- 1Department of Neurosurgery, West China Hospital, Sichuan University, Chengdu, China
- 2West China School of Medicine, Sichuan University, Chengdu, China
- 3State Key Laboratory of Polymer Materials Engineering, College of Polymer Science and Engineering, Sichuan University, Chengdu, China
- 4School of Materials Science and Engineering, Xihua University, Chengdu, China
Intracerebral hemorrhage (ICH), the most devastating subtype of stoke, is of high mortality at 5 years and even those survivors usually would suffer permanent disabilities. Fortunately, various preclinical active drugs have been approached in ICH, meanwhile, the therapeutic effects of these pharmaceutical ingredients could be fully boosted with the assistance of nanotechnology. In this review, besides the pathology of ICH, some ICH therapeutically available active drugs and their employed nanotechnologies, material functions, and therapeutic principles were comprehensively discussed hoping to provide novel and efficient strategies for ICH therapy in the future.
1 Introduction
Stroke is the second leading global cause of death and the third one of death and disability combined (Collaborators, 2021). Thanks to the progress in medicine, the situation of stroke patients has been improved overall (Elkind and Hankey, 2022), nevertheless, stroke is still a great threat to human particularly people in most areas of China where the prevalence of stroke consecutively increased from 2013 to 2019 (Tu et al., 2022). As the most serious subtype of stroke (Cordonnier et al., 2018a), intracerebral hemorrhage (ICH) affects about 2 million people worldwide annually (Krishnamurthi et al., 2013), and is critically life-threatening with a 1-year survival rate of less than 40% (Bejot et al., 2018). Extensive research efforts have led to a growing understanding of this disease, particularly its detrimental mechanisms including primary injury and secondary injury (Figure 1) (Wilkinson et al., 2018b; Campbell and Khatri, 2020; Bautista et al., 2021; Sheth, 2022). The primary injury after ICH is mainly referred to hematoma induced mass effect (Wilkinson et al., 2018a), and the subsequent secondary injury is more complex, including brain edema (Wan et al., 2023), ferroptosis (Wan et al., 2019), oxidative stress (Hu et al., 2016), inflammation (Xue and Yong, 2020), apoptosis (Chen et al., 2020), etc. Although ICH is rather detrimental, as shown in Figure 1, clinical approaches available in ICH are rather limited including medical managements such as blood pressure management, anticoagulation reversal, and intracranial pressure management (Cordonnier et al., 2018b; Kirshner and Schrag, 2021), as well as the surgical ones aiming to remove hematoma such as external ventricular drain, craniotomy, minimally invasive approaches, and so on (de Oliveira Manoel, 2020). However, with all of these treatments, the prognosis of ICH is still hard to be improved profoundly (Rabinstein, 2017). Accordingly, it is imperative to develop more therapeutic agents particularly those targeting the secondary injury, which is strongly associated to the outcomes of ICH (Bautista et al., 2021). In recent years, increasingly active pharmaceutical ingredients were applied against ICH in preclinical studies comprising deferoxamine (Xu et al., 2020b), curcumin (Yang C. et al., 2021), and resveratrol (Mo et al., 2021), etc., however, most of them could be hardly applied to ICH patients since their toxicity, poor bioavailability, and limited functions (Yu H. et al., 2023). Thus, it is practical to improve the intrinsic disadvantages of current drugs through rational design and novel drug loading techniques, particularly by exploiting the nanotechnology (Yu H. et al., 2023). Actually, nanomaterials such as polymer (Xu et al., 2020b), micelles (Zi et al., 2021), nanoemulsions (Marques et al., 2020), liposomes (Xu et al., 2020a), and exosomes (Hu et al., 2023), etc., have been widely designed and applied for ICH therapy, and their properties including biocompatibility, biodegradability, and engineerability, etc., have been roundly demonstrated (Figure 1) (Xu et al., 2022; Zhang et al., 2023). In this review, we mainly summarized the pathological mechanisms in ICH, potential therapeutic targets, and the role of nanotechnologies in drug design and delivery. The aim was to discuss the potential of nanotechnologies in enhancing therapeutic effects of drugs and to provide a better theoretical basis for supporting future therapies of ICH.
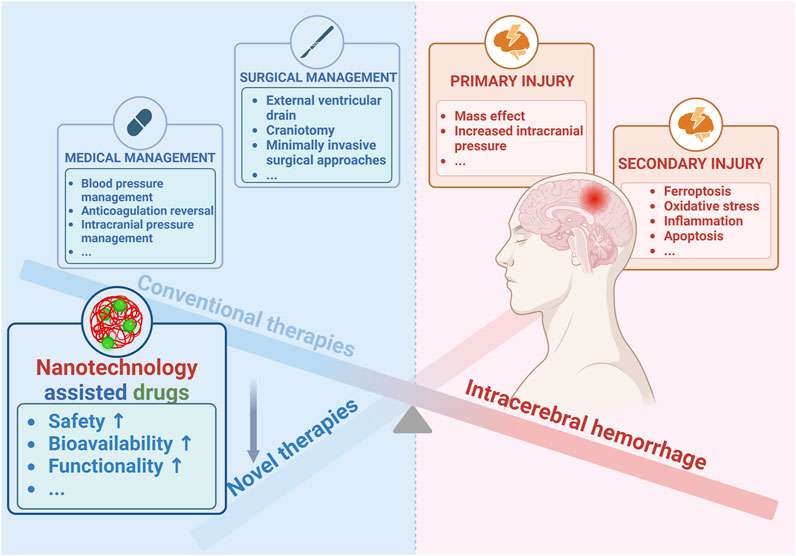
FIGURE 1. Advances of nanotechnology for ICH therapy. Created with Biorender.com.
2 Injuries and mechanisms of ICH
Generally, the damages caused by ICH could be divided into primary and secondary injuries (Puy et al., 2023). The former mainly consists of the hematoma induced mass effect, subsequent increased intracranial pressure, and so on (Wilkinson et al., 2018a; Kalisvaart et al., 2022; Wan et al., 2023). With the formation of hematoma (Wilkinson et al., 2018a), secondary brain injury such as ferroptosis (Wan et al., 2019), oxidative stress (Hu et al., 2016), inflammation (Xue and Yong, 2020), and apoptosis (Chen et al., 2020) gradually emerges (Figure 2). Specifically, ICH occurs when some brain vessels rupture, causing blood to flood into the brain parenchyma and form hematoma (Chang et al., 2018). With the accumulation of the blood, the hematoma might expand (Morotti et al., 2022) and the resultant mechanic compression as well as subsequent increased intracranial pressure would emerge gradually (Puy et al., 2023). Notably, the hematoma is a determining factor for the primary injury, and strongly associated with the subsequent secondary injury especially inflammation and oxidative stress (Xu et al., 2020a; Liu et al., 2022). Within several days after ICH, hemolysis will occur in the hematoma, leading to the release of hemolytic products including hemoglobin and heme, which wound exaggerate inflammation (Wang et al., 2021; Xia et al., 2022). Subsequently, the iron degenerated from heme acts as the catalyst in Haber-Weiss reaction (Zhu et al., 2021), generating overloaded reactive oxygen species (ROS) and leading to detrimental oxidative stress (Wan et al., 2019). Additionally, thrombin and serum proteins derived from hematoma will worsen edema and damages of blood-brain barrier (BBB) (Katsu et al., 2010; Urday et al., 2015). Thus, in clinical practice, the volume of hematoma is a widely used prognostic biomarker and its rapid resolution is vital and urgent for ICH patients (Broderick et al., 1993; de Oliveira Manoel, 2020). In addition to the well-studied oxidative stress and the inflammatory injury (Ohashi et al., 2023; Xu et al., 2023), the apoptosis and ferroptosis induced by ICH had drawn increasing attention and became potential therapeutic targets in recent years since their relationship with the poor neurological outcomes of ICH patients (Wan et al., 2019; Chen et al., 2020). Despite great advances in understanding the pathological process of ICH had been achieved, many mechanisms remain unclear, and effective therapies for ICH patients are still insufficient (Rabinstein, 2017; Kirshner and Schrag, 2021; Gu et al., 2022). Fortunately, by the efforts of researchers, more and more active pharmaceutical ingredients targeting ICH were developed in preclinical studies with the help of nanotechnology (Yu H. et al., 2023). Specifically, by combining nanomaterials, not only many therapeutic agents’ intrinsic disadvantages such as toxicity and poor bioavailability were improved, but also their therapeutic effects were enhanced profoundly (Xu et al., 2022; Zhang et al., 2023).
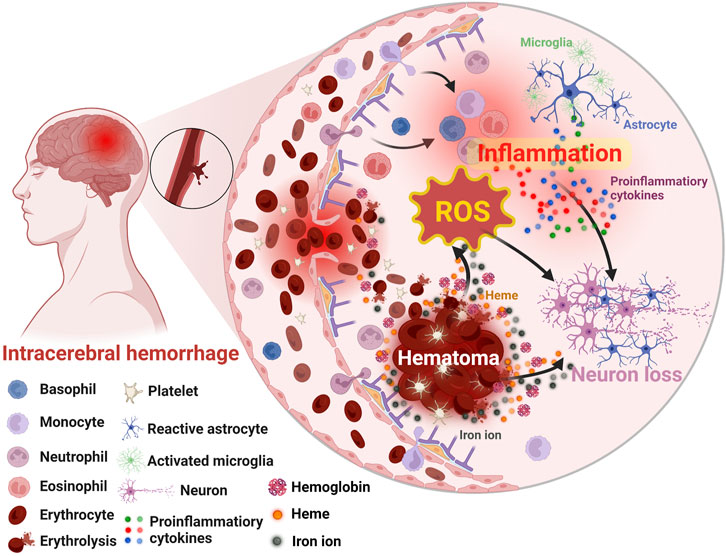
FIGURE 2. Schematic illustration of the secondary brain injury after ICH. Created with Biorender.com.
3 Nanomaterials in ICH
As previously mentioned, ICH is the most fatal stroke type and affects about 2 million people globally each year (Krishnamurthi et al., 2013). Unfortunately, there is a lack of effective therapies for ICH (Rabinstein, 2017). While minimally invasive surgery for ICH is widely accepted particularly in high-income countries, there are still controversial issues that need to be addressed such as the lack of sufficient evidence and the fact that not all patients are suitable candidates for the surgical approach (Wilkinson et al., 2018a). In addition to surgery, many researchers are dedicating their efforts to developing potential therapies against ICH including novel technologies utilization and emerging nanomaterials such as polymer (Xu et al., 2020b), micelles (Zi et al., 2021), nanoemulsions (Marques et al., 2020), liposomes (Xu et al., 2020a), and exosomes (Hu et al., 2023). Noteworthily, some approaches including polymerization, self-assembly and microfluidics play a significant role not only in the design and fabrication of novel drug, but also in improving the efficacy of therapeutic agents for ICH by addressing intrinsic disadvantages such as toxicity, poor bioavailability, and unsatisfactory therapeutic performance (Xu et al., 2022; Yu H. et al., 2023; Zhang et al., 2023). Specifically, in this review, the employed nanotechnological strategies mainly consist of the advanced drug loading techniques, post-synthetic modification (PSM), and self-assembly (Table 1). Specifically, the drug loading techniques, which provide vehicles for delivering drugs to targeted position, can change the drug pharmacokinetics, improve the drug efficacy, and reduce adverse effects (Wang W. et al., 2019; Huang et al., 2019; Mehryab et al., 2020; Yu H. et al., 2023). As one of the widely applied nanotechnologies, PSM refers to functionalization of the synthesized the nanomaterials such as the PEGylation (Xu et al., 2020b), which can improve the properties of the therapeutic agents for requirements (Mandal et al., 2021; Figueroa-Quintero et al., 2023). Moreover, self-assembly, another important method of nanotechnology, by employing which a thermodynamically stable nanostructure can be achieved via weak and polyvalent interactions (Li et al., 2018b). And this structure is of fast response to environmental stimuli since its low energy barrier (Stuart et al., 2010), which is of significance in nanomedicine (Srikanth and Kessler, 2012). Therefore, in the following section, some impressive recent works that focus on the role of nanomaterials in promoting the safety, bioavailability, and functions of therapeutic agents against ICH would be roundly introduced and discussed.
