- 1Graduate School, Inner Mongolia Medical University, Hohhot, Inner Mongolia, China
- 2Clinical Medical Research Center, Inner Mongolia People’s Hospital, Hohhot, Inner Mongolia, China
- 3Inner Mongolia Key Laboratory of Gene Regulation of the Metabolic Disease, Inner Mongolia People’s Hospital, Hohhot, Inner Mongolia, China
- 4Inner Mongolia Academy of Medical Sciences, Inner Mongolia People’s Hospital, Hohhot, Inner Mongolia, China
Cancer has keeping the main threat to the health of human being. Its overall survival rate has shown rare substantial progress in spite of the improving diagnostic and treatment techniques for cancer in recent years. Indeed, such classic strategies for malignant tumor as surgery, radiation and chemotherapy have been developed and bring more hope to the patients, but still been accompanied by certain limitations, which include the challenge of managing large wound sizes, systemic toxic side effects, and harmful to the healthy tissues caused by imprecise alignment with tumors in radiotherapy. Furthermore, immunotherapy exhibits a limited therapeutic effect in advanced tumors which is reported only up to 25%–30%. The combination of nanomaterials and cancer treatment offers new hope for cancer patients, demonstrating strong potential in the field of medical research. Among the extensively utilized nanomaterials, calcium carbonate nanomaterials (CCNM) exhibit a broad spectrum of biomedical applications due to their abundant availability, cost-effectiveness, and exceptional safety profile. CCNM have the potential to elevate intracellular Ca2+ levels in tumor cells, trigger the mitochondrial damage and ultimately lead to tumor cell death. Moreover, compared with other types of nanomaterials, CCNM exhibit remarkable advantages as delivery systems owing to their high loading capacity, biocompatibility and biodegradability. The purpose of this review is to provide an overview of CCNM synthesis, focusing on summarizing its diverse roles in cancer treatment and the benefits and challenges associated with CCNM in cancer therapy. Hoping to present the significance of CCNM as for the clinical application, and summarize information for the design of CCNM and other types of nanomaterials in the future.
1 Introduction
Cancer is the second leading cause of mortality worldwide (Sung et al., 2021). According to data of 2020, there were 19.29 million new cancer cases worldwide, including 10.6 million males and 9.23 million females. Respectively, 5.53 million males and 4.43 million females die from cancer worldwide (Xia et al., 2022). Consequently, cancer has imposed a substantial economic burden on global healthcare systems. Surgery, chemotherapy and radiotherapy have been the classical cancer treatment. Surgery is the most efficient way to remove the solid tumor, however, incomplete resection and the possibility of helping the cancer cell metastasis during resection are the unavoidable problems (Curtis et al., 2020; Yamaguchi et al., 2021). Chemotherapy and radiotherapy bring fatal damage not only to the cancer cells, but the normal cells, let alone the drug resistance and the immunosuppression which is caused by the bone marrow damage (Behranvand et al., 2022). Furthermore, radiotherapy itself could cause cell unknown mutations and the metastasis (Ruysscher et al., 2019). In recent years, the emerging immunotherapy has received widespread attention due to its reasonable theory and the optimistic effects; whereas, the percentage of the patients who could benefit from it is comparatively low because of the significant heterogeneity of cancer (Liu et al., 2021). Nanomaterials have played an active role in the biomedical field, especially in cancer treatment (Kashyap et al., 2023; Pei et al., 2023). The inherent characteristics of the nanomaterial, such as particle size, atomic composition, magnetic and electronic specificity confer the nanoparticles with unparalleled advantages in treating diseases, particularly in targeting therapy for cancer (Wang et al., 2023a). By modifying nanoparticles in physical, chemical, and biological activity aspects, their dissolution is promoted, absorption is improved, and delivery efficiency is enhanced (Zhu and Li, 2023). The use of nanoparticles to control the delivery and release of anticancer drugs has become a hot topic in the research of nanocarrier drugs (Yang et al., 2022). Among numerous nanomaterials, calcium carbonate nanomaterials (CCNM) have distinctive properties: good biocompatibility, easily synthesized, and could be produced in diverse forms and crystal structures (Luo et al., 2020; Li et al., 2021a).
CCNM are widely used in various industries such as food packaging, pharmaceuticals, paint pigments, and polymer fillers (Shou et al., 2022). Meanwhile, it shows great potential in biomedicine, environmental remediation and energy production, etc. (Figure 1). In biomedicine field, CCNM can be used in treating cancer, disease detection and bone regeneration (Vikulina et al., 2021). Due to the biocompatibility and high specific surface area of CCNM, they possess the remarkable advantages in drug delivery (Mao et al., 2021). CCNM could provide Ca2+, attack mitochondria, and further kill malignant cells in cancer therapy (Bai et al., 2022).
In this review, we first reviewed the main synthesis methods of CCNM, followed by summarizing their different functions in cancer treatment, including drug carriers, synergistic therapy, Ca2+ overload therapy, etc. Finally, we delineated the merits and challenges of CCNM in the context of cancer therapy. Hoping to present the significance of CCNM as for the clinical application, and provide some clues for the novel design of CCNM and other types of nanomaterials in the future.
2 Synthesis of CCNM
There are currently various methods for synthesizing CCNM. According to different synthesis techniques, various shapes and sizes of particles can be obtained (Wu et al., 2022). All of the synthesis parameters, including reactant concentration, stirring strength of the reaction mixture, temperature, and solvent type, affect the crystal formation, particle size, and stability of CCNM (Li et al., 2020a; Devi et al., 2023). The main synthesis methods of CCNM, such as precipitation method (Febrida et al., 2021), mechano-chemical method (Preksha et al., 2021), sea shell and eggshell mediated (Azraian and Sutapun, 2022) and gas diffusion method (Gindele et al., 2021) are summarized as follows Table 1.
2.1 Precipitation method
Spontaneous precipitation reaction is that the mixture of calcium and supersaturation solution of carbonate are simply blended. It is the most important and simplest method to prepare CCNM (Sun et al., 2020). Persano et al. (2022) synthesized CCNM using this method and studied in detail the effects of different synthesis parameters. They found that as the stirring speed increased, the nano size of CaCO3 decreased correspondingly. Meanwhile, it was found that the higher the salt concentration, the higher the supersaturation became, and thus the smaller the nano size. Yang et al. (2021a) used CaCl2 and NaCO3 as raw materials, injected NaCO3 into the CaCl2 containing Ca(OH)2 to prepare CCNM, and studied the use of Ca(OH)2 as an additive. As a non-impurity additive, Ca(OH)2 increased the pH value of the suspension. The results showed that the higher the pH value of the reaction system, the smaller the CCNM size. Pérez-Villarejo et al. (2018) reported a simple and rapid method for synthesizing CCNM. The experiment used Ca(NO3)2·4H2O and NaHCO3 as raw materials and mainly observed the role of sucrose as an additive in the synthesis of CCNM. Their conclusion is that sucrose interacts with Ca2+ and water molecules through hydroxyl groups. The higher the concentration of sucrose, the more crystalline forms of synthetic vaterite, and the smaller the size of nanoparticles. Sucrose can stabilize the vaterite crystal form, making it difficult to transform into a more stable calcite crystal form, which provides a fast and simple method for the synthesis of vaterite crystal form in the future.
Based on the application experience, the precipitation method has been reported to involve the addition of different molecules, including synthetic polymers, surfactants and biomolecules, to synthesize CCNM of different sizes and shapes. This method can adjust the crystal morphology and particle size of CCNM by changing the concentration of reactants and adding organic matter. However, the rapid reaction speed of precipitation reaction, which is not conducive to observing and studying the reaction process. This might be its main drawback.
2.2 Mechano-chemical method
The mechano-chemical synthesis method to produce CCNM is the process involving mechanical fragmentation and chemical reactions (Cestari et al., 2021). Its chemical reaction occurs through the absorption of mechanical energy by reactants. This method is divided into the dry mechano-chemical and the wet mechano-chemical method (Mkhize et al., 2021). Gbadenyan et al. (2021) prepared CCNM by mechano-chemical grinding method. They started with using stainless steel balls and cans to grind the snail shell to obtain fine particles. Then, the fine particles are placed in a stainless steel wide-mouthed bottle, solvent is added, and wet grinding is performed to obtain CCNM. Compared with precipitation method, the equipment setup for the mechano-chemical method is cost-effective and enables the agglomeration-free production of nanoparticles with narrow particle size distribution (Piras et al., 2019). But this method also has some drawbacks, such as instrument rusting, which may hinder the synthesis process of nanoparticles and cause certain pollution.
2.3 Sea shell and eggshell mediated
It is advantageous to synthesize CCNM using natural reserves of CaCO3, such as eggshells and seashells, as it is easy to obtain, non-toxic, and biocompatible, making it an ideal candidate for biomedical applications (Prihanto et al., 2023). Rinu et al. (2020) synthesized nanoparticles using eggshells as raw materials. By collecting a large amount of eggshells and drying them at room temperature. Then gently crush the eggshell and place it in a crucible, keeping it in a muffle furnace at 900°C for 2 h. Finally, it is turned into fine powder to synthesize CCNM. Khan et al. (2019) used eggshells and agar as raw materials to calcine the synthesized products at high temperatures, ultimately synthesizing CCNM. The calcination temperature has a significant impact on the morphology, composition, and size of particles. Hussein et al. (2020) crushed the shells into powder and mixed them with HCl to form CaCl2. And the effect of different volumes of double-distilled water (DDW) on the morphology of CCNM derived from shells was studied. Nanoparticles prepared with different volumes of DDW have different shapes and sizes, which is due to the dilution of the solution volume by DDW, resulting in changes in the activity space of the nanoparticles.
