- 1Liaoning Research Institute of Family Planning, China Medical University, Shenyang, China
- 2Medical School, Shandong Modern University, Jinan, China
- 3Department of Plastic Surgery, The First Hospital of China Medical University, Shenyang, China
Achilles tendon (AT) injury is one of the most common tendon injuries, especially in athletes, the elderly, and working-age people. In AT injury, the biomechanical properties of the tendon are severely affected, leading to abnormal function. In recent years, many efforts have been underway to develop effective treatments for AT injuries to enable patients to return to sports faster. For instance, several new techniques for tissue-engineered biological augmentation for tendon healing, growth factors (GFs), gene therapy, and mesenchymal stem cells were introduced. Increasing evidence has suggested that GFs can reduce inflammation, promote extracellular matrix production, and accelerate AT repair. In this review, we highlighted some recent investigations regarding the role of GFs, such as transforming GF-β(TGF-β), bone morphogenetic proteins (BMP), fibroblast GF (FGF), vascular endothelial GF (VEGF), platelet-derived GF (PDGF), and insulin-like GF (IGF), in tendon healing. In addition, we summarized the clinical trials and animal experiments on the efficacy of GFs in AT repair. We also highlighted the advantages and disadvantages of the different isoforms of TGF-β and BMPs, including GFs combined with stem cells, scaffolds, or other GFs. The strategies discussed in this review are currently in the early stages of development. It is noteworthy that although these emerging technologies may potentially develop into substantial clinical treatment options for AT injury, definitive conclusions on the use of these techniques for routine management of tendon ailments could not be drawn due to the lack of data.
1 Introduction
Achilles tendon (AT) injury is a clinically intractable tendon disorder that severely affects patients’ daily activities, imposing a significant clinical burden on healthcare systems worldwide. Naturally healed AT typically have poor quality, as they are prone to developing fibrotic scars and tendon sheath adhesion. These tendons lack the necessary biomechanical properties for proper function and are susceptible to re-injury and re-rupture during exercise and daily activities. This is primarily due to the low number of cells, poor blood supply, and low tendon metabolism (Jiang et al., 2014; Veronesi et al., 2015; Li et al., 2021b). At present, there is a lack of effective clinical treatments to promote functional and anatomical recovery for AT injury (Steinmann et al., 2020). The goal of any therapeutic intervention is to expedite the restoration of complete mechanical strength and deliver a regenerated tendon that closely resembles the original, uninjured state. The healing process of tendons is facilitated and directed by a diverse range of GFs that are produced within the local area. So, recently, the focus has been on the biological pathways by which tendons heal and the GFs involved. GFs involved in cell differentiation, angiogenesis, and extracellular matrix (ECM) production play a vital role in regulating cellular life activities. Therefore, GFs may be promising therapeutics for skin wounds, burns, and nonhealing chronic and diabetic wounds (Legrand and Martino, 2022). GFs also play a critical role in the natural regeneration of tendons as they participate in cell recruitment and stimulation of ECM synthesis. Studies have shown that cells within the paratendinous tissue promote healing in AT injury when stimulated by GFs (Muller et al., 2019). Based on this finding, many studies have focused more closely on how to effectively incorporate GFs into damaged tissues and determine their effects. GFs such as TGF-β, BMP, FGF, VEGF, IGF, and PDGF have shown promising applications in AT repair.
GFs can be administered percutaneously, during open surgery, or through carriers such as scaffolds or coated suture materials. These methods are intended to maintain the concentration of GFs in local areas. However, apart from platelet-rich plasma (PRP), none of these GFs have been successfully implemented in clinical practice due to a lack of compelling preclinical evidence. Single GFs have limited effectiveness in promoting tendon healing, both in laboratory settings and animal models. This suggests that a combination of different GFs/stem cells/ scaffolds may be required to significantly enhance the intricate process of tendon healing. There is ample evidence supporting the use of GFs to improve tendon healing. However, several unresolved issues still exist, such as determining which specific GFs to utilize and the optimal timing and method of their delivery.
In this review, we summarized the advantages and disadvantages of the different isoforms of TGF-β and BMP in AT healing, including GFs combined with stem cells, scaffolds, or other GFs. In addition, we summarized the clinical trials and animal experiments on the efficacy of GFs in AT repair. The findings of studies using PRP had controversial conclusions. Although these emerging basic studies provided extensive information on the therapeutic effects of GFs in AT repair, there is still a long way before they are implemented in clinical use.
2 Anatomical characteristics of the AT
The AT is the strongest and largest tendon and is subjected to the highest loads in the human body (McCartney et al., 2019). The tendon has good elasticity and extensibility and plays a vital role in daily life, especially in sports. The AT begins at the musculotendinous junction of the gastrocnemius and soleus muscles and consists of the combined tendons of the soleus, gastrocnemius, and plantaris muscles. The plantaris muscle varies in size and is absent in approximately 6%–8% of individuals (O'Brien, 2005). Generally, the length and thickness of the AT vary between subjects. In adults, the average size of the AT is 15 cm (range, 11–26 cm), and the mean width is 6.8 cm at its origin, 1.8 cm at the midsection, and 3.4 cm at its insertion to the midpoint of the posterior surface of the calcaneus (Doral et al., 2010). Furthermore, the proportion of gastrocnemius and soleus tendons involved in the composition of the AT slightly varies in different individuals (Wasniewska et al., 2022). At approximately 8–10 cm above the termination, the gastrocnemius and soleus tendons fuse completely (Ahmed et al., 1998). Spiral torsion occurs when the tendon fibers move to the termination. The gastrocnemius tendon attaches to the lateral and posterior sides of the calcaneus, while the soleus tendon attaches to the medial and anterior sides of the calcaneus (Dayton, 2017; Winnicki et al., 2020). Studies have confirmed that spiral torsion results in less fiber bending when the ankle is in plantar flexion and less deformation when tension is applied to the AT (Pekala et al., 2017). However, the spiral torsion site, 2–6 cm from the termination, has a relatively poor blood supply and is most prone to rupture and degeneration (Wolff et al., 2012; Nagelli et al., 2022). The AT is encased in the paratenon, which is rich in blood vessels, rather than a tendon sheath (Lohrer et al., 2008). A synovial bursa is present between the AT and the skin and calcaneus, which is essential in reducing friction and ensuring relative sliding between tissues (Campanelli et al., 2011). The tendon is also surrounded by the epitenon, a well-defined layer of connective tissue that extends inward to become the endotenon surrounding the fiber bundle (Sharma and Maffulli, 2006). Tendon composition varies slightly in different parts of the body, which may be attributed to the physiological environment (Walia and Huang, 2019). In general, tendons in the human body have similar tissue structures, consisting mainly of collagen and scattered cells (Waggett et al., 1998). The AT mainly contains collagen I (Col I) and collagen III (Col III) (Fukuta et al., 1998; Im and Kim, 2020). Three peptide chains form into soluble tropocollagen after enzymatic excision of the terminal peptide. Tropocollagen forms collagen fibrils through lateral connections. Collagen fibril then aggregates to form collagen fiber. Many collagen fibers gather into bundles, creating primary, secondary, and tertiary fiber bundles (Andarawis-Puri et al., 2015). In addition to collagen fibers, the ECM of the AT includes elastin, fibronectin, decorin, biglycan, and fibromodulin (Walia and Huang, 2019). Tissue-resident tendon cells play a vital role in the composition of AT, exhibiting significant heterogeneity. In mature tendons, tenocytes and tenoblasts make up approximately 90%–95% of the tendon cell population, with tenoblasts being predominant in young tendons. Tissue-resident tendon stem/progenitor cells (TSPCs), which share similar characteristics with MSCs, constitute 1%–4% of the tendon resident cells. Telocytes, found in the equine inter-fascicular tendon matrix near blood vessels, express stem cell markers and are localized near the tendon stem cell niche. Additionally, new populations of tenocytes, including tendon fibroblasts 1 and 2, and junctional fibroblasts, have been recently reported. The remaining cells consist of chondrocytes, synovial cells, capillary endothelial cells, and smooth muscle cells (Sharma and Maffulli, 2005). The heterogeneity of tenocytes, along with the interaction between different cell types in tendon tissue, is crucial for the maintenance and repair of tendon tissue during homeostasis and tendinopathies (Russo et al., 2022). In normal tendons, the collagen fibers are continuous, parallel, and closely arranged (Angrisani et al., 2022). Tenocytes are arranged following the direction of the fibers, extending a few thin-winged pseudopodia embedded in fiber bundles (Millar et al., 2021). The AT exhibits a hierarchical structure (Figure 1) composed of fascicles, fibers, and fibrils. These components are encompassed by a delicate collagen membrane known as endotenon, which houses lymphatics, blood vessels, and nerves. Within this intricate arrangement, certain cells, including tenocytes, fibroblasts, and TSPCs, align themselves along the direction of the fibers.
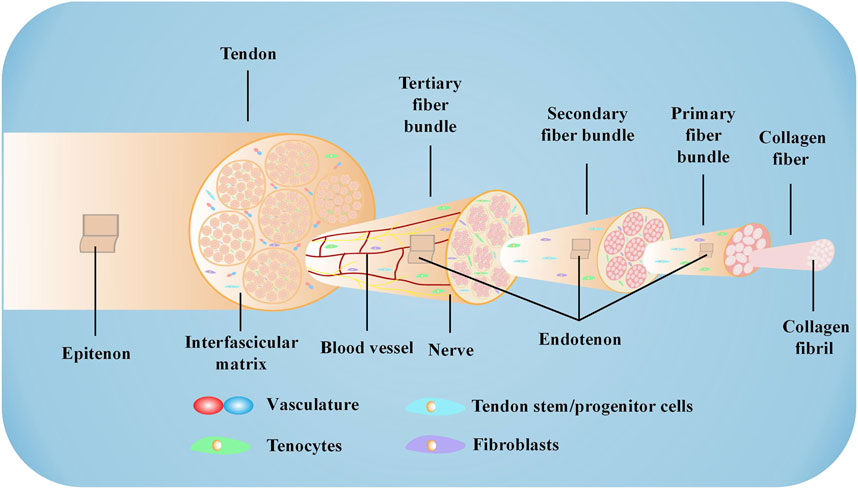
FIGURE 1. Microstructure of the AT The AT comprises collagen fibers, cells, and a small number of blood vessels. Specific proteases hydrolyze the terminal peptide of procollagen formed by three peptide chains to form tropocollagen. Triple-stranded tropocollagen forms collagen fibril through lateral connections. Collagen fibril further aggregates to form collagen fiber. Collagen fiber converges into a primary fiber bundle surrounded by the endotendon to create a secondary fiber bundle. The secondary fiber bundle also assembles into a tertiary fiber bundle, which is surrounded by the epitenon to constitute the tendon.
3 Pathophysiology of AT injury
AT injury can lead to loss of tissue integrity and dysfunction, causing pain, swelling, and stiffness (Martin et al., 2018). In tendinopathy, tenocytes become more extended, thinner, smaller in size, and produce less ECM (Millar et al., 2021), and the collagen fibers are broken and arranged irregularly (Maffulli et al., 2008). Simultaneously, inflammatory cells infiltrate the tissue, triggering an inflammatory response (Ye et al., 2023). During tendon injury, the activation of inflammatory mechanisms and the innate immune system is evident within the tendon matrix microenvironment and probably attributed to the dysregulated homeostasis. The resident tenocytes secrete cytokines and chemokines in both autocrine or paracrine manner and can be activated towards an inflammatory phenotype, they influence on the reactions that submerge after tendon damage by communicating with immune-sensing cells, attracting, and activating the infiltrating immune cells into the injury site, or modulating the secreted implicated cytokines (Russo et al., 2022). In addition, supporting tissues including vascular and nervous play an important in modulating the inflammatory response of the injured tissue and in tissue regeneration. So, new nerves are frequently found in diseased tendons (Millar et al., 2021). A normal AT is not rich in blood vessels; however, in AT injury, there are apparent vascular proliferations (Chen et al., 2011). Furthermore, the increased density of Col III in the ECM leads to an abnormal Col I/Col III ratio, thus severely affecting the mechanical properties of the AT (Millar et al., 2017).
4 Etiology of AT injury
The AT is the most frequently ruptured tendon in the human body, accounting for 20% of all large tendon ruptures. AT injury is classified into closed and open injuries. Closed injury, such as rupture, tendinitis, and degeneration, is mainly caused by strain and often occurs in the middle, insertion, and peritendinous parts of the AT. Aseptic inflammation accompanied by symptoms such as redness, pain, and weakness may present at the local injured site. Open injury is mainly caused by direct violence, such as chopping and slashing with sharp objects. Other factors that can influence AT injury include age, sex, use of fluoroquinolone antibiotics, and uneven force on the AT during exercise (Seeger et al., 2006; Slane et al., 2015; Ganestam et al., 2016; Tramer et al., 2021). Furthermore, with improved quality of life, obesity has gradually become an essential factor affecting AT injury.
5 GFs in AT injury
GFs are a class of cytokines secreted by cells and participate in various biological activities by binding to specific, high-affinity receptors to maintain and regulate the growth and metabolism of the body. A study retrieved 2,332 publications on early tendon development, draw a comparative map of molecules that control tenogenesis, and found several hub genes that plays an important role in tendon development, including TGF-βs, BMPs, FGFs, IGFs (Peserico et al., 2023). These GFs related to tenogenesis paths belonging to macro-categories such as (1) growth, differentiation and survival, (2) morphogenesis and cell motility, (3) nervous system, (4) and endocrine system. GFs such as FGF-4 and TGF-β2, which are critical during embryonic development, influence tendon development. FGF-4 regulates the expression of Scleraxis (Scx), an early marker for tendon cell fate (Glass et al., 2014). As the upstream molecules of Scx, TGF-β and FGF coordinately induce the development of axial and limb tendon progenitors via Scx action. TGF-β plays a central role in tendon development, and TGFB receptors are expressed in tendon progenitor cells, the genetic deletion of TGFBR2 or TGFB2 results in failure of tendon development (Peserico et al., 2023). TGF-β1, -β2, and -β3 have distinct spatiotemporal developmental protein localization patterns in the developing tendon and may probably have independent roles in tendon development (Kuo et al., 2008). TGF-β2 was noted to be tenogenic for tendon progenitor cells at all developmental stages in vitro (Titan et al., 2019). BMP-12 guides the expression of Scx, tenomodulin (Tnmd), Col 1, and tenascin-C (TNC) in tendon progenitor cells in vitro (Liu et al., 2015b). VEGF signaling is vital during tendon development, specifically within developing tendons under traction, and gliding tendons maintain an avascular zone even from the fetal period (Petersen et al., 2002). GFs such as TGF-β, BMPs, PDGF, VEGFs, FGFs, IGF, and PRP have been studied extensively in various tendon healing models (Table Supplementary S1). These GFs play a role in all three stages of AT healing, which include the inflammatory, proliferative, and remodeling phases (Figure 2). Briefly, In the first phase, the focus is on the inflammatory activities, which involve the infiltration of inflammatory cells and fibroblasts from outside the injury site, and various GFs are released during this phase, including VEGF, PDGF, bFGF, TGF-β, and IGF. The proliferative phase is a crucial stage in the healing process, characterized by the proliferation of fibroblasts, synthesis of ECM, activation and differentiation of TSPCs, and extensive growth of blood vessels and nerves. Various growth factors, including VEGF, BMPs, bFGF, TGF-β, and IGF, play important roles in this phase. In the final remodeling phase, the newly synthesized collagen undergoes rearrangement to form mature tissue. During this phase, GFs such as BMPs, bFGF, TGF-β, and PDGF play a crucial role in guiding and regulating the remodeling process.