3.1 Safety
Like other drugs, the development of new therapeutic agents for ICH must prioritize safety to guarantee clinical application. Accordingly, safer pharmaceutical ingredients usually would be considered firstly. Besides, some therapeutic agents are of intrinsic toxicity such as selenium, whose therapeutic dose is close to toxic one and chronic selenium toxicity will damage major organs such as liver, spleen, and kidneys (Spallholz, 1994; Zhai et al., 2017; Yang Y. et al., 2021). And it is necessary to reduce toxicity with advance approaches such as nanotechnologies until meeting safety standards (Figure 3A). Specifically, through improving stability, controlled drug loading and release (Cha et al., 2018), non-specific distribution (Yu H. et al., 2023), and efficacy (Chung et al., 2013), etc., nanotechnology could render the drug administration in a low does and reduced frequency leading to less side-effects and better patient compliance (Xu et al., 2020b). Besides, the shape, size, and surface functionalization of drugs could be engineered by nanotechnology for more biocompatible and better medical application (Xu L. et al., 2020). For instance, inorganic materials with clear therapeutic effects like cerium oxide nanoparticles (CeNPs) have been studied and applied to ICH (Kang et al., 2017; Zheng et al., 2021) due to its superoxide dismutase mimetic and catalase mimetic properties for excess ROS scavenging (Bao et al., 2018; Zeng et al., 2018). To improve its biocompatibility, some researchers constructed new delivery systems such as the customized lipid-coated magnetic mesoporous silica nanoparticle doped with CeNPs (LMCs) (Cha et al., 2018). Briefly, CeNPs was loaded into biodegradable mesoporous silica nanoparticles (MSNs) and then capsulated by lipid bilayers. And the prepared LMCs with a surface charge (−1.35 mV) which is close to that of liposomes indicating the coating was successful and LMCs did demonstrate a great stability without agglomerations and retained the hydrodynamic size over a week. Moreover, with the high loading of CeNPs, LMCs demonstrated potent ROS scavenging capacity and could alleviate inflammation after ICH. In addition to inorganic nanomaterials, some organic materials such as polymer, nanoemulsion, and nanomicelles usually demonstrate low toxicity and biodegradability and are suit for clinical therapy, too. For instance, polyethyleneglycol (PEG) and polybutylcyanoacrylate (PBCA) are famous for low toxicity, non-immunogenicity, and stability, whereby they were usually employed to construct nanomaterials and applied in novel drug fabrication (Chung et al., 2013; Zheng et al., 2021). Specifically, through PEGylation, deferoxamine (DFO), the iron chelator (Holden and Nair, 2019), could be applied to remove excessive iron in ICH, whose cytotoxicity and poor hemocompatibility were improved effectively (Xu et al., 2020b). Specifically, in the cell viability assay, the cell viability of DFO group was 75% at a low concentration (0.05 mM) and decreased to 48.6% at a high concentration (0.5 mM), however, the PEGylated DFO groups demonstrated at least 85% cell viability at all concentration. Though the nanotechnology were extensively applied and elevated the biocompatibility of some drugs, the potential adverse effects or toxicity of the nanomaterials themselves should be concerned, too. There are some critical factors associated with the toxicity of nanomaterials including size, shape, surface charge, hydrophobicity, and the functional groups, however, surface modification techniques are effective in improving the biocompatibility of nanomaterials (Wei et al., 2023). As for central nervous systems, some metal-based nanomaterials such as copper, silver, and aluminum will damage BBB though it will facilitate drug delivery to brain after ICH (Zhang et al., 2023). Moreover, nanomaterials are easier in initiating responses of immune systems than small molecules and may damage organelles and DNA (Pallardy et al., 2017; Alexander et al., 2019). Thus, the safety of nanomaterials should be more considered and more investigation on their toxicity particularly after long term administration are necessary. And for the sake of safety, selecting more biocompatible materials, targeted delivery, and applying advanced technologies such as surface modification to improve the adverse effects of nanomaterials are promising.
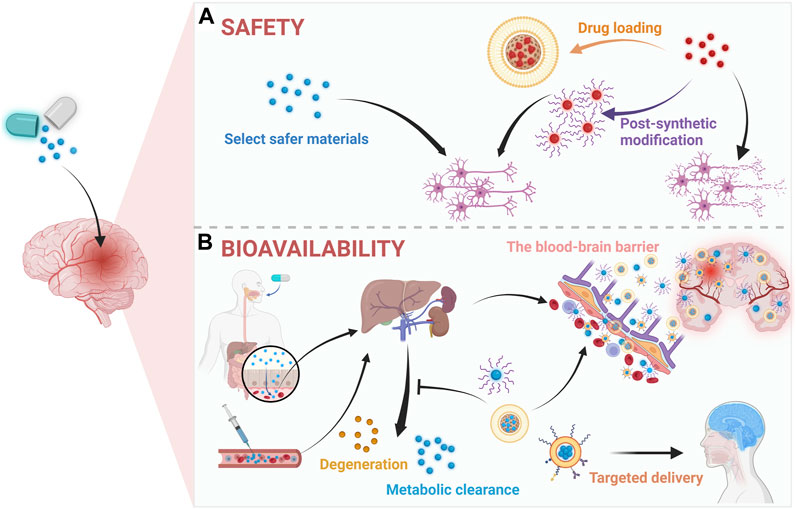
FIGURE 3. Application of nanotechnology in (A) safety and (B) bioavailability for therapeutic agents in ICH. Created with Biorender.com.
3.2 Bioavailability
The ability of a kind of drugs or active pharmaceutical ingredients to exist at a certain concentration in target areas is commonly termed as bioavailability (Al-Kassas et al., 2017; Davis and Walker, 2018). Accordingly, the key to improve drug bioavailability is to maintain the enough drug concentration in the blood circulation or prolong their half-life. In recent years, as shown in Figure 3B, nanomaterials had contributed significantly to achieving this goal, for instance, by PEGylation the half-life of DFO was increased 20 folds in plasma (Xu et al., 2022; Yu H. et al., 2023; Zhang et al., 2023). And a common approach is to employ nanomaterials such as PEG and PBCA (Cha et al., 2018; Dharmalingam et al., 2020), by which the poor solubility and short half-time of drugs will be significantly improved such as the case of Resveratrol (Res) (Mo et al., 2021). Specifically, based on the methoxy poly (ethylene glycol)-poly (L-lactide-co-glycolide) (MPEG-PLGA), the Res-NPs was obtained through anti-solvent precipitation method. And by this, the poor water solubility, short half-life, and blood-brain barrier (BBB) penetration of Res were improved. Specifically, in the pharmacokinetic assessment, the Res-NPs demonstrated a higher half-life than Res in both of plasma (7.32 ± 0.65 h vs. 2.13 ± 0.27 h) and brain (11.36 ± 1.28 h vs. 7.95 ± 0.64 h). In addition to prolonging the half-life, targeted delivery approaches aiming to transport the drug to specific positions or cells are also highly promising (Yu W. et al., 2023). For example, rosuvastatin, a neuroprotective agent, is hard to directly use in ICH due to its toxicity and poor bioavailability (White, 2002). Nevertheless, encapsulating it in self-assembled nanomicelles basing poly (ethylene glycol)-block-poly (ε-caprolactone) (PEG-PCL) copolymers not only extended half-life of rosuvastatin, but also improved the targeted effects towards inflammation for lower dose and frequency (Zi et al., 2021). Specifically, the rosuvastatin nanomicelles demonstrated better efficacy in improving neurological deficit than rosuvastatin alone, which might be attributed to the better bioavailability of rosuvastatin nanomicelles since both of them are orally administrated with the same dose. Moreover, the advancement of nanotechnologies has enabled the integration of many building blocks into nanomaterials, leading to more efficient targeted drug delivery through selective binding between biomaterials such as antibodies and antigens. For instance, through modifying the nanomaterials with anti-CD47 antibodies to block CD47, the drug’s half-life could be extended by reducing clearance by phagocytes (Jaiswal et al., 2009), and more advantages in targeting tumor cells were demonstrated (Luo et al., 2023). Given the outstanding advantages of combining biomimetic functions derived from cell membrane with the flexibility of material chemistry, various types of cell membrane including leukocyte, erythrocyte, platelet, macrophage, and cancer cell, etc., disguised nanomaterials were constructed in recent years for different targets (Li et al., 2018). Noteworthily, cell membrane disguised nanomaterials will be recognized as autogenous cells in vivo, which effectively dampens the immune system elimination whereby the half-life in the circulation is prolonged. Specifically, by coated with macrophage membrane, not only the half-life of nanomaterials will be prolonged, but also the BBB penetration can be enhanced via membrane receptor-ligand interactions, which increases the bioavailability of therapeutic agents for ICH (Lopes et al., 2022). Additionally, by coating with platelet membrane, an effective targeted delivery system for ICH was established basing the biological function of platelet for targeting and repairing damaged vessels, whereby the platelet-membrane-coated polydopamine nanoparticles successfully improved ICH induced injuries (Xu et al., 2023). Thus, the combination of these new technologies and materials could facilitate the treatment of ICH by improving the bioavailability of therapeutic agents.
3.3 Potential targets in ICH and functionalities of nanomaterials
Besides contributing to the improvement of the drug safety and bioavailability, nanotechnology also plays a crucial role in enhancing the therapeutic effects of drugs that target different pathological processes in ICH (Table 2). Specifically, as shown in Figure 4, therapeutic agents assisted by nanomaterials have demonstrated better performance in reducing hematoma (Park et al., 2017; Xu et al., 2023), ferroptosis (Mo et al., 2021), oxidative stress (Zheng et al., 2021), inflammation (Fu et al., 2021), and apoptosis (Chung et al., 2013) in ICH treatment.