Seashells and eggshells are abundant in resources, inexpensive, and easy to obtain, which can reduce environmental pollution levels and have good economic and social benefits. From this, it can be seen that CCNM obtained through shells and eggshells has enormous advantages, but the resulting nanoparticles are impure, which requires further exploration.
2.4 Gas diffusion method
The gas diffusion method has been widely used to synthesize CCNM in the biomedical field. This method involves the thermal decomposition of (NH4)2CO3 or NH4HCO3 to generate CO2 and NH3 diffusion into an ethanol solution containing calcium salts to generate precipitates or the introduction of CO2 gas into the calcium salt ethanol solution at atmospheric pressure to synthesize CCNM (Wang et al., 2020). Chuzeville et al. (2022) used this method to synthesize the dispersed CCNM below 150 nm in ethanol. Ethanol is the main solvent to ultimately obtain stable and uniform spherical nanoparticles, which can inhibit the spontaneous aggregation and crystallization of CCNM. Ju et al. (2022) made some improvements on the basis of the original steps. In the ethanol-water binary system, stable amorphous calcium carbonate (ACC) nanospheres were synthesized by gas diffusion method. And different volumes of ammonia were added to the solution, it was observed that as ammonia was continuously added, the volume of the nanomaterials continued to decrease. Xu et al. (2022a) synthesized ACC by gas diffusion method, added Ca(OH)2 and CO2 in the methanol-water system, reacted in an autoclave and centrifuged to obtain ACC. The research results indicate that with the increase of water content in the solution system, ACC gradually changed into a metastable vaterite crystal.
Compared to other synthesis methods, gas-phase diffusion method has the advantage of controllable reaction speed. There is no need for other additives, and higher product quality could be acquired. Thus, it is often used as the preferred method for studying the biomimetic synthesis of CCNM minerals. But the obtained nanoparticles are still easy to agglomerate. Polyethylene glycol and other substances can be used to modify the surface of CCNM to improve the dispersion and stability in aqueous solution.
3 Application of CCNM in cancer treatment
CCNM not only have great potential in imaging and biosensing but also play an important role in fields such as dental materials and bone regeneration (Li et al., 2021b; Niu et al., 2022). Due to their excellent biocompatibility and pH responsiveness, CCNM have great developing prospects in cancer treatment, especially in delivery systems, tumor diagnosis, Ca2+ overload, pH regulation, and coagulation induction (Novoselova et al., 2021; Zhao et al., 2023) (Figure 2). The different roles of CCNM in cancer treatment are listed in Table 2.
3.1 CCNM as carriers for delivering anticancer drugs
An ideal anticancer drug delivery system is to deliver the drug directly to the target site, with minimal impact on normal cells, thereby improving treatment efficiency and producing minimal toxic side effects. Numerous tumor targeted drug delivery systems have been constructed through nanotechnology, which not only improves drug stability but also limits drug toxicity (Elbaz et al., 2020). CCNM have received great attention among different inorganic nanocarriers. Their natural characteristics such as biocompatibility, pH responsiveness and high encapsulation efficiency make them an ideal carrier for transporting various bioactive substances, especially anticancer drugs and genes (Fu et al., 2019). Therefore, when CCNM are coupled with drugs and genes, they improve the efficiency of disease treatment.
At present, there are two main ways for CCNM to deliver drugs for tumor treatment. One method involves directly binding anti-tumor drugs onto the surface of CCNM, while the other method involves co-doping drugs with CCNM and allowing the complex to enter tumor cells (Teixeira et al., 2022). Zheng et al. (2022) used the one-pot gas diffusion method to co-dope curcumin (CUR) with CCNM, and the obtained CCNM can decompose at low pH to release CUR. Among them, CUR causes cell apoptosis by affecting Ca2+ homeostasis. By using CCNM as a carrier to transport CUR, the programmed release of CUR at the tumor site was achieved, improving the transport efficiency and anticancer activity of CUR.
Popova et al. (2021) loaded DOX onto the surface of CCNM, with the aim of studying the coupling efficiency of CCNM with drugs and the effectiveness of carrying DOX in inhibiting cancer cell proliferation in vitro. In an in vitro model, the composite material of CCNM and DOX was shown to effectively inhibit the growth of cancer cells. The characteristics of CCNM also help prevent unnecessary accumulation of DOX in major organs such as the liver, heart, and kidneys.
On this basis, drug delivery systems can be designed to combine multiple treatment methods into an intelligent carrier, providing a solution for their potential applications in cancer diagnosis and treatment. As shown in Figure 3A, Yu et al. (2023) co-doped CCNM with indocyanine green (IGG) before loading DOX on the surface, then encapsulated them with poly (lactic-co-glycolic acid)-ss-chondroitin sulfate A (PSC). This study combines photothermal therapy (PTT) with chemotherapy to treat tumors. PTT can use photosensitizers to generate heat energy and ultimately eliminate tumor cells. IGG is an effective photosensitizer, but free IGG cannot selectively reach the tumor site. Utilizing CCNM delivered IGG to reach the tumor site for PTT. This method not only enables IGG to accurately reach tumor cells but also significantly increases the metabolic time of IGG and improves treatment efficiency. As shown in Figure 3B, compared with other control groups, the experimental group significantly inhibited the tumor volume of mice. It was observed in the mouse thermal image in Figure 3C that this nanomaterial helps IGG to better exert its therapeutic effect. Observing from the picture, the tumor temperature in the 5% glucose group was slightly increased after 5 min of laser irradiation. The free DOX + ICG group exhibited the tumor temperature with an increase to 45.4°C. Notably, PSC/ICG@ and PSC/ICG@+DOX groups showed an increased temperature of 60.5°C after 5 min irradiation, which could effectively generate hyperthermia for PTT of malignant tumors. From this, it can be seen that CCNM have enormous advantages in delivery systems. At the same time, we can further study its synthesis method and functionalization to generate CCNM with different morphologies, thereby achieving higher loading efficiency and achieving better therapeutic effects.
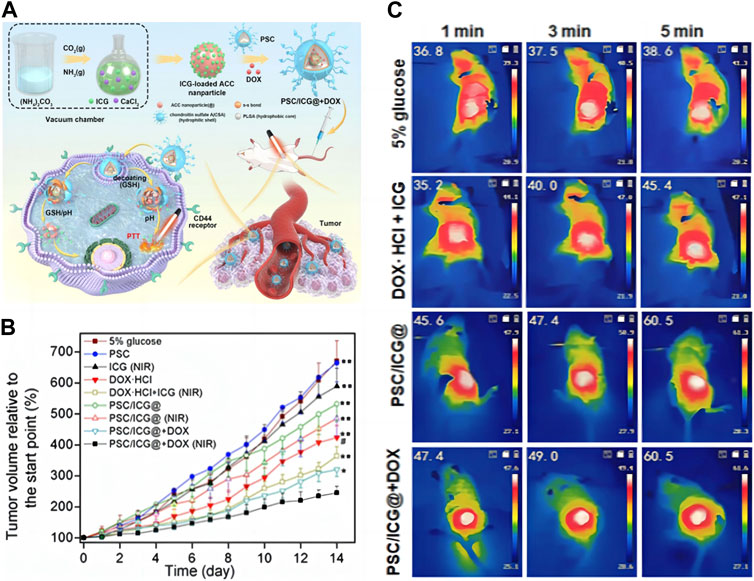
FIGURE 3. CCNM are loaded with active substances to treat tumors: (A) Schematic diagram of PSC/ICG@+DOX nanoparticle synthesis and mediated apoptosis; (B) Tumor growth curves of mice after the treatment; (C) Laser imaging of mice (Yu et al., 2023). Copyright 2023 Dove Medical Press Limited.
3.2 Ca2+ overload
As a pivotal second messenger in cellular signaling, Ca2+ orchestrates the precise regulation of diverse physiological processes through targeted activation of specific proteins (Liu et al., 2020c). Under normal circumstances, the concentration of cytoplasmic free Ca2+ is maintained at a significantly low level of approximately 100 nmol/L. There is a 104 ∼ 105 -fold gradient of Ca2+ concentration between outside and inside of cells (Bai et al., 2022). Without the stimulation of abnormal conditions, the intracellular Ca2+ concentration is lower than the level of extracellular fluid, which plays an active role in regulating cells (Ma et al., 2020; Eisner et al., 2023). But if the concentration of Ca2+ exceeds the normal range, it will cause damage to the cells. Therefore, regulating Ca2+ concentration has broad research prospects in tumor treatment. In recent years, Ca2+ overload treatment has garnered significant attention in the field of tumor treatment, with a close association between Ca2+ and mitochondria (Zhu et al., 2019). When intracellular Ca2+ overload occurs, the mitochondrial Ca2+ uniporter (MCU) sequesters intracellular Ca2+. Upon reaching a certain threshold, this uptake of Ca2+ by mitochondria triggers the opening of the mitochondrial permeability transition pore (PTP) (Glitsch, 2019; Delierneux et al., 2020). When PTP is opened, many large molecules non-selectively diffuse from cytoplasm to mitochondria, leading to the destruction and dysfunction of mitochondrial membrane potential. Mitochondrial Ca2+ overload can lead to the release of cytochrome C and the activation of aspartate proteolytic enzymes, thereby damaging the production of reactive oxygen species related to the respiratory chain and affecting mitochondrial function, ultimately leading to cell apoptosis (Calvo-Rodriguez and Bacskai, 2021). After mitochondrial damage, biological transmission electron microscopy (Bio-TEM) is commonly employed to examine the morphology and abundance of mitochondria. At the same time, Flou-4 AM calcium probe is commonly used to detect intracellular Ca2+ (Zheng et al., 2021), while the Rhod-2 AM probe is used for detecting Ca2+ in mitochondria (Wang et al., 2023b).