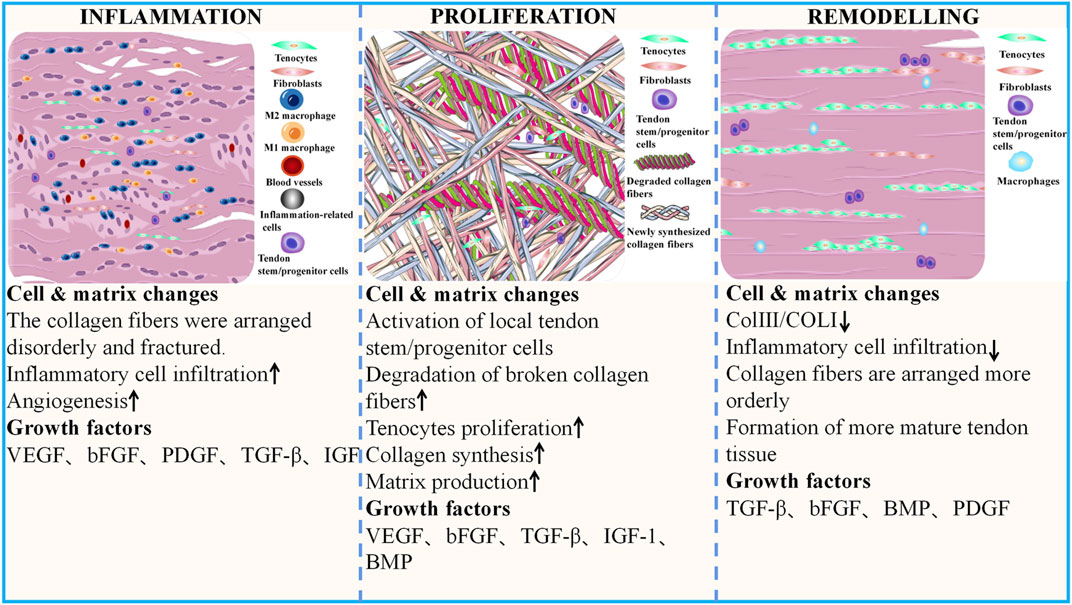
FIGURE 2. Cell and matrix changes during different phases of AT healing and the GFs involved The recovery process following AT injury is triphasic: acute inflammation, proliferation, and remodeling phases. During the inflammatory phase, collagen fibers are disorganized and broken. There is a considerable appearance of inflammatory cells in the AT and a significant increase in neovascularization. With the activation and proliferation of in situ cells, tenocytes and fibroblasts increase significantly, and the recovery phase gradually transitions to the proliferation phase. Here, the content of ECM fractions also gradually increases. The damaged tissue begins to degrade, and new tissues fill the defect site. As rehabilitation progresses, the number of inflammatory cells decreases sharply, the arrangement of collagen fibers becomes more regular, and Col I gradually replace Col III. The biomechanical properties of the AT tissue significantly improve. AT, Achilles tendon; GFs, growth factors.
5.1 TGF-β and AT injury
TGF-β isoforms (TGF-β1, TGF-β2, and TGF-β3) are the prototypical members of the TGF-β superfamily. They are responsible for many cellular activities, including proliferation, differentiation, migration, adhesion, ECM synthesis, immune response, and cell death (Aschner and Downey, 2016; Acharya et al., 2022). The healing response in natural tendon injury is triphasic: inflammation, proliferation, and remodeling phases (Ruiz-Alonso et al., 2021). In the acute inflammation phase, which is the first phase that occurs after tendon injury, proinflammatory factors and inflammatory cell infiltration resist harmful stimuli, mediating the inflammatory response. In contrast, anti-inflammatory and proinflammatory factors act together to control the progression of inflammation (D'Addona et al., 2017). TGF-β expresses at all stages of tendon healing, particularly during the inflammatory and proliferative phases. TGF-β maintains immune homeostasis in several tissues, and the lack of TGF-β exacerbates inflammation, leading to tissue damage and cellular transformation. Its activity decreases with the relief of inflammatory response (Tauriello et al., 2022). Specific genes are selectively expressed during different tendon formation and repair stages to promote normal tendon development (Perucca Orfei et al., 2019). TGF-β isoforms have other effects during wound healing and scarring. While TGF-β3 is a significant inducer of Scx, which is expressed early in tendon development initiating tendon differentiation, it is also an inhibitor of collagen fiber maturation during tenogenic differentiation, especially in the late stage (Perucca Orfei et al., 2019; Zhou et al., 2021a). TGF-β3 alone or combined with other GFs acts as an essential tenogenic inducer in many cell types, such as AD-MSCs (Shojaee et al., 2022), human tenocytes (Tsiapalis et al., 2021), BMSCs (Bottagisio et al., 2017), embryo-derived stem cells (ESCs) (Barsby et al., 2014), tonsil-derived MSCs (TMSCs) (Wee et al., 2022). Contrary to the effects of TGF-β3, TGF-β1 inhibits the expression of Scx, promotes the expression of Tnmd, which is a marker of mature tendon cells, and accelerates tendon development (Hyun et al., 2017).
Proper application of TGF-β1 at the early stage of injury can reduce inflammation and accelerate wound healing (Sun et al., 2021). Collagen content and cross-linking are significant determinants of tendon structural integrity and function. Col Ⅰ and Col Ⅲ are the main contents of the AT. TGF-β1 may participate in matrix remodeling by modulating collagen synthesis (Sun et al., 2015). AT injury treated with TGF-β1-bone marrow mesenchymal stem cells (BMMSCs) healed more rapidly and completely. TGF-β1 accelerates collagen protein synthesis, cross-link formation, and matrix remodeling in tendon healing, thus enhancing mechanical strength (Hou et al., 2009b). Low-magnitude, low-frequency 10 Hz vertical vibration training enhances TGF-β1 expression, subsequently increasing the expression of Tnmd and synthesis of Col Ⅰ and increasing AT stiffness in rats. This treatment improves tendon properties and minimizes the risk of ligament/tendon reinjury during rehabilitation (Chen et al., 2018b). A study demonstrated that in patients with AT rupture, TGF-β1 and VEGF 3 expressions significantly increased 3 months after treatment and significantly decreased 6 months after surgery. TGF-β1 and VEGF expressions decreased after surgery with improvement in the efficacy; therefore, TGF-β1 and VEGF can be considered as observational indexes and predictors of clinical efficacy in patients with AT rupture before and after surgery (Cui et al., 2019). Collagen sponges loaded with GFs, such as basic FGF (bFGF), BMP-12, and TGF-β1, implanted in a rat with transected AT showed a rapid increase in mechanical strength and faster tendon remodeling (Majewski et al., 2018). The TGF-β1 and TGF-β3 changes in the coursing of AT healing in a rat model was higher than that in the sham operation group at all-time points (2w, 4w, 6 weeks after injury), reaching its peak at 2 weeks, decreased at 4 weeks, and significantly reduced at 6 weeks after the operation. Therefore, the expression levels of these two factors may be used as indicators to determine the degree of recovery following an AT injury (Wu et al., 2021). Rat muscle biopsies transduced with recombinant adenovirus-TGF-β1 were transplanted to surgically transected AT of recipient animals, which accelerated the healing, and the repair tissue gained nearly normal histological appearance at 2 weeks post operation (Majewski et al., 2012). In addition, the treatment notably alleviated the inflammatory responses in vivo via downregulation of IL-1β, TNF-α, and IL-6 and promoted the tube formation in tissues through upregulating VEGF, bFGF, TGF-β1, and CD31 (Gong et al., 2022).
Although TGF-β fosters recovery after AT injury, its overexpression can result in abnormal deposition of ECM proteins, leading to tissue fibrosis, induced excessive scar hyperplasia in the injured area, altered tissue structure, and decreased anatomical function (Schroer and Merryman, 2015). In addition, elevated active TGF-β promotes ectopic bone formation. Huang et al. demonstrated that injured AT of rats transfected with TGF-β short hairpin RNA using ultrasound-targeted microbubble destruction technique attenuated AT adhesions and scar formation, and the decreased TGF-β helped to alleviate tendon adhesion by reducing the number of inflammatory cells (Hung et al., 2022). Although attenuating the effect of TGF-β1 can diminish the grading of adhesions, the ultimate strength of repaired tendons was significantly impaired (Zhou et al., 2013).
In contrast, TGF-β3 is well known for its antifibrotic effects. Jiang reported that adding TGF-β3 to tenocytes significantly downregulated the expression of Smad3 and upregulated the expression of Smad7 (Jiang et al., 2016b), minimizing extrinsic scarring via antagonizing the TGF-β1/Smad3 signaling pathway (Jiang et al., 2016a; Deng et al., 2017). This result provides a new therapeutic approach for reducing scar tissue and promoting tendon healing. Cetik et al. used PLGA-b-PEG NPs [poly (lactic-co-glycolic acid)-b-poly (ethylene glycol) nanoparticles (NPs)] loaded TGF-β3 as a sustained-release system to treat rat models with unilateral AT transection and demonstrated that TGF-β3 may positively affect AT midsubstance repair, especially during the remodeling phase, and the NP form will achieve better outcomes (Cetik et al., 2022).
TGF-β2 was the only isoform detected in tenocytes within the fibrillar matrix, and its expression was significantly higher in patient tendons compared to normal cadaver tendons. This elevated presence of TGF-β2 in pathological AT suggests its potential role in regulating cellular activity during the advancement of this disease (Fenwick et al., 2001). TGF-β2 and TGF-β3 expressions fluctuated during bone formation. TGF-β2 was significantly upregulated during HO formation in animal model for HO induced by Achilles tenotomy in rats (Lin et al., 2010). The zinc finger transcription factor (EGR1) plays a role in the production of type I collagen in postnatal tendons. In a rat model of AT injury, the application of EGR1-producing MSCs resulted in an increase in the formation of tendon-like tissues. This effect is partially mediated by TGF-β2 (Guerquin et al., 2013). Mohawk (Mkx) is expressed in developing tendons and is an important regulator of tenogenic differentiation, its expression level was dramatically lower in human tendinopathy tissue and it is activated at specific stages of tendon development. Mkx dramatically upregulated Scx through binding to the TGF-β2 promoter. Mkx activates Scx and tendon ECM genes expression partially through direct activation of TGF-β2 (Liu et al., 2015a).
In conclusion, the TGF-β isoforms have different effects during AT healing and scarring (Table 1), even if the same subtype has a double-sided effect. However, further studies regarding the effective ways to use these factors to promote AT healing and prevent scar formation or their role as indicators of the degree of recovery following injury are warranted.
5.2 BMPs and AT injury
BMPs constitute the largest subdivision of the TGF-β family with nearly 30 different proteins (Lowery and Rosen, 2018). However, significant differences among BMPs regarding their effects on AT repair exist. BMP-1 is significantly upregulated at each time point of traumatic heterotopic ossification (HO). On the contrary, BMP-4 is significantly downregulated in the early stages of traumatic HO. This indicates that BMP-1 plays an essential role in the formation of traumatic HO, whereas BMP-4 plays a protective role in the early stage of HO (Yu et al., 2021a). The BMP-2/4/7 expression in Sprague-Dawley (SD) rats AT increased with aging, especially BMP-2, and this expression remained consistent with the increasing trend of HO and osteogenesis-related gene expression in the tissue. BMP-4/7 might play an essential role in forming ectopic ossification at the early stage and a weaker function at the late phase (Dai et al., 2020). BMP-2 negatively regulates the expression of Prospero homeobox protein 1, thereby inhibiting the formation of lymphatic endothelial cells, aggravating the inflammatory response, and promoting the formation of HO (Dunworth et al., 2014).
BMP-12/13/14 can induce tendon differentiation in vivo. BMP-12/14 induces tendon differentiation of adipose tissue-derived mesenchymal stromal/stem cells (ADMSCs), and BMP-12 induces tendon differentiation of ADMSCs via the Smad1/5/8 pathway in a dose- and time-dependent manner (Shen et al., 2013) (Figure 3). BMP-14 may induce the tenogenic differentiation of BMMSCs via the Sirt1-JNK/Smad1-PPARγ signaling pathway (Wang et al., 2018a) (Figure 3). Adenovirus-mediated gene therapy of BMP-14 expedited tendon healing in SD rats model with transected AT; BMP-14 significantly increased the Col II expression and enhanced the mechanical properties of the AT (Wang et al., 2018a). In another study, BMP-12 was tethered on a book-shaped decellularized tendon matrix and implanted into a rat AT defect model, which proved more beneficial than autograft for AT healing (Xiao et al., 2021). Exogenous BMP-7 in a rat AT has been shown to enhance fibro-chondrocyte differentiation of tendon cells, induce ectopic cartilage formation, promote meniscus regeneration, and prevent cartilage degeneration (Ozeki et al., 2013). Biopsies of autologous skeletal muscle transduced with Ad. BMP-12 and surgically implanted around experimentally transected AT in a rat model improved and accelerated tendon healing and influenced early tissue regeneration, leading to quicker recovery and improved biomechanical properties of the AT (Majewski et al., 2008). Lee et al. showed that BMMSCs implanted into an animal model after loading onto a three-dimensional collagen sponge scaffold and induction by BMP-12 promoted tendon-like tissue formation (Lee et al., 2011). In addition, the number of tenocytes increased, which were arranged regularly along the tension axis. Engineered tendon matrix containing BMMSCs and BMP-13 implanted in an AT injury model significantly improved the fiber alignment, increased the tensile modulus, and increased the ultimate load of the AT (Jiang et al., 2016a). Park et al. showed that 100 ng/mL of BMP-14 significantly promoted the proliferation of ADMSCs and expression of the tendon marker genes Scx, Tnmd, and TNC (Park et al., 2010). Although BMPs combined with stem cells can promote AT healing, differences in the therapeutic effects of different sources of stem cells on AT injury exist. BMMSCs, ADMSCs, and synovial membrane-derived MSCs (SMMSCs) induced with BMP-12 all showed similar fibroblast morphology and expressed typical tendon marker genes, such as Scx, TNC, and Tnmd (Dai et al., 2015). Nonetheless, BMMSCs showed superior tendon differentiation ability, followed by SMMSCs, while ADMSCs showed the least tendon differentiation ability. However, compared with BMMSCs, BMP-14-induced muscle-derived MSCs were more effective in promoting tendon healing (Ozasa et al., 2014). The advantages/disadvantages of BMPs are presented in Table 2.