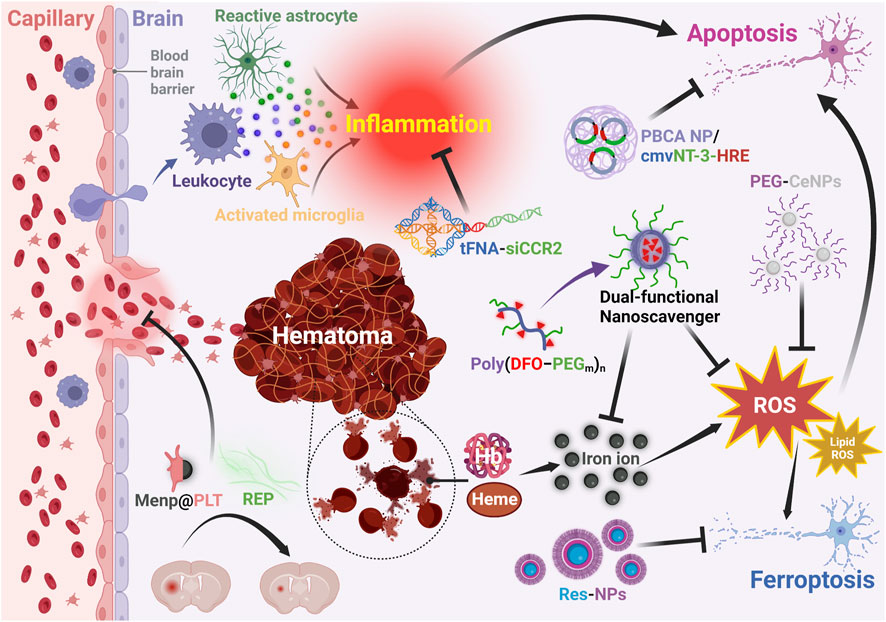
FIGURE 4. Functions of nanotechnology assisted therapeutic agents for ICH. PBCA: polybutylcyanoacrylate; NP: nanoparticle; cmv: cytomegalovirus; NT-3: neurotrophin-3; HRE: containing hormone response element; tFNA: tetrahedral framework nucleic acid; siCCR2: C-C chemokine receptor 2; PEG: polyethylene glycol; CeNPs: cerium oxide nanoparticles; DFO: deferoxamine; ROS: reactive oxygen species; Menp: polydopamine; PLT: platelet; REP: the repetitive integrin-binding ArgGlyAsp peptide -containing elastin-like polypeptide; Res: resveratrol. Created with Biorender.com.
3.3.1 Hematoma
As aforementioned, the hematoma size dictates the outcomes of ICH patients to a great extent (Hanley et al., 2019). Although large hematomas could be eliminated by various surgeries, many patients are not suitable for surgical management due to various reasons (Wilkinson et al., 2018a). Specifically, surgery is deemed of life saving in supratentorial ICH, however, the benefits of surgery are not clear for stable ICH patients or those in coma (Hemphill et al., 2015; Cordonnier et al., 2018b). Further, limited by the deep location of hematoma in the brain for some ICH patients and potential complications of surgery, removing hematoma by surgery failed to demonstrate significantly improved outcomes after ICH (Mendelow et al., 2005). Therefore, many nanomaterials based drugs targeting hematoma resolution were developed such as IL-4 protein loaded liposome NPs, which could facilitate hematoma resolution and function recovery after ICH through IL-4/STAT6/ST2 signaling (Xu et al., 2020a). Besides, platelet-membrane-modified polydopamine NPs (Menp@PLT) also reduced hematoma size after ICH by stopping bleeding through repairing the damaged vessels after ICH (Xu et al., 2023). Noteworthily, the Menp@PLT reduced more hematoma volume than Menp alone at 3 days after ICH. For instance, based on a thermally responsive biopolymer, modified elastin-like polypeptide (ELP), ArgGlyAsp (RGD) peptide containing ELP (REP) was fabricated (Park et al., 2017). And after ICH, REP reduced more hematoma volume significantly than RGD or ELP alone within 48 h after ICH through covering the broken vessels and preventing further bleeding. Thanks to it, not only less blood components were leaked, but also the activation of microglia and the expression of von Willebrand Factor (vWF) were inhibited, which led to reduced injuries in ICH. Moreover, by combining surgical hematoma aspiration with intrastriatally administrated RADA16-I (Sang et al., 2015), the hematoma volume was significantly reduced, and the residual cavity could be replaced that facilitated the neuron protection and sensorimotor functional recovery. In this case, the RADA16-I, a kind of self-assembled peptide nanofiber scaffolds (SAPNS), could form stable β-sheet structures which could further transform into hydrogels due to its regular repeats of ionic hydrophilic and hydrophobic amino acids (Schneider et al., 2008). Owing to this, the SAPNS was proven to provide supportive effects to various mammalian cells such as rat neuronal cells (Holmes et al., 2000; Kisiday et al., 2002), and it could facilitate the recovery of brain damages induced by hematoma in ICH. Noteworthily, there are other hematoma reducing therapeutic agents such as vitamin D (Liu et al., 2022) and bexarotene (Chang et al., 2020), whose effects are also hopefully being enhanced by exploiting nanocarriers, self-assembled nanomaterials, and so on.
3.3.2 Ferroptosis
With the time passed, the erythrocytes in the hematoma will break down leading to an enormous release of hemoglobin (Hb), heme, and so on (Wang et al., 2021). Further degradation of heme causes the release of overwhelming free iron, resulting in iron overload and dysregulation of iron metabolism (Garton et al., 2016). This leads to the exaggeration of secondary brain injuries particularly ferroptosis, a newly identified programmed cell death that exacerbates neurological outcomes in ICH patients (Xie et al., 2016). To alleviate this condition, researchers extensively studied and utilized some pharmaceutical ingredients such as curcumin (Yang C. et al., 2021) and resveratrol (Res) (Mo et al., 2021) based nanomaterials to attenuate ferroptosis development after ICH through suppressing ROS generation and providing neuroprotective effects. For example, to gain better efficacy of Res, nanotechnology was employed to alleviate its poor water solubility, short half-life, and poor BBB penetration. Specifically, by embedding the Res in the MPEG-PLGA based polymer nanomaterials via an antisolvent precipitation method, more bioavailable Res-based NPs were obtained and demonstrated elevated efficacy in suppressing erastin induced ferroptosis in HT22 mouse hippocampal cells, in which the Res-NPs exerted more neuroprotection than Res alone demonstrated in both of cell viability assay and LDH release assay (Mo et al., 2021). Additionally, given the iron play a pivotal role in ferroptosis after ICH, directly removing the excessive iron after ICH is also a promising strategy (Wan et al., 2019). Thus, it is reasonable to further develop iron chelator such as DFO (Xu et al., 2020b) and minocycline (Cao et al., 2018) based nanomaterials against ICH induced ferroptosis.
3.3.3 Oxidative stress
Oxidative stress, characterized by ROS, is a critical threat and a significant therapeutic target in ICH. Excessive ROS derives from various sources including mitochondria dysfunction, Hb-Heme-Iron, and inflammatory factors (Hu et al., 2016). To relieve oxidative stress and maintain the redox balance, nanomaterials including BMSC-exosomal miR-23b (Hu et al., 2023), Se@SiO2 nanocomposite (Yang Y. et al., 2021), LMCs (Cha et al., 2018), CeNPs (Zheng et al., 2021), quercetin-loaded nanoemulsion (Galho et al., 2016), and curcumin-loaded nanoemulsion (Marques et al., 2020) based therapies were developed in recent time. Among these, CeNPs were increasingly applied as potent scavengers of ROS and reactive nitrogen species (RNS), and demonstrated outstanding neuroprotective effects (Kim et al., 2012; Mracsko and Veltkamp, 2014). By passing through the damaged BBB in the hemorrhagic hemisphere, CeNPs effectively reduced oxidative stress, hemin-induced cytotoxicity, and inflammatory responses particularly the microglia/macrophage recruitment and inflammatory mediates release that leads to less brain edema (Kang et al., 2017). Moreover, drug loading or PSM were employed to satisfy more practical demands. For example, CeNPs modified with PEG (PEG-CeNPs) possessed better biocompatibility, less interparticle agglomeration, and success in improving white matter injury after ICH (Zheng et al., 2021). Additionally, loading CeNPs into the MSNs and coating them with a lipid bilayer not only realized the regulation of the loading and release of CeNPs, but also significantly strengthened their biocompatibility and functions (Cha et al., 2018). Apart from CeNPs, the 2,2,6,6-tetramethylpiperidine-1-oxyl (TEMPO) is famous for its potent ROS scavenging capacity as well (Samuni et al., 1988; Hahn et al., 1995), whereas its problems including nonspecific dispersion in normal tissues, fast degradability, and rapid renal clearance remain to be solved before its application in ICH. To this end, based on PEG-b-poly [(TEMPO) amino-methylstyrene] (PMNT) and PEG-b-poly [(TEMPO) oxy-methylstyrene] (PMOT), two kinds of redox polymer self-assembled nitroxide radical-containing nanoparticles (RNPs) were fabricated and demonstrated better bioavailability and great performances in reducing oxidative stress, brain edema, and neurologic deficits, which might be attributed to their longer half-life in plasma and brain than TEMPO (Chonpathompikunlert et al., 2012). Additionally, from the perspective of reducing oxidative stress without breaking the redox balance in normal tissues, it is necessary to develop target nanomaterials such as ROS responsive ones to improve the nonspecific dispersion. Specifically, the therapeutic agents would be released when the nanomaterials meet excessive intracellular ROS whereby a targeted delivery system was established (Yuan et al., 2021; Guo et al., 2023). Additionally, as mentioned before, through the function of platelet for targeting and repairing damaged vessels, polydopamine nanoparticles platelet-membrane-coated polydopamine nanoparticles were precisely delivered to the hemorrhage site after ICH and successfully exerted neuroprotection against oxidative stress (Xu et al., 2023). Given microglia and macrophage play great roles in ICH, targeting them to improve outcomes of ICH through a targeted therapy such as a phosphatidylserine liposome-based nanoparticle system is also worth to be considered (Han et al., 2023).
3.3.4 Inflammation
Inflammatory responses, including the release of various cytokines and the recruitment of inflammatory cells, are common and important in injuries. However, overreacted inflammation will exacerbate the primary diseases (Hajishengallis and Chavakis, 2019). Accordingly, it is of great significance to take measures to palliate inflammatory responses properly in ICH. Several nanomaterials including rosuvastatin-loaded nanomicelles (Zi et al., 2021), dauricine-loaded p-PCa4C12 micelles (Li et al., 2020), iron oxide NPs-loaded human embryonic stem cell-derived spherical neural masses (Kang et al., 2020), and RADA16mix (Zhang et al., 2016) have been developed to target the inflammation. For instance, C-C chemokine receptor 2 (CCR2) is well-known for its roles in inflammatory diseases (Raghu et al., 2017; Tan et al., 2019; Pedragosa et al., 2020), and down-regulating its expression is deemed as a promising target in inflammation after ICH. To achieve this, tFNA-siCCR2 was established by loading siCCR2 into the tetrahedral framework nucleic acid (tFNA), a nanorobotic of editability, biocompatibility and low toxicity. And after intraventricularly administrated, the neuroinflammation in a mouse model of ICH was attenuated through regulating the polarization of microglia from M1 to M2, inhibiting the release of inflammatory mediators, and elevating the expression of anti-inflammatory factors. It led to accelerated hematoma absorption and partial preservation of motor nerve function (Fu et al., 2021). Since the complex effects of inflammation and its duration in ICH (Ohashi et al., 2023), it is important to properly realize the inflammation resolution instead of inhibiting inflammatory responses roughly (Kourtzelis et al., 2019). To this end, further explore drug administration in a controlled releasing method basing nanotechnology might be more appropriate in treating ICH (Hsu and Almutairi, 2021).
