyroid function in lactating women and infants. Endocr. Rev. 43, 469–506. doi:10.1210/endrev/bnab029
Au-Duong, A.-N., and Lee, C.-K. (2018). Flexible metal–organic framework-bacterial cellulose nanocomposite for iodine capture. Cryst. Growth and Des. 18, 356–363. doi:10.1021/acs.cgd.7b01360
Ban-Nai, T., Muramatsu, Y., and Amachi, S. (2006). Rate of iodine volatilization and accumulation by filamentous fungi through laboratory cultures. Chemosphere 65, 2216–2222. doi:10.1016/j.chemosphere.2006.05.047
Bluhm, K., Croot, P., Wuttig, K., and Lochte, K. (2010). Transformation of iodate to iodide in marine phytoplankton driven by cell senescence. Aquat. Biol. 11, 1–15. doi:10.3354/ab00284
Bregnhøj, M., Strunge, K., Sørensen, R. J., Ströbele, M., Hummel, T., Meyer, H. J., et al. (2019). Tungsten iodide clusters as singlet oxygen photosensitizers: exploring the domain of resonant Energy transfer at 1 eV. J. Phys. Chem. A 123, 1730–1739. doi:10.1021/acs.jpca.9b00541
Čerňanský, S., Urik, M., Ševc, J., Littera, P., and Hiller, E. (2007). Biosorption of arsenic and cadmium from aqueous solutions. Afr. J. Biotechnol. 6, 1932–1934. doi:10.5897/ajb2007.000-2293
Challenger, F. (1951). “Biological methylation,” in Advances in enzymology and related areas of molecular biology, 429–491.
Chang, S., Wang, K., Li, Y., Wang, J., Song, X., Zhang, Z., et al. (2022). A novel three-dimensionally ordered macroporous aerogel for capturing radioactive gaseous iodine. Ceram. Int. 48, 35310–35316. doi:10.1016/j.ceramint.2022.08.133
Choi, M. H., Jeong, S.-W., Shim, H. E., Yun, S.-J., Mushtaq, S., Choi, D. S., et al. (2017). Efficient bioremediation of radioactive iodine using biogenic gold nanomaterial-containing radiation-resistant bacterium, Deinococcus radiodurans R1. Chem. Commun. 53, 3937–3940. doi:10.1039/c7cc00720e
Choi, M. H., Shim, H.-E., Yun, S.-J., Park, S.-H., Choi, D. S., Jang, B.-S., et al. (2016). Gold-nanoparticle-immobilized desalting columns for highly efficient and specific removal of radioactive iodine in aqueous media. ACS Appl. Mater. Interfaces 8, 29227–29231. doi:10.1021/acsami.6b11136
Costa, O. Y. A., Raaijmakers, J. M., and Kuramae, E. E. (2018). Microbial extracellular polymeric substances: ecological function and impact on soil aggregation. Front. Microbiol. 9, 1636. doi:10.3389/fmicb.2018.01636
Duborská, E., Balíková, K., Matulová, M., Zvěřina, O., Farkas, B., Littera, P., et al. (2021a). Production of methyl-iodide in the environment. Front. Microbiol. 12, 804081. doi:10.3389/fmicb.2021.804081
Duborská, E., Matulová, M., Vaculovič, T., Matúš, P., and Urík, M. J. F. (2021b). Iodine fractions in soil and their determination. Forests 12, 1512. doi:10.3390/f12111512
Duborská, E., Urík, M., and Bujdoš, M. (2017). Comparison of iodide and iodate accumulation and volatilization by filamentous fungi during static cultivation. Water, Air, and Soil Pollut. 228, 225. doi:10.1007/s11270-017-3407-4
Ekidin, A. A., Antonov, K. L., and Nazarovich, A. V. (2022). Estimation of the specific activity of iodine release to comply with the base principle of INPRO methodology for PWR, BWR NPP. At. Energy 131, 291–297. doi:10.1007/s10512-022-00881-5
Fan, L., Meng, F., Gao, Y., and Liu, P. (2021). Insufficient iodine nutrition may affect the thyroid cancer incidence in China. Br. J. Nutr. 126, 1852–1860. doi:10.1017/s0007114521000593
Farrenkopf, A. M., Luther, G. W., Truesdale, V. W., and Van Der Weijden, C. H. (1997). Sub-surface iodide maxima: evidence for biologically catalyzed redox cycling in Arabian Sea OMZ during the SW intermonsoon. Deep Sea Res. Part II Top. Stud. Oceanogr. 44, 1391–1409. doi:10.1016/s0967-0645(97)00013-1
