- Department of Nursing Care, Shanghai Songjiang District Central Hospital, Shanghai, China
Introduction: The effective treatment of glioblastoma still remains a great challenge. We herein report the development of chlorin e6 (Ce6)-conjugated iron oxide (Fe3O4-Ce6) nanoparticles for ablation of glioblastoma cells via combining photothermal therapy (PTT) with photodynamic therapy (PDT).
Methods: Ce6 was conjugated to the synthesized Fe3O4 nanoparticles to form Fe3O4-Ce6 nanoparticles displaying the optical property of Ce6.
Results and discussion: Under 808 nm laser irradiation, Fe3O4-Ce6 nanoparticles generated heat and the temperature increase did not have obvious changes after five cycles of laser irradiation, suggesting their good photothermal effect and photothermal stability. In addition, 660 nm laser irradiation of Fe3O4-Ce6 nanoparticles produced singlet oxygen (1O2) to mediate PDT. The Fe3O4-Ce6 nanoparticles without laser irradiation showed a low cytotoxicity, but they would obviously kill C6 cancer cells after laser irradiation via the combinational effect of PTT and PDT. Fe3O4-Ce6 nanoparticles thus could be used as a nanotherapeutic agent for combinational ablation of glioblastoma cells.
1 Introduction
Glioblastoma is the most common primary malignant tumor of the nervous system, accounting for about 40%–50% of all primary intracranial tumors (van Landeghem et al., 2009; Xin et al., 2012; Fang et al., 2015; Pinel et al., 2019). Because of the high degree of malignancy and short overall survival of glioblastoma patients, it is still a great challenge for the treatment of glioblastoma (Kuang et al., 2018; Choi et al., 2020; Gregory et al., 2020; Zhang et al., 2021; Wang et al., 2023). At present, the treatment of glioma is mainly based on surgery, which can be used to resect early small tumors in appropriate locations (Lara-Velazquez et al., 2017; Zhang et al., 2019a; De Witt Hamer et al., 2019). As the tumor grows due to its unclear boundaries, it is difficult to completely remove tumor cells (Wang et al., 2022; Dhar et al., 2022; Sandbhor et al., 2022; Zhang et al., 2023). Therefore, chemotherapy, radiotherapy, and immunotherapy have been used to combine surgery to further delay the progression of the disease and improve survival time (Zhang et al., 2019b; Ruan et al., 2019; Han et al., 2020; Lu et al., 2020; Alghamri et al., 2022; Wang et al., 2022; Li et al., 2022; Sun et al., 2022; Zhang et al., 2022; Zhang et al., 2023). However, due to short-term recurrence and drug resistance, the treatment effects of glioblastoma is not satisfactory.
Phototherapy is a type treatment strategy that relies on the light irradiation of tumors (Xie et al., 2020; Li et al., 2021; Zheng et al., 2021; Lee et al., 2022; Roy et al., 2023a). Compared to traditional chemotherapy, phototherapy shows the advantages of high selectivity, low side effects and negligible drug resistance (Cao et al., 2021; Huang et al., 2021; Pivetta et al., 2021; Feng et al., 2022). Photothermal therapy (PTT) utilizes the generated heat after laser irradiation of photothermal agents to ablate tumor cells (Fernandes et al., 2020; Gao et al., 2021; Lv et al., 2021; Huang et al., 2022). Photodynamic therapy (PDT) produces reactive oxygen species (ROS) to kill cancer cells via activating photosensitizers by light (Chen et al., 2020; Pham et al., 2021; Wan et al., 2021; Roy et al., 2023b). Currently, both PTT and PDT have been widely explored for treatments of different tumors. In addition, the combinations of PTT and PDT can lead to better efficacy for suppressing tumors (Curcio et al., 2019; Zhang et al., 2020; Chen et al., 2021).
In this study, we reported the development of chlorin e6 (Ce6)-conjugated iron oxide (Fe3O4-Ce6) nanoparticles for ablation of glioblastoma cells by PTT-combined PDT. Fe3O4 nanoparticles were first synthesized and their surface modification of Ce6 led to the formation of Fe3O4-Ce6 nanoparticles, in which, Fe3O4 nanoparticles and Ce6 were used as photothermal agents and photosensitizers, respectively. The morphology, hydrodynamic size, zeta potential, absorbance and fluorescence properties of Fe3O4-Ce6 nanoparticles were studied. Under 808 and 660 nm laser irradiation, Fe3O4-Ce6 nanoparticles could mediate PTT and PDT by generating heat and ROS. In addition, they were found to have a good photothermal stability after five cycles of laser irradiation. Via combining PTT and PDT, Fe3O4-Ce6 nanoparticles effectively killed C6 cells under 808 and 660 nm laser irradiation. Thus, Fe3O4-Ce6 nanoparticles could be used for ablation of glioblastoma cells via combinational therapy.
2 Materials and methods
2.1 Materials
Ce6 was purchased from America J&K Scientific Ltd. (United States). Bovine serum albumin (BSA), N-(3-dimethylaminopropyl)-N-ethyl-carbodiimide hydrochloride crystalline (EDC), N-hydroxysuccin-imide (NHS), singlet oxygen sensor green (SOSG), 2′,7′-dichlorodihydrofluorescein diacetate (H2DCFDA) and calcein-AM/propidium iodide (PI) double staining kit were purchased from Sigma Aldrich (United States). FeCl3.6H2O and FeCl2.4H2O were obtained from Aladdin Biochemical Technology Co., Ltd. (Shanghai, China). CCK-8 was purchased from Dojindo Laboratories (Japan). All the other chemicals were purchased from National Pharmaceutical Corporation (Shanghai, China).
