- 1Health Science Center, Ningbo University, Ningbo, Zhejiang, China
- 2Department of Orthopaedics, The First Affiliated Hospital of Ningbo University, Ningbo, Zhejiang, China
The intervertebral disc (IVD) is a load-bearing, avascular tissue that cushions pressure and increases flexibility in the spine. Under the influence of obesity, injury, and reduced nutrient supply, it develops pathological changes such as fibular annulus (AF) injury, disc herniation, and inflammation, eventually leading to intervertebral disc degeneration (IDD). Lower back pain (LBP) caused by IDD is a severe chronic disorder that severely affects patients’ quality of life and has a substantial socioeconomic impact. Patients may consider surgical treatment after conservative treatment has failed. However, the broken AF cannot be repaired after surgery, and the incidence of re-protrusion and reoccurring pain is high, possibly leading to a degeneration of the adjacent vertebrae. Therefore, effective treatment strategies must be explored to repair and prevent IDD. This paper systematically reviews recent advances in repairing IVD, describes its advantages and shortcomings, and explores the future direction of repair technology.
1 Introduction
IDD is frequently asymptomatic (Matsumoto et al., 2010; Brinjikji et al., 2015), but it remains one of the leading causes of LBP (Morlion, 2013; Hartvigsen et al., 2018), a severe chronic disorder that is a significant cause of disability (Hicks et al., 2009; March et al., 2014). It affects approximately 1 billion people worldwide (Hoy et al., 2014). It severely reduces patients’ quality of life while imposing a substantial socioeconomic burden, costing $253 billion per year in the United States alone (Hoy et al., 2014). Current measures to treat LBP due to IDD include conservative and surgical treatment. It is commonly recommended to undergo conservative treatment before surgical treatment, and the choice of treatment depends on the effectiveness of conservative treatment and the degree of IDD (Sabnis and Diwan, 2014; Wu et al., 2020). Conservative treatment includes oral pain medication or topical pain relief, physical therapy and physical exercise to strengthen the low back muscles (Wu et al., 2020). Pain will be relieved in some patients, but recurrence is common and cannot be avoided (Hestbaek et al., 2003). Surgical intervention may be considered when conservative treatment fails to improve symptoms (Storm et al., 2002). There are three main types of surgical treatment: percutaneous decompression, fusion, and disc replacement. Percutaneous decompression includes thermal (radiofrequency ablation), chemical (protease) (Kim et al., 2018) and endoscopic decompression (Aichmair et al., 2014). It relieves the protruding IVD from compressing the nerve root by eliminating the protruding tissue. However, due to the low number of AF cells, avascularity (Hampton et al., 1989; Vernon-Roberts et al., 2007) and poor regenerative capacity (Bailey et al., 2013), there is a high incidence of reherniation and recurrent pain after surgery (Andersson, 1999), which may even lead to degeneration of the adjacent vertebrae (Häkkinen et al., 2007; Hughes et al., 2012). Fusion surgery limits the mobility of the fused segment (Helgeson et al., 2013), while the prosthesis is prone to displacement after total disc replacement (van den Eerenbeemt et al., 2010; Costi et al., 2011). Surgical treatment focuses on relieving pressure to relieve pain and does little to regenerate tissue and delay IDD.
Current strategies for treating IDD include AF sutures and tissue-engineered biological repair. AF sutures maintain IVD height and lumbar stability but do not provide high mechanical loading for long periods. Moreover, suture manipulation is difficult with larger defects (Heuer et al., 2008; Rickers et al., 2018; Li et al., 2020; Ren et al., 2020; Chen et al., 2023). In the last decades, tissue-engineered biological repairs, such as hydrogels and scaffolds, has shown great advantages. However, it still has some limitations at present: 1) Tissue-engineered repair may have a series of safety issues such as implant-induced infections, sterility and tumorigenicity (Agnol et al., 2019); 2) Since the benefit-risk ratio of tissue-engineered repair for human treatment is unknown at present, this will bring a series of ethical issues; 3) The process of tissue-engineered repair is generally long, and it needs to be considered whether it can be repaired efficiently and prevented from recurrence; and 4) At the present stage, the majority of tissue-engineered repair materials mostly remain in the experimental stage, and the treatment of IDD remains a challenge. Hence, this article systematically reviews the recent advances in IVD repair and explores the future direction of IVD repair technology.
2 The structure and function of IVD
The IVD is a disc-like fibrocartilage between the vertebral bodies of two adjacent vertebrae consisting of the AF and the nucleus pulposus (NP) (Klein et al., 1983). The peripheral AF wraps around the central NP and anchors within the superior and inferior EPs. The IVD is composed mainly of type I and type II collagen fibres, with a progressive decrease in the distribution of type I collagen from the outside to the inside and the opposite trend for type II collagen. The collagen fibres of the AF make up approximately 70% of its dry weight and the NP approximately 20% (Eyre and Muir, 1976; Eyre and Muir, 1977). The AF is a laminar complex of 15–25 concentric circles (Humzah and Soames, 1988; Marchand and Ahmed, 1990). The angle of the AF fibres varies and cross-aligns between 62° in the outer layer and 20° in the inner layer (Cassidy et al., 1989). The cellular distribution of AF varies, with the cells in the outer annulus showing a tendency to be spindle-shaped fibroblast-like and the inner annulus tending to be oval or spherical (Errington et al., 1998; Bruehlmann et al., 2002). The NP is a heterogeneous structure consisting of glycosaminoglycan (GAG), type II collagen fibres and a small number of chondrocytes (Humzah and Soames, 1988; Mizuno et al., 2004). NP cells are derived from notochordal cells (Choi et al., 2008). The high water content of NP tissue allows it to absorb spinal loads and transmit them to the surrounding tissues (Chan et al., 2011). Endplate (EP) is a layer of hyaline cartilage approximately 0.6 mm thick (Raj, 2008). The cell density in EP is higher than in AF and NP (Maroudas et al., 1975). EP forms a porous, semi-permeable barrier between the vertebrae, regulating the transport of nutrients, mainly small solutes (Chan et al., 2011; Pattappa et al., 2012). IVD loading mechanisms are complex and allow the spine to produce movements such as extension, flexion, bending and rotation (Roberts et al., 2006). IVD features nonlinear, viscoelastic and anisotropic mechanics (Elliott and Setton, 2001), contributing to the spine’s ability to increase flexibility and cushion pressure (O'Connell et al., 2009).
3 Pathophysiology of IDD
The pathophysiology of IDD is complex, and the main alterations include loss of proteoglycans, reduction of collagen fibrils and increase in fibronectin (Oegema et al., 2000). The most significant of these alterations is the loss of proteoglycans. The major influential factors are smoking, obesity (Kim et al., 2020), injury (Osti et al., 1990), abnormal mechanical loading (Lotz et al., 1998), genetic susceptibility (Heikkilä et al., 1989), and reduction in nutrient supply to IVD cells (Nachemson et al., 1970). As age increases, the supply of nutrients to IVD cells decreases, decreasing the oxygen content, pH and extracellular matrix (ECM) synthesis of the IVD (Ishihara and Urban, 1999). However, the reduced ECM synthesis would exacerbate the decline in nutrient supply to the IVD (Kim et al., 2020). Ultimately, it causes various pathological changes such as height loss of IVD, vertebral osteophyte formation, EP sclerosis, AF injury, disc herniation, inflammation and motion disorders (Lotz and Ulrich, 2006; Guterl et al., 2013; Huang et al., 2013).
4 Hydrogel repair strategy
Hydrogel is a class of materials with a polymer network system capable of absorbing vast amounts of fluid. It is capable of mimicking the biological, chemical and mechanical properties of native tissues. Notably, the mechanical and biological properties and degradation kinetics of hydrogels can be customised to meet different demands (Gauvin et al., 2012; Lewns et al., 2023).