Fukuda, S.-Y., Iwamoto, K., Atsumi, M., Yokoyama, A., Nakayama, T., Ishida, K.-I., et al. (2014). Global searches for microalgae and aquatic plants that can eliminate radioactive cesium, iodine and strontium from the radio-polluted aquatic environment: a bioremediation strategy. J. Plant Res. 127, 79–89. doi:10.1007/s10265-013-0596-9
Fuse, H., Inoue, H., Murakami, K., Takimura, O., and Yamaoka, Y. (2003). Production of free and organic iodine by Roseovarius spp. FEMS Microbiol. Lett. 229, 189–194. doi:10.1016/s0378-1097(03)00839-5
Gómez-Consarnau, L., Klein, N. J., Cutter, L. S., and Sañudo-Wilhelmy, S. A. (2021). Growth rate-dependent synthesis of halomethanes in marine heterotrophic bacteria and its implications for the ozone layer recovery. Environ. Microbiol. Rep. 13, 77–85. doi:10.1111/1758-2229.12905
Gómez-Jacinto, V., García-Barrera, T., Garbayo-Nores, I., Vilchez-Lobato, C., and Gómez-Ariza, J.-L. (2012). Metal-metabolomics of microalga Chlorella sorokiniana growing in selenium- and iodine-enriched media. Chem. Pap. 66, 821–828. doi:10.2478/s11696-012-0186-7
Gozlan, R. S., and Margalith, P. (1974). Iodide oxidation by Pseudomonas iodooxidans. J. Appl. Bacteriol. 37, 493–499. doi:10.1111/j.1365-2672.1974.tb00474.x
Grandbois, R. M., Santschi, P. H., Xu, C., Mitchell, J. M., Kaplan, D. I., and Yeager, C. M. (2023). Iodide uptake by forest soils is principally related to the activity of extracellular oxidases. Front. Chem. 11. doi:10.3389/fchem.2023.1105641
Grzybowski, A., Kanclerz, P., and Myers, W. G. (2018). The use of povidone–iodine in ophthalmology. Curr. Opin. Ophthalmol. 29, 19–32. doi:10.1097/icu.0000000000000437
Gschwend, F., Hartmann, M., Mayerhofer, J., Hug, A. S., Enkerli, J., Gubler, A., et al. (2022). Site and land-use associations of soil bacteria and fungi define core and indicative taxa. FEMS Microbiol. Ecol. 97, fiab165. doi:10.1093/femsec/fiab165
Guido-Garcia, F., Law, G. T. W., Lloyd, J. R., Lythgoe, P., and Morris, K. (2015). Bioreduction of iodate in sediment microcosms. Mineral. Mag. 79, 1343–1351. doi:10.1180/minmag.2015.079.6.10
Han, W., Clarke, W., and Pratt, S. (2016). Cycling of iodine by microalgae: iodine uptake and release by a microalgae biofilm in a groundwater holding pond. Ecol. Eng. 94, 286–294. doi:10.1016/j.ecoleng.2016.05.001
Horikami, D., Sayama, N., Sasaki, J., Kusuno, H., Matsuzaki, H., Hayashi, A., et al. (2022). The effect of exposure on cattle thyroid after the Fukushima Daiichi nuclear power plant accident. Sci. Rep. 12, 21754. doi:10.1038/s41598-022-25269-0
Hou, X., Povinec, P. P., Zhang, L., Shi, K., Biddulph, D., Chang, C.-C., et al. (2013). Iodine-129 in seawater offshore Fukushima: distribution, inorganic speciation, sources, and budget. Environ. Sci. Technol. 47, 3091–3098. doi:10.1021/es304460k
Iino, T., Oshima, K., Hattori, M., Ohkuma, M., and Amachi, S. (2021). Iodidimonas gelatinilytica sp. nov., aerobic iodide-oxidizing bacteria isolated from brine water and surface seawater. Antonie Leeuwenhoek 114, 625–631. doi:10.1007/s10482-021-01546-2
Iwamoto, K., and Shiraiwa, Y. (2012). Characterization of intracellular iodine accumulation by iodine-tolerant microalgae. Procedia Environ. Sci. 15, 34–42. doi:10.1016/j.proenv.2012.05.007
Kengen, S. W., Rikken, G. B., Hagen, W. R., Van Ginkel, C. G., and Stams, A. J. (1999). Purification and characterization of (per)chlorate reductase from the chlorate-respiring strain GR-1. J. Bacteriol. 181, 6706–6711. doi:10.1128/jb.181.21.6706-6711.1999
Korobova, E. (2010). Soil and landscape geochemical factors which contribute to iodine spatial distribution in the main environmental components and food chain in the central Russian plain. J. Geochem. Explor. 107, 180–192. doi:10.1016/j.gexplo.2010.03.003
Kupper, C., and Gartner, R. (2007). Risk of breast cancer and iodine deficiency. Ernahrungs-Umschau 54, 324-+.