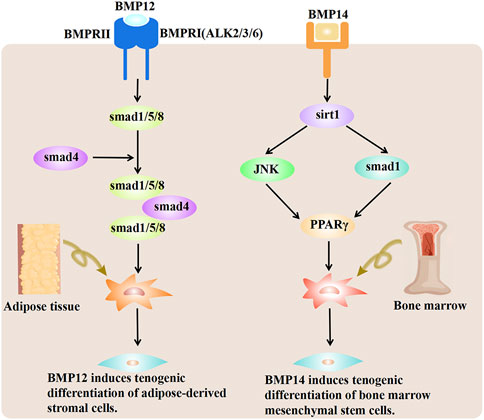
FIGURE 3. BMP-12 and BMP-14 mechanisms promoting tenogenic differentiation of MSCs. BMP-12 induces tenogenic differentiation of ADMSCs via the Smad1/5/8 pathway. BMP-12 triggers the phosphorylation of Smad1/5/8 in ADMSCs. The effect is likely conveyed by type I receptors ALK2/3/6. BMP-14 induces the deacetylation of PPARγ through activation of the Sirt1-Smad1/JNK pathway. Deacetylated PPARγ further promotes the tendon differentiation of BMMSCs. BMP-12, bone morphogenetic protein 12; BMP-14, bone morphogenetic protein 14; MSCs, mesenchymal stem cells; ADMSCs, adipose tissue-derived mesenchymal/stromal stem cells; BMMSCs, bone marrow mesenchymal stem cells.
In summary, BMP isoforms exhibit different expression patterns in various stages of AT repair and play various roles in the AT healing process. BMP-2, -12, −13, and −14 are potent inducers of tenogenic differentiation in different MSCs, promoting AT recovery. BMP-1, -2, -7, and -14 induce HO, whereas BMP-4 inhibits HO. Therefore, to enhance AT healing and decrease HO, the effective use of BMP isoforms is important.
5.3 IGFs and AT injury
The IGF family plays a critical role in normal growth and development. It comprises (i) ligands (IGF-I, IGF-II, and insulin), (ii) six well-characterized high affinity binding proteins (IGF binding protein [IGFBP]-1–6), (iii) IGFBP proteases, and (iv) cell surface receptors that mediate the biological functions of IGFs (IGF-1 receptor [IGF-1R], IGF-2R, and insulin receptor substrates) (Stuard et al., 2020). IGF-1 is critical for tenocyte migration, division, matrix expression, collagen synthesis, phenotypic maintenance, and tendon repair after injury (Muller et al., 2015). A study showed that after regular training, the cross-sectional area of tendons increased significantly in men than in women, which may be related to IGF-1 secretion and sex; the cumulative effect of the amount of IGF-1 secretion makes men more superior to women due to motor-adapted hypertrophy of the AT (Astill et al., 2017). IGF-1 accelerates tendon recovery and promotes tendon-derived stem cell (TDSC) phenotype proliferation and maintenance (Holladay et al., 2016). IGF-1 combined with TGF-β1 induces ADMSCs to differentiate into stable tenocytes (Schneider et al., 2011). IGF-I injections stimulated collagen synthesis in Ehlers-Danlos patients (Nielsen et al., 2014). Tang et al. showed that IGF-1 significantly improved the mechanical properties of the AT, which may be attributed to the promotion of collagen synthesis by IGF-1 (Tang et al., 2015). IGF-1 signaling is required for proper tendon growth in response to mechanical loading through a coordinated induction of collagen synthesis and cell proliferation. Disser et al. demonstrated that IGF-I induces tenocyte proliferation via the RAS/RAF/MEK/ERK signal transduction pathway and stimulates ECM protein synthesis via the PI3K/Akt signaling pathway (Hortensius and Harley, 2013; Disser et al., 2019). After AT injury, resident cells, such as macrophages and mastocytes, recruit immune cells from circulation to build a protective system. Tenocytes participate in the inflammatory response and tissue remodeling through autocrine or paracrine secretion. While low-level inflammation clears damaged tissues and promotes tissue recovery, a too-strong inflammatory response can lead to tissue fibrosis, resulting in poor remodeling and seriously affecting the healing of the AT. IGF-1 may alleviate the functional impairment after AT injury by reducing the inflammatory response (Kurtz et al., 1999). As there are few studies on the effects of IGFs on AT healing, it would be interesting to analyze whether different concentrations of IGF-1 can generate different results.
5.4 FGFs and AT injury
FGFs are broad-spectrum mitogens and play a critical role in development, metabolism, and tissue homeostasis by regulating a wide range of cellular functions, including migration, proliferation, differentiation, and survival. FGFs exert pleiotropic effects by binding to and activating high-affinity tyrosine kinase receptors (FGFR) (Xie et al., 2020). Fibrin clots and vitamin C produce a more robust tendon structure and better quality tendon healing in the surgical treatment of AT ruptures, which may be attributed to the proper stimulation of FGFs secretion during the first phase of AT injury (Celik et al., 2021).
FGF2, also known as bFGF, promotes cell proliferation and migration and accelerates wound healing (Zhang et al., 2018a). bFGF stimulates collagen production, tendon development, tenocyte proliferation, and tendon tissue differentiation and promotes the expression of a series of GF genes in AT repair. bFGF stimulates ECM secretion and slows down ECM degradation by upregulating the expression of tissue inhibitors of metalloproteinase (MMP) (Tang et al., 2016). A study demonstrated that human TDSCs transfected with a lentivirus carrying the FGF2 gene promoted the expression of Col 3A1 and Scx in vitro (Guo et al., 2020). In addition, the rat AT defect model transplanted with FGF2-hTDSCs demonstrated more ECM production and a more orderly arrangement of collagen fibers. Hyun et al. found that FGF2 dose-dependently increased Scx and Tnmd expressions, which are markers of tendinogenesis, in human periodontal ligament stem cells. Simultaneously, FGF2 counteracted the inhibition of early tendinogenic marker expression by TGF-β1 and attenuated the ossification effect of BMP-2/4 (Hyun et al., 2017). The lasting time of GFs used in tendon injury is key in determining their effects. Tissue-engineered scaffolds can sustainably release GFs to promote cell infiltration and tissue formation. bFGF-loaded multiscale fibrous scaffolds (polycaprolactone [PCL]/Col/bFGF) with appropriate porosity provide physical and biochemical cues to facilitate tenocyte proliferation and differentiation. In vivo study of cell-seeded scaffold after dynamic stimulation in the AT defect model showed tendon tissue regeneration, with aligned collagen morphology within 12 weeks of implantation, and inhibition of scar formation, thus playing an essential role in maintaining the tendon’s ultimate tensile strength (UTS) (Jayasree et al., 2019). Chen et al. demonstrated that FGF gene expression was associated with improved patient-reported outcomes and suggested that FGF expression in surgical biopsies could potentially be used as a predictor for healing (Chen et al., 2021).
HO, an extraskeletal bone formation, is a common complication after trauma; however, its underlying mechanisms remain unclear. The degree of ossification depends on the level of damage/inflammation. Zhang et al. revealed that conditional knockout FGFR3 in Col2+ cells promote acquired HO development. Knockdown of FGFR3 in lymphatic endothelial cells inhibits local lymphatic formation in a BMPR1a-pSmad1/5-dependent manner, exacerbating inflammatory levels in the repaired tendon, leading to HO. Therefore, activating FGFR3 in lymphatic endothelial cells may be a therapeutic strategy to inhibit HO formation by increasing local lymphangiogenesis (Zhang et al., 2021). However, further studies evaluating its plausibility and the role of FGF in AT healing are warranted.
5.5 PDGFs and AT injury
PDGF, a serum-derived GF, is essential for the maturation of multiple cells. It includes four isoforms: PDGFA, PDGFB, PDGFC, and PDGFD. These isoforms dimerize to form homodimers PDGF-AA, PDGF-BB, PDGF-CC, and PDGF-DD and heterodimers PDGF-AB (Paolini et al., 2022). PDGF receptor (PDGFR) is a transmembrane glycoprotein belonging to class III receptor tyrosine kinases, including two subtypes, PDGFRα and PDGFRβ, which can polymerize with each other to form three dimers: PDGFRαα, PDGFRαβ, and PDGFRββ (Guerit et al., 2021). Five distinct PDGFs can bind to one or both PDGFRs in a dimeric state contributing to the normal development of different tissues. PDGF-AA, which only activates PDGFRα, drives both tenogenesis and fibrosis. Tubulin polymerization-promoting protein family member 3-expressing (Tppp3+) cell population can generate new tenocytes and self-renew upon injury. PDGF-AA induces new tenocyte production while inactivating PDGFRα in Tppp3+ cells block tendon regeneration. However, PDGF-AA can also act on fibro-adipogenic progenitors and lead to tendon fibrotic scar formation (Harvey et al., 2019). Therefore, studies regarding the use of PDGF-AA to accelerate AT recovery while reducing scar formation are worth conducting.
PDGF-BB is considered the universal isoform of PDGF and approved by the Food and Drug Administration because of its ability to bind to all three PDGF receptors and trigger different signaling pathways (Evrova and Buschmann, 2017). PDGF-BB expresses predominantly during tendon healing. It induces tissue repair through its generic chemotactic, mitogenic, and angiogenic properties and synergistic actions with other GFs. The supplementation of tenocyte culture with PDGF-BB increased tenocyte proliferation in a rabbit AT model, leading to the upregulation of fibronectin, biglycan, and TNC in the cultured tenocytes (Evrova et al., 2020). Chen et al. demonstrated that recombinant human PDGF-BB promoted hADMSC proliferation via the miR-363/PI3K/Akt pathway. PDGF-BB and hADMSC can improve the biomechanical indices of Achilles tendinitis separately, such as stiffness, stress, and maximum load-to-failure, and upregulate Col Ⅰ, Scx, and TNC expressions. PDGF-BB and hADMSC can also enhance these effects further (Chen et al., 2018b). The combined application of two or more GFs can overcome the defects of a single GF and even have a cumulative effect, thereby promoting healing. PDGF-BB combined with growth differentiation factor-6 (GDF-6) can stimulate the tenogenic differentiation of ADMSCs, with results better than that of a single GF (Younesi Soltani et al., 2022). ADMSCs cultured with GDF5/PDGF before implantation can promote tendon repair by improving cellular proliferation, differentiation, tenogenesis, vascular infiltration, proteinogenesis gene SOX9 expression, and tissue remodeling (Fitzgerald et al., 2021).
Degradable biological scaffolds are often used to deliver GFs continuously at the site of injury for long-term effects. Evrova et al. found that PDGF-BB loaded with polyester urethane scaffold significantly increased the expression of α-smooth muscle actin, promoted the proliferation of tenocytes, and improved the UTS of the tendons, with no significant local scar hyperplasia (Evrova et al., 2020). Kang et al. suggested that the long-term local PDGF delivery by porous microspheres modified with heparin has a great potential to enhance tendon healing in a rat model of Achilles tendinitis by suppressing inflammation responses (Kang et al., 2019). PDGF-AA-modified poly (lactide-co-glycolide) acid (PLGA) electrospun fibers (PLGA-PDGF-AA) effectively promoted tendon healing by stimulating collagen synthesis, deposition, and mechanical strength of tendon tissue (Wang et al., 2022). The sustained delivery of PDGF-BB via an electrospun DegraPol tube to the wound site in a full-transection rabbit AT model accelerated tendon wound healing by causing a more uniform cell distribution, with higher proteoglycan content and less fibrotic tissue (Meier Burgisser et al., 2020). Liu et al. constructed a multilayer composite membrane and a GF sustained-release system conforming to the tendon-healing cycle by coating two surfaces of freeze-dried amnions with PCL nanofibers (Liu et al., 2020). In the study, the rabbit tendon injury model treated with the composite membrane effectively isolated the exogenous adhesion tissue and promoted endogenous tendon healing by slowly releasing TGF-β1, bFGF, VEGF, and PDGF and regulating the ERK1/2 and SMAD2/3 pathways.
5.6 VEGFs and AT injury
The VEGF family includes VEGF-A, VEGF-B, VEGF-C, VEGF-D, VEGF-E, placental GF, and endocrine gland-derived VEGF. VEGF promotes endothelial cell mitosis, improves vascular permeability, and stimulates cell migration. In addition, VEGFs binding to VEGF receptors (VEGFR) promote tyrosine kinase enzyme activation and several intracellular signaling pathways (Melincovici et al., 2018).
Although tendons are relatively hypovascular, they become hypervascular during injury and degeneration, and this may be attributed to the formation of new blood vessels in the injured tissue. Neovascularization facilitates healing by controlling the immune response, delivering oxygen and nutrients, removing waste products, and transporting regulatory factors. Vascular ingrowth is necessary for tendon healing; prolonged hypervascularization following tendon injury may not be beneficial. VEGF plays a vital role in angiogenesis. Cui et al. found that during the inflammatory phase of tendon healing, M2 macrophages can release VEGF and promote endothelial cell sprouting, contributing to angiogenesis (Cui et al., 2022). As a potent regulator of angiogenesis, VEGF dose-dependently promotes tenocyte proliferation, enhances the expression of tendon-related genes (Kraus et al., 2018), facilitates the formation of microvessels, and improves the UTS of the AT when used appropriately. A study has shown that reducing VEGF- signaling leads to tendon healing (Tempfer et al., 2018). VEGFR1, VEGFR2, and VEGFR3 expressed in murine and human tendon cells in vivo and VEGFR1, VEGFR3, and VEGF-D expressed in tenocytes respond to inflammatory stimuli and injury both in vitro and in vivo and can affect tenocyte proliferation, stromal disruption, cell migration, and degenerative changes in the AT (Tempfer et al., 2022). After a tendon injury, tenocytes secrete hypoxia-inducible factor 1 (HIF-1) in response to mechanical overload and hypoxia. Simultaneously, HIF-1 promotes VEGF expression, resulting in increased vascularization and accelerated tendon healing (Tempfer and Traweger, 2015). A study showed that the serum TGF-β1 and VEGF expressions in patients with AT rupture significantly increased 3 months and significantly decreased 6 months after surgery, as compared to results before treatment. Therefore, TGF-β1 and VEGF may be considered observational indexes and predictors for clinical efficacy in patients with AT rupture (Cui et al., 2019). A study indicated that compared with normal controls, VEGF and corresponding protein levels significantly downregulated in the healing AT of type 2 diabetic rats 2 weeks post injury; the vascular remodeling ability decreased, and the recovery was slow (Ahmed et al., 2014). Therefore, upregulation of VEGF levels may be a strategy to improve tendon repair in diabetic rats during the early stages of AT injury.