4.1 Synthetic hydrogels
Synthetic hydrogels include polyacrylic acid and its derivatives, polyethene oxide, derived copolymers, polyvinyl alcohol (PVA), poly-vinyl pyrrolidone (PVP), polyethylene glycol (PEG) and polyphosphazene nitrile (Yan et al., 2021). Table 1 outlines recent novel strategies for synthetic hydrogels in IVD repair. The biodegradable electrospun polycaprolactone (PCL) membranes show no mass loss or dimensional change after 6 months of exposure to aqueous solutions (Alexeev et al., 2021). Agnol et al. made a polyurethane (PU) tissue adhesive-U2000-2 prepolymer by combining polycarbonate diol and hexamethylene diisocyanate (HDI) monomers. It features excellent dynamic compression properties and adhesion, especially for covalent binding to gelatine without the use of catalysts. However, sterility, biomechanics of in vivo and biocompatibility are yet to be investigated (Agnol et al., 2019). HDI in copolymers of PEG with trimethylene carbonate 3 (TMC3) and HDI end-groups is capable of forming covalent bonds with natural AF tissue. At the same time, TMC3 has properties such as high adhesion strength, slow degradation, and excellent cytocompatibility, but extrusion occurs under high stress and cyclic loading, requiring further optimization of TMC (Long et al., 2018). Schmitz et al. reported that PEG combined decellularisation of notochordal cell derived matrix (DNCM) showed excellent biocompatibility, but further biomechanical adjustments are required (Schmitz et al., 2023). Regarding the progress of research on PVA hydrogel as an NP replacement material, Leone et al. found that PVA with PVP molar ratio of 1:1 has excellent biomechanical characteristics which is a promising NP replacement material (Leone et al., 2019). Permana et al. reported that the biomechanical properties of PVA combined with Silicone Rubber close to human NP (Permana et al., 2022).
4.2 Natural hydrogels
Natural hydrogels commonly originate from animal and plant extracts, many of which are essential components of human tissues and organs. Its non-toxic and excellent biosafety, biocompatibility and injectability make it widely used for tissue regeneration and repair. The main components include gelatine, collagen, fibrin, hyaluronate, alginate and chitosan. Table 2 overviews recent strategies for natural hydrogels in IVD repair.
A novel temperature-sensitive chitosan hydrogel exhibits mechanical properties similar to those of human IVD with the ability to maintain NP cell activity and promote GAG production (Alinejad et al., 2019). Nanostructured gelatin colloidal hydrogels are characterised by shear thinning, self-healing behaviour, homogeneous porous networks and tunable mechanical properties. Notably, it can also prevent leakage of bone marrow stem cells (BMSCs), maintain cell viability, and support the NP-like differentiation of BMSCs (Wang et al., 2021b). The injectable high-density collagen (HDC) gel reduced degeneration of the NP by alleviating AF breakdown and forming a fibrous cap structure that prevented protrusion of the NP contents and maintained the water content of the NP. However, Moriguchi et al. concluded that the long-term effects of the gel are unknown and that it would need to further research analyses and a longer follow-up period (Moriguchi et al., 2018). Jiang et al. used chondroitinase ABC (ChABC) to improve collagen gel adhesion in a short-term manner by removing proteoglycan locally from AF, which reduced the risk of material migration. Moreover, short-term use has not significantly reduced cell viability (Jiang et al., 2019). Porcine fibrin gel was effective in enhancing the closure of AF sutures, helping maintain the NP’s water content and delaying IDD. However, this study needed more evidence at the molecular level (Du and Zhu, 2019).
Alginate is a naturally occurring polymeric polysaccharide found in algal and bacterial cell walls, with high porosity and adjustable viscosity. Decellularised ultrapure alginate (UPAL) gel promotes ECM production and proliferation of endogenous NP progenitor cells. Notably, it also inhibits TNF-α and IL-6 production, downregulates TrkA expression, inhibits vascular endothelial cell degeneration, and reduces acute postoperative pain (Ura et al., 2021). However, the UPAL gel lacks safety tests for immunogenicity, and there is a risk of re-protrusion on the other side of the IVD after injection. It is not well suited to the elderly population owing to its decellularisation (Tsujimoto et al., 2018). Future studies need to refine immunogenicity testing to assess the body’s rejection of it and incorporate stem cell repair to adapt it to the elderly population.
4.3 Composite hydrogel
Composite hydrogels are composed of both synthetic and natural polymer materials. Synthetic or natural hydrogels are not able to satisfy both excellent biomechanics and biocompatibility, so in recent years more and more research has turned to composite hydrogels. Table 3 outlines recent strategies for composite hydrogels in IVD repair.
4.3.1 Restoration and improvement of IVD biomechanics
Genipin is an excellent natural biological cross-linking agent. Genipin cross-linked fibrin (FibGen) hydrogel forms a dense seal at the defect site (Alexeev et al., 2020). It has better axial compression properties than the semi-synthetic binder consisting of glutaraldehyde and albumin (BioGlue) (Scheibler et al., 2018), which improves the tensile stiffness and axial neutral zone length of IVDs. However, it fails to restore the axial and torsional parameters to normal levels (Fujii et al., 2020). Cruz found that FibGen with a ratio of 140 mg/mL fibrinogen and 6 mg/mL genipin (F140G6) provided excellent initial and long-term mechanical properties without cell proliferation and deposition of ECM, making it suitable as a decellularised adhesive and repair biomaterial (Cruz et al., 2018). Notably, FibGen combined with the NP substitute carboxymethylcellulose - methylcellulose (CMC-MC) (Hom et al., 2019), FibGen combined with silk scaffolds (Frauchiger et al., 2018), and injected hyaluronic acid (HA) combined with photo cross-linked collagen patches for repair strategies enhance the mechanical properties of IVD and prevent IDD (Sloan et al., 2020). PCL-supported electrically densified oriented Collagen type I patches have high tensile strength and modulus in the wet state or at sharp increases in stress values (Dewle et al., 2021).
Polysaccharides have various application values such as immune regulation, antiviral, anticancer, and hypoglycemic effects. GAGs doubly modified by oxidation and methacrylation can covalently bind interpenetrating network (IPN) hydrogels composed of fibronectin and polyethene glycol diacrylate (PEGDA) to natural AF tissue, which produces a higher lap shear bond strength than riboflavin cross-linked and genipin cross-linked fibronectin hydrogels. In particular, the degree of oxidation correlates more closely with the adhesion strength than methacrylation. Notably, it is not cytotoxic (DiStefano et al., 2020). The mechanical properties of cellulose nanofibre-reinforced chitosan hydrogels were enhanced due to the addition of cellulose nanofibres. In particular, the range of activity of the IVD after implantation is close to natural IVD. Nevertheless, because of its non-cellular repair, it fails to ensure that the tissue regenerates in the long term (Doench et al., 2019). Gellan gels are polymeric linear polysaccharides widely used in various applications such as food and pharmaceuticals. Pereira et al. reinforced methacrylated gellan-gum (GGMA) with cellulose nanocrystals (nCell), which enhance the ability of AF to withstand loading and create gradient structures that mimic the internal and external structure of natural AF (Pereira et al., 2018). A photocurable hydrogel consisting of PEGDA and decellularised annular fibrous matrix (DAFM) improves the lack of the DAFM hydrogel’s mechanical strength and maintains the porous structure (Wei et al., 2023).
4.3.2 Restore the functionality of NP and AF
The repair strategy of FibGen and injected HA combined with photo cross-linked collagen patches restores hydration and pressurization of IVD, and the latter delivers cells to the NP (Fujii et al., 2020; Sloan et al., 2020). FinGen with a ratio of 70 mg/mL fibrinogen and 1 mg/mL genipin (F70G1) produces ECM at the early stage (Cruz et al., 2018). Low concentrations of FibGen are effective in both delivering biofactors to the injured IVD to enhance endogenous repair and delivering live cells capable of synthesizing ECM for exogenous repair (Panebianco et al., 2020). The repair strategies of photocurable hydrogel consisting of PEGDA and DAFM and PCL-supported electrically dense directed collagen type I patches promote the deposition of ECM and prevent NP atrophy. The latter repair strategy also enables the adequate proliferation of AF cells while providing sufficient ligands for cell attachment (Dewle et al., 2021; Wei et al., 2023).
The repair strategy of PCL-supported electrically dense directed collagen type I patches enables the adequate proliferation of AF cells while providing sufficient ligands for cell attachment (Dewle et al., 2021). Genipin cross-linked decellularised fibrillar ring hydrogel (g-DAF-G) enables the directed differentiation of BMSCs towards AF cells and possesses excellent bioactivity and cell stretching properties. DAF retains the natural microstructure, which reduces the risk of implant rejection (Peng et al., 2020).