Küpper, F. C., Miller, E. P., Andrews, S. J., Hughes, C., Carpenter, L. J., Meyer-Klaucke, W., et al. (2018). Emission of volatile halogenated compounds, speciation and localization of bromine and iodine in the brown algal genome model Ectocarpus siliculosus. JBIC J. Biol. Inorg. Chem. 23, 1119–1128. doi:10.1007/s00775-018-1539-7
Kusumkar, V. V., Galamboš, M., Viglašová, E., Daňo, M., and Šmelková, J. (2021). Ion-imprinted polymers: synthesis, characterization, and adsorption of radionuclides. Materials 14, 1083. doi:10.3390/ma14051083
Kwak, J., Lee, S.-H., Shin, J., Lee, Y.-G., Kim, S., Son, C., et al. (2022). Synthesis and applications of bismuth-impregnated biochars originated from spent coffee grounds for efficient adsorption of radioactive iodine: a mechanism study. Environ. Pollut. 313, 120138. doi:10.1016/j.envpol.2022.120138
Lee, B. D., Ellis, J. T., Dodwell, A., Eisenhauer, E. E. R., Saunders, D. L., and Lee, M. H. (2018). Iodate and nitrate transformation by Agrobacterium/Rhizobium related strain DVZ35 isolated from contaminated Hanford groundwater. J. Hazard. Mater. 350, 19–26. doi:10.1016/j.jhazmat.2018.02.006
Lee, B. D., Moser, E. L., Brooks, S. M., Saunders, D. L., and Howard, M. H. (2020). Microbial contribution to iodine speciation in hanford's central plateau groundwater: iodide oxidation. Front. Environ. Sci. 7. doi:10.3389/fenvs.2019.00145
Letunova, S. V., Alekseeva, S. A., and Korobova, E. M. (1986). Iodine concentration by the fungus Penicillium chrysogenum inhabiting soils of the nonchernozem zone. Nauchnye Doki Vyss Shkoly Biol. Nauki, 94–98.
Levitskaia, T. G., Qafoku, N. P., Bowden, M. E., Asmussen, R. M., Buck, E. C., Freedman, V. L., et al. (2022). A review of bismuth(III)-Based materials for remediation of contaminated sites. ACS Earth Space Chem. 6, 883–908. doi:10.1021/acsearthspacechem.1c00114
Li, H.-P., Brinkmeyer, R., Jones, W. L., Zhang, S., Xu, C., Schwehr, K., et al. (2011). Iodide accumulation by aerobic bacteria isolated from subsurface sediments of a 129 I-contaminated aquifer at the Savannah River site, South Carolina. Appl. Environ. Microbiol. 77, 2153–2160. doi:10.1128/aem.02164-10
Littera, P., Urík, M., Ševc, J., Kolenčík, M., Gardošová, K., and Molnárová, M. (2011). Removal of arsenic from aqueous environments by native and chemically modified biomass of Aspergillus Niger and Neosartorya fischeri. Environ. Technol. 32, 1215–1222. doi:10.1080/09593330.2010.532510
Ma, J., Ye, X., and Jin, B. (2011). Structure and application of polarizer film for thin-film-transistor liquid crystal displays. Displays 32, 49–57. doi:10.1016/j.displa.2010.12.006
Mégier, C., Dumery, G., and Luton, D. (2023). Iodine and thyroid maternal and fetal metabolism during pregnancy. Metabolites 13, 633. doi:10.3390/metabo13050633
Mironov, V., Kudrjashov, V., Yiou, F., and Raisbeck, G. M. (2002). Use of 129I and 137Cs in soils for the estimation of 131I deposition in Belarus as a result of the Chernobyl accident. J. Environ. Radioact. 59, 293–307. doi:10.1016/s0265-931x(01)00080-7
Mohan, A., Al-Sayah, M. H., Ahmed, A., and El-Kadri, O. M. (2022). Triazine-based porous organic polymers for reversible capture of iodine and utilization in antibacterial application. Sci. Rep. 12, 2638. doi:10.1038/s41598-022-06671-0