VEGF-111 is a biologically active and proteolysis-resistant splice variant of VEGF-A. Local injection of VEGF-111 significantly improved the UTS of the healing ATs 15 and 30 days after surgery and the mechanical stress in the late phase (30 days) of the repair compared to the control group (Kraus et al., 2014). Many studies linked heparin injection to poor outcomes in rat AT repair due to its role in the inhibition of thrombin activity and anticoagulation effect. However, suture loading with heparin has achieved good results. Poly-l-lactic acid/polyamide sutures loaded with heparin can reduce inflammation and accelerate the healing and regeneration of the AT by promoting VEGF secretion (Ye et al., 2018).
Vascularization in healthy tendons is low; however, the production of new vessels after an injury is not necessarily a sign of functional tissue repair. Instead, it may be associated with degeneration. Some studies have demonstrated that antiangiogenic treatment in tendon models may cause improvements in tissue organization and mechanical properties. Bevacizumab, an antiangiogenic drug that is a recombinant humanized monoclonal antibody blocking VEGF-A signaling, can alter tendon vascularity and dose-dependently improve tendon healing (Riggin et al., 2019). In addition, the drug can significantly improve tendon healing in a rat model by reducing angiogenesis, cross-sectional area, stiffness, and Young’s modulus, thereby improving matrix organization and increasing maximum load and stress. The gait pattern of the rat model also improved (Tempfer et al., 2018). ADMSC transplantation into injury sites during tendon repair in a mice model significantly increased VEGF and CD31 positive vessels, repairing the tendinopathy and preventing HO (Kokubu et al., 2020). VEGF can also cause scarring by stimulating the formation of a hypofunctional vascular connective tissue at the injury site, disrupting the typical molecular structure. Using VEGF to promote recovery from AT injury can be complicated. Hence, further studies are needed to assess whether VEGF is appropriate for tendon healing.
5.7 PRPs and AT injury
PRP is an autologous blood product containing high concentrations of GFs and cytokines, such as PDGF, IGF, VEGF, and TGF. It alters the biological processes in many pathogeneses, promotes injured tissue regeneration, accelerates anabolism, and improves healing by stimulating cell proliferation, migration, and angiogenesis (Boesen et al., 2017; Bennell et al., 2021; Park et al., 2021; Xu et al., 2021). Depending on their leukocyte and fibrin content, PRPs can be classified into pure PRP (P-PRP), leukocyte-rich PRP (LR-PRP), pure platelet-rich fibrin (P-PRF), and leucocyte- and platelet-rich fibrin (L-PRF) (Dohan Ehrenfest et al., 2014). Recent clinical and experimental studies have demonstrated the successful application of PRP in treating chronic tendinopathy and acute tendon injury. PRP can promote tissue recovery in the early phase of tendon healing by stimulating tendon cell proliferation and collagen production while inhibiting apoptosis and macrophage infiltration (Yu et al., 2021b). Zou et al. injected PRP into the paratenon sheath and around the ruptured tissue of patients with AT tendon rupture after surgery, and the results demonstrated that PRP improved the short- and mid-term functional outcomes after surgical repair (Zou et al., 2016). Platelets are a major high mobility group box1 (HMGB1) source. Platelets HMGB1 within PRP play a vital role in tendon healing by decreasing inflammation, increasing local HMGB1 levels, and recruiting stem cells to the wound area, suggesting that the efficacy of PRP treatment for tendon injuries in clinics may depend on platelets (Zhang et al., 2021).
Compared to LR-PRP, leukocyte-poor PRP (LP-PRP) is a better choice for treating tendinopathies. The high amounts of proinflammatory factors and catabolic enzymes in leukocytes exacerbate the inflammatory response after injury. LP-PRP may promote AT healing by modulating the balance of MMPs and tissue inhibitors of metalloproteinases and reducing proinflammatory catabolic cytokines release. In addition, the leukocytes present in LR-PRP may provoke further chronic inflammation (Yan et al., 2017). PRP combined with a variety of therapies can enhance AT healing. MSCs combined with PRP significantly increased the inflammatory cell density, mean maximum breaking force, and tendon strength force (Uyar et al., 2022). The primary use of glucocorticoid enhanced the regenerative effects of PRP in early inflammatory tendinopathy (Ruan et al., 2021). However, PRP combined with eccentric training in chronic Achilles tendinopathy proved more effective in improving activity levels and reducing pain, tendon thickness, and intratendinous vascularity (Boesen et al., 2017). A study indicated that PRP injection may be an intensive treatment for patients with AT rupture (Padilla et al., 2021). However, studies have shown that the application of PRP in the nonsurgical treatment of AT rupture showed no special clinical effect or functional improvement and is not as effective as percutaneous fixation in reducing pain (Boesen et al., 2017; Kearney et al., 2021; Kirschner et al., 2021). This difference in results may be attributed to varying treatment regimens. Nonetheless, despite the controversial results of PRP use for tendinopathy, it remains the most used biological treatment (Kirschner et al., 2021).
In conclusion, PRP is the only GF used in clinical studies because of its apparent advantages, such as self-sufficiency, convenient extraction, and high safety. However, the results are inconsistent, and studies regarding using PRP for routine management of tendon ailments are limited to allow definitive conclusions.
5.8 Combination of GFs with other GFs/stem cells/ scaffold in AT injury
As mentioned above, various GFs activate cellular processes, ECM deposition, and tissue regeneration during the different phases of tendon healing. Specific GFs critical in tendon healing are TGF-β; BMP-12, -13, −14; bFGF; PDGF; IGF-1; and VEGF; however, some GFs present complex effects on AT recovery. To improve AT healing and reduce complications, most studies mainly use GFs, often combined with scaffolds or stem cells, to surgically create tendon defects (Table 3). Several types of stem cells, including BMSCs, AD-MSCs, TDSCs, ESCs, and terminated differentiated cells (Muscle cells), were utilized in combination with GFs and scaffolds for AT treatment. These stem cells were derived from various sources. The clinical application of ESCs has been limited due to ethical concerns. On the other hand, MSCs are extensively utilized in musculoskeletal repair due to their ability to self-renew and differentiate into various mesoderm-derived tissues, such as bone, cartilage, muscle, tendon, and fat. BMSCs and AD-MSCs are the two main types of MSCs used in AT injury, and both have shown significant improvements in AT healing in animal models. However, AD-MSCs have certain advantages such as ease of harvesting, ready availability, and low donor site morbidity. On the other hand, the use of BMSCs is limited due to the painful technique of bone marrow aspiration, which can also cause donor site morbidity (Pillai et al., 2017). TDSCs, or tendon-derived stem cells, possess a similar proliferative capacity to other types of stem cells. However, TDSCs have demonstrated higher clonogenicity, faster proliferation, and increased expression of TnmD, Scx, and Col1A1 compared to BMSCs (Tan et al., 2012). TDSCs may be a more favorable cell source for musculoskeletal tissue regeneration. However, obtaining autologous TDSCs without causing donor site morbidity can be challenging. Many results indicated that combining GFs with SCs or scaffolds may be a promising approach to treating AT injury. However, further studies investigating whether the combination of GFs/SCs/scaffolds can achieve the best efficacy with the least adverse effects in AT injury are warranted.
6 Clinical applications and limitations of GFs
GFs play a critical role in AT injury repair. GFs accelerate wound site restoration and improve functional recovery. However, they have some limitations, such as short effective half-life, instability, and promotion of scar and adhesion formation. PRP is the only GF to enter clinical trials for AT repair because of its apparent advantages, such as self-sufficiency, convenient extraction, and high safety. Based on the results of many basic research and animal experiments, some clinicians treated AT injury patients with local injections of autologous PRP. Nonetheless, the conclusions drawn from numerous clinical studies on the efficacy and safety of PRP remain inconsistent (Table 4). The three different isoforms of TGF (TGF-β1, -β2, and -β3) upregulate the production of Col I and Col III, playing an essential role in tendon healing. Contrarily, TGF-β1 appears to be responsible for scar and adhesion formation. A clinical trial of TGF-β for indications outside of the tendon had to be halted due to excessive scar formation; therefore, TGF-β was not tested in AT healing in the clinic. PDGF-AA drives both tenogenesis and fibrosis. However, further studies regarding using PDGF-AA to accelerate AT injury recovery while reducing scars are warranted. VEGF plays a vital role in angiogenesis during AT healing, and vascular ingrowth is necessary for tendon healing. However, hypervascularization may be associated with degeneration. Therefore, the role of GFs in AT repair is a double-edged sword, and the question of how to better apply it remains unsolved. As previously indicated, there is still a long way from basic research to clinical application.
7 Conclusion
Repair and healing after AT injury are low due to the tendon’s low cellularity, vascularity, and metabolic activity. In general, injured tendons rarely regain the structural integrity and mechanical strength of healthy tendons and are, therefore, prone to reinjury. GFs play a significant role in natural tendon regeneration as they participate in cell recruitment and stimulation of ECM synthesis. In recent years, GFs have been a popular treatment option for tendon injuries, which provided new perspectives on the healing of tendon injuries. The combination of multiple GFs with stem cells/scaffolds/other GFs also showed promising efficacy compared with individual GFs.
The GFs discussed in this review have important and varied roles in AT healing, affecting their functions and molecular changes. TGF-β2, IGF-I, and bFGF are involved in multiple signaling pathways and promote the synthesis of ECM components, cell proliferation, migration, and directed differentiation of MSCs. VEGF regulates angiogenesis within the wound site. PDGF shows different effectiveness in promoting tendon healing in varying stages of the injury. TGF-β2 and BMP-2 sometimes hinder AT injury recovery and even cause more severe complications. The effectiveness of PRP in AT repair remains controversial. There are also complex interactions between different cytokines, showing synergistic or antagonistic effects. Although clinical studies have confirmed the definite efficacy of some GFs, insignificant therapeutic effects have also been reported.
Several challenges must be overcome before GF delivery can translate into clinical practice, and further research is warranted (Prabhath et al., 2018). For instance, it is important to (1) further define the roles of each GF in AT healing and selecting the optimal GF(s) or a combination of GFs, (2) identify and clarify the synergistic and antagonistic influences they have on each other when combined, (3) confirm the most efficient stage and duration of delivery of each GF, (4) determine the effective dose of the selected GF and its retention time at the injured site, and (5) determine the most appropriate strategy for GF administration, including using gene therapy, recombinant GFs, or biomaterial scaffolds. If biomaterial scaffolds are used for GF sustained release, the most appropriate material and scaffold form must be selected.
Adhesion and fibrosis, which are critical problems affecting the degree of healing, are essential difficulties to overcome in repairing AT injury. GFs can simultaneously promote healing and cause fibrosis. The direction for further research should be how to effectively use GFs or combine GFs/stem cells/tissue engineering scaffolds in AT repair.
Author contributions
Conceptualization, WL and ML; writing-original draft preparation, ML, WL, XN, HL, and XC; writing-review and editing, YL, MJ, and CW; funding acquisition, ML; All authors contributed to the article and approved the submitted version.
Funding
This research was supported by the following funding: The young and middle-aged science and technology innovation talents support the program of Shenyang, No: RC210454. Basic Research Project of the Education Department of Liaoning Province, No. LJKZ0740.
Conflict of interest
The authors declare that the research was conducted in the absence of any commercial or financial relationships that could be construed as a potential conflict of interest.
Publisher’s note
All claims expressed in this article are solely those of the authors and do not necessarily represent those of their affiliated organizations, or those of the publisher, the editors and the reviewers. Any product that may be evaluated in this article, or claim that may be made by its manufacturer, is not guaranteed or endorsed by the publisher.