3.3.5 Apoptosis
Apoptosis, in addition to its significant roles in physiological conditions, is deeply involved in diseases including ICH (Chen et al., 2020). After the onset of the ICH, the deterioration of the secondary injury results in increased cell death particularly the neuronal apoptosis, which is related to poor prognosis such as neurological deficits and disabilities (Puy et al., 2023). Thus, nanomaterials were being developed as potential approaches to alleviate neuronal apoptosis in ICH, including polymer (Chung et al., 2013) and exosomes (Shen et al., 2018; Duan et al., 2020). For instance, previous studies have reported that neurotrophin-3 (NT-3) had neuroprotective effects particularly in apoptosis models (Bouzas-Rodriguez et al., 2010), neurogenesis (Hao et al., 2017), and differentiation of stem cells (Yang et al., 2010). And some researchers employed cytomegalovirus (cmv) promoter controlled plasmid NT-3 containing hormone response element (HRE) to boost gene regulation and the increased NT-3 reduced ICH induced neuronal apoptosis (Chung et al., 2013). Specifically, polybutylcyanoacrylate nanoparticles (PBCA NPs) were taken as the drug delivery system due to great stability, bioavailability, low toxicity, and non-immunogenicity, which facilitated BBB penetration and prevented rapid degradation of the therapeutic agent. Consequently, the enrichment of PBCA NP/cmvNT-3-HRE complexes in the brain resulted in increased expression of NT-3, which led to significant inhibition of apoptosis-inducing factor, cleaved caspase-3, and DNA fragmentation. By these, the neuronal loss after ICH was effectively reduced by PBCA NP/cmvNT-3-HRE complexes whose neuroprotection was better than cmvNT-3-HRE reflected by both of TUNEL staining and Nissl staining. Pathologically, both of inflammation and oxidative stress will aggravate the neuronal apoptosis (Tian et al., 2023), thus, further developing multifunctional therapeutic agents which could inhibit inflammation, oxidative stress, and apoptosis simultaneously would be reasonable and effective.
3.3.6 Multifunction
Although the aforementioned approaches effectively alleviated the damages in ICH, most of them target a single aspect. However, after ICH, various injuries with complex mechanisms are interconnected and mutually influenced (Bautista et al., 2021). Accordingly, there is a practical and necessary demand to develop some multi-targeted therapies that can simultaneously address different injuries. One example is the use of core-shell hydrogel based multifunctional nanomaterials, which could reduce excessive iron as well as effectively support stem cell-based therapy in ICH (Gong et al., 2020). Noteworthily, deferoxamine (DFO), a naturally siderophore applied in improving iron overload related diseases (Camaschella et al., 2020), has been increasingly integrated into multifunctional nanomaterials construction. For instance, DFO-hydrophilic carbon clusters (HCC)-PEG nanomaterials were developed to target both cellular senescence and ferroptosis after ICH (Dharmalingam et al., 2020). Additionally, by employing the 3,4-dihydroxyhydrocinnamic acid-DFO conjugate (Cat-DFO) as the building block, the polymeric DFO [poly (DFO)n] was then fabricated through horseradish peroxidase (HRP)- and hydrogen peroxide (H2O2)-catalyzed polymerization (Zhu et al., 2021). In which, by modifying the water-insoluble polymeric DFO [poly (DFO)n] with by PEG2k-NH2, it was successfully transformed into amphiphilic [poly (DFO-PEGm)n] with self-assembly property. This dual-functional nanoscavenger effectively decreased much more iron and ROS than DFO alone, resulting in reduced cell death. To further develop the DFO based multi-target nanomaterials, polyphenols with ROS-scavenging capacity were incorporated into carrier-free nanoparticles with high DFO-loading (∼80%) (Zhu et al., 2023b). The participation of polyphenols not only prolonged the drug’s half-life by restricting DFO’s metabolic clearance, but also realized better protection for brain cells after ICH due to the capacity of scavenging both excessive iron and ROS. Similarly, boronic acid modified DFO (PBA-DFO) was further modified with a series of natural polyphenols, resulting in natural polyphenols-boosted DFO NPs through supramolecular assembly (Zhu et al., 2023a). Importantly, this work presented a general strategy in which uniform and stable nanoparticles could be generated by the combination of natural polyphenols moiety and DFO. The NPs in this strategy not only removed excessive iron but also eliminated excessive ROS and attenuated inflammatory responses after ICH. This shed light on the promising prospect of multi-target nanomaterials in ICH. Overall, these complicated works with multi-target drug designs are insufficient in ICH. Apart from iron overload and oxidative stress, other pathological process in ICH comprising hematoma, edema, inflammation, ferroptosis, apoptosis, function recovery, etc., as targets are also worth to be integrated in multifunctional drugs design basing on nanotechnology. Notably, the functional recovery after ICH also is intractable. Thus, combining therapeutic nanomaterials with functional recovery therapies such as stem cell-based therapy is of potential, too (Gong et al., 2020). Further, given the imaging based diagnosis are quite important in ICH (Cordonnier et al., 2018b), it is necessary to develop theragnostic agents through incorporating with advanced nanomaterials based imaging technology (Ran and Xue, 2018; Szwargulski et al., 2020).
4 Conclusion and perspectives
In addition to conventional therapies against ICH (Figure 5A), based on the comprehensive illustration of outstanding works targeting ICH, this review primarily focuses on the role of nanotechnology in novel drugs development particularly in the safety, bioavailability, and functionality (Figure 5B). Safety, as a cornerstone of the drug development, can be ensured through two approaches: selecting safe materials and reducing the drug toxicity with advanced technologies typified by nanotechnologies such as drug loading techniques and PSM. For the bioavailability, the drug’s half-life in blood determines the dosage and administration frequency. However, many potential drug candidates have poor bioavailability due to insolubility in water, fast metabolic clearance in the circulation, first pass effect and difficulty in passing the physiological barriers (Xu et al., 2022; Yu W. et al., 2023; Zhang et al., 2023). To address these challenges, various nanomaterials fabricated through polymerization, self-assembly and microfluidics were employed to modify or carry drugs pursuing enhanced bioavailability. The emergence and application of biomaterials facilitated the introduction of cells and cellular membranes into nano delivery systems, enhanced biocompatibility, prolonged half-life, and facilitated the passage of physiological barriers, such as the blood-brain barrier (BBB) (Xia et al., 2020; Li et al., 2021; Xu et al., 2023). Additionally, in recent years, targeted delivery systems were increasingly preferred by researchers since their high efficiency and less side-effects, leading to better therapeutic performance. Specifically, the drug delivery could be more precise and efficient with the help of the selective banding between the biomaterials including antigens and antibodies, ligands and receptors, etc (Luo et al., 2023; Shang et al., 2023). Apart from safety and bioavailability, the functionality of the therapeutic agent is another determining factor for its clinical application. And in ICH, both the primary injury and the secondary injury are pivotal targets for clinical intervention. Specifically, increasingly preclinical drugs were applied to address the hematoma, ferroptosis, oxidative stress, inflammation, and apoptosis, etc (Xu et al., 2022; Zhang et al., 2023). And in their development, by realizing reduced nonspecific dispersion, controlled loading and release, and enhanced efficacy, these potential drug candidates demonstrated better performances against ICH. Further, benefiting from the outstanding engineerability of nanomaterials, comprehensive treatments for ICH was achieved through integrating pharmaceutical ingredients with different targets. Thus, it seemed to be more promising to develop multi-target drugs. Overall, after ICH, the heavy primary injury could be handled with medical and surgical managements (Puy et al., 2023), however, the secondary injury still needs to be prevented and intervened with effective approaches to improve the prognosis of ICH patients. Specifically, further clearing hematoma, inhibiting ferroptosis, oxidative damages, inflammatory responses, and apoptosis are pivotal targets. For these targets, besides therapeutic nanomaterials, more conventional preclinical drugs could be applied alone or combinedly after being improved in safety, bioavailability, and efficacy by nanotechnology such as modification and nano drug delivery systems (Yu et al., 2022). Apart from reducing injuries, the functional recovery after ICH also needs attention, thus, it is reasonable to improve present functional recovery therapies with nanotechnology (Gong et al., 2020).
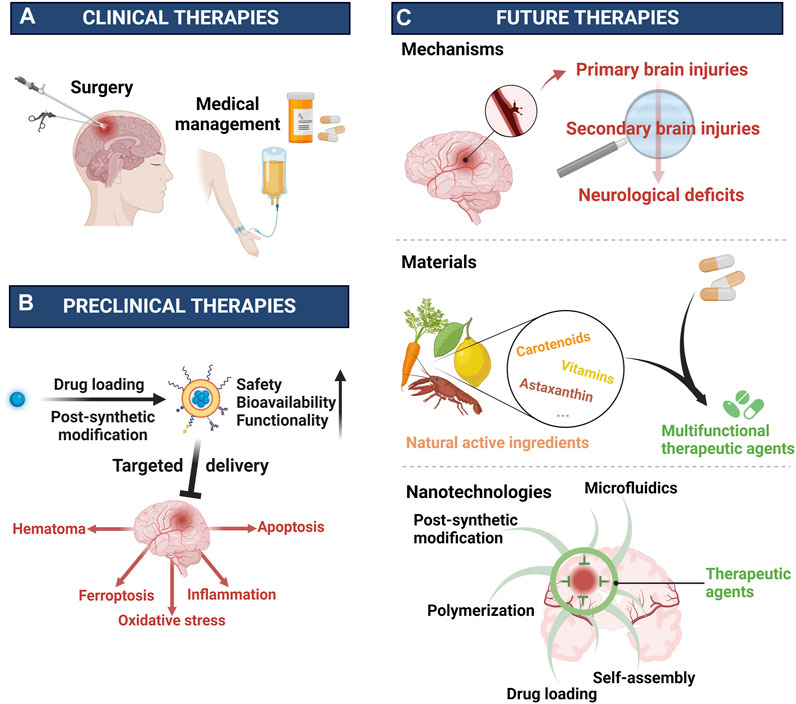
FIGURE 5. Clinical, preclinical, and future therapies for ICH (A) Clinical therapies are surgery and medical management. (B) Preclinical therapies are improved by nanotechnologies including drug loading, post-synthetic modification, etc. (C) Future therapies need to explore more pathological mechanisms underlying ICH, fabricate more multifunctional materials, and develop more advanced technology such as nanotechnology. Created with Biorender.com.
Despite impressive achievements achieved with the support of nanotechnology in ICH, there are still significant challenges in translating research findings from the lab to clinical practice. Frankly, compared with cancer and other diseases, the exploration of mechanisms or therapies in ICH remains insufficient and needs more efforts (Figure 5C) (Shi et al., 2023). Thus, it is reasonable to learn from the latest advances in other diseases particularly cancer (Liang et al., 2020; Peng et al., 2022; Wang et al., 2022), hemorrhagic diseases (Zhuang et al., 2018), ischemic diseases (Chen et al., 2022; Zhang et al., 2022), and iron overloaded diseases (Yang et al., 2020; Zhao et al., 2021; Lu et al., 2022), etc. Besides, further exploration of nanotechnology to design multifunctional nanomaterials holds promising prospects. Polymeric nanomaterials with their diverse structures, offer great convenience in integrating with other therapeutic agents (Xu et al., 2018; Wang Y. et al., 2019; Zhang et al., 2021b). And for the sake of safety and convenience, many natural active ingredients including natural polyphenols (Zhu et al., 2023a), astaxanthin (Cai et al., 2022), vitamins (Lee et al., 2015), carotenoids (Harmatys et al., 2019), etc., are drawing increasingly attention in diseases therapies since they are both of natural therapeutic agents and outstanding building blocks for constructing multifunctional drugs (Figure 5C). For instance, the polyphenols, known for their versatile structures including catechol and pyrogallol moieties, can facilitate chemically crosslinking via oxidation and interact with nucleophilic groups through the oxidized quinone forms (Yang P. et al., 2021). In addition to covalently connection, polyphenols can also engage in hydrogen bonding, electrostatic interactions and π–π electron interactions (Zhang et al., 2021a). This versatility provides significant advantages in designing multi-target drugs by combining different agents. In addition to materials, further exploration of current nanotechnology such as post-synthetic modification, self-assembly and drug loading techniques, as well as employing biomaterials and developing innovative technologies, are critical in this field (Figure 5C). Lastly, besides therapy, prevention and diagnosis are of great importance in diseases management. As for ICH, its diagnosis as well as subsequent therapeutic strategy are largely depending on the imaging technology where nanotechnology is of great potential (Graeser et al., 2019; Szwargulski et al., 2020; Mazurek et al., 2021). Therefore, further developing nanomaterials based theragnostic agents is necessary for the generalization of precision medicine and personalized healthcare in ICH.