2.2 Characterization techniques
Transmission electron microscopy (TEM) images were obtained using Tecnai G2 20 TWIN TEM (FEI, United States). Hydrodynamic sizes and zeta potential values were measured using a Zetasizer (Nano S90, UK). UV-vis absorptions were measured using persee UV-vis spectrophotometer (TU-1810, China). Fluorescence spectra were recorded using fluorescence spectrophotometer (Shimadzu RF-6000, Japan).
2.3 Synthesis of Fe3O4 nanoparticles
FeCl2.4H2O (89.0 mg) and FeCl3.6H2O (157.0 mg) were dissolved in 8.0 mL water, and then 5 mL aqueous solution containing NaOH (1.0 g) and BSA (20.0 mg) was dropped into above solution. The resulted solution was stirred at 80°C for 30 min and black products were formed. Then the solution was cooled to room temperature and the formed products were precipitated by using magnetic beads. After purification through water washing, BSA-coated Fe3O4 nanoparticles were obtained.
2.4 Synthesis of Fe3O4-Ce6 nanoparticles
Ce6 (12.0 mg), EDC (24.6 mg) and NHS (23.0 mg) were dissolved in 5 mL dimethyl sulfoxide and the solution were stirred at room temperature for 3 h. Then above solution was dropped into 5 mL solution of BSA-coated Fe3O4 nanoparticles, and the reaction was contained at room temperature for 24 h. The products were collected using magnetic beads and then further washed with water. After that, Fe3O4-Ce6 nanoparticles were obtained.
2.5 Evaluation of photodynamic efficacy
The solution of Fe3O4-Ce6 nanoparticles were mixed with SOSG, and the resulted solutions were irradiated by 660 nm laser (0.3 W/cm2) for different times. The fluorescence spectra of solutions without or with laser irradiation were recorded. The fluorescence intensities of solutions at 525 nm were used to evaluate the 1O2 generation by calculating the fluorescence enhancement (F/F0).
2.6 Evaluation of photothermal efficacy
The solutions of Fe3O4-Ce6 nanoparticles at different concentrations were irradiated by 808 nm laser (1.0 W/cm2), and the temperatures of solutions under laser irradiation were measured. To evaluate the photothermal stability, the nanoparticle solutions were irradiated by 808 nm laser (1.0 W/cm2) for five times and the temperatures of solutions were measured.
2.7 Evaluation of cell viability
The cell lines (brain endothelial bEnd.3 cells and rat C6 glioma cells) presents in this study were obtained from American Type Culture Collection (ATCC, United States). The bEnd.3 and C6 cancer cells were incubated with Fe3O4-Ce6 nanoparticles at different concentrations for 24 h, and then the cells were washed with PBS. The cells were then incubated in cell culture medium containing CCK-8 agent for 2 h. The supernatant of treated cells was collected to measure the absorbance at 450 nm using a Thermo Scientific Multiskan MK3 ELISA reader (Thermo scientific, United States), and then the cell viabilities were calculated.
2.8 Evaluation of therapeutic efficacy
C6 cancer cells were incubated with Fe3O4-Ce6 nanoparticles for 24 h and then the cells were irradiated by 808 nm laser (1.0 W/cm2) for 5 min and 660 nm laser (0.3 W/cm2) for 5 min. The cells were further incubated for 6 h and then the cell viabilities of cells were measured using CCK-8 analysis.
2.9 Calcein-AM/PI double staining
C6 cancer cells were incubated with Fe3O4-Ce6 nanoparticles for 24 h and then the cells were cultured in cell culture medium containing calcein-AM/PI double staining agent. The 808 nm laser (1.0 W/cm2, 5 min) and 660 nm laser (0.3 W/cm2, 5 min) was used to treat the cells. The fluorescence images of cells in various treatment groups were captured using a fluorescence microscope.
2.10 Intracellular ROS generation evaluation
C6 cancer cells were incubated with Fe3O4-Ce6 nanoparticles for 24 h and then the cells were further cultured in cell culture medium containing H2DCFDA for 30 min. The cells were then irradiated by 660 nm laser (1.0 W/cm2) for 5 min. Fluorescence images of cells in various treatment groups were captured using a fluorescence microscope.
2.11 Cellular uptake evaluation
C6 cancer cells were incubated with Fe3O4-Ce6 nanoparticles at different concentration for 12 h, and then the cells were washed with PBS to remove free nanoparticles. The contents of nanoparticles inside cells were evaluated by measuring intracellular Fe concentration using inductively coupled plasma optical emission spectroscopy (ICP-OES).
2.12 Statistical analysis
The data were provided as mean ± standard deviation (SD). Statistical analysis was carried out using one-way ANOVA statistical analysis. Statistical significance was indicated as (*) p < 0.05, (**) p < 0.01, and (***) p < 0.001.
3 Results and discussion
3.1 Characterization of Fe3O4-Ce6 nanoparticles
TEM image showed that the formed Fe3O4-Ce6 nanoparticles had a spherical morphology and their size distribution was homogeneous (Figure 1A). The hydrodynamic size and zeta potential of Fe3O4-Ce6 nanoparticles was measured to be 80.0 nm and −15.4 mV, respectively (Figure 1B). As shown in UV-vis spectra, the characteristic peaks of Ce6 at 400 nm and 641 nm could be detected in the absorbance spectrum of Fe3O4-Ce6 nanoparticles (Figure 1C), which however could not be detected in absorbance spectrum of Fe3O4 nanoparticles, confirming the conjugation of Ce6 to Fe3O4 nanoparticles. In addition, Fe3O4-Ce6 nanoparticles showed a fluorescence emission at around 670 nm (Figure 1D), and the fluorescence signal was also observed for Ce6. However, Fe3O4 nanoparticles did not have fluorescence property. These results suggested that Fe3O4-Ce6 nanoparticles showed the optical properties of Ce6.