Supplementary material
The Supplementary Material for this article can be found online at: https://www.frontiersin.org/articles/10.3389/fbioe.2023.1250533/full#supplementary-material
References
Acharya, B., Miah, S., and Frett, B. (2022). Targeting TGF-β: triumphs and challenges. Future Med. Chem. 14, 455–458. doi:10.4155/fmc-2021-0344
Ahmed, A. S., Li, J., Schizas, N., Ahmed, M., Ostenson, C. G., Salo, P., et al. (2014). Expressional changes in growth and inflammatory mediators during achilles tendon repair in diabetic rats: new insights into a possible basis for compromised healing. Cell Tissue Res. 357, 109–117. doi:10.1007/s00441-014-1871-3
Ahmed, I. M., Lagopoulos, M., McConnell, P., Soames, R. W., and Sefton, G. K. (1998). Blood supply of the Achilles tendon. J. Orthop. Res. 16, 591–596. doi:10.1002/jor.1100160511
Alsousou, J., Thompson, M., Harrison, P., Willett, K., and Franklin, S. (2015). Effect of platelet-rich plasma on healing tissues in acute ruptured achilles tendon: A human immunohistochemistry study. Lancet 385 (1), S19. doi:10.1016/s0140-6736(15)60334-8
Andarawis-Puri, N., Flatow, E. L., and Soslowsky, L. J. (2015). Tendon basic science: development, repair, regeneration, and healing. J. Orthop. Res. 33, 780–784. doi:10.1002/jor.22869
Angrisani, N., Willbold, E., Kampmann, A., Derksen, A., and Reifenrath, J. (2022). Histology of tendon and enthesis - suitable techniques for specific research questions. Eur. Cell Mater 43, 228–251. doi:10.22203/ecm.v043a16
Arslan, E., Nellesen, T., Bayer, A., Prescher, A., Lippross, S., Nebelung, S., et al. (2016). Effect of platelet mediator concentrate (PMC) on achilles tenocytes: an in vitro study. BMC Musculoskelet. Disord. 17, 307. doi:10.1186/s12891-016-1160-2
Aschner, Y., and Downey, G. P. (2016). Transforming growth factor-β: master regulator of the respiratory system in health and disease. Am. J. Respir. Cell Mol. Biol. 54, 647–655. doi:10.1165/rcmb.2015-0391tr
Astill, B. D., Katsma, M. S., Cauthon, D. J., Greenlee, J., Murphy, M., Curtis, D., et al. (2017). Sex-based difference in Achilles peritendinous levels of matrix metalloproteinases and growth factors after acute resistance exercise. J. Appl. Physiol. (1985) 122, 361–367. doi:10.1152/japplphysiol.00878.2016
Barsby, T., Bavin, E. P., and Guest, D. J. (2014). Three-dimensional culture and transforming growth factor beta3 synergistically promote tenogenic differentiation of equine embryo-derived stem cells. Tissue Eng. Part A 20, 2604–2613. doi:10.1089/ten.tea.2013.0457
Bennell, K. L., Paterson, K. L., Metcalf, B. R., Duong, V., Eyles, J., Kasza, J., et al. (2021). Effect of intra-articular platelet-rich plasma vs placebo injection on pain and medial tibial cartilage volume in patients with knee osteoarthritis: the RESTORE randomized clinical trial. JAMA 326, 2021–2030. doi:10.1001/jama.2021.19415
Boesen, A. P., Boesen, M. I., Hansen, R., Barfod, K. W., Lenskjold, A., Malliaras, P., et al. (2020). Effect of platelet-rich plasma on nonsurgically treated acute achilles tendon ruptures: A randomized, double-blinded prospective study. Am. J. Sports Med. 48, 2268–2276. doi:10.1177/0363546520922541
Boesen, A. P., Hansen, R., Boesen, M. I., Malliaras, P., and Langberg, H. (2017). Effect of high-volume injection, platelet-rich plasma, and sham treatment in chronic midportion achilles tendinopathy: A randomized double-blinded prospective study. Am. J. Sports Med. 45, 2034–2043. doi:10.1177/0363546517702862
Bolt, P., Clerk, A. N., Luu, H. H., Kang, Q., Kummer, J. L., Deng, Z. L., et al. (2007). BMP-14 gene therapy increases tendon tensile strength in a rat model of Achilles tendon injury. J. Bone Jt. Surg. Am. 89, 1315–1320. doi:10.2106/00004623-200706000-00021
Bottagisio, M., Lopa, S., Granata, V., Talo, G., Bazzocchi, C., Moretti, M., et al. (2017). Different combinations of growth factors for the tenogenic differentiation of bone marrow mesenchymal stem cells in monolayer culture and in fibrin-based three-dimensional constructs. Differentiation 95, 44–53. doi:10.1016/j.diff.2017.03.001
Brown, J. P., Finley, V. G., and Kuo, C. K. (2014). Embryonic mechanical and soluble cues regulate tendon progenitor cell gene expression as a function of developmental stage and anatomical origin. J. Biomech. 47, 214–222. doi:10.1016/j.jbiomech.2013.09.018
Brown, J. P., Galassi, T. V., Stoppato, M., Schiele, N. R., and Kuo, C. K. (2015). Comparative analysis of mesenchymal stem cell and embryonic tendon progenitor cell response to embryonic tendon biochemical and mechanical factors. Stem Cell Res. Ther. 6, 89. doi:10.1186/s13287-015-0043-z
Burgisser, G. M., Evrova, O., Heuberger, D. M., Wolint, P., Rieber, J., Miescher, I., et al. (2021). Electrospun tube reduces adhesion in rabbit Achilles tendon 12 weeks post-surgery without PAR-2 overexpression. Sci. Rep. 11, 23293. doi:10.1038/s41598-021-02780-4
Campanelli, V., Fantini, M., Faccioli, N., Cangemi, A., Pozzo, A., and Sbarbati, A. (2011). Three-dimensional morphology of heel fat pad: an in vivo computed tomography study. J. Anat. 219, 622–631. doi:10.1111/j.1469-7580.2011.01420.x
Celik, M., Bayrak, A., Duramaz, A., Basaran, S. H., Kizilkaya, C., Kural, C., et al. (2021). The effect of fibrin clot and C vitamin on the surgical treatment of Achilles tendon injury in the rat model. Foot Ankle Surg. 27, 681–687. doi:10.1016/j.fas.2020.09.006
Cetik, R. M., Yabanoglu Ciftci, S., Arica, B., Baysal, I., Akarca Dizakar, S. O., Erbay Elibol, F. K., et al. (2022). Evaluation of the effects of transforming growth factor–beta 3 (TGF-β3) loaded nanoparticles on healing in a rat achilles tendon injury model. Am. J. Sports Med. 50, 1066–1077. doi:10.1177/03635465211073148
Chamberlain, C. S., Lee, J. S., Leiferman, E. M., Maassen, N. X., Baer, G. S., Vanderby, R., et al. (2015). Effects of BMP-12-releasing sutures on Achilles tendon healing. Tissue Eng. Part A 21, 916–927. doi:10.1089/ten.tea.2014.0001
Chen, C. H., Lin, Y. H., Chen, C. H., Wang, Y. H., Yeh, M. L., Cheng, T. L., et al. (2018a). Transforming growth factor beta 1 mediates the low-frequency vertical vibration enhanced production of tenomodulin and type I collagen in rat Achilles tendon. PLoS One 13, e0205258. doi:10.1371/journal.pone.0205258
Chen, H., Wang, Z., Zhou, L., Wu, B., Lu, H., Zhang, C., et al. (2021). Recombinant human bone morphogenetic protein-4 enhances tendon-to-bone attachment healing in a murine model of rotator cuff tear. Ann. Transl. Med. 9, 565. doi:10.21037/atm-20-6761
Chen, J., Yu, Q., Wu, B., Lin, Z., Pavlos, N. J., Xu, J., et al. (2011). Autologous tenocyte therapy for experimental Achilles tendinopathy in a rabbit model. Tissue Eng. Part A 17, 2037–2048. doi:10.1089/ten.tea.2010.0492
Chen, L., Dong, S. W., Liu, J. P., Tao, X., Tang, K. L., and Xu, J. Z. (2012). Synergy of tendon stem cells and platelet-rich plasma in tendon healing. J. Orthop. Res. 30, 991–997. doi:10.1002/jor.22033
Chen, Q. J., Chen, L., Wu, S. K., Wu, Y. J., and Pang, Q. J. (2018b). rhPDGF-BB combined with ADSCs in the treatment of Achilles tendinitis via miR-363/PI3 K/Akt pathway. Mol. Cell Biochem. 438, 175–182. doi:10.1007/s11010-017-3124-8
Chien, C., Pryce, B., Tufa, S. F., Keene, D. R., and Huang, A. H. (2018). Optimizing a 3D model system for molecular manipulation of tenogenesis. Connect. Tissue Res. 59, 295–308. doi:10.1080/03008207.2017.1383403
Chiou, G. J., Crowe, C., McGoldrick, R., Hui, K., Pham, H., and Chang, J. (2015). Optimization of an injectable tendon hydrogel: the effects of platelet-rich plasma and adipose-derived stem cells on tendon healing in vivo. Tissue Eng. Part A 21, 1579–1586. doi:10.1089/ten.tea.2014.0490
Cui, J., Chen, Z., and Wu, W. (2019). Expression of TGF-β1 and VEGF in patients with Achilles tendon rupture and the clinical efficacy. Exp. Ther. Med. 18, 3502–3508. doi:10.3892/etm.2019.7968
Cui, J., Ning, L. J., Wu, F. P., Hu, R. N., Li, X., He, S. K., et al. (2022). Biomechanically and biochemically functional scaffold for recruitment of endogenous stem cells to promote tendon regeneration. NPJ Regen. Med. 7, 26. doi:10.1038/s41536-022-00220-z
D'Addona, A., Maffulli, N., Formisano, S., and Rosa, D. (2017). Inflammation in tendinopathy. Surgeon 15, 297–302. doi:10.1016/j.surge.2017.04.004
Dai, G., Li, Y., Liu, J., Zhang, C., Chen, M., Lu, P., et al. (2020). Higher BMP expression in tendon stem/progenitor cells contributes to the increased heterotopic ossification in achilles tendon with aging. Front. Cell Dev. Biol. 8, 570605. doi:10.3389/fcell.2020.570605
Dai, L., Hu, X., Zhang, X., Zhu, J., Zhang, J., Fu, X., et al. (2015). Different tenogenic differentiation capacities of different mesenchymal stem cells in the presence of BMP-12. J. Transl. Med. 13, 200. doi:10.1186/s12967-015-0560-7
Darshan, G. D., Chen, C. H., Kuo, C. Y., Shalumon, K. T., Chien, Y. M., Kao, H. H., et al. (2022). Development of high resilience spiral wound suture-embedded gelatin/PCL/heparin nanofiber membrane scaffolds for tendon tissue engineering. Int. J. Biol. Macromol. 221, 314–333. doi:10.1016/j.ijbiomac.2022.09.001
Dayton, P. (2017). Anatomic, vascular, and mechanical overview of the achilles tendon. Clin. Podiatr. Med. Surg. 34, 107–113. doi:10.1016/j.cpm.2016.10.002
de Vos, R. J., Weir, A., Tol, J. L., Verhaar, J. A., Weinans, H., and van Schie, H. T. (2011). No effects of PRP on ultrasonographic tendon structure and neovascularisation in chronic midportion Achilles tendinopathy. Br. J. Sports Med. 45, 387–392. doi:10.1136/bjsm.2010.076398
de Vos, R. J., Weir, A., van Schie, H. T., Bierma-Zeinstra, S. M., Verhaar, J. A., Weinans, H., et al. (2010). Platelet-rich plasma injection for chronic achilles tendinopathy: A randomized controlled trial. JAMA 303, 144–149. doi:10.1001/jama.2009.1986
Deng, L., Huang, L., Guo, Q., Shi, X., and Xu, K. (2017). CREB1 and Smad3 mediate TGF-β3-induced Smad7 expression in rat hepatic stellate cells. Mol. Med. Rep. 16, 8455–8462. doi:10.3892/mmr.2017.7654
Disser, N. P., Sugg, K. B., Talarek, J. R., Sarver, D. C., Rourke, B. J., and Mendias, C. L. (2019). Insulin-like growth factor 1 signaling in tenocytes is required for adult tendon growth. FASEB J. 33, 12680–12695. doi:10.1096/fj.201901503r
Dohan Ehrenfest, D. M., Andia, I., Zumstein, M. A., Zhang, C. Q., Pinto, N. R., and Bielecki, T. (2014). Classification of platelet concentrates (Platelet-Rich plasma-PRP, platelet-rich fibrin-PRF) for topical and infiltrative use in orthopedic and sports medicine: current consensus, clinical implications and perspectives. Muscles Ligaments Tendons J. 4, 3–9.
Doral, M. N., Alam, M., Bozkurt, M., Turhan, E., Atay, O. A., Donmez, G., et al. (2010). Functional anatomy of the Achilles tendon. Knee Surg. Sports Traumatol. Arthrosc. 18, 638–643. doi:10.1007/s00167-010-1083-7
Dunworth, W. P., Cardona-Costa, J., Bozkulak, E. C., Kim, J. D., Meadows, S., Fischer, J. C., et al. (2014). Bone morphogenetic protein 2 signaling negatively modulates lymphatic development in vertebrate embryos. Circ. Res. 114, 56–66. doi:10.1161/circresaha.114.302452
Eliasson, P., Fahlgren, A., and Aspenberg, P. (2008). Mechanical load and BMP signaling during tendon repair: A role for follistatin? Clin. Orthop. Relat. Res. 466, 1592–1597. doi:10.1007/s11999-008-0253-0
Evrova, O., Burgisser, G. M., Ebnother, C., Adathala, A., Calcagni, M., Bachmann, E., et al. (2020). Elastic and surgeon friendly electrospun tubes delivering PDGF-BB positively impact tendon rupture healing in a rabbit Achilles tendon model. Biomaterials 232, 119722. doi:10.1016/j.biomaterials.2019.119722
Evrova, O., and Buschmann, J. (2017). In vitro and in vivo effects of PDGF-BB delivery strategies on tendon healing: A review. Eur. Cell Mater 34, 15–39. doi:10.22203/ecm.v034a02
Fenwick, S. A., Curry, V., Harrall, R. L., Hazleman, B. L., Hackney, R., and Riley, G. P. (2001). Expression of transforming growth factor-beta isoforms and their receptors in chronic tendinosis. J. Anat. 199, 231–240. doi:10.1046/j.1469-7580.2001.19930231.x
Fitzgerald, M. J., Mustapich, T., Liang, H., Larsen, C. G., Nellans, K. W., and Grande, D. A. (2021). Tendon transection healing can Be improved with adipose-derived stem cells cultured with growth differentiation factor 5 and platelet-derived growth factor. Hand (N Y) 18, 436–445. doi:10.1177/15589447211028929
Font Tellado, S., Chiera, S., Bonani, W., Poh, P. S. P., Migliaresi, C., Motta, A., et al. (2018). Heparin functionalization increases retention of TGF-β2 and GDF5 on biphasic silk fibroin scaffolds for tendon/ligament-to-bone tissue engineering. Acta Biomater. 72, 150–166. doi:10.1016/j.actbio.2018.03.017
Fukuta, S., Oyama, M., Kavalkovich, K., Fu, F. H., and Niyibizi, C. (1998). Identification of types II, IX and X collagens at the insertion site of the bovine achilles tendon. Matrix Biol. 17, 65–73. doi:10.1016/s0945-053x(98)90125-1
Ganestam, A., Kallemose, T., Troelsen, A., and Barfod, K. W. (2016). Increasing incidence of acute Achilles tendon rupture and a noticeable decline in surgical treatment from 1994 to 2013. A nationwide registry study of 33,160 patients. Knee Surg. Sports Traumatol. Arthrosc. 24, 3730–3737. doi:10.1007/s00167-015-3544-5