5 Tissue engineering scaffold repair strategy
Tissue engineering repair is a new therapeutic strategy for repairing IDD using seed cells as the core, scaffolds as the support vehicle and bioactive factors as a facilitating adjunct. Table 4 outlines recent strategies for tissue-engineered scaffolds in IVD repair.
5.1 Mechanical support and imitation of natural AF structures
The mechanical support of the scaffold is important for restoring the biomechanics of the IVD. The fibre angle, diameter and spacing influence the mechanical support of the scaffold (Page and Puttlitz, 2019). A new hybrid scaffold of 50% PCL and 50% poly (L-lactic acid) (PLLA) provides adequate and long-term mechanical support and tensile properties. It is cost-effective compared to 20% PCL and 80% PLLA (Shamsah et al., 2019; Shamsah et al., 2020). PCL scaffolds with an angular layer structure show excellent elastic response and radial tensile modulus, with axial compressibility exceeding natural AF tissue (Christiani et al., 2019).
Mimicking the structure of AF enables scaffolds to exhibit mechanical properties similar to natural AF, restoring NP volume and promoting cell colonisation, cell proliferation and ECM generation (Kang et al., 2018; Gluais et al., 2019). The admixture of GAG in fibrous scaffolds featuring interlaminar structures further enhances the ability of the scaffold to withstand simulated impact loading (Borem et al., 2019). It is insufficient to mimic natural AF tissue by simply regulating fibre diameter and orientation. To better simulate the microenvironment of AF, multifactorial modulation is essential (Zhou et al., 2021). Notably, the biocomposite laminate prepared by Sharabi et al. using alginate hydrogel-embedded long collagen fibres mimics the entire stress-strain mechanical behaviour of the AF lamina in both the longitudinal and circumferential directions, which has great potential for application in IVD repair (Sharabi et al., 2019).
5.2 The effect of scaffold aperture size and construction on the repair of IVDs
The porosity and structure of the scaffold are critical for cell migration and diffusion deep into the scaffold, transport of nutrients and metabolites, deposition of ECM and information transfer between cells (Zhu et al., 2021). Although the mechanical support of the nanoyarn scaffold (AYS) is strong, its pore size is small, and the rate of cell diffusion to depth is slow (Wang et al., 2020). The electrospun AYS and nanoyarn/three-dimensional porous nanofibrous hybrid scaffolds (HS) modify the small porosity of AYS, leading to faster cell infiltration efficiency (Ma et al., 2018). The type I collagen-PU scaffold contributes to maintaining the phenotype of human AF cells. However, its random porous structure fails to promote ECM deposition and the formation of a corneal lamellar microstructure similar to natural AF. It would require pretreatment with TGF-β1 to promote cell proliferation and matrix production (Du et al., 2020).
6 Several potential repair strategies
Table 5 outlines recent strategies with the potential for IVD restoration.
The strategies of timely and controlled hypotonic traction and photobiomodulation (PBM) improve the microenvironment of IDD by reducing catabolic genes such as MMP-1 and MMP-3 to inhibit ECM degradation, which offers the possibility of non-surgical interventions for patients (Che et al., 2019; Guo et al., 2020; Hwang et al., 2020; Che et al., 2021; Che et al., 2022).
Low hydrostatic pressure facilitates the maintenance of cell survival and ECM homeostasis by upregulating N-cadherin, prominently expressed in NP, and integrin β1, prominently expressed in AF. High hydrostatic pressure triggers apoptosis via the Hippo-YAP/TAZ pathway. Therefore, the repair strategy to keep IVD hydrostatic pressure in a physiologically low load state shows potential (Wang et al., 2021a).
Short-term local injection of bleomycin induces AF cells and BMSCs to promote fibrosis and maintain IVD height through the TGFβ-TGFβR1-Smad2/3 pathway without causing significant changes in cell cycle and apoptosis rates, which preserves the possibility of future clinical application (Yang et al., 2021).
ADAM8 may regulate inflammation and collagen fibril assembly, so partial inhibition of ADAM8 may serve as an intervention to delay IDD (Zhang et al., 2021).
Link N is a glycoprotein consisting of 16 amino acids that stabilize proteoglycan aggregates by combining with HA and integrins. Low doses of short Link N, consisting of one to eight amino acids, inhibit cell death and promote ECM synthesis (Mwale et al., 2018).
The injection of autologous platelet-rich plasma (PRP) within the IVD facilitates early recovery after injury. However, additional studies and more extended follow-up periods are required (Gelalis et al., 2019).
Cell leakage dramatically affects the effectiveness of the repair. Some repair strategies have been shown to reduce cell leakage (Hom et al., 2019; Wang et al., 2021b). Further research on reducing cell leakage is required in the future.
7 Currently marketed available IVD repair devices
Suturing of the IVD is a common repair method in clinical practice today. Advantages of AF suturing include 1) maintaining the pressurising effect of the NP and reducing the risk of recurrence of herniation; 2) decreasing mechanical irritation to the nerve root and alleviating postoperative symptoms of low back and leg pain; 3) diminishing the release of inflammatory mediators from the IVD and reducing the incidence of chemical radiculitis; 4) facilitating the healing of the scar in the AF (Bateman et al., 2016; Li et al., 2020). Ahlgren et al. found that sutured sheep IVDs exhibited a greater tendency to heal than non-sutured IVDs (Ahlgren et al., 2000).
In recent years, AF suture repair of IVD has been carried out in clinical practice and has shown promising results. The currently marketed available devices include Beijing 2020 Medical Science & Technology’s Disposable AF Suture Devices (EFIT-I-II-III-IV-V, ELAS-A, SMILE, STAR), The Xclose® Tissue Repair System, The AnchorKnot® Suture-Passing Device and The Barricaid® Annular Closure Device. The devices are shown in Figure 1 and Figure 2. The Xclose® Tissue Repair System reduces the risk of re-protrusion and re-operation, favouring short-term patient outcomes (2 years). It carries no additional risk of surgery. However, postoperative back and leg pain is significant (Bailey et al., 2013; Bartlett et al., 2013; Choy et al., 2018).
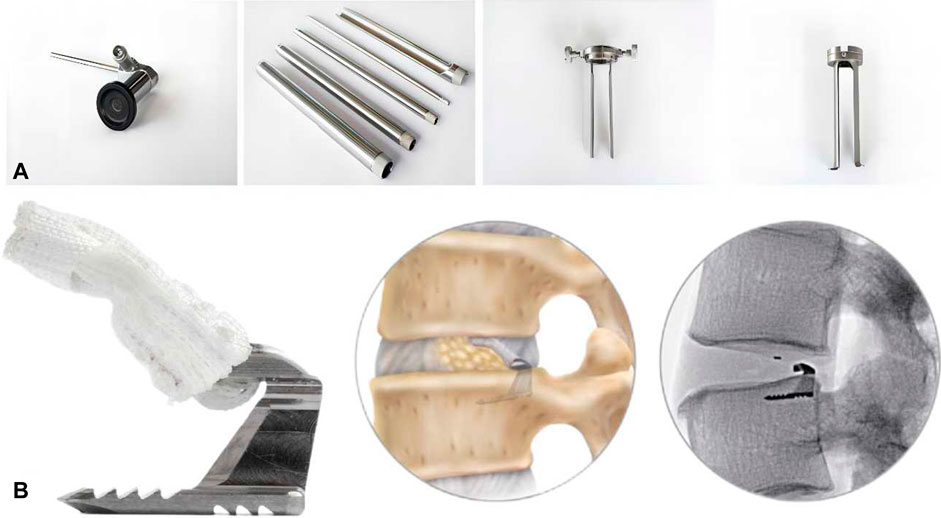
FIGURE 1. (A) The AnchorKnot® Suture-Passing Device. (B) The Barricaid® Annular Closure Device and post-implantation simulation.