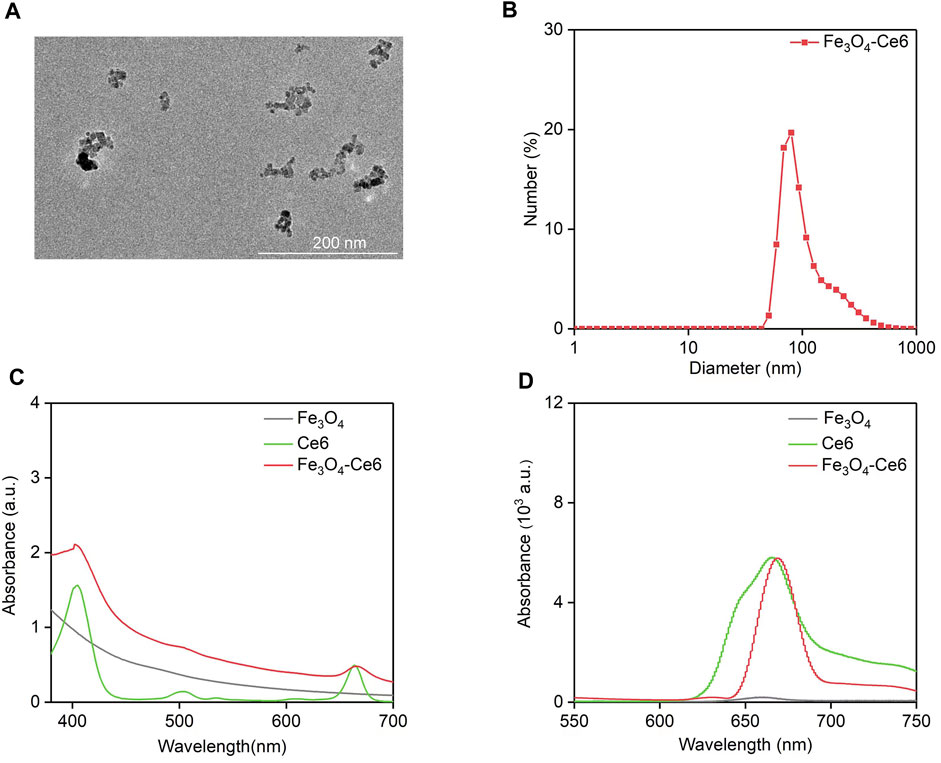
FIGURE 1. Characterization of Fe3O4-Ce6 nanoparticles. (A) TEM image of Fe3O4-Ce6 nanoparticles. (B) Hydrodynamic size of Fe3O4-Ce6 nanoparticles. (C) UV-Vis absorbance spectra of Fe3O4 nanoparticles, Ce6 and Fe3O4-Ce6 nanoparticles. (D) Fluorescence spectra of Fe3O4 nanoparticles, Ce6 and Fe3O4-Ce6 nanoparticles.
3.2 Photothermal property of Fe3O4-Ce6 nanoparticles
The photothermal property of Fe3O4-Ce6 nanoparticles under 808 nm laser irradiation was evaluated. Under laser irradiation, the temperature of solutions containing Fe3O4-Ce6 nanoparticles gradually increased, which reached around 58.8°C after 6 min of laser irradiation (Figure 2A). This result verified the good photothermal property of Fe3O4-Ce6 nanoparticles. The temperature increase for Fe3O4-Ce6 nanoparticles was found to be concentration-dependent, as higher concentration led to a higher temperature (Figure 2B). At the concentration of 500 μg/mL, the temperature increased to 58.8°C after 6 min of laser irradiation. In addition, the temperature increase did not have obvious changes after 5 cycles of laser on and laser off (Figure 2C). These results verified the good photothermal stability of Fe3O4-Ce6 nanoparticles. The good photothermal effect and good photothermal stability were similarly observed for Fe3O4 nanoparticles as reported in a previous study (Chen et al., 2023).
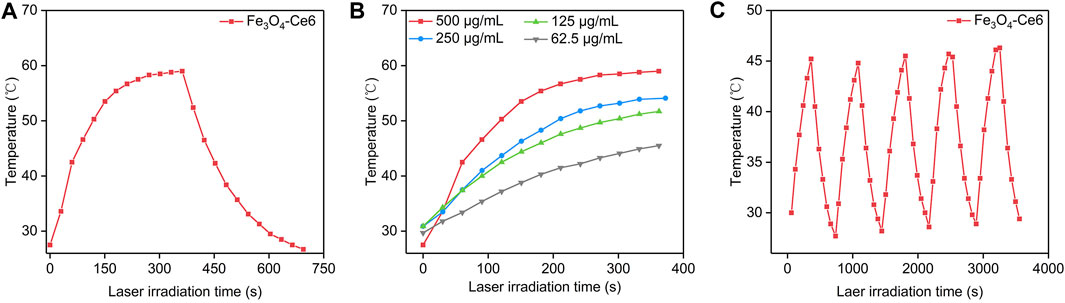
FIGURE 2. Photothermal property of Fe3O4-Ce6 nanoparticles. (A) Temperature changes of Fe3O4-Ce6 nanoparticles under 808 nm laser irradiation for laser on (6 min) and laser off (6 min). (B) Temperature increase for Fe3O4-Ce6 nanoparticles at different concentrations under 808 nm laser irradiation. (C) Temperature changes of Fe3O4-Ce6 nanoparticles after five cycles of laser on and laser off.