Inadequate Ca2+ at the tumor site, intracellular Ca2+ channels can also effectively regulate the concentration of Ca2+, so it is difficult to achieve effective Ca2+ overload. Providing Ca2+ solely through calcium materials cannot achieve satisfactory results (Kong et al., 2021). Therefore, more and more collaborative treatment methods have been developed and have attracted widespread attention. For example, combining calcium nanomaterials with treatment methods such as chemotherapy can not only provide Ca2+, but also disrupt calcium homeostasis, thereby achieving more effective Ca2+ overload. As shown in Figure 4A, Li et al. (2021c) designed the M@CaCO3@KAE nano platform, thus realizing the cooperative and targeted therapy of tumors. Among them, Kaempferol-3-O-Rutinoside (KAE) can effectively disrupt the regulation of Ca2+ homeostasis and promote Ca2+ influx, while CCNM can provide Ca2+, ultimately achieving Ca2+ overload and mitochondrial structure and function are disrupted, causing cytoskeletal collapse and oxidative stress, leading to cancer cell apoptosis. In Figures 4B, C, it was observed that the tumor volume of mice was effectively suppressed, indicating that this nanomaterial can to some extent inhibit tumor growth, which is of great significance for clinical applications. This experiment cleverly combines KAE with CaCO3, greatly increasing the concentration of intracellular Ca2+, thereby achieving more efficient treatment of tumors with Ca2+ overload, providing a unique and novel approach for our future Ca2+ overload experiments. In addition, as shown in Figure 4D, Zheng et al. (2021) successfully prepared CCNM codoped with cisplatin (CDDP) and CUR. They destroyed the structure and function of mitochondria through CDDP, and destroyed the endoplasmic reticulum through CUR, so that a large amount of Ca2+ entered the cytoplasm, and CaCO3 provided a large amount of Ca2+, thus achieving efficient Ca2+ overload. Due to the fluorescence characteristics of CUR, it was observed in Figure 4E that the fluorescence signal of the mouse tumor site became stronger within the first 12 h, further indicating that the CCNM nano platform has a certain degree of targeting and can accumulate within the tumor. The Ca2+ nanomodulator designed in this experiment achieves Ca2+ overload in multiple directions, targeting cancer through mitochondria, and has great prospects in clinical practice. Ca2+ overload is one of the most effective methods to induce cancer cell apoptosis. Upregulation of Ca2+ concentration in mitochondria can lead to a series of mitochondrial diseases, including decreased mitochondrial membrane potential, decreased ATP levels, altered mitochondrial morphology, and mitochondrial respiratory disorders (Guan et al., 2020). The use of ion interference to treat tumors is extremely ingenious in concept, providing a new perspective for tumor treatment. Ca2+ overload has gradually become a research hotspot, but simply killing tumor cells through Ca2+ overload is far from enough. We can use other methods for combined therapy to greatly improve the treatment efficiency of tumors.
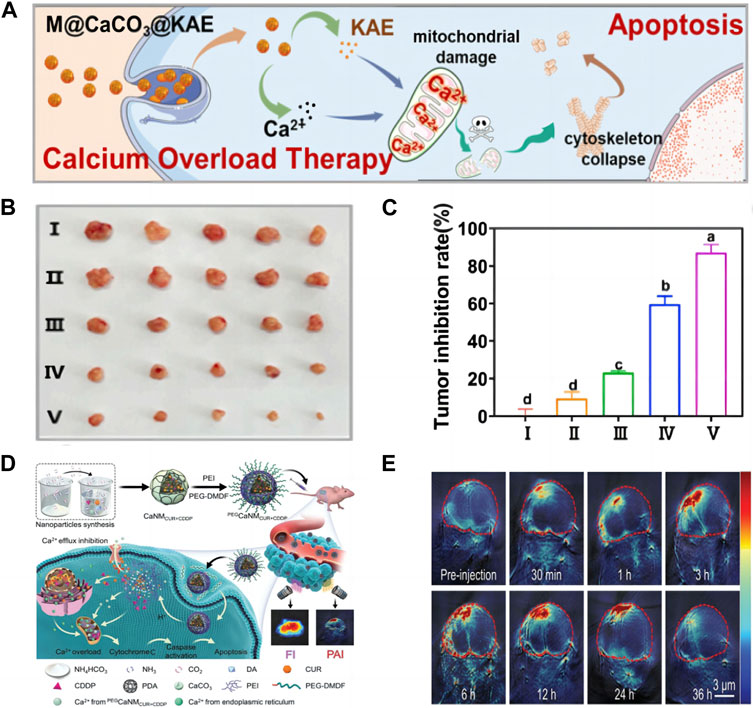
FIGURE 4. (A) Schematic illustration of M@CaCO3@KAE NP-mediated apoptosis; (B) The photos of tumors; (C) The inhibition rate of tumor weights I: Control; II: CaCO3 NPs; III: KAE; Ⅳ: CaCO3@KAE NPs; Ⅴ: M@CaCO3@KAE NPs (Li et al., 2021c) Copyright 2021 Elsevier; (D) Schematic diagram of cell apoptosis mediated by PEGCaNMCUR+CDDP nanoparticles; (E) PA imaging of mice (Zheng et al., 2021). Copyright 2021 WILEY-VCH Verlag GmbH & Co. KGaA, Weinheim.
3.3 CCNM modulate tumor pH
Due to metabolic disorders in tumors, the extracellular pH of solid tumors is lower than that of normal tissues. The acidic extracellular environment of tumors enhances their invasiveness and metastasis, but there are few methods to selectively regulate the extracellular pH environment of tumors. It is impractical and non-selective to flush biological system instantaneously with alkaline liquid or proton pump inhibitor (Worsley et al., 2022). Among various methods for regulating the acidic environment of tumor cells, CCNM are particularly effective because they have efficient buffering ability and can directly regulate pH values.
On this basis, Som et al. (2016) prepare monodisperse CCNM with a size range of 20–300 nm, intentionally adjusting the pH value of the tumor microenvironment (TME). It utilized a mouse cancer model and repeated daily injections of CCNM, which significantly inhibited tumor growth. And in detecting dynamic pH values in tumor mice, it was observed that the pH value increased almost linearly during the first 30 min. This experiment demonstrates that CCNM can increase the pH value of tumors and inhibit tumor growth over time in tumors. It demonstrated for the first time the ability of using CCNM to regulate the pH value in solid tumors and observed that the effective alkalization of tumor acidic pH depends on the size of the nanoparticles. It should be noted that in order to achieve sustained tumor suppression effects in future experiments, it is necessary to optimize the dosage and use CCNM in combination with other therapies to improve the targeting of CCNM to tumors. Lam et al. (2021) co incubated cancer cells and fibroblasts and then treated them with CCNM. The results showed that nanoparticles selectively inhibited the growth of tumor cells, and reduced the cell migration of these cells, but had no effect on fibroblasts. This indicates that CCNM can limit the invasiveness of tumor cells without affecting the growth and behavior of surrounding stromal cells. The advantage of this experiment compared to other experiments is that it uses microfluidic devices to simulate the flow parameters in the body to determine the impact of pH changes on tumor survival and migration. Research has shown that CCNM can indeed alter the acidic microenvironment of tumors, but relying solely on CCNM to alter the acidic environment is still not enough.
3.4 CCNM induce coagulation
To remedy the intrinsic deficits in energy production, cancer cells typically increase their uptake of extracellular glucose. Thus, the glucose deprivation is an effective way to cause the rapid and massive death of cancer cells, which is the essence of starving cancer cells. Based on the concept of cancer hunger treatment, as shown in Figure 5A, Li et al. (2020b) explored the effect of CCNM on coagulation. In Figure 5B, it was observed that in a mouse breast tumor model, CCNM were injected locally and physiological saline was used as the control. Through naked eye, HE, and Masson trichrome staining, it was found that CCNM, under the influence of acid stimulation, can cause coagulation reactions and thrombosis in tumor blood vessels, resulting in abnormal blood circulation and glucose supply. Therefore, CCNM have great prospects in the field of cancer treatment. Among them, Ca2+ activates prothrombin to thrombin, thereby causing blood to coagulate. And as the pH decreases, CCNM release more Ca2+, causing faster blood coagulation. The characteristic of CCNM that are pH sensitive and induce blood coagulation makes them a promising material for cancer hunger treatment. Although this study demonstrates that CCNM can induce coagulation in tumor blood vessels, it still cannot cover all tumor blood vessels, which requires further exploration.