Mok, J. K., Toporek, Y. J., Shin, H.-D., Lee, B. D., Lee, M. H., and Dichristina, T. J. (2018). Iodate reduction by Shewanella oneidensis does not involve nitrate reductase. Geomicrobiol. J. 35, 570–579. doi:10.1080/01490451.2018.1430189
Muhire, C., Tesfay Reda, A., Zhang, D., Xu, X., and Cui, C. (2022). An overview on metal Oxide-based materials for iodine capture and storage. Chem. Eng. J. 431, 133816. doi:10.1016/j.cej.2021.133816
Müller, E., Von Gunten, U., Bouchet, S., Droz, B., and Winkel, L. H. E. (2021). Reaction of DMS and HOBr as a sink for marine DMS and an inhibitor of bromoform formation. Environ. Sci. Technol. 55, 5547–5558. doi:10.1021/acs.est.0c08189
Nightingale, P. D., Malin, G., and Liss, P. S. (1995). Production of chloroform and other low molecular-weight halocarbons by some species of macroalgae. Limnol. Oceanogr. 40, 680–689. doi:10.4319/lo.1995.40.4.0680
Nihei, R., Usami, M., Taguchi, T., and Amachi, S. (2018). Role of fungal laccase in iodide oxidation in soils. J. Environ. Radioact. 189, 127–134. doi:10.1016/j.jenvrad.2018.03.016
Nobukuni, K. (2009). “Chapter 96 - influence of iodine-containing pharmaceuticals on iodine status and thyroid function: iodine-induced hyperthyroidism and hypothyroidism,” in Comprehensive handbook of iodine. Editors V. R. Preedy, G. N. Burrow, and R. Watson (San Diego: Academic Press), 927–935.
Okuda, Y., Hiraiwa, M., Shimizu, N., and Hashimoto, S. (2023). Production of volatile organic iodine compounds by the marine cyanobacterium Calothrix parasitica under different light intensities. Mar. Chem. 248, 104211. doi:10.1016/j.marchem.2023.104211
Pasternak, J. J., and Williamson, E. E. (2012). Clinical pharmacology, uses, and adverse reactions of iodinated contrast agents: a primer for the non-radiologist. Mayo Clin. Proc. 87, 390–402. doi:10.1016/j.mayocp.2012.01.012
Pearce, A. A. (1940). On the so-called "iodide oxidase". Mechanism of iodide oxidation by Aspergillus. Biochem. J. 34, 1493–1500. doi:10.1042/bj0341493
Petrov, S. A., Yusubov, M. S., Beloglazkina, E. K., and Nenajdenko, V. G. (2022). Synthesis of radioiodinated compounds. Classical approaches and achievements of recent years. Class. Approaches Achiev. Recent Years 23, 13789. doi:10.3390/ijms232213789
Punitha, T., Phang, S.-M., Juan, J. C., and Beardall, J. (2018). Environmental control of vanadium haloperoxidases and halocarbon emissions in macroalgae. Mar. Biotechnol. 20, 282–303. doi:10.1007/s10126-018-9820-x
Redeker, K. R., Treseder, K. K., and Allen, M. F. (2004). Ectomycorrhizal fungi: a new source of atmospheric methyl halides? Glob. Change Biol. 10, 1009–1016. doi:10.1111/j.1529-8817.2003.00782.x
Reyes-Umana, V., Henning, Z., Lee, K., Barnum, T. P., and Coates, J. D. (2022). Genetic and phylogenetic analysis of dissimilatory iodate-reducing bacteria identifies potential niches across the world’s oceans. ISME J. 16, 38–49. doi:10.1038/s41396-021-01034-5
Sasamura, S., Ohnuki, T., Kozai, N., and Amachi, S. (2023). Iodate respiration by Azoarcus sp. DN11 and its potential use for removal of radioiodine from contaminated aquifers. Front. Microbiol. 14, 1162788. doi:10.3389/fmicb.2023.1162788
Seki, M., Oikawa, J.-I., Taguchi, T., Ohnuki, T., Muramatsu, Y., Sakamoto, K., et al. (2013). Laccase-catalyzed oxidation of iodide and formation of organically bound iodine in soils. Environ. Sci. Technol. 47, 390–397. doi:10.1021/es303228n