Glass, Z. A., Schiele, N. R., and Kuo, C. K. (2014). Informing tendon tissue engineering with embryonic development. J. Biomech. 47, 1964–1968. doi:10.1016/j.jbiomech.2013.12.039
Gong, F., Li, X., Zhang, H., Wu, J., Ma, G., Zhang, B., et al. (2022). Comparison of the effects of open surgery and minimally invasive surgery on the achilles tendon rupture healing based on angiogenesis. Comput. Intell. Neurosci. 2022, 1–8. doi:10.1155/2022/1447129
Guerit, E., Arts, F., Dachy, G., Boulouadnine, B., and Demoulin, J. B. (2021). PDGF receptor mutations in human diseases. Cell Mol. Life Sci. 78, 3867–3881. doi:10.1007/s00018-020-03753-y
Guerquin, M. J., Charvet, B., Nourissat, G., Havis, E., Ronsin, O., Bonnin, M. A., et al. (2013). Transcription factor EGR1 directs tendon differentiation and promotes tendon repair. J. Clin. Invest. 123, 3564–3576. doi:10.1172/jci67521
Guo, D., Li, H., Liu, Y., Yu, X., Zhang, X., Chu, W., et al. (2020). Fibroblast growth factor-2 promotes the function of tendon-derived stem cells in Achilles tendon restoration in an Achilles tendon injury rat model. Biochem. Biophys. Res. Commun. 521, 91–97. doi:10.1016/j.bbrc.2019.10.082
Harvey, T., Flamenco, S., and Fan, C. M. (2019). A Tppp3(+)Pdgfra(+) tendon stem cell population contributes to regeneration and reveals a shared role for PDGF signalling in regeneration and fibrosis. Nat. Cell Biol. 21, 1490–1503. doi:10.1038/s41556-019-0417-z
Hoffmann, A., Pelled, G., Turgeman, G., Eberle, P., Zilberman, Y., Shinar, H., et al. (2006). Neotendon formation induced by manipulation of the Smad8 signalling pathway in mesenchymal stem cells. J. Clin. Invest. 116, 940–952. doi:10.1172/jci22689
Holladay, C., Abbah, S. A., O'Dowd, C., Pandit, A., and Zeugolis, D. I. (2016). Preferential tendon stem cell response to growth factor supplementation. J. Tissue Eng. Regen. Med. 10, 783–798. doi:10.1002/term.1852
Hortensius, R. A., and Harley, B. A. (2013). The use of bioinspired alterations in the glycosaminoglycan content of collagen-GAG scaffolds to regulate cell activity. Biomaterials 34, 7645–7652. doi:10.1016/j.biomaterials.2013.06.056
Hou, Y., Mao, Z., Wei, X., Lin, L., Chen, L., Wang, H., et al. (2009a). Effects of transforming growth factor-β1 and vascular endothelial growth factor 165 gene transfer on Achilles tendon healing. Matrix Biol. 28, 324–335. doi:10.1016/j.matbio.2009.04.007
Hou, Y., Mao, Z., Wei, X., Lin, L., Chen, L., Wang, H., et al. (2009b). The roles of TGF-β1 gene transfer on collagen formation during Achilles tendon healing. Biochem. Biophys. Res. Commun. 383, 235–239. doi:10.1016/j.bbrc.2009.03.159
Hung, C. Y., Lin, S. J., Yeh, C. Y., and Yeh, W. L. (2022). Effect of platelet-rich plasma augmentation on endoscopy-assisted percutaneous achilles tendon repair. J. Clin. Med. 11, 5389. doi:10.3390/jcm11185389
Hyun, S. Y., Lee, J. H., Kang, K. J., and Jang, Y. J. (2017). Effect of FGF-2, TGF-beta-1, and BMPs on teno/ligamentogenesis and osteo/cementogenesis of human periodontal ligament stem cells. Mol. Cells 40, 550–557. doi:10.14348/molcells.2017.0019
Im, G. I., and Kim, T. K. (2020). Stem cells for the regeneration of tendon and ligament: A perspective. Int. J. Stem Cells 13, 335–341. doi:10.15283/ijsc20091
Jayasree, A., Kottappally Thankappan, S., Ramachandran, R., Sundaram, M. N., Chen, C. H., Mony, U., et al. (2019). Bioengineered braided micro-nano (multiscale) fibrous scaffolds for tendon reconstruction. ACS Biomater. Sci. Eng. 5, 1476–1486. doi:10.1021/acsbiomaterials.8b01328
Jiang, D., Gao, P., Zhang, Y., and Yang, S. (2016a). Combined effects of engineered tendon matrix and GDF-6 on bone marrow mesenchymal stem cell-based tendon regeneration. Biotechnol. Lett. 38, 885–892. doi:10.1007/s10529-016-2037-z
Jiang, D., Xu, B., Yang, M., Zhao, Z., Zhang, Y., and Li, Z. (2014). Efficacy of tendon stem cells in fibroblast-derived matrix for tendon tissue engineering. Cytotherapy 16, 662–673. doi:10.1016/j.jcyt.2013.07.014
Jiang, K., Chun, G., Wang, Z., Du, Q., Wang, A., and Xiong, Y. (2016b). Effect of transforming growth factor-β3 on the expression of Smad3 and Smad7 in tenocytes. Mol. Med. Rep. 13, 3567–3573. doi:10.3892/mmr.2016.4944
Kang, S., Yoon, J. S., Lee, J. Y., Kim, H. J., Park, K., and Kim, S. E. (2019). Long-term local PDGF delivery using porous microspheres modified with heparin for tendon healing of rotator cuff tendinitis in a rabbit model. Carbohydr. Polym. 209, 372–381. doi:10.1016/j.carbpol.2019.01.017
Kearney, R. S., Ji, C., Warwick, J., Parsons, N., Brown, J., Harrison, P., et al. (2021). Effect of platelet-rich plasma injection vs sham injection on tendon dysfunction in patients with chronic midportion achilles tendinopathy: A randomized clinical trial. JAMA 326, 137–144. doi:10.1001/jama.2021.6986
Keene, D. J., Alsousou, J., Harrison, P., O'Connor, H. M., Wagland, S., Dutton, S. J., et al. (2022). Platelet-rich plasma injection for acute achilles tendon rupture: two-year follow-up of the PATH-2 randomized, placebo-controlled, superiority trial. Bone Jt. J. 104-B, 1256–1265. doi:10.1302/0301-620x.104b11.bjj-2022-0653.r1
Ker, E. D., Nain, A. S., Weiss, L. E., Wang, J., Suhan, J., Amon, C. H., et al. (2011). Bioprinting of growth factors onto aligned sub-micron fibrous scaffolds for simultaneous control of cell differentiation and alignment. Biomaterials 32, 8097–8107. doi:10.1016/j.biomaterials.2011.07.025
Kim, H. J., Kang, S. W., Lim, H. C., Han, S. B., Lee, J. S., Prasad, L., et al. (2007). The role of transforming growth factor-beta and bone morphogenetic protein with fibrin glue in healing of bone-tendon junction injury. Connect. Tissue Res. 48, 309–315. doi:10.1080/03008200701692610
Kim, H. J., Nam, H. W., Hur, C. Y., Park, M., Yang, H. S., Kim, B. S., et al. (2011). The effect of platelet rich plasma from bone marrow aspirate with added bone morphogenetic protein-2 on the Achilles tendon-bone junction in rabbits. Clin. Orthop. Surg. 3, 325–331. doi:10.4055/cios.2011.3.4.325
Kirschner, J. S., Cheng, J., Hurwitz, N., Santiago, K., Lin, E., Beatty, N., et al. (2021). Ultrasound-guided percutaneous needle tenotomy (pnt) alone versus pnt plus platelet-rich plasma injection for the treatment of chronic tendinosis: A randomized controlled trial. PM R. 13, 1340–1349. doi:10.1002/pmrj.12583
Koch, D. W., Schnabel, L. V., Ellis, I. M., Bates, R. E., and Berglund, A. K. (2022). TGF-β2 enhances expression of equine bone marrow-derived mesenchymal stem cell paracrine factors with known associations to tendon healing. Stem Cell Res. Ther. 13, 477. doi:10.1186/s13287-022-03172-9
Kokubu, S., Inaki, R., Hoshi, K., and Hikita, A. (2020). Adipose-derived stem cells improve tendon repair and prevent ectopic ossification in tendinopathy by inhibiting inflammation and inducing neovascularization in the early stage of tendon healing. Regen. Ther. 14, 103–110. doi:10.1016/j.reth.2019.12.003
Kraus, A., Sattler, D., Wehland, M., Luetzenberg, R., Abuagela, N., and Infanger, M. (2018). Vascular endothelial growth factor enhances proliferation of human tenocytes and promotes tenogenic gene expression. Plast. Reconstr. Surg. 142, 1240–1247. doi:10.1097/prs.0000000000004920
Kraus, T. M., Imhoff, F. B., Reinert, J., Wexel, G., Wolf, A., Hirsch, D., et al. (2016). Stem cells and bFGF in tendon healing: effects of lentiviral gene transfer and long-term follow-up in a rat achilles tendon defect model. BMC Musculoskelet. Disord. 17, 148. doi:10.1186/s12891-016-0999-6
Kraus, T. M., Imhoff, F. B., Wexel, G., Wolf, A., Hirsch, D., Lenz, L., et al. (2014). Stem cells and basic fibroblast growth factor failed to improve tendon healing: an in vivo study using lentiviral gene transfer in a rat model. J. Bone Jt. Surg. Am. 96, 761–769. doi:10.2106/jbjs.l.01794
Kuo, C. K., Petersen, B. C., and Tuan, R. S. (2008). Spatiotemporal protein distribution of TGF-βs, their receptors, and extracellular matrix molecules during embryonic tendon development. Dev. Dyn. 237, 1477–1489. doi:10.1002/dvdy.21547
Kurtz, C. A., Loebig, T. G., Anderson, D. D., DeMeo, P. J., and Campbell, P. G. (1999). Insulin-like growth factor I accelerates functional recovery from Achilles tendon injury in a rat model. Am. J. Sports Med. 27, 363–369. doi:10.1177/03635465990270031701
Lee, J. Y., Zhou, Z., Taub, P. J., Ramcharan, M., Li, Y., Akinbiyi, T., et al. (2011). BMP-12 treatment of adult mesenchymal stem cells in vitro augments tendon-like tissue formation and defect repair in vivo. PLoS One 6, e17531. doi:10.1371/journal.pone.0017531
Legrand, J. M. D., and Martino, M. M. (2022). Growth factor and cytokine delivery systems for wound healing. Cold Spring Harb. Perspect. Biol. 14, a041234. doi:10.1101/cshperspect.a041234
Li, M., Jia, J., Li, S., Cui, B., Huang, J., Guo, Z., et al. (2021a). Exosomes derived from tendon stem cells promote cell proliferation and migration through the TGF beta signal pathway. Biochem. Biophys. Res. Commun. 536, 88–94. doi:10.1016/j.bbrc.2020.12.057
Li, P., Zhou, H., Tu, T., and Lu, H. (2021b). Dynamic exacerbation in inflammation and oxidative stress during the formation of peritendinous adhesion resulted from acute tendon injury. J. Orthop. Surg. Res. 16, 293. doi:10.1186/s13018-021-02445-y
Lin, L., Shen, Q., Xue, T., and Yu, C. (2010). Heterotopic ossification induced by achilles tenotomy via endochondral bone formation: expression of bone and cartilage related genes. Bone 46, 425–431. doi:10.1016/j.bone.2009.08.057
Liu, C., Tian, S., Bai, J., Yu, K., Liu, L., Liu, G., et al. (2020). <p>Regulation of ERK1/2 and SMAD2/3 pathways by using multi-layered electrospun PCL–amnion nanofibrous membranes for the prevention of post-surgical tendon adhesion</p>. Int. J. Nanomedicine 15, 927–942. doi:10.2147/ijn.s231538
Liu, C. J., Yu, K. L., Bai, J. B., Tian, D. H., and Liu, G. L. (2019). Platelet-rich plasma injection for the treatment of chronic achilles tendinopathy: A meta-analysis. Med. Baltim. 98, e15278. doi:10.1097/md.0000000000015278
Liu, H., Zhang, C., Zhu, S., Lu, P., Zhu, T., Gong, X., et al. (2015a). Mohawk promotes the tenogenesis of mesenchymal stem cells through activation of the TGFβ signaling pathway. Stem Cells 33, 443–455. doi:10.1002/stem.1866
Liu, J., Tao, X., Chen, L., Han, W., Zhou, Y., and Tang, K. (2015b). CTGF positively regulates BMP12 induced tenogenic differentiation of tendon stem cells and signaling. Cell Physiol. Biochem. 35, 1831–1845. doi:10.1159/000373994
Liu, X., Li, Y., Wang, S., Lu, M., Zou, J., Shi, Z., et al. (2022). PDGF-loaded microneedles promote tendon healing through p38/cyclin D1 pathway mediated angiogenesis. Mater Today Bio 16, 100428. doi:10.1016/j.mtbio.2022.100428
Lohrer, H., Arentz, S., Nauck, T., Dorn-Lange, N. V., and Konerding, M. A. (2008). The achilles tendon insertion is crescent-shaped: an in vitro anatomic investigation. Clin. Orthop. Relat. Res. 466, 2230–2237. doi:10.1007/s11999-008-0298-0
Lowery, J. W., and Rosen, V. (2018). The BMP pathway and its inhibitors in the skeleton. Physiol. Rev. 98, 2431–2452. doi:10.1152/physrev.00028.2017
Maffulli, N., Longo, U. G., Franceschi, F., Rabitti, C., and Denaro, V. (2008). Movin and Bonar scores assess the same characteristics of tendon histology. Clin. Orthop. Relat. Res. 466, 1605–1611. doi:10.1007/s11999-008-0261-0
Majewski, M., Betz, O., Ochsner, P. E., Liu, F., Porter, R. M., and Evans, C. H. (2008). Ex vivo adenoviral transfer of bone morphogenetic protein 12 (BMP-12) cDNA improves Achilles tendon healing in a rat model. Gene Ther. 15, 1139–1146. doi:10.1038/gt.2008.48
Majewski, M., Heisterbach, P., Jaquiery, C., Durselen, L., Todorov, A., Martin, I., et al. (2018). Improved tendon healing using bFGF, BMP-12 and TGFβ1 in a rat model. Eur. Cell Mater 35, 318–334. doi:10.22203/ecm.v035a22
Majewski, M., Porter, R. M., Betz, O. B., Betz, V. M., Clahsen, H., Fluckiger, R., et al. (2012). Improvement of tendon repair using muscle grafts transduced with TGF-β1 cDNA. Eur. Cell Mater 23, 94–102. doi:10.22203/ecm.v023a07
Martin, R. L., Chimenti, R., Cuddeford, T., Houck, J., Matheson, J. W., McDonough, C. M., et al. (2018). Achilles pain, stiffness, and muscle power deficits: midportion achilles tendinopathy revision 2018. J. Orthop. Sports Phys. Ther. 48, A1–A38. doi:10.2519/jospt.2018.0302
McCartney, W., Ober, C., Benito, M., and MacDonald, B. (2019). Suturing achilles tendon and mesh simultaneously in augmented repair resists gap formation foremost: an experimental study. J. Orthop. Surg. Res. 14, 332. doi:10.1186/s13018-019-1390-8
Meier Burgisser, G., Evrova, O., Calcagni, M., Scalera, C., Giovanoli, P., and Buschmann, J. (2020). Impact of PDGF-BB on cellular distribution and extracellular matrix in the healing rabbit Achilles tendon three weeks post-operation. FEBS Open Bio 10, 327–337. doi:10.1002/2211-5463.12736
Melincovici, C. S., Bosca, A. B., Susman, S., Marginean, M., Mihu, C., Istrate, M., et al. (2018). Vascular endothelial growth factor (VEGF) - key factor in normal and pathological angiogenesis. Rom. J. Morphol. Embryol. 59, 455–467.