3.3 Photodynamic property of Fe3O4-Ce6 nanoparticles
The photodynamic property of Fe3O4-Ce6 nanoparticles was evaluated by measuring the generation of 1O2 under 660 nm laser irradiation using SOSG as the 1O2 probe. The fluorescence intensity of SOSG for solutions containing Fe3O4-Ce6 nanoparticles gradually increased under 660 nm laser irradiation (Figure 3A). This should be because the generated 1O2 turned on the fluorescence signals of SOSG. The fluorescence intensity of SOSG for solutions containing Fe3O4-Ce6 nanoparticles increased by 1.2-, 1.4-, 1.6-, 1.7-, 1.9-, 2.1-, 2.2-, 2.3-, 2.5-, and 2.6-fold after 1, 2, 3, 4, 5, 6, 7, 8, 9, and 10 min of laser irradiation (Figure 3B). These results confirmed the generation of 1O2 via photodynamic effect for Fe3O4-Ce6 nanoparticles under 660 nm laser irradiation. The 1O2 generating efficacy of Fe3O4-Ce6 nanoparticles was higher than that of protoporphyrin IX (PpIX)-modified Fe3O4 nanoparticles (Ding et al., 2022).
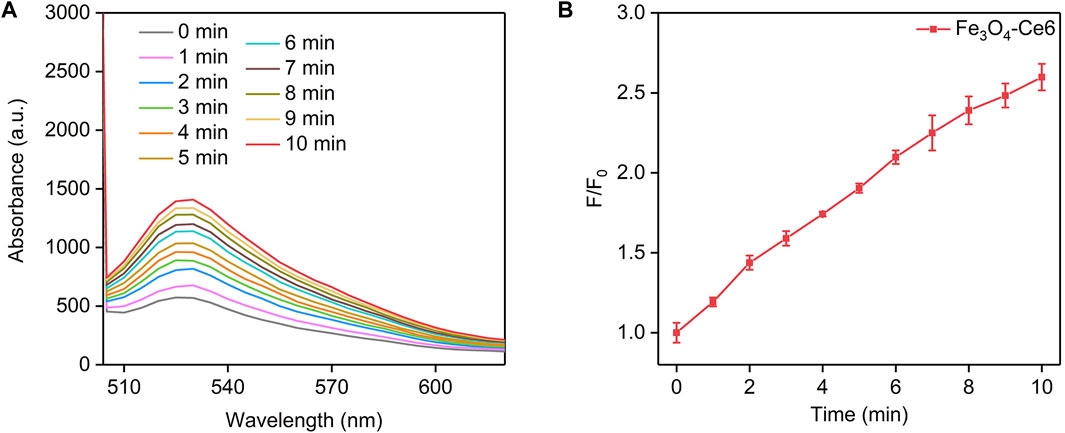
FIGURE 3. Photodynamic property of Fe3O4-Ce6 nanoparticles. (A) Fluorescence spectra of SOSG in solutions containing Fe3O4-Ce6 nanoparticles under 660 nm laser irradiation for different time. (B) Fluorescence changes of SOSG in solutions containing Fe3O4-Ce6 nanoparticles under 660 nm laser irradiation for different time.
3.4 Cell viability and therapeutic efficacy evaluation
To evaluate the cytotoxicity of nanoparticles to normal cells, bEnd.3 cells were incubated with these nanoparticles. After 24 h of incubation, the cell viability did not have obvious decline (Figure 4A). C6 cancer cells were incubated with Fe3O4-Ce6 nanoparticles at different concentrations for 24 h, and the CCK-8 analysis showed that the cell viability of these treated cells was still higher than 85.0% (Figure 4B), which suggested the low cytotoxicity for Fe3O4-Ce6 nanoparticles. To evaluate the in vitro therapeutic efficacy, C6 cells were incubated with Fe3O4-Ce6 nanoparticles and then treated by 808 and 660 nm laser. The cell viability for PBS + laser and Fe3O4-Ce6 nanoparticle-treated groups was similar to that in PBS control group (Figure 4C). These results suggested that laser irradiation and sole Fe3O4-Ce6 nanoparticle treatment did not have obvious therapeutic effect. In contrast, the cell viability of C6 cells in Fe3O4-Ce6 + laser group was only 19.6%, which suggested the good cell killing effect for Fe3O4-Ce6 nanoparticles plus laser irradiation via the combinational effect of PTT and PDT. The therapeutic efficacy of Fe3O4-Ce6 nanoparticles via PTT-combined PDT was higher than that of Fe3O4 nanoparticles via a sole PTT effect (Chen et al., 2023).
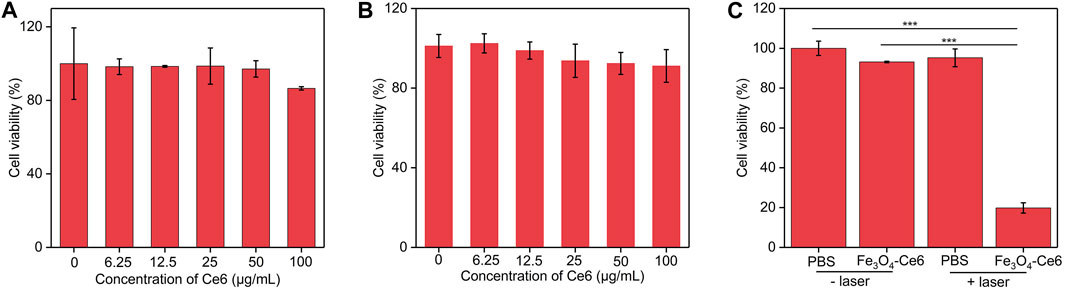
FIGURE 4. Cell viability and therapeutic efficacy evaluation. (A) Cell viability of bEnd.3 cells after incubation with Fe3O4-Ce6 nanoparticles at different concentrations for 24 h (B) Cell viability of C6 cells after incubation with Fe3O4-Ce6 nanoparticles at different concentrations for 24 h (C) Cell viability of C6 cells in PBS, PBS plus laser irradiation, Fe3O4-Ce6 nanoparticle treatment, and Fe3O4-Ce6 nanoparticle treatment plus laser irradiation groups.