Shim, H. E., Yang, J. E., Jeong, S.-W., Lee, C. H., Song, L., Mushtaq, S., et al. (2018). Silver nanomaterial-immobilized desalination systems for efficient removal of radioactive iodine species in water. Nanomaterials 8, 660. doi:10.3390/nano8090660
Shimada, A., Taniguchi, Y., Kakiuchi, K., Ohira, S., Iida, Y., Sugiyama, T., et al. (2022). Radiochemical analysis of the drain water sampled at the exhaust stack shared by Units 1 and 2 of the Fukushima Daiichi Nuclear Power Station. Sci. Rep. 12, 2086. doi:10.1038/s41598-022-05924-2
Shin, H.-D., Toporek, Y., Mok, J. K., Maekawa, R., Lee, B. D., Howard, M. H., et al. (2022). Iodate reduction by Shewanella oneidensis requires genes encoding an extracellular dimethylsulfoxide reductase. Front. Microbiol. 13, 852942. doi:10.3389/fmicb.2022.852942
Shiroyama, K., Kawasaki, Y., Unno, Y., and Amachi, S. (2015). A putative multicopper oxidase, IoxA, is involved in iodide oxidation by Roseovarius sp. strain A-2. Biosci. Biotechnol. Biochem. 79, 1898–1905. doi:10.1080/09168451.2015.1052767
Sorrenti, S., Baldini, E., Pironi, D., Lauro, A., D’orazi, V., Tartaglia, F., et al. (2021). Iodine: its role in thyroid hormone biosynthesis and beyond. Nutrients 13, 4469. doi:10.3390/nu13124469
Strickland, C. E., Johnson, C. D., Lee, B. D., Qafoku, N. P., Szecsody, J. E., Truex, M. J., et al. (2021). Identification of promising remediation technologies for iodine in the UP-1 operable unit.
Sun, Z., Liang, M., and Chen, J. (2015). Kinetics of iodine-free redox shuttles in dye-sensitized solar cells: interfacial recombination and dye regeneration. Accounts Chem. Res. 48, 1541–1550. doi:10.1021/ar500337g
Suzuki, M., Eda, Y., Ohsawa, S., Kanesaki, Y., Yoshikawa, H., Tanaka, K., et al. (2012). Iodide oxidation by a novel multicopper oxidase from the alphaproteobacterium strain Q-1. Appl. Environ. Microbiol. 78, 3941–3949. doi:10.1128/aem.00084-12
Suzuki, T., Otosaka, S., Kuwabara, J., Kawamura, H., and Kobayashi, T. (2013). Iodine-129 concentration in seawater near Fukushima before and after the accident at the Fukushima daiichi nuclear power plant. Biogeosciences 10, 3839–3847. doi:10.5194/bg-10-3839-2013
Tang, W., Duan, J., Zhang, Y., and Luo, X. (2022). Cross-linked sponge fungal hyphae: an efficient and environmentally friendly sorbent addition of iodine. Biomass Convers. Biorefinery. doi:10.1007/s13399-022-02706-8
Taurog, A., Howells, E. M., and Nachimson, H. I. (1966). Conversion of iodate to iodide in vivo and in vitro. J. Biol. Chem. 241, 4686–4693. doi:10.1016/s0021-9258(18)99701-2
Thorenz, U. R., Carpenter, L. J., Huang, R. J., Kundel, M., Bosle, J., and Hoffmann, T. (2014). Emission of iodine-containing volatiles by selected microalgae species. Atmos. Chem. Phys. 14, 13327–13335. doi:10.5194/acp-14-13327-2014
Toporek, Y. J., Mok, J. K., Shin, H. D., Lee, B. D., Lee, M. H., and Dichristina, T. J. (2019). Metal reduction and protein secretion genes required for iodate reduction by Shewanella oneidensis. Appl. Environ. Microbiol. 85, e02115-18. doi:10.1128/aem.02115-18
Tsunogai, S., and Sase, T. (1969). Formation of iodide-iodine in the ocean. Deep Sea Res. Oceanogr. Abstr. 16, 489–496. doi:10.1016/0011-7471(69)90037-0
Urik, M., Antogka, R., Littera, P., Gardosova, K., Kolencik, M., and Korenkova, L. (2011). Biotransformation and biosorption of se(iv) by aspergillus niger strain. Fresenius Environ. Bull. 20, 3387–3393.