The AnchorKnot® Suture-Passing Device allows minimally invasive visualisation of the surgical field and minimises removal of IVD tissue, but safety and efficacy need to be evaluated. The Barricaid® Annular Cloure Device is a titanium body anchored to the adjacent vertebral body that maintains IVD height, reduces pain, decreases reherniation rates and slows the progression of small joint degeneration. However, there are risks of EP fracture, device dislodgement, inflammation, fibrosis, osteolysis, and osteophyte formation (Strenge et al., 2019; Miller et al., 2020; Zengerle et al., 2020; Kienzler et al., 2021; Nunley et al., 2021; Peredo et al., 2021). Parker et al. found that The Barricaid® Annular Closure Device maintained the height of IVD and alleviated symptoms of pain. According to their statistics, the potential savings is approximately $220,000 per 100 surgeries (Parker et al., 2013). A study by Ardeshiri et al. showed that the application of The Barricaid® Annular Closure Device was safe, with a significant reduction in IVD reherniation in postoperative patients (Ardeshiri et al., 2019).
Ren et al. reported that Beijing 2020 Medical Science & Technology’s Disposable AF Suture Devices maintained the height of IVD, relieved postoperative pain and improved function. Its postoperative recurrence rate is lower than that of Percutaneous Transforaminal Endoscopic Discectomy (PTED), but there is no statistically significant difference between them. Table 6 outlines currently marketed available IVD repair devices (Ren et al., 2020).
8 Conclusion and outlook
As the pathophysiology of IDD has been increasingly studied in recent years, various repair strategies for IVD have been proposed, including hydrogel repair, tissue-engineered scaffold repair and several promising repair modalities. These therapeutic strategies aim to restore the mechanical properties of IVD, promote cell proliferation and differentiation to repair AF and promote the production of ECM to maintain the water content of NP. However, mechanical properties and biocompatibility are not well satisfied simultaneously, and clinical application is still far off.
Synthetic hydrogel repair strategies are poorly biocompatible, with advantages in terms of mechanical properties. Natural hydrogel repair strategies are poor in mechanical properties, with advantages in maintaining cell activity, promoting cell proliferation and differentiation, promoting ECM synthesis and maintaining the water content of NP. Composite hydrogel repair strategies can improve the biomechanics of IVDs while ensuring biocompatibility, but strategies to enhance mechanical properties still require exploration. In future, hydrogel repair strategies should guarantee biocompatibility with continuous enhancement of mechanical properties or guarantee mechanical properties with continuous enhancement of biocompatibility.
The priority of the scaffold repair strategy is that the scaffold is in place and provides sufficient mechanical strength. Secondly, it is necessary to achieve the appropriate porosity. If the scaffold is less pore size, it is less conducive to cellular penetration, and if the pore size is too large, it is less conducive to mechanical support. Finally, mimicking the AF structure shows excellent potential to exhibit mechanical properties similar to natural AF and facilitate the deposition of ECM.
Author contributions
YY: Data curation, Methodology, Software, Writing–original draft. KC: Writing–review and editing. XC: Data curation, Writing–review and editing. KZ: Writing–review and editing. RQ: Writing–review and editing. GJ: Writing–review and editing. KL: Writing–review and editing.
Funding
The author(s) declare financial support was received for the research, authorship, and/or publication of this article. This work was supported by Medical and Health Research Project of Zhejiang Province (Grant No: 2021427071).
Acknowledgments
We thank all the members who contributed to this study.
Conflict of interest
The authors declare that the research was conducted in the absence of any commercial or financial relationships that could be construed as a potential conflict of interest.
Publisher’s note
All claims expressed in this article are solely those of the authors and do not necessarily represent those of their affiliated organizations, or those of the publisher, the editors and the reviewers. Any product that may be evaluated in this article, or claim that may be made by its manufacturer, is not guaranteed or endorsed by the publisher.
References
Agnol, L. D., Dias, F. T. G., Nicoletti, N. F., Marinowic, D., Moura E Silva, S., Marcos-Fernandez, A., et al. (2019). Polyurethane tissue adhesives for annulus fibrosus repair: mechanical restoration and cytotoxicity. J. Biomaterials Appl. 34 (5), 673–686. doi:10.1177/0885328219864901
Ahlgren, B. D., Lui, W., Herkowitz, H. N., Panjabi, M. M., and Guiboux, J. P. (2000). Effect of anular repair on the healing strength of the intervertebral disc: a sheep model. Spine 25 (17), 2165–2170. doi:10.1097/00007632-200009010-00004
Aichmair, A., Du, J. Y., Shue, J., Evangelisti, G., Sama, A. A., Hughes, A. P., et al. (2014). Microdiscectomy for the treatment of lumbar disc herniation: an evaluation of reoperations and long-term outcomes. Evidence-based Spine-care J. 5 (2), 077–086. doi:10.1055/s-0034-1386750
Alexeev, D., Cui, S., Grad, S., Li, Z., and Ferguson, S. J. (2020). Mechanical and biological characterization of a composite annulus fibrosus repair strategy in an endplate delamination model. JOR Spine 3 (4), e1107. doi:10.1002/jsp2.1107
Alexeev, D., Tschopp, M., Helgason, B., and Ferguson, S. J. (2021). Electrospun biodegradable poly(ε-caprolactone) membranes for annulus fibrosus repair: long-term material stability and mechanical competence. JOR Spine 4 (1), e1130. doi:10.1002/jsp2.1130
Alinejad, Y., Adoungotchodo, A., Grant, M. P., Epure, L. M., Antoniou, J., Mwale, F., et al. (2019). Injectable chitosan hydrogels with enhanced mechanical properties for nucleus pulposus regeneration. Tissue Eng. 25 (5-6), 303–313. doi:10.1089/ten.TEA.2018.0170
Andersson, G. B. (1999). Epidemiological features of chronic low-back pain. Lancet (London, Engl. 354 (9178), 581–585. doi:10.1016/s0140-6736(99)01312-4
Ardeshiri, A., Miller, L. E., Synowitz, M., and Jadik, S. (2019). Surgical experience and complications in 50 patients treated with an anular closure device following lumbar discectomy. Orthop. Surg. 11 (3), 431–437. doi:10.1111/os.12495
Bailey, A., Araghi, A., Blumenthal, S., and Huffmon, G. V. (2013). Prospective, multicenter, randomized, controlled study of anular repair in lumbar discectomy: two-year follow-up. Spine 38 (14), 1161–1169. doi:10.1097/BRS.0b013e31828b2e2f
Bartlett, A., Wales, L., Houfburg, R., Durfee, W. K., Griffith, S. L., and Bentley, I. (2013). Optimizing the effectiveness of a mechanical suture-based anulus fibrosus repair construct in an acute failure laboratory simulation. J. Spinal Disord. Tech. 26 (7), 393–399. doi:10.1097/BSD.0b013e31824c8224
Bateman, A. H., Balkovec, C., Akens, M. K., Chan, A. H. W., Harrison, R. D., Oakden, W., et al. (2016). Closure of the annulus fibrosus of the intervertebral disc using a novel suture application device-in vivo porcine and ex vivo biomechanical evaluation. Spine J. 16 (7), 889–895. doi:10.1016/j.spinee.2016.03.005
Borem, R., Madeline, A., Vela, R., Gill, S., and Mercuri, J. (2019). Multi-laminate annulus fibrosus repair scaffold with an interlamellar matrix enhances impact resistance, prevents herniation and assists in restoring spinal kinematics. J. Mech. Behav. Biomed. Mater. 95, 41–52. doi:10.1016/j.jmbbm.2019.03.030