3.5 Dead/living staining analysis
Dead/living staining was then used to evaluate the therapeutic efficacy. As shown in the fluorescence images, only green fluorescence signals (living cells) were observed for cells in PBS + laser and Fe3O4-Ce6 nanoparticle-treated groups, which was similar to those in PBS control group (Figure 5A). In contrast, both green and red fluorescence signals could be detected for cells in Fe3O4-Ce6 + laser group. The red fluorescence signals in this group indicated the death of cancer cells after treatment. Quantitative analysis showed that the percentage of dead cells and living cells in Fe3O4-Ce6 + laser group was 83.6% and 16.4%, respectively (Figure 5B). The percentages of living cells in PBS, PBS + laser and Fe3O4-Ce6 nanoparticle-treated groups were around 99.0%. These results further confirmed the good therapeutic efficacy for Fe3O4-Ce6 nanoparticles plus laser irradiation.
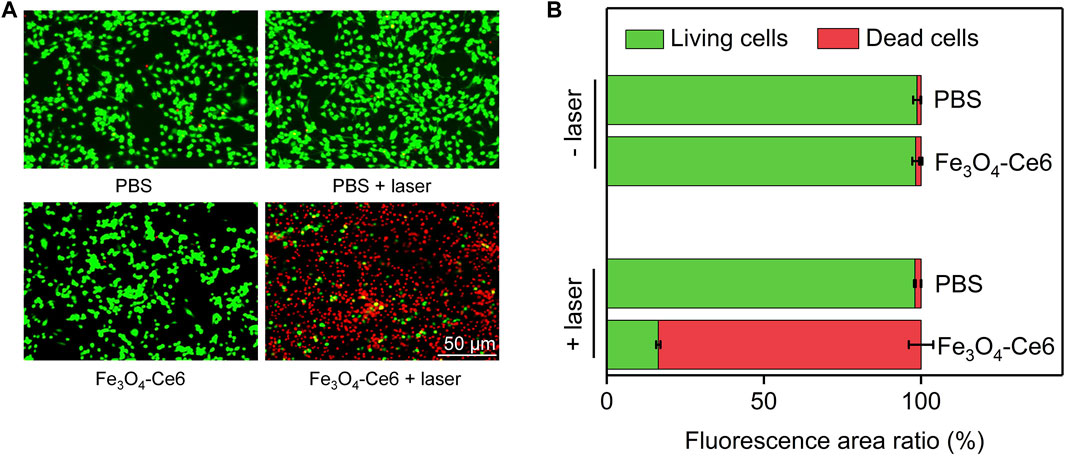
FIGURE 5. Dead/living staining analysis. (A) Dead and living fluorescence staining images of C6 cells after different treatments. (B) Quantitative analysis of the percentages of dead and living cells in different groups.
3.6 Intracellular ROS generation evaluation
To confirm the photodynamic effect, the generation of ROS inside cells after treatments was evaluated using H2DCFHDA as the ROS probe. Obvious green fluorescence signals could be detected in Fe3O4-Ce6 + laser group (Figure 6A), which verified the generation of ROS in this group. However, nearly no green fluorescence signals were observed in PBS and PBS + laser group. The very weak green fluorescence signal in Fe3O4-Ce6 nanoparticle-treated group may be due to the generation of a little ROS via Fenton reaction. The fluorescence intensity of green signals in Fe3O4-Ce6 + laser group was at least 82.0-fold higher than that in the other groups (Figure 6B). These results confirmed the generation of ROS inside cancer cells via photodynamic effect after Fe3O4-Ce6 nanoparticle treatment plus 660 nm laser irradiation.
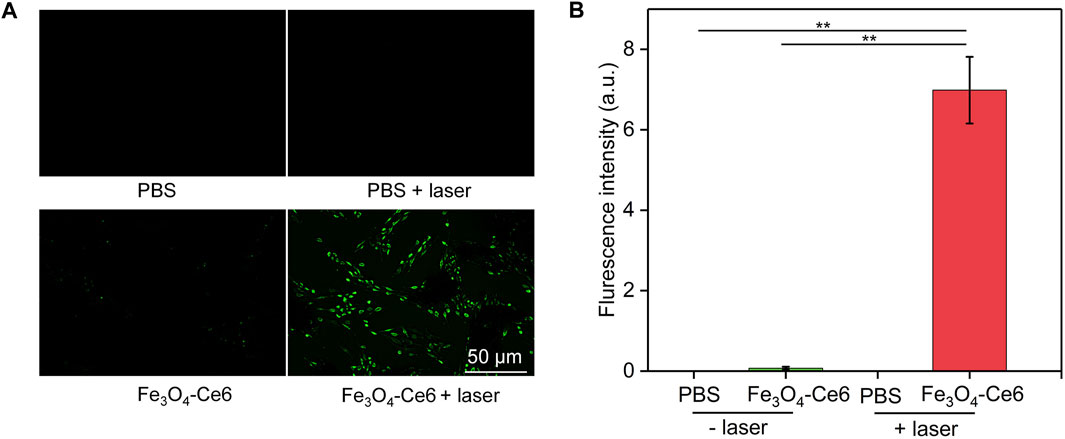
FIGURE 6. Intracellular ROS generation evaluation. (A) Fluorescence images of Ce6 cells in different groups to show the generation of ROS inside cells. (B) Quantitative fluorescence intensity of ROS in different groups.