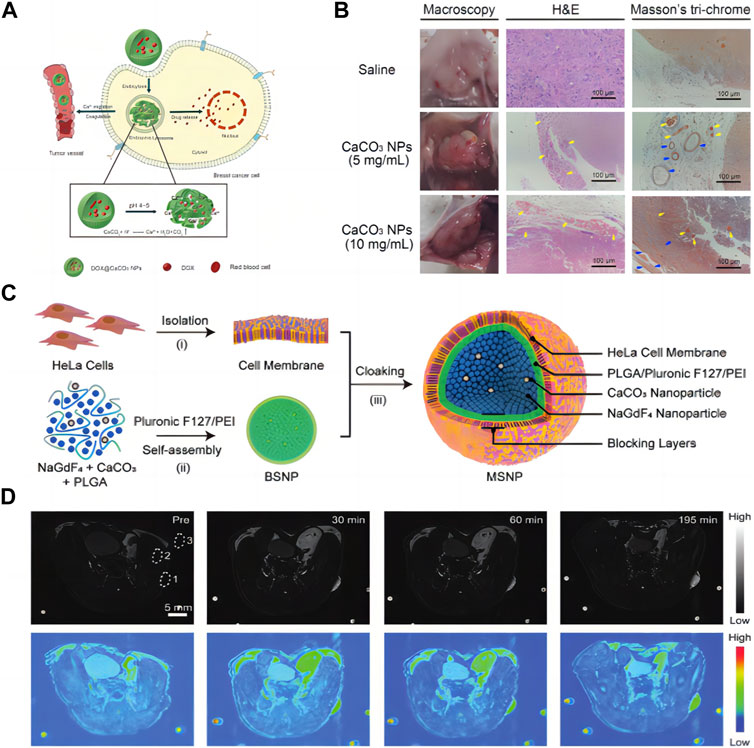
FIGURE 5. (A) CCNM induces blood coagulation; (B) Observation of blood coagulation in vivo (Li et al., 2020b) Copyright 2020 Royal Society of Chemistry; (C) Schematic diagram of synthesis of nanomaterials; (D) MRI of mice (Yi et al., 2019). Copyright 2019 WILEY-VCH Verlag GmbH & Co. KGaA, Weinheim.
3.5 Application of CCNM in tumor diagnosis
In recent years, the development of high-performance contrast agents in magnetic resonance imaging (MRI) has received great attention. As shown in Figure 5C, Yi et al. (2019) designed and synthesized a new type of nanoparticle contrast agent, including self-assembled NaGdF4 and CaCO3 nanoconjugates, providing an integrated MRI nano platform with high tumor selectivity and good biocompatibility. In this design, the spatial confinement of the Gd3+ leads to an “OFF” MRI signal due to insufficient interaction between the protons and the crystal lattices. However, when immersed in the mildly acidic TME the embedded CCNM generate CO2 bubbles and subsequently disconnect the nanoconjugates, thus resulting in an “ON” MRI signal. Due to the excellent performance of nanomaterials in vitro, Yi et al. (2019) investigated their comparative ability in vivo (Figure 5D). T1-weighted MR images were acquired in vivo before and after intravenous injection of nanoconjugates. The tumor site is relatively dark before injection of nanoconjugates, and it starts to light up about 30 min after injection. The contrast enhancement at the tumor site reached a tumor-to-background ratio of approximately 48 at 195 min postinjection. Compared with commonly used magnetic contrast agents, the in vivo performance of NaGdF4-CaCO3 shows a 60-fold enhancement in tumor visualization. It is suitable for deep tissue imaging, with higher sensitivity and selectivity, which is of great significance for constructing intelligent magnetic resonance imaging.
In addition, CCNM can generate CO2 bubbles under acidic conditions, which can then enhance ultrasound imaging signals. Huang et al. (2020) developed a diagnostic nanoparticle system for ultrasound and fluorescence dual-mode imaging. The nanoparticle loaded with DOX showed the ability to simultaneously treat cancer. Therefore, loading DOX onto CCNM yields CaCO3-DOX nanoparticles. Under acidic conditions, CCNM generate CO2 to enhance ultrasound imaging and increase the release of DOX, thereby improving the quality of ultrasound imaging. Also, ICG was encapsulated into CCNM, to further detect the tumor with fluorescence. Overall, this study integrates imaging and therapeutic functions to provide a promising and attractive strategy for cancer treatment. Through research, it has been found that CCNM have certain help and advantages in the diagnosis of tumors. But there are still shortcomings such as instability and weak targeting of CCNM. It is necessary to improve their targeting and stability in order to maximize the diagnostic role of CCNM in tumors. Meanwhile, Vidallon et al. (2020) synthesized hybrid CCNM with biocompatibility and pH sensitivity through rapid precipitation method. Among them, CCNM can undergo decomposition under acidic conditions to generate high echo CO2 bubbles, increasing the contrast intensity of ultrasound and demonstrating its potential as an ultrasound contrast agent.
Compared to traditional ultrasound contrast agents, CCNM not only achieve long-term stability of ultrasound imaging but also can quickly clear in the body without causing harm to the human body (Ma et al., 2022). At the same time, appropriate surface modification can also endow particles with specific targeting. The mesoporous structure generated by CCNM polymerization can encapsulate therapeutic drugs such as chemotherapy and sound sensitizers with good drug loading and release performance (Qiao et al., 2020). Therefore, combining the diagnosis and treatment functions of CCNM play an important role in improving the diagnosis and treatment technology of tumors.
4 Advantages of CCNM
Now the enormous potential of CCNM have gradually been discovered in the field of biomedicine. CCNM not only have unique characteristics such as good biocompatibility and low cost but also have a large specific surface area and are easy to functionalize on the surface (Ren et al., 2023; Torgbo et al., 2023). Therefore, CCNM play a huge advantage in delivery systems. In addition, the acid responsive properties of CCNM have great application value in passive and active targeting of cancer cells and have received widespread attention in cancer treatment, bringing new hope to cancer treatment (Wang et al., 2022).
4.1 Acid sensitivity
The acid sensitivity of CCNM make them particularly attractive in cancer treatment because micro acidity is a characteristic of TME (Lu et al., 2021). CaCO3 can be dissolved into non-toxic products (Ca2+, CO32−) in some acidic environments, which has been used as a drug delivery system (Zhao et al., 2022b; Chiang et al., 2022). Xu et al. (2022a) loaded anticancer drugs 5-fluorouracil (5-FU) and CUR with CCNM as carriers and placed the drug-loaded CCNM in phosphate buffers (pH 5.8, 6.6, 7.4) to observe the pH-dependent release of drugs. It was found that the release of 5-FU increased with the decrease of pH value, and the results showed that CCNM had good pH control drug release performance. Therefore, CCNM have great potential in acid responsive drug delivery.
4.2 Biocompatibility
The biosafety of nanoparticles is crucial in biomedical applications. The reduction in particle size and larger surface area makes them better absorbed by body fluids and tissues, thereby increasing their toxicity (Dong et al., 2022). Unlike other nanoparticles, CCNM have natural biocompatibility because Ca2+ and CO32− ions are already widely present in the human body and have not shown cytotoxic effects in normal cell and in vivo studies (Yang et al., 2021b). Li et al. (2020b) observed through the use of rabbit red blood cells that CCNM did not cause any cell aggregation or dissolution, indicating that it has good blood compatibility.
4.3 High load capacity
The relatively large specific surface area of CCNM gives them high loading capacity. At the same time, the high porosity and well-developed internal structure of CCNM allow for the accommodation of molecules with different properties (Atchuda et al., 2022). These particles can effectively capture various bioactive substances, including low molecular weight compounds and large molecules. These bioactive substances are loaded onto CaCO3 through physical adsorption into pores or co precipitation during particle formation, which is very important in delivery systems (Kanwal et al., 2022).
4.4 Low cost and easy synthesis
Due to high costs and potential material safety issues, new nano formulations used for targeted drug delivery are significantly hindered when transitioning from the laboratory to the clinical setting. CaCO3 is the most abundant mineral in nature, its production cost is significantly lower than that of other nanoparticles, making it a cheap inorganic material (Kaiping et al., 2023). Therefore, CCNM are an extremely important material in the biomedical field. At the same time, compared with other nanomaterials, the preparation of CCNM only requires the use of basic salts (calcium salts and carbonates) and often does not require the use of organic solvents. This simple manufacturing process reduces the cost of CCNM (Trushina et al., 2022).
4.5 Easy functionalization
Easy functionalization is also one of the huge advantages of CCNM. Many studies have functionalized it through dry, wet, and in-situ modifications to enhance stability and targeting (Han et al., 2019). Due to its small particle size, high specific surface area, and high specific surface energy, CCNM are prone to particle aggregation and agglomeration during preparation and post-treatment, resulting in the formation of secondary particles, increasing particle size and losing the functionality of ultrafine particles during final use, thereby affecting practical application results (Lee et al., 2019; Ippolito et al., 2020). The key to solving this problem lies in the surface modification of CCNM, reducing the adhesion between particles, and improving its dispersibility and dispersion stability in the matrix (Li et al., 2019; Shen et al., 2019). Meanwhile, CCNM still cannot achieve precisely targeted therapy solely relying on passive targeting and acid responsiveness. Therefore, it is possible to endow the delivery system with targeting ability by coupling CCNM to targeted molecules. After the functionalization of CCNM, its in vivo stability in the blood will be higher, avoiding macrophage capture and possessing certain targeting characteristics, allowing the carrier to accumulate at specific sites (Dang et al., 2019). Li. et al. (2021d) developed a multifunctional CCNM for the targeted treatment of breast cancer and inhibition of metastasis. The CCNM were functionalized with folic acid molecules (FA) and coated with the T-cell membrane. Within the acidic TME, the CCNM undergo decomposition, leading to the release of Ca2+ ions and FA. The liberated FA molecules selectively bind to cancer cells via folate receptors and effectively suppress cancer cell migration, invasion, and proliferation. Notably, the incorporation of T cell membranes imparts tumor-targeting capabilities to the material while also preventing immune clearance within the body. This gives CCNM a prominent advantage in drug delivery systems for cancer treatment.
5 The challenges and recommendations for future studies of CCNM
CCNM have been developed, and their biocompatibility, pH responsiveness, and simple preparation have good prospects in the field of tumor treatment. However, the challenges faced in treatment cannot be ignored.