Brinjikji, W., Luetmer, P. H., Comstock, B., Bresnahan, B. W., Chen, L. E., Deyo, R. A., et al. (2015). Systematic literature review of imaging features of spinal degeneration in asymptomatic populations. AJNR. Am. J. Neuroradiol. 36 (4), 811–816. doi:10.3174/ajnr.A4173
Bruehlmann, S. B., Rattner, J. B., Matyas, J. R., and Duncan, N. A. (2002). Regional variations in the cellular matrix of the annulus fibrosus of the intervertebral disc. J. Anat. 201 (2), 159–171. doi:10.1046/j.1469-7580.2002.00080.x
Cassidy, J. J., Hiltner, A., and Baer, E. (1989). Hierarchical structure of the intervertebral disc. Connect. Tissue Res. 23 (1), 75–88. doi:10.3109/03008208909103905
Chan, S. C. W., Ferguson, S. J., and Gantenbein-Ritter, B. (2011). The effects of dynamic loading on the intervertebral disc. Eur. Spine J. 20 (11), 1796–1812. doi:10.1007/s00586-011-1827-1
Che, Y.-J., Guo, J.-B., Hao, Y. F., and Luo, Z.-P. (2022). Regenerating and repairing degenerative intervertebral discs by regulating the micro/nano environment of degenerative bony endplates based on low-tension mechanics. BMC Musculoskelet. Disord. 23 (1), 462. doi:10.1186/s12891-022-05422-6
Che, Y.-J., Guo, J.-B., Liang, T., Chen, X., Zhang, W., Yang, H.-L., et al. (2019). Controlled immobilization-traction based on intervertebral stability is conducive to the regeneration or repair of the degenerative disc: an in vivo study on the rat coccygeal model. Spine J. 19 (5), 920–930. doi:10.1016/j.spinee.2018.10.018
Che, Y.-J., Hou, J.-J., Guo, J.-B., Liang, T., Zhang, W., Lu, Y., et al. (2021). Low energy extracorporeal shock wave therapy combined with low tension traction can better reshape the microenvironment in degenerated intervertebral disc regeneration and repair. Spine J. 21 (1), 160–177. doi:10.1016/j.spinee.2020.08.004
Chen, J.-X., Li, Y.-H., Wen, J., Li, Z., Yu, B.-S., and Huang, Y.-C. (2023). Annular defects impair the mechanical stability of the intervertebral disc. Glob. Spine J. 13 (3), 724–729. doi:10.1177/21925682211006061
Choi, K.-S., Cohn, M. J., and Harfe, B. D. (2008). Identification of nucleus pulposus precursor cells and notochordal remnants in the mouse: implications for disk degeneration and chordoma formation. Dev. Dyn. 237 (12), 3953–3958. doi:10.1002/dvdy.21805
Choy, W. J., Phan, K., Diwan, A. D., Ong, C. S., and Mobbs, R. J. (2018). Annular closure device for disc herniation: meta-analysis of clinical outcome and complications. BMC Musculoskelet. Disord. 19 (1), 290. doi:10.1186/s12891-018-2213-5
Christiani, T. R., Baroncini, E., Stanzione, J., and Vernengo, A. J. (2019). In vitro evaluation of 3D printed polycaprolactone scaffolds with angle-ply architecture for annulus fibrosus tissue engineering. Regen. Biomater. 6 (3), 175–184. doi:10.1093/rb/rbz011
Costi, J. J., Freeman, B. J. C., and Elliott, D. M. (2011). Intervertebral disc properties: challenges for biodevices. Expert Rev. Med. Devices 8 (3), 357–376. doi:10.1586/erd.11.1
Cruz, M. A., Hom, W. W., DiStefano, T. J., Merrill, R., Torre, O. M., Lin, H. A., et al. (2018). Cell-Seeded adhesive biomaterial for repair of annulus fibrosus defects in intervertebral discs. Tissue Eng. Part A 24 (3-4), 187–198. doi:10.1089/ten.TEA.2017.0334
Dewle, A., Rakshasmare, P., and Srivastava, A. (2021). A polycaprolactone (PCL)-Supported electrocompacted aligned collagen type-I patch for annulus fibrosus repair and regeneration. ACS Appl. Bio Mater. 4 (2), 1238–1251. doi:10.1021/acsabm.0c01084
DiStefano, T. J., Shmukler, J. O., Danias, G., Di Pauli von Treuheim, T., Hom, W. W., Goldberg, D. A., et al. (2020). Development of a two-part biomaterial adhesive strategy for annulus fibrosus repair and ex vivo evaluation of implant herniation risk. Biomaterials 258, 120309. doi:10.1016/j.biomaterials.2020.120309
Doench, I., Ahn Tran, T., David, L., Montembault, A., Viguier, E., Gorzelanny, C., et al. (2019). Cellulose nanofiber-reinforced chitosan hydrogel composites for intervertebral disc tissue repair. Biomimetics (Basel, Switz. 4 (1), 19. doi:10.3390/biomimetics4010019
Du, J., Long, R. G., Nakai, T., Sakai, D., Benneker, L. M., Zhou, G., et al. (2020). Functional cell phenotype induction with TGF-β1 and collagen-polyurethane scaffold for annulus fibrosus rupture repair. Eur. Cells Mater. 39, 1–17. doi:10.22203/eCM.v039a01
Du, Z.-C., and Zhu, L.-X. (2019). A heterologous fibrin glue enhances the closure effect of surgical suture on the repair of annulus fibrous defect in a sheep model. Curr. Med. Sci. 39 (4), 597–603. doi:10.1007/s11596-019-2079-2
Elliott, D. M., and Setton, L. A. (2001). Anisotropic and inhomogeneous tensile behavior of the human anulus fibrosus: experimental measurement and material model predictions. J. Biomechanical Eng. 123 (3), 256–263. doi:10.1115/1.1374202
Errington, R. J., Puustjarvi, K., White, I. R., Roberts, S., and Urban, J. P. (1998). Characterisation of cytoplasm-filled processes in cells of the intervertebral disc. J. Anat. 192 (3), 369–378. doi:10.1046/j.1469-7580.1998.19230369.x
Eyre, D. R., and Muir, H. (1977). Quantitative analysis of types I and II collagens in human intervertebral discs at various ages. Biochimica Biophysica Acta 492 (1), 29–42. doi:10.1016/0005-2795(77)90211-2
Eyre, D. R., and Muir, H. (1976). Types I and II collagens in intervertebral disc. Interchanging radial distributions in annulus fibrosus. Biochem. J. 157 (1), 267–270. doi:10.1042/bj1570267
Frauchiger, D. A., May, R. D., Bakirci, E., Tekari, A., Chan, S. C. W., Wöltje, M., et al. (2018). Genipin-enhanced fibrin hydrogel and novel silk for intervertebral disc repair in a loaded bovine organ culture model. J. Funct. Biomaterials 9 (3), 40. doi:10.3390/jfb9030040
Fujii, K., Lai, A., Korda, N., Hom, W. W., Evashwick-Rogler, T. W., Nasser, P., et al. (2020). Ex-vivo biomechanics of repaired rat intervertebral discs using genipin crosslinked fibrin adhesive hydrogel. J. Biomechanics 113, 110100. doi:10.1016/j.jbiomech.2020.110100
Gauvin, R., Parenteau-Bareil, R., Dokmeci, M. R., Merryman, W. D., and Khademhosseini, A. (2012). Hydrogels and microtechnologies for engineering the cellular microenvironment. Wiley Interdiscip. Rev. Nanomedicine Nanobiotechnology 4 (3), 235–246. doi:10.1002/wnan.171
Gelalis, I. D., Christoforou, G., Charchanti, A., Gkiatas, I., Pakos, E., Papadopoulos, D., et al. (2019). Autologous platelet-rich plasma (PRP) effect on intervertebral disc restoration: an experimental rabbit model. Eur. J. Orthop. Surg. Traumatology 29 (3), 545–551. doi:10.1007/s00590-018-2337-1
Gluais, M., Clouet, J., Fusellier, M., Decante, C., Moraru, C., Dutilleul, M., et al. (2019). In vitro and in vivo evaluation of an electrospun-aligned microfibrous implant for Annulus fibrosus repair. Biomaterials 205, 81–93. doi:10.1016/j.biomaterials.2019.03.010
Guo, J.-B., Che, Y.-J., Hou, J.-J., Liang, T., Zhang, W., Lu, Y., et al. (2020). Stable mechanical environments created by a low-tension traction device is beneficial for the regeneration and repair of degenerated intervertebral discs. Spine J. 20 (9), 1503–1516. doi:10.1016/j.spinee.2020.04.005
Guterl, C. C., See, E. Y., Blanquer, S. B. G., Pandit, A., Ferguson, S. J., Benneker, L. M., et al. (2013). Challenges and strategies in the repair of ruptured annulus fibrosus. Eur. Cells Mater. 25, 1–21. doi:10.22203/ecm.v025a01
Häkkinen, A., Kiviranta, I., Neva, M. H., Kautiainen, H., and Ylinen, J. (2007). Reoperations after first lumbar disc herniation surgery; a special interest on residives during a 5-year follow-up. BMC Musculoskelet. Disord. 8, 2. doi:10.1186/1471-2474-8-2