3.7 Cellular uptake evaluation
The cellular uptake of Fe3O4-Ce6 nanoparticles by C6 cancer cells were investigated. The results showed that the intracellular Fe levels in the treated cells gradually increased in a concentration depend manner (Figure 7). A higher concentration of nanoparticles led to a higher intracellular Fe level. These results confirmed the effective cellular uptake of Fe3O4-Ce6 nanoparticles by C6 cancer cells.
4 Conclusion
We have developed a nanoparticle system containing Ce6 and Fe3O4 nanoparticles for in vitro ablation of glioblastoma cells via combining PTT with PDT. Fe3O4-Ce6 nanoparticles were synthesized through conjugating Ce6 to Fe3O4 nanoparticles that showed negative surface potential and the optical property of Ce6. Fe3O4-Ce6 nanoparticles could mediate PTT and PDT via producing heat and ROS under 808 and 660 nm laser irradiation. The treatment of Fe3O4-Ce6 nanoparticles plus laser irradiation obviously killed cancer cells and reduced the cell viability, which were verified using CCK-8 analysis and living/dead staining. Fluorescence imaging confirmed the generation of ROS inside cancer cells for Fe3O4-Ce6 nanoparticle treatment plus laser irradiation. In view of the good fluorescence property of Fe3O4-Ce6 nanoparticles, they may be used for fluorescence imaging-guided combination therapy of cancer.
Data availability statement
The original contributions presented in the study are included in the article/supplementary material, further inquiries can be directed to the corresponding author.
Author contributions
J-YZ: Corresponding authors, conception, design of the study and revising the manuscript; HY: acquisition, analysis, interpretation of the data, and drafting the article. All authors contributed to the article and approved the submitted version.
Funding
This study was supported by the Fundamental Research Funds in Shanghai Songjiang District Central Hospital.
Conflict of interest
The authors declare that the research was conducted in the absence of any commercial or financial relationships that could be construed as a potential conflict of interest.
Publisher’s note
All claims expressed in this article are solely those of the authors and do not necessarily represent those of their affiliated organizations, or those of the publisher, the editors and the reviewers. Any product that may be evaluated in this article, or claim that may be made by its manufacturer, is not guaranteed or endorsed by the publisher.
References
Alghamri, M. S., Banerjee, K., Mujeeb, A. A., Mauser, A., Taher, A., Thalla, R., et al. (2022). Systemic delivery of an adjuvant CXCR4–CXCL12 signaling inhibitor encapsulated in synthetic protein nanoparticles for glioma immunotherapy. ACS Nano 16, 8729–8750. doi:10.1021/acsnano.1c07492
Cao, S., Shao, J., Wu, H., Song, S., De Martino, M. T., Pijpers, I. A., et al. (2021). Photoactivated nanomotors via aggregation induced emission for enhanced phototherapy. Nat. Commun. 12, 2077. doi:10.1038/s41467-021-22279-w
Chen, J., Fan, T., Xie, Z., Zeng, Q., Xue, P., Zheng, T., et al. (2020). Advances in nanomaterials for photodynamic therapy applications: Status and challenges. Biomaterials 237, 119827. doi:10.1016/j.biomaterials.2020.119827
Chen, S., Lv, Y., Wang, Y., Kong, D., Xia, J., Li, J., et al. (2023). Tumor acidic microenvironment-responsive promodulator iron oxide nanoparticles for photothermal-enhanced chemodynamic immunotherapy of cancer. ACS Biomater. Sci. Eng. 9, 773–783. doi:10.1021/acsbiomaterials.2c01287
Chen, T., Yao, T., Peng, H., Whittaker, A. K., Li, Y., Zhu, S., et al. (2021). An injectable hydrogel for simultaneous photothermal therapy and photodynamic therapy with ultrahigh efficiency based on carbon dots and modified cellulose nanocrystals. Adv. Funct. Mat. 31, 2106079. doi:10.1002/adfm.202106079
Choi, J., Kim, G., Cho, S. B., and Im, H.-J. (2020). Radiosensitizing high-Z metal nanoparticles for enhanced radiotherapy of glioblastoma multiforme. J. Nanobiotechnol. 18, 122. doi:10.1186/s12951-020-00684-5
Curcio, A., Silva, A. K., Cabana, S., Espinosa, A., Baptiste, B., Menguy, N., et al. (2019). Iron oxide nanoflowers@ CuS hybrids for cancer tri-therapy: Interplay of photothermal therapy, magnetic hyperthermia and photodynamic therapy. Theranostics 9, 1288–1302. doi:10.7150/thno.30238
De Witt Hamer, P. C., Ho, V. K., Zwinderman, A. H., Ackermans, L., Ardon, H., Boomstra, S., et al. (2019). Between-hospital variation in mortality and survival after glioblastoma surgery in the Dutch Quality Registry for Neuro Surgery. J. Neuro-Oncol. 144, 313–323. doi:10.1007/s11060-019-03229-5
Dhar, D., Ghosh, S., Das, S., and Chatterjee, J. (2022). A review of recent advances in magnetic nanoparticle-based theranostics of glioblastoma. Nanomedicine 17, 107–132. doi:10.2217/nnm-2021-0348