Mikic, B., Rossmeier, K., and Bierwert, L. (2009). Sexual dimorphism in the effect of GDF-6 deficiency on murine tendon. J. Orthop. Res. 27, 1603–1611. doi:10.1002/jor.20916
Millar, N. L., Murrell, G. A., and McInnes, I. B. (2017). Inflammatory mechanisms in tendinopathy - towards translation. Nat. Rev. Rheumatol. 13, 110–122. doi:10.1038/nrrheum.2016.213
Millar, N. L., Silbernagel, K. G., Thorborg, K., Kirwan, P. D., Galatz, L. M., Abrams, G. D., et al. (2021). Tendinopathy. Nat. Rev. Dis. Prim. 7, 1. doi:10.1038/s41572-020-00234-1
Morimoto, S., Iseki, T., Nakayama, H., Shimomura, K., Nishikawa, T., Nakamura, N., et al. (2021). Return to the original sport at only 3 months after an achilles tendon rupture by a combination of intra-tissue injection of freeze-dried platelet-derived factor concentrate and excessively early rehabilitation after operative treatment in a male basketball player: A case report. Regen. Ther. 18, 112–116. doi:10.1016/j.reth.2021.05.002
Muller, S. A., Quirk, N. P., Muller-Lebschi, J. A., Heisterbach, P. E., Durselen, L., Majewski, M., et al. (2019). Response of the injured tendon to growth factors in the presence or absence of the paratenon. Am. J. Sports Med. 47, 462–467. doi:10.1177/0363546518814534
Muller, S. A., Todorov, A., Heisterbach, P. E., Martin, I., and Majewski, M. (2015). Tendon healing: an overview of physiology, biology, and pathology of tendon healing and systematic review of state of the art in tendon bioengineering. Knee Surg. Sports Traumatol. Arthrosc. 23, 2097–2105. doi:10.1007/s00167-013-2680-z
Nagelli, C. V., Hooke, A., Quirk, N., De Padilla, C. L., Hewett, T. E., van Griensven, M., et al. (2022). Mechanical and strain behaviour of human Achilles tendon during in vitro testing to failure. Eur. Cell Mater 43, 153–161. doi:10.22203/ecm.v043a12
Nielsen, R. H., Holm, L., Jensen, J. K., Heinemeier, K. M., Remvig, L., and Kjaer, M. (2014). Tendon protein synthesis rate in classic Ehlers-Danlos patients can be stimulated with insulin-like growth factor-I. J. Appl. Physiol. (1985) 117, 694–698. doi:10.1152/japplphysiol.00157.2014
Noack, S., Seiffart, V., Willbold, E., Laggies, S., Winkel, A., Shahab-Osterloh, S., et al. (2014). Periostin secreted by mesenchymal stem cells supports tendon formation in an ectopic mouse model. Stem Cells Dev. 23, 1844–1857. doi:10.1089/scd.2014.0124
O'Brien, M. (2005). The anatomy of the Achilles tendon. Foot Ankle Clin. 10, 225–238. doi:10.1016/j.fcl.2005.01.011
Owens, R. F., Ginnetti, J., Conti, S. F., and Latona, C. (2011). Clinical and magnetic resonance imaging outcomes following platelet rich plasma injection for chronic midsubstance Achilles tendinopathy. Foot Ankle Int. 32, 1032–1039. doi:10.3113/fai.2011.1032
Ozasa, Y., Gingery, A., Thoreson, A. R., An, K. N., Zhao, C., and Amadio, P. C. (2014). A comparative study of the effects of growth and differentiation factor 5 on muscle-derived stem cells and bone marrow stromal cells in an in vitro tendon healing model. J. Hand Surg. Am. 39, 1706–1713. doi:10.1016/j.jhsa.2014.05.005
Ozeki, N., Muneta, T., Koga, H., Katagiri, H., Otabe, K., Okuno, M., et al. (2013). Transplantation of Achilles tendon treated with bone morphogenetic protein 7 promotes meniscus regeneration in a rat model of massive meniscal defect. Arthritis Rheum. 65, 2876–2886. doi:10.1002/art.38099
Padilla, S., Sanchez, M., Vaquerizo, V., Malanga, G. A., Fiz, N., Azofra, J., et al. (2021). Platelet-rich plasma applications for achilles tendon repair: A bridge between biology and surgery. Int. J. Mol. Sci. 22, 824. doi:10.3390/ijms22020824
Pakshir, P., and Hinz, B. (2018). The big five in fibrosis: macrophages, myofibroblasts, matrix, mechanics, and miscommunication. Matrix Biol. 68-69, 81–93. doi:10.1016/j.matbio.2018.01.019
Paolini, C., Agarbati, S., Benfaremo, D., Mozzicafreddo, M., Svegliati, S., and Moroncini, G. (2022). PDGF/PDGFR: A possible molecular target in scleroderma fibrosis. Int. J. Mol. Sci. 23, 3904. doi:10.3390/ijms23073904
Park, A., Hogan, M. V., Kesturu, G. S., James, R., Balian, G., and Chhabra, A. B. (2010). Adipose-derived mesenchymal stem cells treated with growth differentiation factor-5 express tendon-specific markers. Tissue Eng. Part A 16, 2941–2951. doi:10.1089/ten.tea.2009.0710
Park, Y. B., Kim, J. H., Ha, C. W., and Lee, D. H. (2021). Clinical efficacy of platelet-rich plasma injection and its association with growth factors in the treatment of mild to moderate knee osteoarthritis: A randomized double-blind controlled clinical trial as compared with hyaluronic acid. Am. J. Sports Med. 49, 487–496. doi:10.1177/0363546520986867
Pekala, P. A., Henry, B. M., Ochala, A., Kopacz, P., Taton, G., Mlyniec, A., et al. (2017). The twisted structure of the achilles tendon unraveled: A detailed quantitative and qualitative anatomical investigation. Scand. J. Med. Sci. Sports 27, 1705–1715. doi:10.1111/sms.12835
Pelled, G., Snedeker, J. G., Ben-Arav, A., Rigozzi, S., Zilberman, Y., Kimelman-Bleich, N., et al. (2012). Smad8/BMP2-engineered mesenchymal stem cells induce accelerated recovery of the biomechanical properties of the Achilles tendon. J. Orthop. Res. 30, 1932–1939. doi:10.1002/jor.22167
Perucca Orfei, C., Vigano, M., Pearson, J. R., Colombini, A., De Luca, P., Ragni, E., et al. (2019). In vitro induction of tendon-specific markers in tendon cells, adipose- and bone marrow-derived stem cells is dependent on TGFβ3, BMP-12 and ascorbic acid stimulation. Int. J. Mol. Sci. 20, 149. doi:10.3390/ijms20010149
Peserico, A., Barboni, B., Russo, V., Bernabo, N., El Khatib, M., Prencipe, G., et al. (2023). Mammal comparative tendon biology: advances in regulatory mechanisms through a computational modeling. Front. Vet. Sci. 10, 1175346. doi:10.3389/fvets.2023.1175346
Petersen, W., Pufe, T., Kurz, B., Mentlein, R., and Tillmann, B. (2002). Angiogenesis in fetal tendon development: spatial and temporal expression of the angiogenic peptide vascular endothelial cell growth factor. Anat. Embryol. Berl. 205, 263–270. doi:10.1007/s00429-002-0241-1
Pillai, D. S., Dhinsa, B. S., and Khan, W. S. (2017). Tissue engineering in achilles tendon reconstruction; the role of stem cells, growth factors and scaffolds. Curr. Stem Cell Res. Ther. 12, 506–512. doi:10.2174/1574888x12666170523162214
Prabhath, A., Vernekar, V. N., Sanchez, E., and Laurencin, C. T. (2018). Growth factor delivery strategies for rotator cuff repair and regeneration. Int. J. Pharm. 544, 358–371. doi:10.1016/j.ijpharm.2018.01.006
Rickert, M. (2008). BMP-14 gene therapy increases tendon tensile strength in a rat model of achilles tendon injury. J. Bone Jt. Surg. Am. 90, 445–446.
Rickert, M., Jung, M., Adiyaman, M., Richter, W., and Simank, H. G. (2001). A growth and differentiation factor-5 (GDF-5)-coated suture stimulates tendon healing in an Achilles tendon model in rats. Growth factors. 19, 115–126. doi:10.3109/08977190109001080
Riggin, C. N., Schultz, S. M., Sehgal, C. M., and Soslowsky, L. J. (2019). Ultrasound evaluation of anti-vascular endothelial growth factor-induced changes in vascular response following tendon injury. Ultrasound Med. Biol. 45, 1841–1849. doi:10.1016/j.ultrasmedbio.2019.03.002
Ruan, D., Fei, Y., Qian, S., Huang, Z., Chen, W., Tang, C., et al. (2021). Early-stage primary anti-inflammatory therapy enhances the regenerative efficacy of platelet-rich plasma in a rabbit achilles tendinopathy model. Am. J. Sports Med. 49, 3357–3371. doi:10.1177/03635465211037354
Ruiz-Alonso, S., Lafuente-Merchan, M., Ciriza, J., Saenz-Del-Burgo, L., and Pedraz, J. L. (2021). Tendon tissue engineering: cells, growth factors, scaffolds and production techniques. J. Control Release 333, 448–486. doi:10.1016/j.jconrel.2021.03.040
Russo, V., El Khatib, M., Prencipe, G., Citeroni, M. R., Faydaver, M., Mauro, A., et al. (2022). Tendon immune regeneration: insights on the synergetic role of stem and immune cells during tendon regeneration. Cells 11, 434. doi:10.3390/cells11030434
Schepull, T., Kvist, J., Norrman, H., Trinks, M., Berlin, G., and Aspenberg, P. (2011). Autologous platelets have no effect on the healing of human achilles tendon ruptures: A randomized single-blind study. Am. J. Sports Med. 39, 38–47. doi:10.1177/0363546510383515
Schneider, P. R., Buhrmann, C., Mobasheri, A., Matis, U., and Shakibaei, M. (2011). Three-dimensional high-density co-culture with primary tenocytes induces tenogenic differentiation in mesenchymal stem cells. J. Orthop. Res. 29, 1351–1360. doi:10.1002/jor.21400
Schroer, A. K., and Merryman, W. D. (2015). Mechanobiology of myofibroblast adhesion in fibrotic cardiac disease. J. Cell Sci. 128, 1865–1875. doi:10.1242/jcs.162891
Seeger, J. D., West, W. A., Fife, D., Noel, G. J., Johnson, L. N., and Walker, A. M. (2006). Achilles tendon rupture and its association with fluoroquinolone antibiotics and other potential risk factors in a managed care population. Pharmacoepidemiol Drug Saf. 15, 784–792. doi:10.1002/pds.1214
Sharma, P., and Maffulli, N. (2006). Biology of tendon injury: healing, modeling and remodeling. J. Musculoskelet. Neuronal Interact. 6, 181–190.
Sharma, P., and Maffulli, N. (2005). Tendon injury and tendinopathy: healing and repair. J. Bone Jt. Surg. Am. 87, 187–202. doi:10.2106/jbjs.d.01850
Shen, H., Gelberman, R. H., Silva, M. J., Sakiyama-Elbert, S. E., and Thomopoulos, S. (2013). BMP12 induces tenogenic differentiation of adipose-derived stromal cells. PLoS One 8, e77613. doi:10.1371/journal.pone.0077613
Shojaee, A., Ejeian, F., Parham, A., and Nasr Esfahani, M. H. (2022). Optimizing tenogenic differentiation of equine adipose-derived mesenchymal stem cells (eq-ASC) using TGFB3 along with BMP antagonists. Cell J. 24, 370–379. doi:10.22074/cellj.2022.7892
Slane, L. C., DeWall, R., Martin, J., Lee, K., and Thelen, D. G. (2015). Middle-aged adults exhibit altered spatial variations in Achilles tendon wave speed. Physiol. Meas. 36, 1485–1496. doi:10.1088/0967-3334/36/7/1485
Steinmann, S., Pfeifer, C. G., Brochhausen, C., and Docheva, D. (2020). Spectrum of tendon pathologies: triggers, trails and end-state. Int. J. Mol. Sci. 21, 844. doi:10.3390/ijms21030844
Stuard, W. L., Titone, R., and Robertson, D. M. (2020). The IGF/Insulin-IGFBP Axis in corneal development, wound healing, and disease. Front. Endocrinol. (Lausanne) 11, 24. doi:10.3389/fendo.2020.00024
Sun, H., Wang, Y., He, T., He, D., Hu, Y., Fu, Z., et al. (2021). Hollow polydopamine nanoparticles loading with peptide RL-QN15: A new pro-regenerative therapeutic agent for skin wounds. J. Nanobiotechnology 19, 304. doi:10.1186/s12951-021-01049-2
Sun, L., Jin, H., Sun, L., Chen, S., Huang, Y., Liu, J., et al. (2015). Hydrogen sulfide alleviates myocardial collagen remodeling in association with inhibition of TGF-β/smad signaling pathway in spontaneously hypertensive rats. Mol. Med. 20, 503–515. doi:10.2119/molmed.2013.00096
Suwalski, A., Dabboue, H., Delalande, A., Bensamoun, S. F., Canon, F., Midoux, P., et al. (2010). Accelerated Achilles tendon healing by PDGF gene delivery with mesoporous silica nanoparticles. Biomaterials 31, 5237–5245. doi:10.1016/j.biomaterials.2010.02.077
Tan, Q., Lui, P. P., Rui, Y. F., and Wong, Y. M. (2012). Comparison of potentials of stem cells isolated from tendon and bone marrow for musculoskeletal tissue engineering. Tissue Eng. Part A 18, 840–851. doi:10.1089/ten.tea.2011.0362
Tang, J. B., Wu, Y. F., Cao, Y., Chen, C. H., Zhou, Y. L., Avanessian, B., et al. (2016). Basic FGF or VEGF gene therapy corrects insufficiency in the intrinsic healing capacity of tendons. Sci. Rep. 6, 20643. doi:10.1038/srep20643
Tang, Y., Leng, Q., Xiang, X., Zhang, L., Yang, Y., and Qiu, L. (2015). Use of ultrasound-targeted microbubble destruction to transfect IGF-1 cDNA to enhance the regeneration of rat wounded Achilles tendon in vivo. Gene Ther. 22, 610–618. doi:10.1038/gt.2015.32
Tauriello, D. V. F., Sancho, E., and Batlle, E. (2022). Overcoming TGFβ-mediated immune evasion in cancer. Nat. Rev. Cancer 22, 25–44. doi:10.1038/s41568-021-00413-6
Tempfer, H., Kaser-Eichberger, A., Lehner, C., Gehwolf, R., Korntner, S., Kunkel, N., et al. (2018). Bevacizumab improves achilles tendon repair in a rat model. Cell Physiol. Biochem. 46, 1148–1158. doi:10.1159/000489057
Tempfer, H., Spitzer, G., Lehner, C., Wagner, A., Gehwolf, R., Fierlbeck, J., et al. (2022). VEGF-D-mediated signaling in tendon cells is involved in degenerative processes. FASEB J. 36, e22126. doi:10.1096/fj.202100773rrr