Hampton, D., Laros, G., McCarron, R., and Franks, D. (1989). Healing potential of the anulus fibrosus. Spine 14 (4), 398–401. doi:10.1097/00007632-198904000-00009
Hartvigsen, J., Hancock, M. J., Kongsted, A., Louw, Q., Ferreira, M. L., Genevay, S., et al. (2018). What low back pain is and why we need to pay attention. Lancet (London, Engl. 391 (10137), 2356–2367. doi:10.1016/S0140-6736(18)30480-X
Heikkilä, J. K., Koskenvuo, M., Heliövaara, M., Kurppa, K., Riihimäki, H., Heikkilä, K., et al. (1989). Genetic and environmental factors in sciatica. Evidence from a nationwide panel of 9365 adult twin pairs. Ann. Med. 21 (5), 393–398. doi:10.3109/07853898909149227
Helgeson, M. D., Bevevino, A. J., and Hilibrand, A. S. (2013). Update on the evidence for adjacent segment degeneration and disease. Spine J. 13 (3), 342–351. doi:10.1016/j.spinee.2012.12.009
Hestbaek, L., Leboeuf-Yde, C., and Manniche, C. (2003). Low back pain: what is the long-term course? A review of studies of general patient populations. Eur. Spine J. 12 (2), 149–165. doi:10.1007/s00586-002-0508-5
Heuer, F., Ulrich, S., Claes, L., and Wilke, H.-J. (2008). Biomechanical evaluation of conventional anulus fibrosus closure methods required for nucleus replacement. Laboratory investigation. J. Neurosurg. 9 (3), 307–313. doi:10.3171/spi/2008/9/9/307
Hicks, G. E., Morone, N., and Weiner, D. K. (2009). Degenerative lumbar disc and facet disease in older adults: prevalence and clinical correlates. Spine 34 (12), 1301–1306. doi:10.1097/BRS.0b013e3181a18263
Hom, W. W., Tschopp, M., Lin, H. A., Nasser, P., Laudier, D. M., Hecht, A. C., et al. (2019). Composite biomaterial repair strategy to restore biomechanical function and reduce herniation risk in an ex vivo large animal model of intervertebral disc herniation with varying injury severity. PloS One 14 (5), e0217357. doi:10.1371/journal.pone.0217357
Hoy, D., March, L., Brooks, P., Blyth, F., Woolf, A., Bain, C., et al. (2014). The global burden of low back pain: estimates from the global burden of disease 2010 study. Ann. Rheumatic Dis. 73 (6), 968–974. doi:10.1136/annrheumdis-2013-204428
Huang, Y.-C., Leung, V. Y. L., Lu, W. W., and Luk, K. D. K. (2013). The effects of microenvironment in mesenchymal stem cell-based regeneration of intervertebral disc. Spine J. 13 (3), 352–362. doi:10.1016/j.spinee.2012.12.005
Hughes, S. P. F., Freemont, A. J., Hukins, D. W. L., McGregor, A. H., and Roberts, S. (2012). The pathogenesis of degeneration of the intervertebral disc and emerging therapies in the management of back pain. J. Bone Jt. Surg. 94 (10), 1298–1304. doi:10.1302/0301-620x.94b10.28986
Humzah, M. D., and Soames, R. W. (1988). Human intervertebral disc: structure and function. Anatomical Rec. 220 (4), 337–356. doi:10.1002/ar.1092200402
Hwang, M. H., Lee, J. W., Son, H.-G., Kim, J., and Choi, H. (2020). Effects of photobiomodulation on annulus fibrosus cells derived from degenerative disc disease patients exposed to microvascular endothelial cells conditioned medium. Sci. Rep. 10 (1), 9655. doi:10.1038/s41598-020-66689-0
Ishihara, H., and Urban, J. P. (1999). Effects of low oxygen concentrations and metabolic inhibitors on proteoglycan and protein synthesis rates in the intervertebral disc. J. Orthop. Res. 17 (6), 829–835. doi:10.1002/jor.1100170607
Jiang, E. Y., Sloan, S. R., Wipplinger, C., Kirnaz, S., Härtl, R., and Bonassar, L. J. (2019). Proteoglycan removal by chondroitinase ABC improves injectable collagen gel adhesion to annulus fibrosus. Acta Biomater. 97, 428–436. doi:10.1016/j.actbio.2019.08.024
Kang, R., Li, H., Xi, Z., Ringgard, S., Baatrup, A., Rickers, K., et al. (2018). Surgical repair of annulus defect with biomimetic multilamellar nano/microfibrous scaffold in a porcine model. J. Tissue Eng. Regen. Med. 12 (1), 164–174. doi:10.1002/term.2384
Kienzler, J. C., Heidecke, V., Assaker, R., Fandino, J., and Barth, M. (2021). Intraoperative findings, complications, and short-term results after lumbar microdiscectomy with or without implantation of annular closure device. Acta Neurochir. 163 (2), 545–559. doi:10.1007/s00701-020-04612-2
Kim, H. S., Adsul, N., Yudoyono, F., Paudel, B., Kim, K. J., Choi, S. H., et al. (2018). Transforaminal epiduroscopic basivertebral nerve laser ablation for chronic low back pain associated with modic changes: A preliminary open-label study. Pain Res. Manag. 2018, 1–7. doi:10.1155/2018/6857983
Kim, H. S., Wu, P. H., and Jang, I.-T. (2020). Lumbar degenerative disease Part 1: anatomy and pathophysiology of intervertebral discogenic pain and radiofrequency ablation of basivertebral and sinuvertebral nerve treatment for chronic discogenic back pain: A prospective case series and review of literature. Int. J. Mol. Sci. 21 (4), 1483. doi:10.3390/ijms21041483
Klein, J. A., Hickey, D. S., and Hukins, D. W. (1983). Radial bulging of the annulus fibrosus during compression of the intervertebral disc. J. Biomechanics 16 (3), 211–217. doi:10.1016/0021-9290(83)90128-8
Leone, G., Consumi, M., Lamponi, S., Bonechi, C., Tamasi, G., Donati, A., et al. (2019). Thixotropic PVA hydrogel enclosing a hydrophilic PVP core as nucleus pulposus substitute. Mater. Sci. Eng. 98, 696–704. doi:10.1016/j.msec.2019.01.039
Lewns, F. K., Tsigkou, O., Cox, L. R., Wildman, R. D., Grover, L. M., and Poologasundarampillai, G. (2023). Hydrogels and bioprinting in bone tissue engineering: creating artificial stem-cell niches for in vitro models. Adv. Mater. 2023, e2301670. doi:10.1002/adma.202301670
Li, Z.-Z., Cao, Z., Zhao, H.-L., Shang, W.-L., and Hou, S.-X. (2020). A pilot study of full-endoscopic annulus fibrosus suture following lumbar discectomy: technique notes and one-year follow-up. Pain Physician 23 (5), E497–E505. doi:10.36076/ppj.2020/23/e497
Long, R. G., Rotman, S. G., Hom, W. W., Assael, D. J., Illien-Jünger, S., Grijpma, D. W., et al. (2018). In vitro and biomechanical screening of polyethylene glycol and poly(trimethylene carbonate) block copolymers for annulus fibrosus repair. J. Tissue Eng. Regen. Med. 12 (2), e727–e736. doi:10.1002/term.2356
Lotz, J. C., Colliou, O. K., Chin, J. R., Duncan, N. A., and Liebenberg, E. (1998). Compression-induced degeneration of the intervertebral disc: an in vivo mouse model and finite-element study. Spine 23 (23), 2493–2506. doi:10.1097/00007632-199812010-00004
Lotz, J. C., and Ulrich, J. A. (2006). Innervation, inflammation, and hypermobility may characterize pathologic disc degeneration: review of animal model data. J. Bone Jt. Surg. 88 (2), 76–82. doi:10.2106/jbjs.e.01448
Ma, J., He, Y., Liu, X., Chen, W., Wang, A., Lin, C.-Y., et al. (2018). A novel electrospun-aligned nanoyarn/three-dimensional porous nanofibrous hybrid scaffold for annulus fibrosus tissue engineering. Int. J. Nanomedicine 13, 1553–1567. doi:10.2147/IJN.S143990
March, L., Smith, E. U. R., Hoy, D. G., Cross, M. J., Sanchez-Riera, L., Blyth, F., et al. (2014). Burden of disability due to musculoskeletal (MSK) disorders. Best Pract. Res. Clin. Rheumatology 28 (3), 353–366. doi:10.1016/j.berh.2014.08.002
Marchand, F., and Ahmed, A. M. (1990). Investigation of the laminate structure of lumbar disc anulus fibrosus. Spine 15 (5), 402–410. doi:10.1097/00007632-199005000-00011
Maroudas, A., Stockwell, R. A., Nachemson, A., and Urban, J. (1975). Factors involved in the nutrition of the human lumbar intervertebral disc: cellularity and diffusion of glucose in vitro. J. Anat. 120 (1), 113–130.