5.1 Therapeutic effect of CCNM
In normal individuals, there is also a certain amount of Ca2+, so it is difficult to achieve effective therapeutic effects. Moreover, the Ca2+ channel/pump on the cell membrane has a strong regulatory function, which makes the cell Ca2+ overload quickly return to the normal level, resulting in poor anti-cancer effects (Marchi et al., 2020; Tinawi, 2021). Therefore, combination therapy can further increase the Ca2+ content to achieve effective therapeutic concentration. In recent years, drug delivery systems constructed using CCNM biomaterials have been widely studied, but the concentration of drugs delivered is limited and cannot achieve clinically effective concentrations, which cannot achieve the desired clinical effects. Some studies have shown that various molecular adjuvants, such as biopolymers or synthetic polymers, can be added to the CCNM to load more drugs and make the drug release more slowly (Zhou et al., 2023).
5.2 Biosafety of CCNM
Ca2+ is an element contained in the human body, which has good biocompatibility and biodegradability (Wang et al., 2021). However, when the Ca2+ content exceeds the limit, the effect of the Ca2+ steady-state regulation system is not very clear. Meanwhile, the inevitable leakage of Ca2+ in body fluids is a long-term issue for nanomedicine delivery systems (Bai et al., 2021). Excessive Ca2+ can trigger coagulation reactions, leading to the formation of blood clots and posing a threat to organisms (Wang et al., 2021). Moreover, a substantial number of studies solely assess the short-term toxicity of CCNM in mice by delineating organ damage subsequent to CCNM injection, which still poses challenges for accurately evaluating the biosafety of CCNM. So it is imperative to acknowledge the long-term potential risks associated with CCNM. Therefore, in order to facilitate the clinical translation of CCNM, it is imperative to conduct a comprehensive evaluation of the long-term effects of CCNM across various animal models, ranging from rodents to mammals.
5.3 Clinical research on CCNM
CCNM have been widely studied in the field of tumor diagnosis and treatment, but there are still some challenges before clinical transformation (Dong et al., 2020). The present preparation processes of CCNM are instable, which easily leads to large particles (Vavaev et al., 2022). Hence, it is imperative to devise precise techniques enabling control over size, composition, and surface modification in order to facilitate large-scale production of CCNM. In addition, the prediction of drug release kinetics for CCNM remain challenging. Despite extensive research on the pH sensitivity of CCNM, their release behavior under normal physiological conditions has not been comprehensively evaluated.
6 Conclusion
We have summarized the different roles of CCNM in treating cancer based on the synthesis methods and advantages of CCNM. Among all of the producing methods of CCNM, gas diffusion method is mostly accepted in the medical research field. How to create simpler, more effective methods to produce CCNM with wanted size, crystal forms, and morphologies has been still the research focus as for the drug delivery system in treating cancer; plus, large-scale and controllable industrial production methods to further reduce the cost of CCNM and obtain carriers with higher targeting and drug loading are needed to further explore in the future. It is the characteristics of high loading efficiency and acid responsiveness to TME that making us give more attention to CCNM than other nanomaterial. Actually, Ca2+ itself is the universal ion in the cell, functions in nearly all aspects of the living activity, thus, Ca2+ overload in certain cells as cancer cells would do no harm to other normal cells if the perfect precision are achieved. On the other hand, CCNM are the drug delivering carrier per se, which can carry whether the chemotherapy agents or novel targeting drugs, even gene editing tools; to some extent, making precise targeting treatment more easier to realize. The most interesting thing is that the Warburg effects happens in cancer metabolism, which acts as the lure for CCNM because of the acid respond property of CCNM, that is, CCNM are not simply as the carrier, it is the metabolic interference in the cancer cells. Based on all these points, CCNM might be the ideal material in fighting the cancers.
Frankly, their low stability in aqueous solutions and insufficient targeting still require researchers’ attention. Moreover, the researchers still need to provide animal models for extensive research to evaluate the long-term effects of CCNM on the living body before clinical conversion.
Author contributions
TtL: Conceptualization, Data curation, Investigation, Methodology, Project administration, Writing–original draft, Writing–review and editing. ZF: Conceptualization, Investigation, Methodology, Software, Supervision, Writing–original draft, Writing–review and editing. XZ: Investigation, Methodology, Project administration, Software, Writing–review and editing. TfL: Formal Analysis, Methodology, Software, Writing–review and editing. TY: Formal Analysis, Supervision, Writing–review and editing. LY: Conceptualization, Funding acquisition, Project administration, Resources, Supervision, Writing–review and editing.
Funding
The author(s) declare financial support was received for the research, authorship, and/or publication of this article. This work was supported by National Natural Science Foundation of China (No. 82160299); Inner Mongolia Natural Science Foundation (No. 2023LHMS08022).
Conflict of interest
The authors declare that the research was conducted in the absence of any commercial or financial relationships that could be construed as a potential conflict of interest.
Publisher’s note
All claims expressed in this article are solely those of the authors and do not necessarily represent those of their affiliated organizations, or those of the publisher, the editors and the reviewers. Any product that may be evaluated in this article, or claim that may be made by its manufacturer, is not guaranteed or endorsed by the publisher.
References
Atchudan, R., Perumal, S., Joo, J., and Lee, Y. R. (2022). Synthesis and characterization of monodispersed spherical calcium oxide and calcium carbonate nanoparticles via simple pyrolysis. Nanomaterials-Basel 12 (14), 2424. doi:10.3390/nano12142424
Azraian, M. H., and Sutapun, W. (2022). Biogenic calcium carbonate derived from waste shells for advanced material applications: A review. Front. Mater 9. doi:10.3389/fmats.2022.1024977
Bai, S., Lan, Y., Fu, S., Cheng, H., Lu, Z., and Liu, G. (2022). Connecting calcium-based nanomaterials and cancer: from diagnosis to therapy. Nano-Micro Lett. 14, 145. doi:10.1007/s40820-022-00894-6
Bai, S., Zhang, Y., Li, D., Shi, X., Lin, G., and Liu, G. (2021). Gain an advantage from both sides: smart size-shrinkable drug delivery nanosystems for high accumulation and deep penetration. Nano Today 36, 101038. doi:10.1016/j.nantod.2020.101038
Behranvand, N., Nasri, F., Zolfaghari Emameh, R., Khani, P., Hosseini, A., Garssen, J., et al. (2022). Chemotherapy: A double-edged sword in cancer treatment. Cancer Immunol. Immun. 71 (3), 507–526. doi:10.1007/s00262-021-03013-3
Calvo-Rodriguez, M., and Bacskai, B. J. (2021). Mitochondria and calcium in alzheimer’s disease: from cell signaling to neuronal cell death. Trends Neurosci. 44, 136–151. doi:10.1016/j.tins.2020.10.004
Cestari, F., Agostinacchio, F, Galotta, A., Chemello, G., Motta, A., and M. Sglavo, V. (2021). Nano-hydroxyapatite derived from biogenic and bioinspired calcium carbonates: synthesis and in vitro bioactivity. Nanomater. Basel 11 (2), 264. doi:10.3390/nano11020264
Chang, M. Y., Hou, Z. Y., Jin, D. Y., Zhou, J., Wang, M., Wang, M., et al. (2020). Colorectal tumor microenvironment-activated bio-decomposable and metabolizable Cu2O@CaCO3 nanocomposites for synergistic oncotherapy. Adv. Mater 32, 2004647. doi:10.1002/adma.202004647
Chiang, P. H., Fan, C. H., Jin, Q., and Yeh, C. K. (2022). Enhancing doxorubicin delivery in solid tumor by superhydrophobic amorphous calcium carbonate-doxorubicin silica nanoparticles with focused ultrasound. Mol. Pharm. 19 (11), 3894–3905. doi:10.1021/acs.molpharmaceut.2c00384
Chuzeville, L., Boury, F., Duday, D., Anand, R., Moretto, E., and Thomann, J. S. (2022). Eco-friendly processes for the synthesis of amorphous calcium carbonate nanoparticles in ethanol and their stabilisation in aqueous media. Green Chem. 24, 1270–1284. doi:10.1039/d1gc03396d
Curtis, N. J., Foster, J. D., Miskovic, D., Brown, C. S. B., Hewett, P. J., Abbott, S., et al. (2020). Association of surgical skill assessment with clinical outcomes in cancer surgery. JAMA Surg. 155 (7), 590–598. doi:10.1001/jamasurg.2020.1004
Dang, H. C., Xu, Z. Z., Chen, Z. S., Wu, W., Feng, J., Sun, Y., et al. (2019). A facile and controllable method to in situ synthesize stable hydrophobic vaterite particles. Cryst. Res. Technol. 54, 1–7. doi:10.1002/crat.201800243
Delierneux, C., Kouba, S., Shanmughapriya, S., Potier-Cartereau, M., Trebak, M., and Hempel, N. (2020). Mitochondrial calcium regulation of redox signaling in cancer. Cells 9 (2), 432. doi:10.3390/cells9020432
Devi, G., Al-Lezami, H. A. A., et al. (2023). Green synthesis, characterization and application of calcium carbonate nanoparticles in the effective treatment of grey water for sustainable water management. J. Iran. Chem. Soc. 20, 1417–1426. doi:10.1007/s13738-023-02766-1
Ding, Y., Yang, J. L., Wang, J. C., Huang, S., Yang, S., et al. (2023). Construction of pH-Sensitive nanovaccines encapsulating tumor cell lysates and immune adjuvants for breast cancer therapy. Small, 2301420. doi:10.1002/smll.202301420
Dong, Z., Hao, Y., Li, Q., Yang, Z., Zhu, Y., Liu, Z., et al. (2020). Metal-polyphenol-network coated CaCO3 as pH-responsive nanocarriers toenable effective intratumoral penetration and reversal of multidrug resistance for augmented cancer treatments. Nano Res. 13 (11), 3057–3067. doi:10.1007/s12274-020-2972-9
Dong, Z. L., Wang, C. J., Gong, W. M., Zhang, Y., Fan, Q., Hao, Y., et al. (2022). Chemical modulation of glucose metabolism with a fluorinated CaCO3 nanoregulator can potentiate radiotherapy by programming antitumor immunity. ACS Nano 16 (9), 13884–13899. doi:10.1021/acsnano.2c02688
Eisner, D., Neher, E., Taschenberger, H., and Smith, G. (2023). Physiology of intracellular calcium buffering. Physiol. Rev. 103, 2767–2845. doi:10.1152/physrev.00042.2022
Elbaz, N. M., Owen, A., Rannard, S., and McDonald, T. O. (2020). Controlled synthesis of calcium carbonate nanoparticles and stimuli-responsive multi-layered nanocapsules for oral drug delivery. Int. J. Pharm. 574, 118866. doi:10.1016/j.ijpharm.2019.118866
Febrida, R., Cahyanto, A., Herda, E., Muthukanan, V., Djustiana, N., Faizal, F., et al. (2021). Synthesis and characterization of porous CaCO3 vaterite particles by simple solution method. Materials 14 (16), 4425. doi:10.3390/ma14164425
Fu, W. L., Ibrahim, T. A. T., Yusof, L. M., et al. (2019). In vivo evaluation of anticancer effificacy of drug loaded cockle shell-derived aragonite nanoparticles. J. Biomed. Mater Res. B 107, 1898–1907.