Ding, M., Fan, Y., Lv, Y., Liu, J., Yu, N., Kong, D., et al. (2022). A prodrug hydrogel with tumor microenvironment and near-infrared light dual-responsive action for synergistic cancer immunotherapy. Acta Biomater. 149, 334–346. doi:10.1016/j.actbio.2022.06.041
Fang, C., Wang, K., Stephen, Z. R., Mu, Q., Kievit, F. M., Chiu, D. T., et al. (2015). Temozolomide nanoparticles for targeted glioblastoma therapy. ACS Appl. Mat. Interfaces 7, 6674–6682. doi:10.1021/am5092165
Feng, L., Li, C., Liu, L., Wang, Z., Chen, Z., Yu, J., et al. (2022). Acceptor planarization and donor rotation: A facile strategy for realizing synergistic cancer phototherapy via type I PDT and PTT. ACS Nano 16, 4162–4174. doi:10.1021/acsnano.1c10019
Fernandes, N., Rodrigues, C. F., Moreira, A. F., and Correia, I. J. (2020). Overview of the application of inorganic nanomaterials in cancer photothermal therapy. Biomater. Sci. 8, 2990–3020. doi:10.1039/d0bm00222d
Gao, G., Sun, X., and Liang, G. (2021). Nanoagent-promoted mild-temperature photothermal therapy for cancer treatment. Adv. Funct. Mat. 31, 2100738. doi:10.1002/adfm.202100738
Gregory, J. V., Kadiyala, P., Doherty, R., Cadena, M., Habeel, S., Ruoslahti, E., et al. (2020). Systemic brain tumor delivery of synthetic protein nanoparticles for glioblastoma therapy. Nat. Commun. 11, 5687. doi:10.1038/s41467-020-19225-7
Han, H., Zhang, Y., Jin, S., Chen, P., Liu, S., Xie, Z., et al. (2020). Paclitaxel-loaded dextran nanoparticles decorated with RVG29 peptide for targeted chemotherapy of glioma: An in vivo study. New J. Chem. 44, 5692–5701. doi:10.1039/c9nj05366b
Huang, H., Ali, A., Liu, Y., Xie, H., Ullah, S., Roy, S., et al. (2022). Advances in image-guided drug delivery for antibacterial therapy. Adv. Drug Deliv. Rev. 192, 114634. doi:10.1016/j.addr.2022.114634
Huang, X., Sun, X., Wang, W., Shen, Q., Shen, Q., Tang, X., et al. (2021). Nanoscale metal-organic frameworks for tumor phototherapy. J. Mat. Chem. B 9, 3756–3777. doi:10.1039/d1tb00349f
Kuang, J., Song, W., Yin, J., Zeng, X., Han, S., Zhao, Y. P., et al. (2018). iRGD modified chemo-immunotherapeutic nanoparticles for enhanced immunotherapy against glioblastoma. Adv. Funct. Mat. 28, 1800025. doi:10.1002/adfm.201800025
Lara-Velazquez, M., Al-Kharboosh, R., Jeanneret, S., Vazquez-Ramos, C., Mahato, D., Tavanaiepour, D., et al. (2017). Advances in brain tumor surgery for glioblastoma in adults. Brain Sci. 7, 166. doi:10.3390/brainsci7120166
Lee, J. S., Kim, J., Ye, Y.-S., and Kim, T.-I. (2022). Materials and device design for advanced phototherapy systems. Adv. Drug Deliv. Rev. 186, 114339. doi:10.1016/j.addr.2022.114339
Li, B., Zhao, S., Huang, L., Wang, Q., Xiao, J., and Lan, M. (2021). Recent advances and prospects of carbon dots in phototherapy. Chem. Eng. J. 408, 127245. doi:10.1016/j.cej.2020.127245
Li, R., Wang, H., Liang, Q., Chen, L., and Ren, J. (2022). Radiotherapy for glioblastoma: Clinical issues and nanotechnology strategies. Biomater. Sci. 10, 892–908. doi:10.1039/d1bm01401c
Lu, V. M., Jue, T. R., and Mcdonald, K. L. (2020). Cytotoxic lanthanum oxide nanoparticles sensitize glioblastoma cells to radiation therapy and temozolomide: An in vitro rationale for translational studies. Sci. Rep. 10, 18156. doi:10.1038/s41598-020-75372-3
Lv, Z., He, S., Wang, Y., and Zhu, X. (2021). Noble metal nanomaterials for NIR-triggered photothermal therapy in cancer. Adv. Healthc. Mat. 10, 2001806. doi:10.1002/adhm.202001806
Pham, T. C., Nguyen, V.-N., Choi, Y., Lee, S., and Yoon, J. (2021). Recent strategies to develop innovative photosensitizers for enhanced photodynamic therapy. Chem. Rev. 121, 13454–13619. doi:10.1021/acs.chemrev.1c00381
Pinel, S., Thomas, N., Boura, C., and Barberi-Heyob, M. (2019). Approaches to physical stimulation of metallic nanoparticles for glioblastoma treatment. Adv. Drug Deliv. Rev. 138, 344–357. doi:10.1016/j.addr.2018.10.013
Pivetta, T. P., Botteon, C. E., Ribeiro, P. A., Marcato, P. D., and Raposo, M. (2021). Nanoparticle systems for cancer phototherapy: An overview. Nanomaterials 11, 3132. doi:10.3390/nano11113132
Roy, S., Bag, N., Bardhan, S., Hasan, I., and Guo, B. (2023a). Recent progress in NIR-II fluorescence imaging-guided drug delivery for cancer theranostics. Adv. Drug Deliv. Rev. 197, 114821. doi:10.1016/j.addr.2023.114821
Roy, S., Hasan, I., and Guo, B. (2023b). Recent advances in nanoparticle-mediated antibacterial applications. Coord. Chem. Rev. 482, 215075. doi:10.1016/j.ccr.2023.215075
Ruan, S., Xie, R., Qin, L., Yu, M., Xiao, W., Hu, C., et al. (2019). Aggregable nanoparticles-enabled chemotherapy and autophagy inhibition combined with anti-PD-L1 antibody for improved glioma treatment. Nano Lett. 19, 8318–8332. doi:10.1021/acs.nanolett.9b03968