Author contributions
JW: Writing–original draft. TW: Writing–original draft. MF: Writing–original draft. ZW: Investigation, Visualization, Writing–original draft. WX: Investigation, Visualization, Writing–original draft. BT: Investigation, Visualization, Writing–original draft. QY: Conceptualization, Supervision, Writing–review and editing. XH: Conceptualization, Supervision, Writing–review and editing.
Funding
The authors declare financial support was received for the research, authorship, and/or publication of this article. This work was supported by the 1·3·5 Projects for Disciplines of Excellence of West China Hospital of Sichuan University (grant numbers: ZY2016102 and 2021HXFH045), the National Natural Science Foundation of China (grant numbers: 81601155 and 82201453), the Sichuan Science and Technology Program (grant numbers: 2020YFQ0009 and 2023NSFSC1557), and the Project funded by China Postdoctoral Science Foundation (grant number: 2022M722271).
Conflict of interest
The authors declare that the research was conducted in the absence of any commercial or financial relationships that could be construed as a potential conflict of interest.
The reviewer CH declared a shared parent affiliation with the authors to the handling editor at the time of review.
Publisher’s note
All claims expressed in this article are solely those of the authors and do not necessarily represent those of their affiliated organizations, or those of the publisher, the editors and the reviewers. Any product that may be evaluated in this article, or claim that may be made by its manufacturer, is not guaranteed or endorsed by the publisher.
References
Al-Kassas, R., Bansal, M., and Shaw, J. (2017). Nanosizing techniques for improving bioavailability of drugs. J. Control. Release 260, 202–212. doi:10.1016/j.jconrel.2017.06.003
Alexander, A., Agrawal, M., Uddin, A., Siddique, S., Shehata, A. M., Shaker, M. A., et al. (2019). <p>Recent expansions of novel strategies towards the drug targeting into the brain</p>. Int. J. Nanomed. 14, 5895–5909. doi:10.2147/IJN.S210876
Bao, Q., Hu, P., Xu, Y., Cheng, T., Wei, C., Pan, L., et al. (2018). Simultaneous blood-brain barrier crossing and protection for stroke treatment based on edaravone-loaded ceria nanoparticles. ACS Nano 12 (7), 6794–6805. doi:10.1021/acsnano.8b01994
Bautista, W., Adelson, P. D., Bicher, N., Themistocleous, M., Tsivgoulis, G., and Chang, J. J. (2021). Secondary mechanisms of injury and viable pathophysiological targets in intracerebral hemorrhage. Ther. Adv. Neurol. Disord. 14, 175628642110492. doi:10.1177/17562864211049208
Bejot, Y., Blanc, C., Delpont, B., Thouant, P., Chazalon, C., Daumas, A., et al. (2018). Increasing early ambulation disability in spontaneous intracerebral hemorrhage survivors. Neurology 90 (23), e2017–e2024. doi:10.1212/WNL.0000000000005633
Bouzas-Rodriguez, J., Cabrera, J. R., Delloye-Bourgeois, C., Ichim, G., Delcros, J. G., Raquin, M. A., et al. (2010). Neurotrophin-3 production promotes human neuroblastoma cell survival by inhibiting TrkC-induced apoptosis. J. Clin. Invest. 120 (3), 850–858. doi:10.1172/JCI41013
Broderick, J. P., Brott, T. G., Duldner, J. E., Tomsick, T., and Huster, G. (1993). Volume of intracerebral hemorrhage. A powerful and easy-to-use predictor of 30-day mortality. Stroke 24 (7), 987–993. doi:10.1161/01.str.24.7.987
Cai, X., Hua, S., Deng, J., Du, Z., Zhang, D., Liu, Z., et al. (2022). Astaxanthin activated the Nrf2/HO-1 pathway to enhance autophagy and inhibit ferroptosis, ameliorating acetaminophen-induced liver injury. ACS Appl. Mat. Interfaces 14 (38), 42887–42903. doi:10.1021/acsami.2c10506
Camaschella, C., Nai, A., and Silvestri, L. (2020). Iron metabolism and iron disorders revisited in the hepcidin era. Haematologica 105 (2), 260–272. doi:10.3324/haematol.2019.232124
Campbell, B. C. V., and Khatri, P. (2020). Stroke. Lancet 396 (10244), 129–142. doi:10.1016/s0140-6736(20)31179-x
Cao, S., Hua, Y., Keep, R. F., Chaudhary, N., and Xi, G. (2018). Minocycline effects on intracerebral hemorrhage-induced iron overload in aged rats: brain iron quantification with magnetic resonance imaging. Stroke 49 (4), 995–1002. doi:10.1161/STROKEAHA.117.019860
Cha, B. G., Jeong, H. G., Kang, D. W., Nam, M. J., Kim, C. K., Kim, D. Y., et al. (2018). Customized lipid-coated magnetic mesoporous silica nanoparticle doped with ceria nanoparticles for theragnosis of intracerebral hemorrhage. Nano Res. 11 (7), 3582–3592. doi:10.1007/s12274-017-1924-5
Chang, C. F., Goods, B. A., Askenase, M. H., Hammond, M. D., Renfroe, S. C., Steinschneider, A. F., et al. (2018). Erythrocyte efferocytosis modulates macrophages towards recovery after intracerebral hemorrhage. J. Clin. Invest. 128 (2), 607–624. doi:10.1172/JCI95612
Chang, C. F., Massey, J., Osherov, A., Angenendt da Costa, L. H., and Sansing, L. H. (2020). Bexarotene enhances macrophage erythrophagocytosis and hematoma clearance in experimental intracerebral hemorrhage. Stroke 51 (2), 612–618. doi:10.1161/STROKEAHA.119.027037
Chen, S. J., Yuan, X. Q., Xue, Q., Lu, H. F., and Chen, G. (2022). Current research progress of isoflurane in cerebral ischemia/reperfusion injury: a narrative review. Med. Gas. Res. 12 (3), 73–76. doi:10.4103/2045-9912.330689
Chen, S., Peng, J., Sherchan, P., Ma, Y., Xiang, S., Yan, F., et al. (2020). TREM2 activation attenuates neuroinflammation and neuronal apoptosis via PI3K/Akt pathway after intracerebral hemorrhage in mice. J. Neuroinflamm. 17 (1), 168. doi:10.1186/s12974-020-01853-x
Chonpathompikunlert, P., Fan, C. H., Ozaki, Y., Yoshitomi, T., Yeh, C. K., and Nagasaki, Y. (2012). Redox nanoparticle treatment protects against neurological deficit in focused ultrasound-induced intracerebral hemorrhage. Nanomedicine 7 (7), 1029–1043. doi:10.2217/nnm.12.2
Chung, C. Y., Yang, J. T., and Kuo, Y. C. (2013). Polybutylcyanoacrylate nanoparticles for delivering hormone response element-conjugated neurotrophin-3 to the brain of intracerebral hemorrhagic rats. Biomaterials 34 (37), 9717–9727. doi:10.1016/j.biomaterials.2013.08.083
Collaborators, G. B. D. S., Stark, B. A., Johnson, C. O., Roth, G. A., Bisignano, C., Abady, G. G., et al. (2021). Global, regional, and national burden of stroke and its risk factors, 1990-2019: a systematic analysis for the global burden of disease study 2019. Lancet Neurol. 20 (10), 795–820. doi:10.1016/S1474-4422(21)00252-0
Cordonnier, C., Demchuk, A., Ziai, W., and Anderson, C. S. (2018a). Intracerebral haemorrhage: current approaches to acute management. Lancet 392 (10154), 1257–1268. doi:10.1016/s0140-6736(18)31878-6
Cordonnier, C., Demchuk, A., Ziai, W., and Anderson, C. S. (2018b). Intracerebral haemorrhage: current approaches to acute management. Lancet 392 (10154), 1257–1268. doi:10.1016/S0140-6736(18)31878-6
Davis, M., and Walker, G. (2018). Recent strategies in spray drying for the enhanced bioavailability of poorly water-soluble drugs. J. Control. Release 269, 110–127. doi:10.1016/j.jconrel.2017.11.005
de Oliveira Manoel, A. L. (2020). Surgery for spontaneous intracerebral hemorrhage. Crit. Care 24 (1), 45. doi:10.1186/s13054-020-2749-2
Dharmalingam, P., Talakatta, G., Mitra, J., Wang, H., Derry, P. J., Nilewski, L. G., et al. (2020). Pervasive genomic damage in experimental intracerebral hemorrhage: therapeutic potential of a mechanistic-based carbon nanoparticle. ACS Nano 14 (3), 2827–2846. doi:10.1021/acsnano.9b05821
Duan, S., Wang, F., Cao, J., and Wang, C. (2020). <p>Exosomes derived from MicroRNA-146a-5p-enriched bone marrow mesenchymal stem cells alleviate intracerebral hemorrhage by inhibiting neuronal apoptosis and microglial M1 polarization</p>. Drug Des. devel. Ther. 14, 3143–3158. doi:10.2147/DDDT.S255828
Elkind, M. S. V., and Hankey, G. J. (2022). Advances in epidemiology, outcomes, and population science. Stroke 53 (11), 3481–3484. doi:10.1161/STROKEAHA.122.039313
Figueroa-Quintero, L., Villalgordo-Hernández, D., Delgado-Marín, J. J., Narciso, J., Velisoju, V. K., Castaño, P., et al. (2023). Post-synthetic surface modification of metal–organic frameworks and their potential applications. Small Methods 7 (4), e2201413. doi:10.1002/smtd.202201413
Fu, W., Ma, L., Ju, Y., Xu, J. G., Li, H., Shi, S. R., et al. (2021). Therapeutic siCCR2 loaded by tetrahedral framework DNA nanorobotics in therapy for intracranial hemorrhage. Adv. Funct. Mat. 31 (33), 2101435. doi:10.1002/adfm.202101435
Galho, A. R., Cordeiro, M. F., Ribeiro, S. A., Marques, M. S., Antunes, M. F., Luz, D. C., et al. (2016). Protective role of free and quercetin-loaded nanoemulsion against damage induced by intracerebral haemorrhage in rats. Nanotechnology 27 (17), 175101. doi:10.1088/0957-4484/27/17/175101
Garton, T., Keep, R. F., Hua, Y., and Xi, G. (2016). Brain iron overload following intracranial haemorrhage. Stroke Vasc. Neurol. 1 (4), 172–184. doi:10.1136/svn-2016-000042
