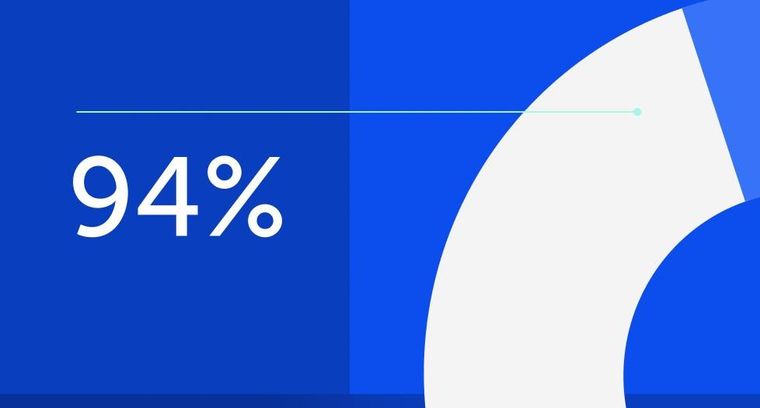
94% of researchers rate our articles as excellent or good
Learn more about the work of our research integrity team to safeguard the quality of each article we publish.
Find out more
ORIGINAL RESEARCH article
Front. Bioeng. Biotechnol., 07 December 2023
Sec. Biomechanics
Volume 11 - 2023 | https://doi.org/10.3389/fbioe.2023.1242166
This article is part of the Research TopicOcular Biomechanics in Health and PathophysiologyView all 18 articles
Introduction: The role of ocular rigidity and biomechanics remains incompletely understood in glaucoma, including assessing an individual’s sensitivity to intraocular pressure (IOP). In this regard, the clinical assessment of ocular biomechanics represents an important need. The purpose of this study was to determine a possible relationship between the G661R missense mutation in the ADAMTS10 gene and the ocular pulse amplitude (OPA), the difference between diastolic and systolic intraocular pressure (IOP), in a well-established canine model of open-angle glaucoma (OAG).
Methods: Animals studied included 39 ADAMTS10-mutant dogs with different stages of OAG and 14 unaffected control male and female dogs between 6 months and 12 years (median: 3.2 years). Dogs were sedated intravenously with butorphanol tartrate and midazolam HCl, and their IOPs were measured with the Icare® Tonovet rebound tonometer. The Reichert Model 30™ Pneumotonometer was used to measure OPA. Central corneal thickness (CCT) was measured via Accutome® PachPen, and A-scan biometry was assessed with DGH Technology Scanmate. All outcome measures of left and right eyes were averaged for each dog. Data analysis was conducted with ANOVA, ANCOVA, and regression models.
Results: ADAMTS10-OAG-affected dogs displayed a greater IOP of 23.0 ± 7.0 mmHg (mean ± SD) compared to 15.3 ± 3.6 mmHg in normal dogs (p < 0.0001). Mutant dogs had a significantly lower OPA of 4.1 ± 2.0 mmHg compared to 6.5 ± 2.8 mmHg of normal dogs (p < 0.01). There was no significant age effect, but OPA was correlated with IOP in ADAMTS10-mutant dogs.
Conclusion: The lower OPA in ADAMTS10-mutant dogs corresponds to the previously documented weaker and biochemically distinct posterior sclera, but a direct relationship remains to be confirmed. The OPA may be a valuable clinical tool to assess ocular stiffness and an individual’s susceptibility to IOP elevation.
Glaucoma is a common, incurable neurodegenerative disease and the leading cause of irreversible blindness worldwide, affecting over 70 million people (Quigley and Broman, 2006). Open-angle glaucoma (OAG) and angle-closure glaucoma are the two primary disease types, depending on the particular abnormalities of the aqueous humor outflow pathways within the iridocorneal angle that increase intraocular pressure (IOP) (Weinreb et al., 2014; Jonas et al., 2017). The pathogenic mechanisms of glaucomatous optic neuropathy leading to retinal ganglion cell (RGC) death and vision loss remain only partly understood. The three primary risk factors are advancing age, genetics, and IOP-related biomechanical stress on the optic nerve head (ONH) (Weinreb et al., 2016; Jonas et al., 2017). Current glaucoma treatment is limited to lowering IOP by medical and surgical means (Weinreb et al., 2014; Jonas et al., 2017).
Even though the prevalence of glaucoma increases with IOP elevation, glaucomatous ONH damage can develop at any IOP level based on significant interindividual variabilities in susceptibility (Sommer et al., 1991; Weinreb et al., 2016). At diagnosis, approximately half of primary OAG (POAG) patients have normal IOP between 10–20 mmHg (Collaborative Normal-Tension Glaucoma Study Group, 1998a; Koz et al., 2009; Weinreb et al., 2016). Nevertheless, IOP-related biomechanical stress is important in these normal tension glaucoma (NTG) patients because a 30% IOP reduction significantly slowed disease progression (Collaborative Normal-Tension Glaucoma Study Group, 1998b). In contrast, individuals with ocular hypertension (OHT) can tolerate increased IOP >21 mmHg without ONH damage (Weinreb et al., 2016). Susceptibility to IOP may be based on connective tissue properties of the fibrous tunic of the globe consisting of cornea, sclera, and lamina cribrosa of the ONH (Bellezza et al., 2000; Burgoyne et al., 2005; Downs et al., 2009; Burgoyne, 2011; Park and Komaromy, 2021).