Gbadenyan, O. J., Adali, S., Bright, G., and Sithole, B. (2021). The investigation of reinforcement properties of nano-CaCO3 synthesized from Achatina fulica snail shell through mechanochemical methods on epoxy nanocomposites. Nanocomposites 7, 79–86. doi:10.1080/20550324.2021.1936972
Gindele, M. B., Steingrube, L. V., Gebauer, D., et al. (2021). Generality of liquid precursor phases in gas diffusion-based calcium carbonate synthesis. CrystEngComm 23, 7938–7943. doi:10.1039/d1ce00225b
Glitsch, M. (2019). Mechano-and pH-sensing convergence on Ca2+-mobilising proteins-A recipe for cancer? Cell. Calcium 80, 38–45. doi:10.1016/j.ceca.2019.03.010
Guan, Q., Zhou, L. L., Lv, F. H., Li, W., Li, Y., and Dong, Y. (2020). A Glycosylated covalent organic framework equipped with BODIPY and CaCO3 for synergistic tumor therapy. Angew. Chem. Int. Ed. Engl. 59 (41), 18042–18047. doi:10.1002/anie.202008055
Han, C. L., Hu, Y. P., Wang, K., and Luo, G. (2019). Preparation and in-situ surface modification of CaCO3 nanoparticles with calcium stearate in a microreaction system. Powder Technol. 356, 414–422. doi:10.1016/j.powtec.2019.08.054
Han, Y. K., Dong, Z. L., Wang, C. J., Li, Q., Hao, Y., Yang, Z., et al. (2022). Ferrous ions doped calcium carbonate nanoparticles potentiate chemotherapy by inducing ferroptosis. J. Control Release 348, 346–356. doi:10.1016/j.jconrel.2022.06.002
Huang, H., Zhang, W., Liu, Z., Guo, H., and Zhang, P. (2020). Smart responsive-calcium carbonate nanoparticles for dual-model cancer imaging and treatment. Ultrasonics 108, 106198. doi:10.1016/j.ultras.2020.106198
Hussein, A. I., Husein, A., Ab-Ghani, Z., Ab Ghani, N. A., and Ab. Rahman, I. (2020). Synthesis and characterization of spherical calcium carbonate nanoparticles derived from cockle shells. Appl. Sci. 10 (20), 7170. doi:10.3390/app10207170
Ippolito, F., Hubner, G., Claypole, T., and Gane, P. (2020). Influence of the surface modification of calcium carbonate on polyamide 12 composites. Polym. (Basel) 12, 1295. doi:10.3390/polym12061295
Ju, Y. M., Zhao, Y., Guan, Q. F., Yang, S., Wang, W., Yan, B., et al. (2022). Amorphous calcium carbonate cluster nanospheres in water-deficient organic solvents. Angew. Chem. Int. Ed. 61 (47), e202211254. doi:10.1002/anie.202211254
Kaiping, T., Wanfen, P., Siying, L., Hongzhou, W., Wei, H., and Minghong, X. (2023). Preparation of hydrophobic nano calcium carbonate and its application in EOR. IOP Conf. Ser. Earth Environ. Sci. 1152, 012012. doi:10.1088/1755-1315/1152/1/012012
Kanwal, A., Uzair, B., Sajjad, S., Samin, G., Ali Khan, B., Khan Leghari, S. A., et al. (2022). Synthesis and characterization of carbon dots coated CaCO3 nanocarrier for levofloxacin against multidrug resistance extended-spectrum beta-lactamase escherichia coli of urinary tract infection origin. Microb. Drug Resist 28, 106–119. doi:10.1089/mdr.2020.0621
Kashyap, B. K., Singh, V. V., Solanki, M. K., Kumar, A., Ruokolainen, J., and Kesari, K. K. (2023). Smart nanomaterials in cancer theranostics: challenges and opportunities. ACS Omega 8 (16), 14290–14320. doi:10.1021/acsomega.2c07840
Khan, M. W., Zou, C., Hassan, S., Din, F. U., Abdoul Razak, M. Y., Nawaz, A., et al. (2022). Cisplatin and oleanolic acid Co-loaded pH-sensitive CaCO3 nanoparticles for synergistic chemotherapy. RSC Adv. 12, 14808–14818. doi:10.1039/d2ra00742h
Khan, S. R., Jamil, S., Rashid, H., Ali, S., and Janjua, M. R. S. A. (2019). Agar and egg shell derived calcium carbonate and calcium hydroxide nanoparticles: synthesis, characterization and applications. Chem. Phys. Lett. 732, 136662. doi:10.1016/j.cplett.2019.136662
Kong, H., Chu, Q., Fang, C., Cao, G., Han, G., and Li, X. (2021). Cu–ferrocene-functionalized CaO2 nanoparticles to enable tumor-specific synergistic therapy with GSH depletion and calcium overload. Adv. Sci. 8 (14), e2100241. doi:10.1002/advs.202100241
Lam, S. F., Bishop, K. W., Mintz, R., Fang, L., and Achilefu, S. (2021). Calcium carbonate nanoparticles stimulate cancer cell reprogramming to suppress tumor growth and invasion in an organ-on-a-chip system. Sci. Rep-UK 11, 9246. doi:10.1038/s41598-021-88687-6
Lee, J., Jo, S. H., and Lim, J. (2019). Effect of surface modification of CaCO3 nanoparticles by a silane coupling agent methyltrimethoxysilane on the stability of foam and emulsion. J. Ind. Eng. Chem. 74, 63–70. doi:10.1016/j.jiec.2019.02.002
Li, C. Q., Liang, C., Di, Y. H., Zheng, S. l., Wei, S., et al. (2021b). Surface modification of calcium carbonate: A review of theories, methods and applications. J. Cent. South Univ. 28, 2589–2611. doi:10.1007/s11771-021-4795-6
Li, G. R., Lingland, K., Larsen, R. H., and Westrøm, S. (2021a). A novel single-step-labeled 212Pb-CaCO3 microparticle for internal alpha therapy: preparation, stability, and preclinical data from mice. Materials 14 (23), 7130. doi:10.3390/ma14237130
Li, H. R., Zhang, X. Y., Lin, X. L., Zhuang, S., Wu, Y., Liu, Z., et al. (2020b). CaCO3 nanoparticles pH-sensitively induce blood coagulation as a potential strategy for starving tumor therapy. J. Mater Chem. B 8, 1223–1234. doi:10.1039/c9tb02684c
Li, J., Yang, S., Liu, Y., Muhammad, Y., and Su, Z. (2019). Studies on the properties of modified heavy calcium carbonate and SBS composite modified asphalt. Constr. Build. Mater 218, 413–423. doi:10.1016/j.conbuildmat.2019.05.139
Li, L., Yang, Y., Lv, Y., Yin, P., and Lei, T. (2020a). Porous calcite CaCO3 microspheres: preparation, characterization and release behavior as doxorubicin carrier. Colloid Surf. B 186, 110720. doi:10.1016/j.colsurfb.2019.110720
Liu, J., Zhu, C., Xu, L., Wang, D., Liu, W., Zhang, K., et al. (2020c). Nanoenabled intracellular calcium bursting for safe and efficient reversal of drug resistance in tumor cells. Nano Lett. 20 (11), 8102–8111. doi:10.1021/acs.nanolett.0c03042
Li, Y., Zhou, S., Song, H., Yu, T., Zheng, X., and Chu, Q. (2021c). CaCO3 nanoparticles incorporated with KAE to enable amplified calcium overload cancer therapy. Biomaterials 277, 121080. doi:10.1016/j.biomaterials.2021.121080
Li, Y. H., Zhang, X., Liu, X. H., Pan, W., and Tang, B. (2021d). A mineralization strategy based on T-cell membrane coated CaCO3 nanoparticles against breast cancer and metastasis. Mater Chem. Front. 5, 5738–5745. doi:10.1039/d1qm00464f
Liu, X., Su, Q., Song, H., Shi, X., Zhang, Y., Zhang, C., et al. (2021). PolyTLR7/8a-conjugated, antigen-trapping gold nanorods elicit anticancer immunity against abscopal tumors by photothermal therapy-induced in situ vaccination. Biomaterials 275, 120921. doi:10.1016/j.biomaterials.2021.120921
Lu, J., Jiao, Y. P., Cao, G. C., and Liu, Z. (2021). Multimode CaCO3/pneumolysin antigen delivery systems for inducing efficient cellular immunity for anti-tumor immunotherapy. Chem. Eng. J. 420, 129746. doi:10.1016/j.cej.2021.129746
Luo, W. L., Li, Z. J., Zhang, L., and Xie, X. (2020). Polyethylenimine-CO2 adduct templated CaCO3 nanoparticles as anticancer drug carrier. Cancer Nanotechnol. 186, 7. doi:10.1186/s12645-023-00156-z
Ma, X. X., Wang, C., Dong, Z. L., et al. (2022). Lipid-coated CaCO3-PDA nanoparticles as a versatile nanocarrier to enable pH-responsive dual modal imaging-guided combination cancer therapy. J. Mat. Chem. B, 10.