Gong, Y., Wang, Y., Qu, Q., Hou, Z., Guo, T., Xu, Y., et al. (2020). Nanoparticle encapsulated core-shell hydrogel for on-site BMSCs delivery protects from iron overload and enhances functional recovery. J. Control. Release 320, 381–391. doi:10.1016/j.jconrel.2020.01.029
Graeser, M., Thieben, F., Szwargulski, P., Werner, F., Gdaniec, N., Boberg, M., et al. (2019). Human-sized magnetic particle imaging for brain applications. Nat. Commun. 10 (1), 1936. doi:10.1038/s41467-019-09704-x
Gu, L., Sun, M., Li, R., Tao, Y., Luo, X., Xu, J., et al. (2022). Activation of RKIP binding ASC attenuates neuronal pyroptosis and brain injury via caspase-1/GSDMD signaling pathway after intracerebral hemorrhage in mice. Transl. Stroke Res. 13 (6), 1037–1054. doi:10.1007/s12975-022-01009-4
Guo, B., Yang, F., Zhang, L., Zhao, Q., Wang, W., Yin, L., et al. (2023). Cuproptosis induced by ROS responsive nanoparticles with elesclomol and copper combined with αpd-L1 for enhanced cancer immunotherapy. Adv. Mat. 35 (22), e2212267. doi:10.1002/adma.202212267
Hahn, S. M., Lepinski, D. L., DeLuca, A. M., Mitchell, J. B., and Pellmar, T. C. (1995). Neurophysiological consequences of nitroxide antioxidants. Can. J. Physiol. Pharmacol. 73 (3), 399–403. doi:10.1139/y95-051
Hajishengallis, G., and Chavakis, T. (2019). DEL-1-Regulated immune plasticity and inflammatory disorders. Trends Mol. Med. 25 (5), 444–459. doi:10.1016/j.molmed.2019.02.010
Han, R., Lan, X., Han, Z., Ren, H., Aafreen, S., Wang, W., et al. (2023). Improving outcomes in intracerebral hemorrhage through microglia/macrophage-targeted IL-10 delivery with phosphatidylserine liposomes. Biomaterials 301, 122277. doi:10.1016/j.biomaterials.2023.122277
Hanley, D. F., Thompson, R. E., Rosenblum, M., Yenokyan, G., Lane, K., McBee, N., et al. (2019). Efficacy and safety of minimally invasive surgery with thrombolysis in intracerebral haemorrhage evacuation (MISTIE III): a randomised, controlled, open-label, blinded endpoint phase 3 trial. Lancet 393 (10175), 1021–1032. doi:10.1016/S0140-6736(19)30195-3
Hao, P., Duan, H., Hao, F., Chen, L., Sun, M., Fan, K. S., et al. (2017). Neural repair by NT3-chitosan via enhancement of endogenous neurogenesis after adult focal aspiration brain injury. Biomaterials 140, 88–102. doi:10.1016/j.biomaterials.2017.04.014
Harmatys, K. M., Overchuk, M., and Zheng, G. (2019). Rational design of photosynthesis-inspired nanomedicines. Accounts Chem. Res. 52 (5), 1265–1274. doi:10.1021/acs.accounts.9b00104
Hemphill, J. C., Greenberg, S. M., Anderson, C. S., Becker, K., Bendok, B. R., Cushman, M., et al. (2015). Guidelines for the management of spontaneous intracerebral hemorrhage: A guideline for healthcare professionals from the American heart association/American stroke association. Stroke 46 (7), 2032–2060. doi:10.1161/STR.0000000000000069
Holden, P., and Nair, L. S. (2019). Deferoxamine: an angiogenic and antioxidant molecule for tissue regeneration. Tissue Eng. Part b-rev. 25 (6), 461–470. doi:10.1089/ten.TEB.2019.0111
Holmes, T. C., de Lacalle, S., Su, X., Liu, G., Rich, A., and Zhang, S. (2000). Extensive neurite outgrowth and active synapse formation on self-assembling peptide scaffolds. Proc. Natl. Acad. Sci. U. S. A. 97 (12), 6728–6733. doi:10.1073/pnas.97.12.6728
Hsu, P. H., and Almutairi, A. (2021). Recent progress of redox-responsive polymeric nanomaterials for controlled release. J. Mat. Chem. B 9 (9), 2179–2188. doi:10.1039/d0tb02190c
Hu, L. T., Wang, B. Y., Fan, Y. H., He, Z. Y., and Zheng, W. X. (2023). Exosomal miR-23b from bone marrow mesenchymal stem cells alleviates oxidative stress and pyroptosis after intracerebral hemorrhage. Neural Regen. Res. 18 (3), 560–567. doi:10.4103/1673-5374.346551
Hu, X., Tao, C., Gan, Q., Zheng, J., Li, H., and You, C. (2016). Oxidative stress in intracerebral hemorrhage: sources, mechanisms, and therapeutic targets. Oxidative Med. Cell. Longev. 2016, 1–12. doi:10.1155/2016/3215391
Huang, P. S., Wang, X. L., Liang, X. Y., Yang, J., Zhang, C. N., Kong, D. L., et al. (2019). Nano-micro-and macroscale drug delivery systems for cancer immunotherapy. Acta Biomater. 85, 1–26. doi:10.1016/j.actbio.2018.12.028
Jaiswal, S., Jamieson, C. H., Pang, W. W., Park, C. Y., Chao, M. P., Majeti, R., et al. (2009). CD47 is upregulated on circulating hematopoietic stem cells and leukemia cells to avoid phagocytosis. Cell 138 (2), 271–285. doi:10.1016/j.cell.2009.05.046
Kalisvaart, A. C. J., Abrahart, A. H., Coney, A. T., Gu, S., and Colbourne, F. (2022). Intracranial pressure dysfunction following severe intracerebral hemorrhage in middle-aged rats. Transl. Stroke Res. doi:10.1007/s12975-022-01102-8
Kang, D. W., Kim, C. K., Jeong, H. G., Soh, M., Kim, T., Choi, I. Y., et al. (2017). Biocompatible custom ceria nanoparticles against reactive oxygen species resolve acute inflammatory reaction after intracerebral hemorrhage. Nano Res. 10 (8), 2743–2760. doi:10.1007/s12274-017-1478-6
Kang, M. K., Kim, T. J., Kim, Y. J., Kang, L., Kim, J., Lee, N., et al. (2020). Targeted delivery of iron oxide nanoparticle-loaded human embryonic stem cell-derived spherical neural masses for treating intracerebral hemorrhage. Int. J. Mol. Sci. 21 (10), 3658. doi:10.3390/ijms21103658
Katsu, M., Niizuma, K., Yoshioka, H., Okami, N., Sakata, H., and Chan, P. H. (2010). Hemoglobin-induced oxidative stress contributes to matrix metalloproteinase activation and blood-brain barrier dysfunction in vivo. J. Cereb. Blood Flow. Metab. 30 (12), 1939–1950. doi:10.1038/jcbfm.2010.45
Kim, C. K., Kim, T., Choi, I. Y., Soh, M., Kim, D., Kim, Y. J., et al. (2012). Ceria nanoparticles that can protect against ischemic stroke. Angew. Chem.-Int. Ed. 51 (44), 11039–11043. doi:10.1002/anie.201203780
Kirshner, H., and Schrag, M. (2021). Management of intracerebral hemorrhage: update and future therapies. Curr. Neurol. Neurosci. Rep. 21 (10), 57. doi:10.1007/s11910-021-01144-9
Kisiday, J., Jin, M., Kurz, B., Hung, H., Semino, C., Zhang, S., et al. (2002). Self-assembling peptide hydrogel fosters chondrocyte extracellular matrix production and cell division: implications for cartilage tissue repair. Proc. Natl. Acad. Sci. U. S. A. 99 (15), 9996–10001. doi:10.1073/pnas.142309999
Kourtzelis, I., Li, X., Mitroulis, I., Grosser, D., Kajikawa, T., Wang, B., et al. (2019). DEL-1 promotes macrophage efferocytosis and clearance of inflammation. Nat. Immunol. 20 (1), 40–49. doi:10.1038/s41590-018-0249-1
Krishnamurthi, R. V., Feigin, V. L., Forouzanfar, M. H., Mensah, G. A., Connor, M., Bennett, D. A., et al. (2013). Global and regional burden of first-ever ischaemic and haemorrhagic stroke during 1990-2010: findings from the global burden of disease study 2010. Lancet Glob. Health 1 (5), e259–e281. doi:10.1016/S2214-109X(13)70089-5
Lee, J. S., Kim, K., Lee, K., Park, J. P., Yang, K., Cho, S. W., et al. (2015). Surface chemistry of vitamin: pyridoxal 5′-phosphate (vitamin B6) as a multifunctional compound for surface functionalization. Adv. Funct. Mat. 25 (30), 4754–4760. doi:10.1002/adfm.201501471
Li, C., Zhao, Z., Luo, Y., Ning, T., Liu, P., Chen, Q., et al. (2021). Macrophage-disguised manganese dioxide nanoparticles for neuroprotection by reducing oxidative stress and modulating inflammatory microenvironment in acute ischemic stroke. Adv. Sci. 8 (20), e2101526. doi:10.1002/advs.202101526
Li, M., Liu, G., Wang, K., Wang, L., Fu, X., Lim, L. Y., et al. (2020). Metal ion-responsive nanocarrier derived from phosphonated calix[4]arenes for delivering dauricine specifically to sites of brain injury in a mouse model of intracerebral hemorrhage. J. Nanobiotechnol. 18 (1), 61. doi:10.1186/s12951-020-00616-3
Li, R., He, Y., Zhang, S., Qin, J., and Wang, J. (2018). Cell membrane-based nanoparticles: a new biomimetic platform for tumor diagnosis and treatment. Acta Pharm. Sin. B 8 (1), 14–22. doi:10.1016/j.apsb.2017.11.009
Li, Y., Wang, Y. G., Huang, G., and Gao, J. M. (2018). Cooperativity principles in self-assembled nanomedicine. Chem. Rev. 118 (11), 5359–5391. doi:10.1021/acs.chemrev.8b00195
Liang, S., Deng, X., Ma, P., Cheng, Z., and Lin, J. (2020). Recent advances in nanomaterial-assisted combinational sonodynamic cancer therapy. Adv. Mat. 32 (47), e2003214. doi:10.1002/adma.202003214
Liu, J., Li, N., Zhu, Z., Kiang, K. M., Ng, A. C. K., Dong, C. M., et al. (2022). Vitamin D enhances hematoma clearance and neurologic recovery in intracerebral hemorrhage. Stroke 53 (6), 2058–2068. doi:10.1161/STROKEAHA.121.037769
Lopes, J., Lopes, D., Pereira-Silva, M., Peixoto, D., Veiga, F., Hamblin, M. R., et al. (2022). Macrophage cell membrane-cloaked nanoplatforms for biomedical applications. Small Methods 6 (8), e2200289. doi:10.1002/smtd.202200289
Lu, C., Tan, C., Ouyang, H., Chen, Z., Yan, Z., and Zhang, M. (2022). Ferroptosis in intracerebral hemorrhage: A panoramic perspective of the metabolism, mechanism and theranostics. Aging Dis. 13 (5), 1348–1364. doi:10.14336/AD.2022.01302