Tempfer, H., and Traweger, A. (2015). Tendon vasculature in health and disease. Front. Physiol. 6, 330. doi:10.3389/fphys.2015.00330
Theodossiou, S. K., Tokle, J., and Schiele, N. R. (2019). TGFβ2-induced tenogenesis impacts cadherin and connexin cell-cell junction proteins in mesenchymal stem cells. Biochem. Biophys. Res. Commun. 508, 889–893. doi:10.1016/j.bbrc.2018.12.023
Thermann, H., Fischer, R., Gougoulias, N., Cipollaro, L., and Maffulli, N. (2020). Endoscopic debridement for non-insertional Achilles tendinopathy with and without platelet-rich plasma. J. Sport Health Sci. 12, 275–280. doi:10.1016/j.jshs.2020.06.012
Titan, A. L., Foster, D. S., Chang, J., and Longaker, M. T. (2019). Flexor tendon: development, healing, adhesion formation, and contributing growth factors. Plast. Reconstr. Surg. 144, 639e–647e. doi:10.1097/prs.0000000000006048
Tramer, J. S., Khalil, L. S., Buckley, P., Ziedas, A., Kolowich, P. A., and Okoroha, K. R. (2021). Effect of achilles tendon rupture on player performance and longevity in women's national basketball association players. Orthop. J. Sports Med. 9, 232596712198998. doi:10.1177/2325967121989982
Tsiapalis, D., Kearns, S., Kelly, J. L., and Zeugolis, D. I. (2021). Growth factor and macromolecular crowding supplementation in human tenocyte culture. Biomater. Biosyst. 1, 100009. doi:10.1016/j.bbiosy.2021.100009
Uyar, I., Altuntas, Z., Findik, S., Yildirim, M. E. C., Yarar, S., Aktan, M., et al. (2022). The effects of a combination treatment with mesenchymal stem cell and platelet-rich plasma on tendon healing: an experimental study. Turk J. Med. Sci. 52, 237–247. doi:10.3906/sag-2105-145
Uysal, C. A., Tobita, M., Hyakusoku, H., and Mizuno, H. (2012). Adipose-derived stem cells enhance primary tendon repair: biomechanical and immunohistochemical evaluation. J. Plast. Reconstr. Aesthet. Surg. 65, 1712–1719. doi:10.1016/j.bjps.2012.06.011
Veronesi, F., Torricelli, P., Della Bella, E., Pagani, S., and Fini, M. (2015). In vitro mutual interaction between tenocytes and adipose-derived mesenchymal stromal cells. Cytotherapy 17, 215–223. doi:10.1016/j.jcyt.2014.10.006
Waggett, A. D., Ralphs, J. R., Kwan, A. P., Woodnutt, D., and Benjamin, M. (1998). Characterization of collagens and proteoglycans at the insertion of the human Achilles tendon. Matrix Biol. 16, 457–470. doi:10.1016/s0945-053x(98)90017-8
Walia, B., and Huang, A. H. (2019). Tendon stem progenitor cells: understanding the biology to inform therapeutic strategies for tendon repair. J. Orthop. Res. 37, 1270–1280. doi:10.1002/jor.24156
Wang, D., Jiang, X., Lu, A., Tu, M., Huang, W., and Huang, P. (2018a). BMP14 induces tenogenic differentiation of bone marrow mesenchymal stem cells in vitro. Exp. Ther. Med. 16, 1165–1174. doi:10.3892/etm.2018.6293
Wang, H., Yu, R., Wang, M., Wang, S., Ouyang, X., Yan, Z., et al. (2023). Insulin-like growth factor binding protein 4 loaded electrospun membrane ameliorating tendon injury by promoting retention of IGF-1. J. Control Release 356, 162–174. doi:10.1016/j.jconrel.2023.02.039
Wang, L., Yang, T., Ding, L., Ye, X., and Wu, L. (2022). Platelet-derived growth factor AA-modified electrospun fibers promote tendon healing. J. Biomater. Appl. 37 (6), 1018-1028. doi:10.1177/08853282221139274
Wang, X., Li, F., Xie, L., Crane, J., Zhen, G., Mishina, Y., et al. (2018b). Inhibition of overactive TGF-beta attenuates progression of heterotopic ossification in mice. Nat. Commun. 9, 551. doi:10.1038/s41467-018-02988-5
Wasniewska, A., Olewnik, L., and Polguj, M. (2022). Morphometric profile in foetuses and evolution of Achilles tendon. Folia Morphol. Warsz. 81, 144–149. doi:10.5603/fm.a2021.0013
Wee, J., Kim, H., Shin, S. J., Lee, T., and Lee, S. Y. (2022). Influence of mechanical and TGF-β3 stimulation on the tenogenic differentiation of tonsil-derived mesenchymal stem cells. BMC Mol. Cell Biol. 23, 3. doi:10.1186/s12860-021-00400-7
Wei, B., Ji, M., Lin, Y., Geng, R., Wang, Q., and Lu, J. (2023). Investigation of the medium-term effect of osteoprotegerin/bone morphogenetic protein 2 combining with collagen sponges on tendon-bone healing in a rabbit. J. Orthop. Surg. Hong. Kong) 31, 102255362311634. doi:10.1177/10225536231163467
Wei, X., Mao, Z., Hou, Y., Lin, L., Xue, T., Chen, L., et al. (2011). Local administration of TGFβ-1/VEGF165 gene-transduced bone mesenchymal stem cells for Achilles allograft replacement of the anterior cruciate ligament in rabbits. Biochem. Biophys. Res. Commun. 406, 204–210. doi:10.1016/j.bbrc.2011.02.015
Winnicki, K., Ochala-Klos, A., Rutowicz, B., Pekala, P. A., and Tomaszewski, K. A. (2020). Functional anatomy, histology and biomechanics of the human Achilles tendon - a comprehensive review. Ann. Anat. 229, 151461. doi:10.1016/j.aanat.2020.151461
Wolff, K. S., Wibmer, A. G., Binder, H., Grissmann, T., Heinrich, K., Schauer, S., et al. (2012). The avascular plane of the achilles tendon: A quantitative anatomic and angiographic approach and a base for a possible new treatment option after rupture. Eur. J. Radiol. 81, 1211–1215. doi:10.1016/j.ejrad.2011.03.015
Wu, L. M., Wang, J. K., Liu, J., Fan, C. C., Wang, Y. J., and Xiong, Y. (2021). Gait analysis combined with the expression of TGF-β1, TGF-β3 and CREB during Achilles tendon healing in rat. Chin. J. Traumatol. 24, 360–367. doi:10.1016/j.cjtee.2021.10.002
Xiang, X., Leng, Q., Tang, Y., Wang, L., Huang, J., Zhang, Y., et al. (2018). Ultrasound-targeted microbubble destruction delivery of insulin-like growth factor 1 cDNA and transforming growth factor beta short hairpin RNA enhances tendon regeneration and inhibits scar formation in vivo. Hum. Gene Ther. Clin. Dev. 29, 198–213. doi:10.1089/humc.2018.121
Xiao, H., Chen, Y., Li, M., Shi, Q., Xu, Y., Hu, J., et al. (2021). Cell-free book-shaped decellularized tendon matrix graft capable of controlled release of BMP-12 to improve tendon healing in a rat model. Am. J. Sports Med. 49, 1333–1347. doi:10.1177/0363546521994555
Xie, Y., Su, N., Yang, J., Tan, Q., Huang, S., Jin, M., et al. (2020). FGF/FGFR signaling in health and disease. Signal Transduct. Target Ther. 5, 181. doi:10.1038/s41392-020-00222-7
Xu, S. Y., Li, S. F., and Ni, G. X. (2016). Strenuous treadmill running induces a chondrocyte phenotype in rat achilles tendons. Med. Sci. Monit. 22, 3705–3712. doi:10.12659/msm.897726
Xu, Z., He, Z., Shu, L., Li, X., Ma, M., and Ye, C. (2021). Intra-Articular platelet-rich plasma combined with hyaluronic acid injection for knee osteoarthritis is superior to platelet-rich plasma or hyaluronic acid alone in inhibiting inflammation and improving pain and function. Arthroscopy 37, 903–915. doi:10.1016/j.arthro.2020.10.013
Yan, R., Gu, Y., Ran, J., Hu, Y., Zheng, Z., Zeng, M., et al. (2017). Intratendon delivery of leukocyte-poor platelet-rich plasma improves healing compared with leukocyte-rich platelet-rich plasma in a rabbit achilles tendinopathy model. Am. J. Sports Med. 45, 1909–1920. doi:10.1177/0363546517694357
Ye, T., Chen, Z., Zhang, J., Luo, L., Gao, R., Gong, L., et al. (2023). Large extracellular vesicles secreted by human iPSC-derived MSCs ameliorate tendinopathy via regulating macrophage heterogeneity. Bioact. Mater 21, 194–208. doi:10.1016/j.bioactmat.2022.08.007
Ye, Y. J., Zhou, Y. Q., Jing, Z. Y., Liu, Y. Y., and Yin, D. C. (2018). Electrospun heparin-loaded core-shell nanofiber sutures for achilles tendon regeneration in vivo. Macromol. Biosci. 18, e1800041. doi:10.1002/mabi.201800041
Yoshimoto, Y., Uezumi, A., Ikemoto-Uezumi, M., Tanaka, K., Yu, X., Kurosawa, T., et al. (2022). Tenogenic induction from induced pluripotent stem cells unveils the trajectory towards tenocyte differentiation. Front. Cell Dev. Biol. 10, 780038. doi:10.3389/fcell.2022.780038
You, T., Yuan, S., Bai, L., Zhang, X., Chen, P., and Zhang, W. (2020). Benzyl alcohol accelerates recovery from Achilles tendon injury, potentially via TGF-β1/Smad2/3 pathway. Injury 51, 1515–1521. doi:10.1016/j.injury.2020.03.058
Younesi Soltani, F., Javanshir, S., Dowlati, G., Parham, A., and Naderi-Meshkin, H. (2022). Differentiation of human adipose-derived mesenchymal stem cells toward tenocyte by platelet-derived growth factor-BB and growth differentiation factor-6. Cell Tissue Bank. 23, 237–246. doi:10.1007/s10561-021-09935-7
Yu, D., Ju, J., Xue, F., Zhao, Y., Shi, W., and Xiao, H. (2021a). Expression and significance of related genes in the early stage of post-traumatic heterotopic ossification in a rat model of Achilles tenotomy. Acta Orthop. Traumatol. Turc 55, 94–101. doi:10.5152/j.aott.2021.18480
Yu, T. Y., Pang, J. S., Lin, L. P., Cheng, J. W., Liu, S. J., and Tsai, W. C. (2021b). Platelet-rich plasma releasate promotes early healing in tendon after acute injury. Orthop. J. Sports Med. 9, 232596712199037. doi:10.1177/2325967121990377
Yu, X., Biedrzycki, A. H., Khalil, A. S., Hess, D., Umhoefer, J. M., Markel, M. D., et al. (2017). Nanostructured mineral coatings stabilize proteins for therapeutic delivery. Adv. Mater 29, 1701255. doi:10.1002/adma.201701255
Yuksel, S., Gulec, M. A., Gultekin, M. Z., Adanir, O., Caglar, A., Beytemur, O., et al. (2016). Comparison of the early period effects of bone marrow-derived mesenchymal stem cells and platelet-rich plasma on the Achilles tendon ruptures in rats. Connect. Tissue Res. 57, 360–373. doi:10.1080/03008207.2016.1189909
Zhang, B., Luo, Q., Deng, B., Morita, Y., Ju, Y., and Song, G. (2018a). Construction of tendon replacement tissue based on collagen sponge and mesenchymal stem cells by coupled mechano-chemical induction and evaluation of its tendon repair abilities. Acta Biomater. 74, 247–259. doi:10.1016/j.actbio.2018.04.047
Zhang, C. H., Jiang, Y. L., Ning, L. J., Li, Q., Fu, W. L., Zhang, Y. J., et al. (2018b). Evaluation of decellularized bovine tendon sheets for achilles tendon defect reconstruction in a rabbit model. Am. J. Sports Med. 46, 2687–2699. doi:10.1177/0363546518787515
Zhang, J., Li, F., Augi, T., Williamson, K. M., Onishi, K., Hogan, M. V., et al. (2021). Platelet HMGB1 in Platelet-Rich Plasma (PRP) promotes tendon wound healing. PLoS One 16, e0251166. doi:10.1371/journal.pone.0251166
Zhao, T., Qi, Y., Xiao, S., Ran, J., Wang, J., Ghamor-Amegavi, E. P., et al. (2019). Integration of mesenchymal stem cell sheet and bFGF-loaded fibrin gel in knitted PLGA scaffolds favorable for tendon repair. J. Mater Chem. B 7, 2201–2211. doi:10.1039/c8tb02759e
Zhou, J. J., Chang, Y. J., Chen, Y. L., Wang, X. D., Liao, Q., Shi, R. H., et al. (2021a). Comparison of myosepta development and transcriptome profiling between blunt snout bream with and Tilapia without intermuscular bones. Biol. (Basel) 10, 1311. doi:10.3390/biology10121311
Zhou, Y., Zhang, L., Zhao, W., Wu, Y., Zhu, C., and Yang, Y. (2013). Nanoparticle-mediated delivery of TGF-β1 miRNA plasmid for preventing flexor tendon adhesion formation. Biomaterials 34, 8269–8278. doi:10.1016/j.biomaterials.2013.07.072
Zhou, Y. L., Yang, Q. Q., Zhang, L., and Tang, J. B. (2021b). Nanoparticle-coated sutures providing sustained growth factor delivery to improve the healing strength of injured tendons. Acta Biomater. 124, 301–314. doi:10.1016/j.actbio.2021.01.008
Keywords: Achilles tendon injury, growth factors, tendon healing, clinical trial, combined application
Citation: Lin M, Li W, Ni X, Sui Y, Li H, Chen X, Lu Y, Jiang M and Wang C (2023) Growth factors in the treatment of Achilles tendon injury. Front. Bioeng. Biotechnol. 11:1250533. doi: 10.3389/fbioe.2023.1250533
Received: 03 July 2023; Accepted: 04 September 2023;
Published: 14 September 2023.
Edited by:
Sibylle Grad, AO Research Institute, SwitzerlandReviewed by:
Mohammad El Khatib, University of Teramo, ItalyBurhan Gharaibeh, University of Pittsburgh, United States
Copyright © 2023 Lin, Li, Ni, Sui, Li, Chen, Lu, Jiang and Wang. This is an open-access article distributed under the terms of the Creative Commons Attribution License (CC BY). The use, distribution or reproduction in other forums is permitted, provided the original author(s) and the copyright owner(s) are credited and that the original publication in this journal is cited, in accordance with accepted academic practice. No use, distribution or reproduction is permitted which does not comply with these terms.
*Correspondence: Chenchao Wang, d2FuZ2NoZW5jaGFvQDEzOS5jb20=; Miao Jiang, amlhbmdtaWFvQGNtdS5lZHUuY24=; Yongping Lu, dmFsZW5zdTQyM0AxNjMuY29t
†These authors have contributed equally to this work and share first authorship