Ma, Z., Zhang, J., Zhang, W., Foda, M. F., Zhang, Y., Ge, L., et al. (2020). Intracellular Ca2+ cascade guided by NIR-II photothermal switch for specific tumor therapy. i Sci. 23 (5), 101049. doi:10.1016/j.isci.2020.101049
Mao, S. D., Liu, Y., and Zhang, Y. A. (2021). Nano-CaCO3 synthesis by tangential jet from carbide slag. Mater Res. Express 8 (9), 095005. doi:10.1088/2053-1591/ac2285
Marchi, S., Giorgi, C., Galluzzi, L., and Pinton, P. (2020). Ca2+ fuxes and cancer. Mol. Cell. 78 (6), 1055–1069. doi:10.1016/j.molcel.2020.04.017
Mkhize, S. C., Onwubu, S. C., Mlambo, M., and Mdluli, P. S. (2021). An in vitro assessment of the acid resistance characteristics of nanohydroxyapatite/silica biocomposite synthesized using mechanochemistry. J. Nanomater 2021, 1–6. doi:10.1155/2021/4438100
Niu, Y. Q., Liu, H. J., Aymonier, C., Fermani, S., Kralj, D., Falini, G., et al. (2022). Calcium carbonate: controlled synthesis, surface functionalization, and nanostructured materials. Chem. Soc. Rev. 51, 7883–7943. doi:10.1039/d1cs00519g
Novoselova, M. V., German, S. V., Abakumova, T. O., Perevoschikov, S. V., Sergeeva, O. V., Nesterchuk, M. V., et al. (2021). Multifunctional nanostructured drug delivery carriers for cancer therapy: multimodal imaging and ultrasound-induced drug release. Colloid surfaces B 200, 111576. doi:10.1016/j.colsurfb.2021.111576
Pei, Z. F., Lei, H. L., Cheng, L., et al. (2023). Bioactive inorganic nanomaterials for cancer theranostics. Chem. Soc. Rev. 52, 2031–2081. doi:10.1039/d2cs00352j
Pérez-Villarejo, L., Takabait, F., Mahtout, L., Carrasco-Hurtado, B., Eliche-Quesada, D., and Sánchez-Soto, P. J. (2018). Synthesis of vaterite CaCO3 as submicron and nanosized particles using inorganic precursors and sucrose in aqueous medium. Ceram. Int. 44 (5), 5291–5296. doi:10.1016/j.ceramint.2017.12.142
Persano, F., Nobile, C., Piccirillo, C., Gigli, G., and Leporatti, S. (2022). Monodisperse and nanometric-sized calcium carbonate particles synthesis optimization. Nanomaterials 12, 1494. doi:10.3390/nano12091494
Piras, C. C., Fernandez, P. S., Borggraeve, W. M. D., et al. (2019). Ball milling: A green technology for the preparation and functionalisation of nanocellulose derivatives. Nanoscale Adv. 1, 937–947. doi:10.1039/c8na00238j
Popova, V., Poletaeva, Y., Pyshnaya, I., Pyshnyi, D., and Dmitrienko, E. (2021). Designing pH-dependent systems based on nanoscale calcium carbonate for the delivery of an antitumor drug. Nanomaterials-Basel 11 (11), 2794. doi:10.3390/nano11112794
Preksha, F., Simona, T., Stuti, B., Nair, A., Panchal, P., Dave, H., et al. (2021). Calcium carbonate nano- and microparticles: synthesis methods and biological applications. Bio Tech. 11, 457. doi:10.1007/s13205-021-02995-2
Prihanto, A., Muryanto, S., Vaquer, A. S., Schmahl, W., Ismail, R., Jamari, J., et al. (2023). In-depth knowledge of the low-temperature hydrothermal synthesis of nanocrystalline hydroxyapatite from waste green mussel shell (Perna Viridis). EnvironTechnol, 1–13. doi:10.1080/09593330.2023.2173087
Qiao, B., Luo, Y., Cheng, H. B., Ren, J., Cao, J., Yang, C., et al. (2020). Artificial nanotargeted cells with stable photothermal performance for multimodal imaging-guided tumor-specific therapy. ACS Nano 14 (10), 12652–12667. doi:10.1021/acsnano.0c00771
Ren, Q. D., Qian, W. F., Chen, B. Q., Shuai, Q., and Yan, Y. (2023). Flash nanoprecipitation fabrication of PEI@Amorphous calcium carbonate hybrid nanoparticles for siRNA delivery. Macromol. Biosci. 7, 2300085. doi:10.1002/mabi.202300085
Rinu, S. K., Queen, M. A. J., Udhaya, P. A., et al. (2020). Synthesis, structural characterization and antibacterial applications of calcium nanoparticles. J. Adv. Sci. Res. 11 (1), 83–87.
Ruysscher, D. D., Niedermann, G., Burnet, N. G., Siva, S., Lee, A. W. M., and Hegi-Johnson, F. (2019). Radiotherapy toxicity. Nat. Rev. Dis. Prim. 5 (1), 13. doi:10.1038/s41572-019-0064-5
Shen, C. C., Li, R., Pei, J. Z., Cai, J., Liu, T., and Li, Y. (2019). Preparation and the effect of surface-functionalized calcium carbonate nanoparticles on asphalt binder. Appl. Sci. 10, 91–16. doi:10.3390/app10010091
Shen, J. C., Liang, X. X., Wu, W. J., Feng, T., Karges, J., Lin, M., et al. (2022). A pH-responsive iridium (III) two-photon photosensitizer loaded CaCO3 nanoplatform for combined Ca2+ overload and photodynamic therapy. Inorg. Chem. Front. 9, 4171–4183. doi:10.1039/d2qi00951j
Shou, H., Wu, J. C., Tang, N., and Wang, B. (2022). Calcification-based cancer diagnosis and therapy. Chem. Med. Chem. 17 (4), e202100339. doi:10.1002/cmdc.202100339
Som, A., Raliya, R., Tian, L., Akers, W., Ippolito, J. E., Singamaneni, S., et al. (2016). Monodispersed calcium carbonate nanoparticles modulate local pH and inhibit tumor growth in vivo. Nanoscale 8, 12639–12647. doi:10.1039/c5nr06162h
Sun, X. H., Miao, L. C., Wu, L. Y., et al. (2020). Applicability and theoretical calculation of enzymatic calcium carbonate precipitation for sand improvement. Geomicrobiol. J. 37, 389–399. doi:10.1080/01490451.2019.1710625
Sung, H., Ferlay, J., Siegel, R. L., Laversanne, M., Soerjomataram, I., Jemal, A., et al. (2021). Global cancer statistics 2020: GLOBOCAN estimates of incidence and mortality worldwide for 36 cancers in 185 countries. CA Cancer J. Clin. 71 (3), 209–249. doi:10.3322/caac.21660
Teixeira, S., Carvalho, M. A., and Castanheira, E. M. S. (2022). Functionalized liposome and albumin-based systems as carriers for poorly water-soluble anticancer drugs: an updated review. Biomedicines 10 (2), 486. doi:10.3390/biomedicines10020486
Tinawi, M. (2021). Disorders of calcium metabolism: hypocalcemia and hypercalcemia. Cureus 13 (1), e12420. doi:10.7759/cureus.12420
Torgbo, S., Sukyai, P., Watthanasakphuban, N., and Kamonsutthipaijit, N. (2023). Filter cake-derived calcium carbonate polymorphs from sugar refinery for hydroxyapatite production as a sustainable material for biomedical application. Ceram. Int. 49, 23417–23425. doi:10.1016/j.ceramint.2023.04.174
Trushina, D. B., Borodina, T. N., Belyakov, S., and Antipina, M. N. (2022). Calcium carbonate vaterite particles for drug delivery: advances and challenges. Mater Today Adv. 14, 100214. doi:10.1016/j.mtadv.2022.100214
Vavaev, E. S., Novoselova, M, Shchelkunov, N. M., German, S., Komlev, A. S., Mokrousov, M. D., et al. (2022). CaCO3 nanoparticles coated with alternating layers of poly-l-arginine hydrochloride and Fe3O4 nanoparticles as navigable drug carriers and hyperthermia agents. ACS Appl. Nano Mater 5, 2994–3006. doi:10.1021/acsanm.2c00338
Vidallon, M. L. P., Douek, A. M., Quek, A., McLiesh, H., Kaslin, J., Tabor, R. F., et al. (2020). Gas-generating, pH-responsive calcium carbonate hybrid particles with biomimetic coating for contrast-enhanced ultrasound imaging. Part Part Syst. Char 37 (2), 1900471. doi:10.1002/ppsc.201900471
Vikulina, A., Webster, J., Voronin, D., Ivanov, E., Fakhrullin, R., Vinokurov, V., et al. (2021). Mesoporous additive-free vaterite CaCO3 crystals of untypical sizes: from submicron to giant. Mater Des. 197, 109220. doi:10.1016/j.matdes.2020.109220
Wang, C., Chen, S., Bao, L., Liu, X., Hu, F., and Yuan, H. (2020). <p>Size-Controlled preparation and behavior study of phospholipid–calcium carbonate hybrid nanoparticles</p>. Intern. J. Nanomed 15, 4049–4062. doi:10.2147/ijn.s237156