Luo, J. Q., Liu, R., Chen, F. M., Zhang, J. Y., Zheng, S. J., Shao, D., et al. (2023). Nanoparticle-Mediated CD47-sirpα blockade and calreticulin exposure for improved cancer chemo-immunotherapy. ACS Nano 17 (10), 8966–8979. doi:10.1021/acsnano.2c08240
Mandal, S., Natarajan, S., Mani, P., and Pankajakshan, A. (2021). Post-synthetic modification of metal-organic frameworks toward applications. Adv. Funct. Mat. 31 (4), 2006291. doi:10.1002/adfm.202006291
Marques, M. S., Cordeiro, M. F., Marinho, M. A. G., Vian, C. O., Vaz, G. R., Alves, B. S., et al. (2020). Curcumin-loaded nanoemulsion improves haemorrhagic stroke recovery in wistar rats. Brain Res. 1746, 147007. doi:10.1016/j.brainres.2020.147007
Mazurek, M. H., Cahn, B. A., Yuen, M. M., Prabhat, A. M., Chavva, I. R., Shah, J. T., et al. (2021). Portable, bedside, low-field magnetic resonance imaging for evaluation of intracerebral hemorrhage. Nat. Commun. 12 (1), 5119. doi:10.1038/s41467-021-25441-6
Mehryab, F., Rabbani, S., Shahhosseini, S., Shekari, F., Fatahi, Y., Baharvand, H., et al. (2020). Exosomes as a next-generation drug delivery system: an update on drug loading approaches, characterization, and clinical application challenges. Acta Biomater. 113, 42–62. doi:10.1016/j.actbio.2020.06.036
Mendelow, A. D., Gregson, B. A., Fernandes, H. M., Murray, G. D., Teasdale, G. M., Hope, D. T., et al. (2005). Early surgery versus initial conservative treatment in patients with spontaneous supratentorial intracerebral haematomas in the international surgical trial in intracerebral haemorrhage (STICH): a randomised trial. Lancet 365 (9457), 387–397. doi:10.1016/s0140-6736(05)70233-6
Mo, Y., Duan, L., Yang, Y., Liu, W., Zhang, Y., Zhou, L., et al. (2021). Nanoparticles improved resveratrol brain delivery and its therapeutic efficacy against intracerebral hemorrhage. Nanoscale 13 (6), 3827–3840. doi:10.1039/d0nr06249a
Morotti, A., Boulouis, G., Dowlatshahi, D., Li, Q., Shamy, M., Salman, R. A., et al. (2022). Intracerebral haemorrhage expansion: definitions, predictors, and prevention. Lancet Neurol. 22 (2), 159–171. doi:10.1016/S1474-4422(22)00338-6
Mracsko, E., and Veltkamp, R. (2014). Neuroinflammation after intracerebral hemorrhage. Front. Cell. Neurosci. 8, 388. doi:10.3389/fncel.2014.00388
Ohashi, S. N., DeLong, J. H., Kozberg, M. G., Mazur-Hart, D. J., van Veluw, S. J., Alkayed, N. J., et al. (2023). Role of inflammatory processes in hemorrhagic stroke. Stroke 54 (2), 605–619. doi:10.1161/STROKEAHA.122.037155
Pallardy, M. J., Turbica, I., and Biola-Vidamment, A. (2017). Why the immune system should Be concerned by nanomaterials? Front. Immunol. 8, 544. doi:10.3389/fimmu.2017.00544
Park, J., Kim, J. Y., Choi, S. K., Kim, J. Y., Kim, J. H., Jeon, W. B., et al. (2017). Thermo-sensitive assembly of the biomaterial REP reduces hematoma volume following collagenase-induced intracerebral hemorrhage in rats. Nanomedicine 13 (6), 1853–1862. doi:10.1016/j.nano.2017.04.001
Pedragosa, J., Miro-Mur, F., Otxoa-de-Amezaga, A., Justicia, C., Ruiz-Jaen, F., Ponsaerts, P., et al. (2020). CCR2 deficiency in monocytes impairs angiogenesis and functional recovery after ischemic stroke in mice. J. Cereb. Blood Flow. Metab. 40 (1), S98–S116. doi:10.1177/0271678X20909055
Peng, W., Zhang, M. L., Zhang, J., and Chen, G. (2022). Potential role of hydrogen sulfide in central nervous system tumors: a narrative review. Med. Gas. Res. 12 (1), 6–9. doi:10.4103/2045-9912.324590
Puy, L., Parry-Jones, A. R., Sandset, E. C., Dowlatshahi, D., Ziai, W., and Cordonnier, C. (2023). Intracerebral haemorrhage. Nat. Rev. Dis. Prim. 9 (1), 14. doi:10.1038/s41572-023-00424-7
Rabinstein, A. A. (2017). Intracerebral haemorrhage: no good treatment but treatment helps. Lancet 389 (10069), 575–576. doi:10.1016/S0140-6736(17)30002-8
Raghu, H., Lepus, C. M., Wang, Q., Wong, H. H., Lingampalli, N., Oliviero, F., et al. (2017). CCL2/CCR2, but not CCL5/CCR5, mediates monocyte recruitment, inflammation and cartilage destruction in osteoarthritis. Ann. Rheum. Dis. 76 (5), 914–922. doi:10.1136/annrheumdis-2016-210426
Ran, W., and Xue, X. (2018). Theranostical application of nanomedicine for treating central nervous system disorders. Sci. China-Life Sci. 61 (4), 392–399. doi:10.1007/s11427-017-9292-7
Samuni, A., Krishna, C. M., Riesz, P., Finkelstein, E., and Russo, A. (1988). A novel metal-free low molecular weight superoxide dismutase mimic. J. Biol. Chem. 263 (34), 17921–17924. doi:10.1016/s0021-9258(19)81304-2
Sang, L. Y., Liang, Y. X., Li, Y., Wong, W. M., Tay, D. K., So, K. F., et al. (2015). A self-assembling nanomaterial reduces acute brain injury and enhances functional recovery in a rat model of intracerebral hemorrhage. Nanomedicine 11 (3), 611–620. doi:10.1016/j.nano.2014.05.012
Schneider, A., Garlick, J. A., and Egles, C. (2008). Self-assembling peptide nanofiber scaffolds accelerate wound healing. PLoS One 3 (1), e1410. doi:10.1371/journal.pone.0001410
Shang, Y., Lu, H., Liao, L., Li, S., Xiong, H., and Yao, J. (2023). Bioengineered nanospores selectively blocking LC3-associated phagocytosis in tumor-associated macrophages potentiate antitumor immunity. ACS Nano 17 (11), 10872–10887. doi:10.1021/acsnano.3c02657
Shen, H., Yao, X., Li, H., Li, X., Zhang, T., Sun, Q., et al. (2018). Role of exosomes derived from miR-133b modified MSCs in an experimental rat model of intracerebral hemorrhage. J. Mol. Neurosci. 64 (3), 421–430. doi:10.1007/s12031-018-1041-2
Sheth, K. N. (2022). Spontaneous intracerebral hemorrhage. N. Engl. J. Med. 387 (17), 1589–1596. doi:10.1056/NEJMra2201449
Shi, S. X., Xiu, Y., Li, Y., Yuan, M., Shi, K., Liu, Q., et al. (2023). CD4(+) T cells aggravate hemorrhagic brain injury. Sci. Adv. 9 (23), eabq0712. doi:10.1126/sciadv.abq0712
Spallholz, J. E. (1994). On the nature of selenium toxicity and carcinostatic activity. Free Radic. Biol. Med. 17 (1), 45–64. doi:10.1016/0891-5849(94)90007-8
Srikanth, M., and Kessler, J. A. (2012). Nanotechnology-novel therapeutics for CNS disorders. Nat. Rev. Neurol. 8 (6), 307–318. doi:10.1038/nrneurol.2012.76
Stuart, M. A. C., Huck, W. T. S., Genzer, J., Muller, M., Ober, C., Stamm, M., et al. (2010). Emerging applications of stimuli-responsive polymer materials. Nat. Mat. 9 (2), 101–113. doi:10.1038/Nmat2614
Szwargulski, P., Wilmes, M., Javidi, E., Thieben, F., Graeser, M., Koch, M., et al. (2020). Monitoring intracranial cerebral hemorrhage using multicontrast real-time magnetic particle imaging. ACS Nano 14 (10), 13913–13923. doi:10.1021/acsnano.0c06326
Tan, X., Hu, L., Shu, Z., Chen, L., Li, X., Du, M., et al. (2019). Role of CCR2 in the development of streptozotocin-treated diabetic cardiomyopathy. Diabetes 68 (11), 2063–2073. doi:10.2337/db18-1231
Tian, Q., Liu, S., Han, S. M., Zhang, W., Qin, X. Y., Chen, J. H., et al. (2023). The mechanism and relevant mediators associated with neuronal apoptosis and potential therapeutic targets in subarachnoid hemorrhage. Neural Regen. Res. 18 (2), 244–252. doi:10.4103/1673-5374.346542
Tu, W. J., Hua, Y., Yan, F., Bian, H., Yang, Y., Lou, M., et al. (2022). Prevalence of stroke in China, 2013–2019: A population-based study. Lancet Reg. Health-W. pac. 28, 100550. doi:10.1016/j.lanwpc.2022.100550
Urday, S., Kimberly, W. T., Beslow, L. A., Vortmeyer, A. O., Selim, M. H., Rosand, J., et al. (2015). Targeting secondary injury in intracerebral haemorrhage-perihaematomal oedema. Nat. Rev. Neurol. 11 (2), 111–122. doi:10.1038/nrneurol.2014.264
Wan, J., Ren, H., and Wang, J. (2019). Iron toxicity, lipid peroxidation and ferroptosis after intracerebral haemorrhage. Stroke Vasc. Neurol. 4 (2), 93–95. doi:10.1136/svn-2018-000205
Wan, Y., Holste, K. G., Hua, Y., Keep, R. F., and Xi, G. (2023). Brain edema formation and therapy after intracerebral hemorrhage. Neurobiol. Dis. 176, 105948. doi:10.1016/j.nbd.2022.105948
Wang, M., Xia, F., Wan, S., Hua, Y., Keep, R. F., and Xi, G. (2021). Role of complement component 3 in early erythrolysis in the hematoma after experimental intracerebral hemorrhage. Stroke 52 (8), 2649–2660. doi:10.1161/STROKEAHA.121.034372
Wang, W. J., Ding, J. S., Sun, Q., Xu, X., and Chen, G. (2022). Role of hyperbaric oxygen in glioma: a narrative review. Med. Gas. Res. 12 (1), 1–5. doi:10.4103/2045-9912.324589
Wang, W., Lu, K. J., Yu, C. H., Huang, Q. L., and Du, Y. Z. (2019a). Nano-drug delivery systems in wound treatment and skin regeneration. J. Nanobiotechnol. 17 (1), 82. doi:10.1186/s12951-019-0514-y
Wang, Y., Wang, Z., Xu, C., Tian, H., and Chen, X. (2019b). A disassembling strategy overcomes the EPR effect and renal clearance dilemma of the multifunctional theranostic nanoparticles for cancer therapy. Biomaterials 197, 284–293. doi:10.1016/j.biomaterials.2019.01.025
Wei, J., Mu, J., Tang, Y., Qin, D., Duan, J., and Wu, A. (2023). Next-generation nanomaterials: advancing ocular anti-inflammatory drug therapy. J. Nanobiotechnol. 21 (1), 282. doi:10.1186/s12951-023-01974-4
White, C. M. (2002). A review of the pharmacologic and pharmacokinetic aspects of rosuvastatin. J. Clin. Pharmacol. 42 (9), 963–970. doi:10.1177/009127002401102876