Matsumoto, M., Okada, E., Ichihara, D., Watanabe, K., Chiba, K., Toyama, Y., et al. (2010). Age-related changes of thoracic and cervical intervertebral discs in asymptomatic subjects. Spine 35 (14), 1359–1364. doi:10.1097/BRS.0b013e3181c17067
Miller, L. E., Allen, R. T., Duhon, B., and Radcliff, K. E. (2020). Expert review with meta-analysis of randomized and nonrandomized controlled studies of Barricaid annular closure in patients at high risk for lumbar disc reherniation. Expert Rev. Med. Devices 17 (5), 461–469. doi:10.1080/17434440.2020.1745061
Mizuno, H., Roy, A. K., Vacanti, C. A., Kojima, K., Ueda, M., and Bonassar, L. J. (2004). Tissue-engineered composites of anulus fibrosus and nucleus pulposus for intervertebral disc replacement. Spine 29 (12), 1290–1297. doi:10.1097/01.brs.0000128264.46510.27
Moriguchi, Y., Borde, B., Berlin, C., Wipplinger, C., Sloan, S. R., Kirnaz, S., et al. (2018). In vivo annular repair using high-density collagen gel seeded with annulus fibrosus cells. Acta Biomater. 79, 230–238. doi:10.1016/j.actbio.2018.07.008
Morlion, B. (2013). Chronic low back pain: pharmacological, interventional and surgical strategies. Nat. Rev. Neurol. 9 (8), 462–473. doi:10.1038/nrneurol.2013.130
Mwale, F., Masuda, K., Grant, M. P., Epure, L. M., Kato, K., Miyazaki, S., et al. (2018). Short Link N promotes disc repair in a rabbit model of disc degeneration. Arthritis Res. Ther. 20 (1), 201. doi:10.1186/s13075-018-1625-9
Nachemson, A., Lewin, T., Maroudas, A., and Freeman, M. A. (1970). In vitro diffusion of dye through the end-plates and the annulus fibrosus of human lumbar inter-vertebral discs. Acta Orthop. Scand. 41 (6), 589–607. doi:10.3109/17453677008991550
Nunley, P., Strenge, K. B., Huntsman, K., Bae, H., DiPaola, C., T, A. R., et al. (2021). Lumbar discectomy with Barricaid device implantation in patients at high risk of reherniation: initial results from a postmarket study. Cureus 13 (12), e20274. doi:10.7759/cureus.20274
O'Connell, G. D., Guerin, H. L., and Elliott, D. M. (2009). Theoretical and uniaxial experimental evaluation of human annulus fibrosus degeneration. J. Biomechanical Eng. 131 (11), 111007. doi:10.1115/1.3212104
Oegema, T. R., Johnson, S. L., Aguiar, D. J., and Ogilvie, J. W. (2000). Fibronectin and its fragments increase with degeneration in the human intervertebral disc. Spine 25 (21), 2742–2747. doi:10.1097/00007632-200011010-00005
Osti, O. L., Vernon-Roberts, B., and Fraser, R. D. (1990). 1990 volvo award in experimental studies: anulus tears and intervertebral disc degeneration: an experimental study using an animal model. Spine 15 (8), 762–767. doi:10.1097/00007632-199008010-00005
Page, M., and Puttlitz, C. (2019). Biaxial mechanics of 3D fiber deposited ply-laminate scaffolds for soft tissue engineering part II: finite element analyses. J. Mech. Behav. Biomed. Mater. 100, 103395. doi:10.1016/j.jmbbm.2019.103395
Panebianco, C. J., DiStefano, T. J., Mui, B., Hom, W. W., and Iatridis, J. C. (2020). Crosslinker concentration controls TGFβ-3 release and annulus fibrosus cell apoptosis in genipin-crosslinked fibrin hydrogels. Eur. Cells Mater. 39, 211–226. doi:10.22203/eCM.v039a14
Parker, S. L., Grahovac, G., Vukas, D., Ledic, D., Vilendecic, M., and McGirt, M. J. (2013). Cost savings associated with prevention of recurrent lumbar disc herniation with a novel annular closure device: a multicenter prospective cohort study. J. Neurological Surg. 74 (5), 285–289. doi:10.1055/s-0033-1341416
Pattappa, G., Li, Z., Peroglio, M., Wismer, N., Alini, M., and Grad, S. (2012). Diversity of intervertebral disc cells: phenotype and function. J. Anat. 221 (6), 480–496. doi:10.1111/j.1469-7580.2012.01521.x
Peng, Y., Huang, D., Li, J., Liu, S., Qing, X., and Shao, Z. (2020). Genipin-crosslinked decellularized annulus fibrosus hydrogels induces tissue-specific differentiation of bone mesenchymal stem cells and intervertebral disc regeneration. J. Tissue Eng. Regen. Med. 14 (3), 497–509. doi:10.1002/term.3014
Peredo, A. P., Gullbrand, S. E., Mauck, R. L., and Smith, H. E. (2021). A challenging playing field: identifying the endogenous impediments to annulus fibrosus repair. JOR Spine 4 (1), e1133. doi:10.1002/jsp2.1133
Pereira, D. R., Silva-Correia, J., Oliveira, J. M., Reis, R. L., Pandit, A., and Biggs, M. J. (2018). Nanocellulose reinforced gellan-gum hydrogels as potential biological substitutes for annulus fibrosus tissue regeneration. Nanomedicine 14 (3), 897–908. doi:10.1016/j.nano.2017.11.011
Permana, G. I., Bajamal, A. H., Subagio, E. A., Parenrengi, M. A., Rasyida, A., and Utomo, B. (2022). Novel Silicone rubber and polyvinyl alcohol (PVA) compound as nucleus pulposus replacement in intervertebral disc herniation surgery. Turk. Neurosurg. 32 (5), 779–785. doi:10.5137/1019-5149.JTN.35925-21.3
Raj, P. P. (2008). Intervertebral disc: anatomy-physiology-pathophysiology-treatment. Pain Pract. 8 (1), 18–44. doi:10.1111/j.1533-2500.2007.00171.x
Ren, C., Qin, R., Li, Y., and Wang, P. (2020). Microendoscopic discectomy combined with annular suture versus percutaneous transforaminal endoscopic discectomy for lumbar disc herniation: A prospective observational study. Pain Physician 23 (6), E713–E721.