Wang, C. J., Dong, Z. L., Hao, Y., Zhu, Y., Ni, J., Li, Q., et al. (2022). Coordination polymer-coated CaCO3 reinforces radiotherapy by reprogramming the immunosuppressive metabolic microenvironment. Adv. Mater 34 (3), 2106520. doi:10.1002/adma.202106520
Wang, C. Y., Zhang, Q. N., Chen, M. Y., Hu, A., Wei, B., and Yang, Z. (2023a). Nanomaterials mediated multimodal combined treatment for cancer. Mini-Rev Med. Chem. 23, 1623–1641. doi:10.2174/1389557523666230117103157
Wang, W. L., Yu, J., Lin, Y., Li, M., Pan, Y., He, Y., et al. (2023b). NIR absorptive croconic acid/quercetin/CaO2nanoplatform for tumor calcium overload therapy combined mild photothermal therapy. Mater. Sci. Eng. C-Materials Biol. Appl. 149, 213418. doi:10.1016/j.bioadv.2023.213418
Wang, X. W., Zhong, X. Y., Li, J. X., Liu, Z., and Cheng, L. (2021). Inorganic nanomaterials with rapid clearance for biomedical applications. Chem. Soc. Rev. 50 (15), 8669–8742. doi:10.1039/d0cs00461h
Worsley, C. M., Veale, R. B., and Mayne, E. S. (2022). The acidic tumour microenvironment: manipulating the immune response to elicit escape. Hum. Immunol. 83 (5), 399–408. doi:10.1016/j.humimm.2022.01.014
Wu, Y. X., Huang, M. Y., He, C. L., Wang, K., Nhung, N. T. H., Lu, S., et al. (2022). The influence of air nanobubbles on controlling the synthesis of calcium carbonate crystals. Colloids Surfaces A Physicochem. Eng. Aspects 15 (21), 7437. doi:10.3390/ma15217437
Xia, C., Dong, X., Li, H., Cao, M., Sun, D., He, S., et al. (2022). Cancer statistics in China and United States, 2022: profiles, trends, and determinants. Chin. Med. J. 135 (5), 584–590. doi:10.1097/cm9.0000000000002108
Xu, D., Jin, T. T., Song, L. J., Zhu, N., Han, L., and Hong, H. (2022a). Synthesis of stable calcium carbonate nanoparticles for pH-responsive controlled drug release. Mater Lett. 333, 133635. doi:10.1016/j.matlet.2022.133635
Xu, M. Q., Zhang, J., Mu, L. Y., Foda, M. F., and Han, H. (2022b). Activation of TRPV1 by capsaicin-loaded CaCO3 nanoparticle for tumor-specific therapy. Biomaterials 284, 121520. doi:10.1016/j.biomaterials.2022.121520
Yamaguchi, T., Kitahara, S., Kusuda, K., Okamoto, J., Horise, Y., Masamune, K., et al. (2021). Current landscape of sonodynamic therapy for treating cancer. Cancers 13 (24), 6184. doi:10.3390/cancers13246184
Yang, A. M., Huang, Z. Q., Zhu, Y., Han, Y., and Tong, Z. (2021a). Preparation of nano-sized calcium carbonate in solution mixing process. J. Cryst. Growth 571, 126247. doi:10.1016/j.jcrysgro.2021.126247
Yang, K., Yang, Z., Yu, G., Nie, Z., Wang, R., and Chen, X. (2022). Polyprodrug nanomedicines: an emerging paradigm for cancer therapy. Adv. Mater 34 (6), 2107434. doi:10.1002/adma.202107434
Yang, S. N., Zhang, Y. M., Lu, S. J., Yang, L., Yu, S., and Yang, H. (2021b). CaCO3-encapsulated Au nanoparticles modulate macrophages toward M1-like phenotype. ACS Appl. Bio Mater 4 (4), 3214–3223. doi:10.1021/acsabm.0c01608
Yi, Z., Luo, Z., Barth, N. D., Meng, X., Liu, H., Bu, W., et al. (2019). In vivo tumor visualization through MRI off-on switching of NaGdF4-CaCO3 nanoconjugates. Adv. Mater. 31, 1901851. doi:10.1002/adma.201901851
Yu, J. M., Wang, L. L., Xie, X., Zhu, W., Lei, Z., Lv, L., et al. (2023). Multifunctional nanoparticles codelivering doxorubicin and amorphous calcium carbonate preloaded with indocyanine green for enhanced chemo-photothermal cancer therapy. Inter J. Nanomed 18, 323–337. doi:10.2147/ijn.s394896
Zhao, P., Tian, Y., Lu, Y., et al. (2022b). Biomimetic calcium carbonate nanoparticles delivered IL-12 mRNA for targeted glioblastoma sono-immunotherapy by ultrasound-induced necroptosis. J. Nanobiotechnol 525, 20.
Zhao, Y. J., Bian, Y. L., Xiao, X., Liu, B., Ding, B., Cheng, Z., et al. (2022a). Tumor microenvironment-responsive Cu/CaCO3-based nanoregulator for mitochondrial homeostasis disruption-enhanced chemodynamic/sonodynamic therapy. Small 18, 2204047. doi:10.1002/smll.202204047
Zhao, Y. M., Zheng, Y. L., Zhu, Y., Ding, Kai, Zhou, M., and Liu, T. (2023). Co-delivery of gemcitabine and Triapine by calcium carbonate nanoparticles against chemoresistant pancreatic cancer. Int. J. Pharm. 636, 122844. doi:10.1016/j.ijpharm.2023.122844
Zheng, P., Ding, B. B., Shi, R., Jiang, Z., Xu, W., Li, G., et al. (2021). A multichannel Ca2+ nanomodulator for multilevel mitochondrial destruction-mediated cancer therapy. Adv. Mater, 33 (15), 2007426. doi:10.1002/adma.202007426
Zheng, P., Ding, B. B., Zhu, G. Q., Li, C., and Lin, J. (2022). Biodegradable Ca2+ nanomodulators activate pyroptosis through mitochondrial Ca2+ overload for cancer immunotherapy. Angew. Chem. 36, 61. doi:10.1002/ange.202204904
Zhong, W. Z., Wong, K. H., Xu, F. J., Zhao, N., and Chen, M. (2022). NIR-responsive polydopamine-based calcium carbonate hybrid nanoparticles delivering artesunate for cancer chemo-photothermal therapy. Acta biomater. 145, 135–145. doi:10.1016/j.actbio.2022.03.051
Zhou, F., Li, H. R., Liu, Y. Y., Deng, H., Rong, J., and Zhao, J. (2023). Hyaluronan derivative decorated calcium carbonate nanoparticle as a potential platform for breast cancer synergistic therapy via blood coagulation and drug delivery. J. Drug Deliv. Sci. Technol. 83, 104406. doi:10.1016/j.jddst.2023.104406
Zhou, Y., Jing, S. S., Liu, S. N., Shen, X., Cai, L., Zhu, C., et al. (2022). Double-activation of mitochondrial permeability transition pore opening via calcium overload and reactive oxygen species for cancer therapy. J. Nanobiotechnol 20, 188. doi:10.1186/s12951-022-01392-y
Zhu, L., Wang, G., Shi, W., Ma, X., Yang, X., Yang, H., et al. (2019). In situ generation of biocompatible amorphous calcium carbonate onto cell membrane to block membrane transport protein-A new strategy for cancer therapy via mimicking abnormal mineralization. J. Colloid Interface Sci. 541, 339–347. doi:10.1016/j.jcis.2019.01.090
Keywords: calcium carbonate nanomaterials, nanotechnology, drug carrier, pH-responsive, cancer treatment
Citation: Liang T, Feng Z, Zhang X, Li T, Yang T and Yu L (2023) Research progress of calcium carbonate nanomaterials in cancer therapy: challenge and opportunity. Front. Bioeng. Biotechnol. 11:1266888. doi: 10.3389/fbioe.2023.1266888
Received: 26 July 2023; Accepted: 11 September 2023;
Published: 21 September 2023.
Edited by:
Hamed Barabadi, Shahid Beheshti University of Medical Sciences, IranReviewed by:
Yilun Wu, The University of Queensland, AustraliaChuang Liu, Harvard Medical School, United States
Xuan Mei, Harvard Medical School, United States
Copyright © 2023 Liang, Feng, Zhang, Li, Yang and Yu. This is an open-access article distributed under the terms of the Creative Commons Attribution License (CC BY). The use, distribution or reproduction in other forums is permitted, provided the original author(s) and the copyright owner(s) are credited and that the original publication in this journal is cited, in accordance with accepted academic practice. No use, distribution or reproduction is permitted which does not comply with these terms.
*Correspondence: Lan Yu, yulan@imph.ac.cn
†These authors have contributed equally to this work