Urik, M., Kramarova, Z., Sevc, J., Cernansky, S., Kalis, M., Medved, J., et al. (2010). Biosorption and bioaccumulation of thallium(I) and its effect on growth of Neosartorya fischeri strain. Pol. J. Environ. Stud. 19, 457–460.
Van Bergeijk, S. A., Hernández, L., Zubía, E., and Cañavate, J. P. (2016). Iodine balance, growth and biochemical composition of three marine microalgae cultured under various inorganic iodine concentrations. Mar. Biol. 163, 107. doi:10.1007/s00227-016-2884-0
Wadley, M. R., Stevens, D. P., Jickells, T. D., Hughes, C., Chance, R., Hepach, H., et al. (2020). A global model for iodine speciation in the upper ocean. Glob. Biogeochem. Cycles 34, e2019GB006467. doi:10.1029/2019GB006467
Xu, S., Freeman, S. P. H. T., Hou, X., Watanabe, A., Yamaguchi, K., and Zhang, L. (2013). Iodine isotopes in precipitation: temporal responses to 129I emissions from the Fukushima nuclear accident. Environ. Sci. Technol. 47, 10851–10859. doi:10.1021/es401527q
Yamazaki, C., Kashiwa, S., Horiuchi, A., Kasahara, Y., Yamamura, S., and Amachi, S. (2020). A novel dimethylsulfoxide reductase family of molybdenum enzyme, Idr, is involved in iodate respiration by Pseudomonas sp. SCT. SCT 22, 2196–2212. doi:10.1111/1462-2920.14988
Yang, J., Tai, W., Wu, F., Shi, K., Jia, T., Su, Y., et al. (2022). Enhanced removal of radioactive iodine anions from wastewater using modified bentonite: experimental and theoretical study. Chemosphere 292, 133401. doi:10.1016/j.chemosphere.2021.133401
Yuliana, T., Ebihara, K., Suzuki, M., Shimonaka, C., and Amachi, S. (2015). A novel enzyme-based antimicrobial system comprising iodide and a multicopper oxidase isolated from Alphaproteobacterium strain Q-1. Appl. Microbiol. Biotechnol. 99, 10011–10018. doi:10.1007/s00253-015-6862-0
Zaruba, S., Bozóová, V., Vishnikin, A. B., Bazeľ, Y. R., Šandrejová, J., Gavazov, K., et al. (2017). Vortex-assisted liquid-liquid microextraction procedure for iodine speciation in water samples. Microchem. J. 132, 59–68. doi:10.1016/j.microc.2017.01.004
Zhang, X., Liu, Y., Zhang, S., Zhang, Z., Feng, Y., Zhang, Y., et al. (2022). Immobilization of iodine waste via moderate temperature sintering of (Ag)iodosodalite. J. Solid State Chem. 316, 123553. doi:10.1016/j.jssc.2022.123553
Zhao, D., Lim, C.-P., Miyanaga, K., and Tanji, Y. (2013). Iodine from bacterial iodide oxidization by Roseovarius spp. inhibits the growth of other bacteria. Appl. Microbiol. Biotechnol. 97, 2173–2182. doi:10.1007/s00253-012-4043-y
Keywords: iodine, bioreduction, biooxidation, biomethylation, bioremediation
Citation: Duborská E, Vojtková H, Matulová M, Šeda M and Matúš P (2023) Microbial involvement in iodine cycle: mechanisms and potential applications. Front. Bioeng. Biotechnol. 11:1279270. doi: 10.3389/fbioe.2023.1279270
Received: 17 August 2023; Accepted: 17 October 2023;
Published: 30 October 2023.
Edited by:
Balaram Mohapatra, Gujarat Biotechnology Research Centre (GBRC), IndiaReviewed by:
Y. V. Nancharaiah, Bhabha Atomic Research Centre (BARC), IndiaSubhasis Das, The Energy and Resources Institute (TERI), India
Copyright © 2023 Duborská, Vojtková, Matulová, Šeda and Matúš. This is an open-access article distributed under the terms of the Creative Commons Attribution License (CC BY). The use, distribution or reproduction in other forums is permitted, provided the original author(s) and the copyright owner(s) are credited and that the original publication in this journal is cited, in accordance with accepted academic practice. No use, distribution or reproduction is permitted which does not comply with these terms.
*Correspondence: Eva Duborská, ZHVib3Jza2EuZXZhQGdtYWlsLmNvbQ==