Wilkinson, D. A., Keep, R. F., Hua, Y., and Xi, G. (2018a). Hematoma clearance as a therapeutic target in intracerebral hemorrhage: from macro to micro. J. Cereb. Blood Flow. Metab. 38 (4), 741–745. doi:10.1177/0271678X17753590
Wilkinson, D. A., Pandey, A. S., Thompson, B. G., Keep, R. F., Hua, Y., and Xi, G. (2018b). Injury mechanisms in acute intracerebral hemorrhage. Neuropharmacology 134, 240–248. doi:10.1016/j.neuropharm.2017.09.033
Xia, F., Keep, R. F., Ye, F., Holste, K. G., Wan, S., Xi, G., et al. (2022). The fate of erythrocytes after cerebral hemorrhage. Transl. Stroke Res. 13 (5), 655–664. doi:10.1007/s12975-021-00980-8
Xia, Y., Rao, L., Yao, H., Wang, Z., Ning, P., and Chen, X. (2020). Engineering macrophages for cancer immunotherapy and drug delivery. Adv. Mat. 32 (40), e2002054. doi:10.1002/adma.202002054
Xie, Y., Hou, W., Song, X., Yu, Y., Huang, J., Sun, X., et al. (2016). Ferroptosis: process and function. Cell Death Differ. 23 (3), 369–379. doi:10.1038/cdd.2015.158
Xu, C., Pan, Y., Zhang, H., Sun, Y., Cao, Y., Qi, P., et al. (2023). Platelet-membrane-coated polydopamine nanoparticles for neuroprotection by reducing oxidative stress and repairing damaged vessels in intracerebral hemorrhage. Adv. Healthc. Mat., e2300797. doi:10.1002/adhm.202300797
Xu, C., Wang, Y., Yu, H., Tian, H., and Chen, X. (2018). Multifunctional theranostic nanoparticles derived from fruit-extracted anthocyanins with dynamic disassembly and elimination abilities. ACS Nano 12 (8), 8255–8265. doi:10.1021/acsnano.8b03525
Xu, J., Chen, Z., Yu, F., Liu, H., Ma, C., Xie, D., et al. (2020a). IL-4/STAT6 signaling facilitates innate hematoma resolution and neurological recovery after hemorrhagic stroke in mice. Proc. Natl. Acad. Sci. U. S. A. 117 (51), 32679–32690. doi:10.1073/pnas.2018497117
Xu, J., Sun, T., Zhong, R., You, C., and Tian, M. (2020b). PEGylation of deferoxamine for improving the stability, cytotoxicity, and iron-overload in an experimental stroke model in rats. Front. Bioeng. Biotechnol. 8, 592294. doi:10.3389/fbioe.2020.592294
Xu, L., Wang, Y. Y., Huang, J., Chen, C. Y., Wang, Z. X., and Xie, H. (2020c). Silver nanoparticles: synthesis, medical applications and biosafety. Theranostics 10 (20), 8996–9031. doi:10.7150/thno.45413
Xu, Y., Chen, A., Wu, J., Wan, Y., You, M., Gu, X., et al. (2022). Nanomedicine: an emerging novel therapeutic strategy for hemorrhagic stroke. Int. J. Nanomed. 17, 1927–1950. doi:10.2147/IJN.S357598
Xue, M., and Yong, V. W. (2020). Neuroinflammation in intracerebral haemorrhage: immunotherapies with potential for translation. Lancet Neurol. 19 (12), 1023–1032. doi:10.1016/S1474-4422(20)30364-1
Yang, C., Han, M., Li, R., Zhou, L., Zhang, Y., Duan, L., et al. (2021a). Curcumin nanoparticles inhibiting ferroptosis for the enhanced treatment of intracerebral hemorrhage. Int. J. Nanomed. 16, 8049–8065. doi:10.2147/IJN.S334965
Yang, L., Wang, H., Yang, X., Wu, Q., An, P., Jin, X., et al. (2020). Auranofin mitigates systemic iron overload and induces ferroptosis via distinct mechanisms. Signal Transduct. Target. Ther. 5 (1), 138. doi:10.1038/s41392-020-00253-0
Yang, P., Zhu, F., Zhang, Z., Cheng, Y., Wang, Z., and Li, Y. (2021b). Stimuli-responsive polydopamine-based smart materials. Chem. Soc. Rev. 50 (14), 8319–8343. doi:10.1039/d1cs00374g
Yang, Y., Deng, G., Wang, P., Lv, G., Mao, R., Sun, Y., et al. (2021c). A selenium nanocomposite protects the mouse brain from oxidative injury following intracerebral hemorrhage. Int. J. Nanomed. 16, 775–788. doi:10.2147/IJN.S293681
Yang, Z., Duan, H., Mo, L., Qiao, H., and Li, X. (2010). The effect of the dosage of NT-3/chitosan carriers on the proliferation and differentiation of neural stem cells. Biomaterials 31 (18), 4846–4854. doi:10.1016/j.biomaterials.2010.02.015
Yu, C. C., Liu, L. B., Chen, S. Y., Wang, X. F., Wang, L., and Du, Y. J. (2022). Ancient Chinese herbal recipe huanglian jie du decoction for ischemic stroke: an overview of current evidence. Aging Dis. 13 (6), 1733–1744. doi:10.14336/AD.2022.0311
Yu, H., Zhang, S., Yang, H., Miao, J., Ma, X., Xiong, W., et al. (2023a). Specific interaction based drug loading strategies. Nanoscale Horiz. doi:10.1039/d3nh00165b
Yu, W., Liu, B., Zhou, L., Gong, E., Che, C., Zhou, J., et al. (2023b). The development of drug delivery systems for efficient intracranial hemorrhage therapy. Adv. Healthc. Mat. 12, e2203141. doi:10.1002/adhm.202203141
Yuan, J., Li, L., Yang, Q., Ran, H., Wang, J., Hu, K., et al. (2021). Targeted treatment of ischemic stroke by bioactive nanoparticle-derived reactive oxygen species responsive and inflammation-resolving nanotherapies. ACS Nano 15 (10), 16076–16094. doi:10.1021/acsnano.1c04753
Zeng, F., Wu, Y., Li, X., Ge, X., Guo, Q., Lou, X., et al. (2018). Custom-made ceria nanoparticles show a neuroprotective effect by modulating phenotypic polarization of the microglia. Angew. Chem.-Int. Ed. 57 (20), 5808–5812. doi:10.1002/anie.201802309
Zhai, X., Zhang, C., Zhao, G., Stoll, S., Ren, F., and Leng, X. (2017). Antioxidant capacities of the selenium nanoparticles stabilized by chitosan. J. Nanobiotechnol. 15 (1), 4. doi:10.1186/s12951-016-0243-4
Zhang, N., Luo, Y., He, L., Zhou, L., and Wu, W. (2016). A self-assembly peptide nanofibrous scaffold reduces inflammatory response and promotes functional recovery in a mouse model of intracerebral hemorrhage. Nanomedicine 12 (5), 1205–1217. doi:10.1016/j.nano.2015.12.387
Zhang, X., Khan, S., Wei, R., Zhang, Y., Liu, Y., Wee Yong, V., et al. (2023). Application of nanomaterials in the treatment of intracerebral hemorrhage. J. Tissue Eng. 14, 204173142311570. doi:10.1177/20417314231157004
Zhang, X., Li, Z., Yang, P., Duan, G., Liu, X., Gu, Z., et al. (2021a). Polyphenol scaffolds in tissue engineering. Mat. Horizons 8 (1), 145–167. doi:10.1039/d0mh01317j
Zhang, X., Si, Z., Wang, Y., Li, Y., Xu, C., and Tian, H. (2021b). Polymerization and coordination synergistically constructed photothermal agents for macrophages-mediated tumor targeting diagnosis and therapy. Biomaterials 264, 120382. doi:10.1016/j.biomaterials.2020.120382
Zhang, Z., Dalan, R., Hu, Z., Wang, J. W., Chew, N. W., Poh, K. K., et al. (2022). Reactive oxygen species scavenging nanomedicine for the treatment of ischemic heart disease. Adv. Mat. 34 (35), e2202169. doi:10.1002/adma.202202169
Zhao, T., Guo, X., and Sun, Y. (2021). Iron accumulation and lipid peroxidation in the aging retina: implication of ferroptosis in age-related macular degeneration. Aging Dis. 12 (2), 529–551. doi:10.14336/AD.2020.0912
Zheng, J., Lu, J., Mei, S., Wu, H., Sun, Z., Fang, Y., et al. (2021). Ceria nanoparticles ameliorate white matter injury after intracerebral hemorrhage: microglia-astrocyte involvement in remyelination. J. Neuroinflamm. 18 (1), 43. doi:10.1186/s12974-021-02101-6
Zhu, F., Zhang, J., Zhong, J., Wang, T., Li, Y., and Gu, Z. (2023a). Natural polyphenol-based nanoparticles for the treatment of Iron-overload disease. J. Control. Release. 356, 84–92. doi:10.1016/j.jconrel.2023.02.027
Zhu, F., Zhong, J., Hu, J., Yang, P., Zhang, J., Zhang, M., et al. (2023b). Carrier-free deferoxamine nanoparticles against iron overload in brain. CCS Chem. 5 (1), 257–270. doi:10.31635/ccschem.022.202101696
Zhu, F., Zi, L., Yang, P., Wei, Y., Zhong, R., Wang, Y., et al. (2021). Efficient iron and ROS nanoscavengers for brain protection after intracerebral hemorrhage. ACS Appl. Mat. Interfaces 13 (8), 9729–9738. doi:10.1021/acsami.1c00491
Zhuang, J., Ying, M., Spiekermann, K., Holay, M., Zhang, Y., Chen, F., et al. (2018). Biomimetic nanoemulsions for oxygen delivery in vivo. Adv. Mat. 30 (49), e1804693. doi:10.1002/adma.201804693
Keywords: intracerebral hemorrhage, therapeutic agents, nanotechnologies, safety, bioavailability, functionality
Citation: Wang J, Wang T, Fang M, Wang Z, Xu W, Teng B, Yuan Q and Hu X (2023) Advances of nanotechnology for intracerebral hemorrhage therapy. Front. Bioeng. Biotechnol. 11:1265153. doi: 10.3389/fbioe.2023.1265153
Received: 24 July 2023; Accepted: 01 September 2023;
Published: 12 September 2023.
Edited by:
Ming-Wei Chang, Ulster University, United KingdomReviewed by:
Qin Hu, Shanghai Jiao Tong University, ChinaCheng Hu, Sichuan University, China
Joanna Ward, Ulster University, United Kingdom
Copyright © 2023 Wang, Wang, Fang, Wang, Xu, Teng, Yuan and Hu. This is an open-access article distributed under the terms of the Creative Commons Attribution License (CC BY). The use, distribution or reproduction in other forums is permitted, provided the original author(s) and the copyright owner(s) are credited and that the original publication in this journal is cited, in accordance with accepted academic practice. No use, distribution or reproduction is permitted which does not comply with these terms.
*Correspondence: Xin Hu, aHV4aW5neHh5QGdtYWlsLmNvbQ==; Qijuan Yuan, eXVhbl9kb3Vkb3VAMTYzLmNvbQ==
†These authors have contributed equally to this work and share first authorship