Rickers, K., Bendtsen, M., Le, D. Q. S., Veen, A. J. d., and Bünger, C. E. (2018). Biomechanical evaluation of annulus fibrosus repair with scaffold and soft anchors in an ex vivo porcine model. SICOT-J 4, 38. doi:10.1051/sicotj/2018020
Roberts, S., Evans, H., Trivedi, J., and Menage, J. (2006). Histology and pathology of the human intervertebral disc. J. Bone Jt. Surg. Am. Volume 88 (2), 10–14. doi:10.2106/jbjs.f.00019
Sabnis, A. B., and Diwan, A. D. (2014). The timing of surgery in lumbar disc prolapse: A systematic review. Indian J. Orthop. 48 (2), 127–135. doi:10.4103/0019-5413.128740
Scheibler, A.-G., Götschi, T., Widmer, J., Holenstein, C., Steffen, T., Camenzind, R. S., et al. (2018). Feasibility of the annulus fibrosus repair with in situ gelating hydrogels - a biomechanical study. PloS One 13 (12), e0208460. doi:10.1371/journal.pone.0208460
Schmitz, T. C., van Genabeek, B., Pouderoijen, M. J., Janssen, H. M., van Doeselaar, M., Crispim, J. F., et al. (2023). Semi-synthetic degradable notochordal cell-derived matrix hydrogel for use in degenerated intervertebral discs: initial in vitro characterization. J. Biomed. Mater. Res. 2023, 37594. doi:10.1002/jbm.a.37594
Shamsah, A. H., Cartmell, S. H., Richardson, S. M., and Bosworth, L. A. (2020). Material characterization of PCL:PLLA electrospun fibers following six months degradation in vitro. Polymers 12 (3), 700. doi:10.3390/polym12030700
Shamsah, A. H., Cartmell, S. H., Richardson, S. M., and Bosworth, L. A. (2019). Mimicking the annulus fibrosus using electrospun polyester blended scaffolds. Nanomater. (Basel, Switz. 9 (4), 537. doi:10.3390/nano9040537
Sharabi, M., Wertheimer, S., Wade, K. R., Galbusera, F., Benayahu, D., Wilke, H.-J., et al. (2019). Towards intervertebral disc engineering: bio-mimetics of form and function of the annulus fibrosus lamellae. J. Mech. Behav. Biomed. Mater. 94, 298–307. doi:10.1016/j.jmbbm.2019.03.023
Sloan, S. R., Wipplinger, C., Kirnaz, S., Navarro-Ramirez, R., Schmidt, F., McCloskey, D., et al. (2020). Combined nucleus pulposus augmentation and annulus fibrosus repair prevents acute intervertebral disc degeneration after discectomy. Sci. Transl. Med. 12 (534), eaay2380. doi:10.1126/scitranslmed.aay2380
Storm, P. B., Chou, D., and Tamargo, R. J. (2002). Surgical management of cervical and lumbosacral radiculopathies: indications and outcomes. Phys. Med. Rehabilitation Clin. N. Am. 13 (3), 735–759. doi:10.1016/s1047-9651(02)00014-1
Strenge, K. B., DiPaola, C. P., Miller, L. E., Hill, C. P., and Whitmore, R. G. (2019). Multicenter study of lumbar discectomy with Barricaid annular closure device for prevention of lumbar disc reherniation in US patients: A historically controlled post-market study protocol. Medicine 98 (35), e16953. doi:10.1097/MD.0000000000016953
Tsujimoto, T., Sudo, H., Todoh, M., Yamada, K., Iwasaki, K., Ohnishi, T., et al. (2018). An acellular bioresorbable ultra-purified alginate gel promotes intervertebral disc repair: A preclinical proof-of-concept study. EBioMedicine 37, 521–534. doi:10.1016/j.ebiom.2018.10.055
Ura, K., Yamada, K., Tsujimoto, T., Ukeba, D., Iwasaki, N., and Sudo, H. (2021). Ultra-purified alginate gel implantation decreases inflammatory cytokine levels, prevents intervertebral disc degeneration, and reduces acute pain after discectomy. Sci. Rep. 11 (1), 638. doi:10.1038/s41598-020-79958-9
van den Eerenbeemt, K. D., Ostelo, R. W., van Royen, B. J., Peul, W. C., and van Tulder, M. W. (2010). Total disc replacement surgery for symptomatic degenerative lumbar disc disease: a systematic review of the literature. Eur. Spine J. 19 (8), 1262–1280. doi:10.1007/s00586-010-1445-3
Vernon-Roberts, B., Moore, R. J., and Fraser, R. D. (2007). The natural history of age-related disc degeneration: the pathology and sequelae of tears. Spine 32 (25), 2797–2804. doi:10.1097/BRS.0b013e31815b64d2
Wang, S., He, Y.-F., Ma, J., Yu, L., Wen, J.-K., and Ye, X.-J. (2020). Dynamic bioreactor culture for infiltration of bone mesenchymal stem cells within electrospun nanofibrous scaffolds for annulus fibrosus repair. Orthop. Surg. 12 (1), 304–311. doi:10.1111/os.12615
Wang, Y., Bai, B., Hu, Y., Wang, H., Liu, N., Li, Y., et al. (2021a). Hydrostatic pressure modulates intervertebral disc cell survival and extracellular matrix homeostasis via regulating hippo-YAP/TAZ pathway. Stem Cells Int. 2021, 1–17. doi:10.1155/2021/5626487
Wang, Y., Zhang, Y., Chen, K., Shao, F., Wu, Y., Guo, C., et al. (2021b). Injectable nanostructured colloidal gels resembling native nucleus pulposus as carriers of mesenchymal stem cells for the repair of degenerated intervertebral discs. Mater. Sci. Eng. C, Mater. For Biol. Appl. 128, 112343. doi:10.1016/j.msec.2021.112343
Wei, Q., Liu, D., Chu, G., Yu, Q., Liu, Z., Li, J., et al. (2023). TGF-β1-supplemented decellularized annulus fibrosus matrix hydrogels promote annulus fibrosus repair. Bioact. Mater. 19, 581–593. doi:10.1016/j.bioactmat.2022.04.025
Wu, P. H., Kim, H. S., and Jang, I.-T. (2020). Intervertebral disc diseases PART 2: A review of the current diagnostic and treatment strategies for intervertebral disc disease. Int. J. Mol. Sci. 21 (6), 2135. doi:10.3390/ijms21062135
Yan, C., Wang, X., Xiang, C., Wang, Y., Pu, C., Chen, L., et al. (2021). Applications of functionalized hydrogels in the regeneration of the intervertebral disc. BioMed Res. Int. 2021, 1–19. doi:10.1155/2021/2818624
Yang, X., Chen, Z., Chen, C., Han, C., Zhou, Y., Li, X., et al. (2021). Bleomycin induces fibrotic transformation of bone marrow stromal cells to treat height loss of intervertebral disc through the TGFβR1/Smad2/3 pathway. Stem Cell Res. Ther. 12 (1), 34. doi:10.1186/s13287-020-02093-9
Zengerle, L., Köhler, A., Debout, E., Hackenbroch, C., and Wilke, H.-J. (2020). Nucleus replacement could get a new chance with annulus closure. Eur. Spine J. 29 (7), 1733–1741. doi:10.1007/s00586-020-06419-2
Zhang, Y., Tian, Z., Gerard, D., Yao, L., Shofer, F. S., Cs-Szabo, G., et al. (2021). Elevated inflammatory gene expression in intervertebral disc tissues in mice with ADAM8 inactivated. Sci. Rep. 11 (1), 1804. doi:10.1038/s41598-021-81495-y
Zhou, P., Chu, G., Yuan, Z., Wang, H., Zhang, W., Mao, Y., et al. (2021). Regulation of differentiation of annulus fibrosus-derived stem cells using heterogeneous electrospun fibrous scaffolds. J. Orthop. Transl. 26, 171–180. doi:10.1016/j.jot.2020.02.003
Keywords: intervertebral disc, fibrous annulus, degeneration, repair, tissue engineering, biomaterials, hydrogel, scaffold
Citation: Ying Y, Cai K, Cai X, Zhang K, Qiu R, Jiang G and Luo K (2023) Recent advances in the repair of degenerative intervertebral disc for preclinical applications. Front. Bioeng. Biotechnol. 11:1259731. doi: 10.3389/fbioe.2023.1259731
Received: 16 July 2023; Accepted: 14 September 2023;
Published: 22 September 2023.
Edited by:
Farnaz Ghorbani, University of Bristol, United KingdomReviewed by:
Sudarshan Singh, Chiang Mai University, ThailandDeepanjan Datta, Manipal University, India
Copyright © 2023 Ying, Cai, Cai, Zhang, Qiu, Jiang and Luo. This is an open-access article distributed under the terms of the Creative Commons Attribution License (CC BY). The use, distribution or reproduction in other forums is permitted, provided the original author(s) and the copyright owner(s) are credited and that the original publication in this journal is cited, in accordance with accepted academic practice. No use, distribution or reproduction is permitted which does not comply with these terms.
*Correspondence: Kefeng Luo, Drluo2022@163.com