Sandbhor, P., Goda, J. S., Mohanty, B., Chaudhari, P., Dutt, S., and Banerjee, R. (2022). Bio-polymeric transferrin-targeted temozolomide nanoparticles in gel for synergistic post-surgical GBM therapy. Nanoscale 14, 12773–12788. doi:10.1039/d2nr00171c
Sun, R., Liu, M., Lu, J., Chu, B., Yang, Y., Song, B., et al. (2022). Bacteria loaded with glucose polymer and photosensitive ICG silicon-nanoparticles for glioblastoma photothermal immunotherapy. Nat. Commun. 13, 5127. doi:10.1038/s41467-022-32837-5
Van Landeghem, F. K., Maier-Hauff, K., Jordan, A., Hoffmann, K.-T., Gneveckow, U., Scholz, R., et al. (2009). Post-mortem studies in glioblastoma patients treated with thermotherapy using magnetic nanoparticles. Biomaterials 30, 52–57. doi:10.1016/j.biomaterials.2008.09.044
Wan, Y., Fu, L. H., Li, C., Lin, J., and Huang, P. (2021). Conquering the hypoxia limitation for photodynamic therapy. Adv. Mat. 33, 2103978. doi:10.1002/adma.202103978
Wang, J., Liu, Y., Liu, F., Gan, S., Roy, S., Hasan, I., et al. (2023). Emerging extracellular vesicle-based carriers for glioblastoma diagnosis and therapy. Nanoscale 15, 10904–10938. doi:10.1039/d3nr01667f
Wang, X., Ye, L., He, W., Teng, C., Sun, S., Lu, H., et al. (2022a). In situ targeting nanoparticles-hydrogel hybrid system for combined chemo-immunotherapy of glioma. J. Control. Release 345, 786–797. doi:10.1016/j.jconrel.2022.03.050
Wang, Z., Zhang, M., Chi, S., Zhu, M., Wang, C., and Liu, Z. (2022b). Brain tumor cell membrane-coated lanthanide-doped nanoparticles for NIR-IIb luminescence imaging and surgical navigation of glioma. Adv. Healthc. Mat. 11, 2200521. doi:10.1002/adhm.202200521
Xie, Z., Fan, T., An, J., Choi, W., Duo, Y., Ge, Y., et al. (2020). Emerging combination strategies with phototherapy in cancer nanomedicine. Chem. Soc. Rev. 49, 8065–8087. doi:10.1039/d0cs00215a
Xin, H., Sha, X., Jiang, X., Zhang, W., Chen, L., and Fang, X. (2012). Anti-glioblastoma efficacy and safety of paclitaxel-loading Angiopep-conjugated dual targeting PEG-PCL nanoparticles. Biomaterials 33, 8167–8176. doi:10.1016/j.biomaterials.2012.07.046
Zhang, H., Wang, R., Yu, Y., Liu, J., Luo, T., and Fan, F. (2019a). Glioblastoma treatment modalities besides surgery. J. Cancer 10, 4793–4806. doi:10.7150/jca.32475
Zhang, H., Wang, T., Liu, H., Ren, F., Qiu, W., Sun, Q., et al. (2019b). Second near-infrared photodynamic therapy and chemotherapy of orthotopic malignant glioblastoma with ultra-small Cu2-xSe nanoparticles. Nanoscale 11, 7600–7608. doi:10.1039/c9nr01789e
Zhang, L., Liu, Y., Huang, H., Xie, H., Zhang, B., Xia, W., et al. (2022). Multifunctional nanotheranostics for near infrared optical imaging-guided treatment of brain tumors. Adv. Drug Deliv. Rev. 190, 114536. doi:10.1016/j.addr.2022.114536
Zhang, P., Rashidi, A., Zhao, J., Silvers, C., Wang, H., Castro, B., et al. (2023). STING agonist-loaded, CD47/PD-L1-targeting nanoparticles potentiate antitumor immunity and radiotherapy for glioblastoma. Nat. Commun. 14, 1610. doi:10.1038/s41467-023-37328-9
Zhang, Y., Cheng, Y., Yang, F., Yuan, Z., Wei, W., Lu, H., et al. (2020). Near-infrared triggered Ti3C2/g-C3N4 heterostructure for mitochondria-targeting multimode photodynamic therapy combined photothermal therapy. Nano Today 34, 100919. doi:10.1016/j.nantod.2020.100919
Zhang, Y., Xi, K., Fu, X., Sun, H., Wang, H., Yu, D., et al. (2021). Versatile metal-phenolic network nanoparticles for multitargeted combination therapy and magnetic resonance tracing in glioblastoma. Biomaterials 278, 121163. doi:10.1016/j.biomaterials.2021.121163
Keywords: glioblastoma, iron oxide nanoparticles, photothermal therapy, photodynamic therapy, cancer therapy
Citation: Yao H and Zhou J-Y (2023) Chlorin e6-modified iron oxide nanoparticles for photothermal-photodynamic ablation of glioblastoma cells. Front. Bioeng. Biotechnol. 11:1248283. doi: 10.3389/fbioe.2023.1248283
Received: 27 June 2023; Accepted: 10 July 2023;
Published: 19 July 2023.
Edited by:
Chi Zhang, Nanyang Technological University, SingaporeReviewed by:
Xiaojun Zhou, Donghua University, ChinaBing Guo, Harbin Institute of Technology, China
Xiuhui Wang, Shanghai University, China
Copyright © 2023 Yao and Zhou. This is an open-access article distributed under the terms of the Creative Commons Attribution License (CC BY). The use, distribution or reproduction in other forums is permitted, provided the original author(s) and the copyright owner(s) are credited and that the original publication in this journal is cited, in accordance with accepted academic practice. No use, distribution or reproduction is permitted which does not comply with these terms.
*Correspondence: Jian-Ying Zhou, emp5c2oxMjM0NTZAMTYzLmNvbQ==