The biomechanical properties of the eye have been studied in great detail in the laboratory setting using human cadaver eyes and animal models (Coudrillier et al., 2016; Hopkins et al., 2020; Park and Komaromy, 2021; Safa et al., 2022). Clinical measurements of connective tissue properties in human glaucoma eyes are limited to the cornea and include central corneal thickness (CTT) and corneal hysteresis by use of Ocular Response Analyzer® (ORA) (Gordon et al., 2002; Medeiros et al., 2013; Ayyalasomayajula et al., 2016; Weinreb et al., 2016). These measurements exclude the posterior pole of the globe, including the peripapillary sclera (PPS) and the lamina cribrosa, whose biomechanical factors likely impact the ONH’s IOP susceptibility (Downs et al., 2009; Burgoyne, 2011; Safa et al., 2022). There is a critical need for additional clinical tools to measure ocular biomechanics that are not limited to the cornea to evaluate susceptibility to IOP in patients.
In this study, we tested the hypothesis that the measurement of ocular pulse amplitude (OPA) may allow the comparison of biomechanical properties of the entire fibrous tunic in vivo. The OPA is defined as the IOP difference between systole and diastole of the pulsatile choroidal blood flow, and appears to be correlated with ocular rigidity based on observations in glaucoma patients (Tonjum, 1972; Dastiridou et al., 2009). We took advantage of a well-defined, clinically relevant canine glaucoma model, the OAG-affected Beagle, with a G661R missense mutation in the ADAMTS10 gene (Kuchtey et al., 2011). Differences in IOP susceptibilities are well recognized by veterinary ophthalmologists when comparing glaucoma-affected dog breeds (Park and Komaromy, 2021). For example, Beagles with ADAMTS10-OAG tolerate elevated IOP much better, with slower progression of vision loss, than other glaucoma-affected breeds with comparable IOPs (Kuchtey et al., 2011; Park and Komaromy, 2021). Compared to normal dogs, the posterior scleral of ADAMTS10-mutant Beagles is biochemically distinct and weaker with a lower normalized ocular rigidity (Palko et al., 2013; Boote et al., 2016; Palko et al., 2016). This study shows a significantly smaller OPA in ADAMTS10-mutants whose sclera is softer than the normal, supporting the potential clinical value of OPA as a measure of glaucoma susceptibility.
A total of 101 eyes of 53 purpose-bred dogs (both eyes of 48 dogs and one eye of 5 dogs each), 39 at different stages of ADAMTS10-OAG, and 14 normal, glaucoma-unaffected dogs were used. Twenty-six males and 27 females were included between the ages of 6 months and 12 years (median: 3.2 years) (Figure 1). In addition, a separate independent experiment was performed to determine the repeatability of OPA measurements in dogs: A total of 34 eyes of 18 purpose-bred dogs (both eyes of 16 dogs and one eye of 2 dogs each), 14 at different stages of ADAMTS10-OAG, and 4 normal, glaucoma-unaffected dogs were used. Ten males and 8 females were included between the ages of 1–8 years (median: 2.8 years).
FIGURE 1. Age distribution. This histogram compares the age distribution for normal and ADAMTS10-mutant dogs enrolled in the study.
Genotypes were confirmed based on the ADAMTS10 gene sequence: glaucomatous dogs were homozygous for the G661R missense mutation, which is responsible for OAG in Beagles, while the normal dogs were either carriers of the mutation or homozygous for the wildtype allele (Kuchtey et al., 2011). Glaucomatous dogs that received pressure-lowering medication were excluded from this study. The dogs were group-housed in the same environment at the Michigan State University College of Veterinary Medicine with a 12 h/12 h light/dark cycle and fed the same diet.
Dogs were sedated intravenously with a combination of 0.3 mg/kg butorphanol tartrate (Bayer Healthcare LLC, Animal Health Division, Shawnee Mission, KS, United States) and 0.3 mg/kg midazolam HCl (Avet Pharmaceuticals Inc., East Brunswick, NJ, United States). Dogs were placed in a lateral recumbent position for blood pressure measurements. Systolic, diastolic, and mean arterial blood pressures were measured with Mindray Passport® 12 Patient Monitor and blood pressure cuff (Mindray, Shenzhen Mindray Bio-Medical Electronics Co., Ltd., Nanshan, Shenzhen, Guangdong Province, China) placed over the left or right dorsal pedal artery. Intraocular pressure was measured manually via iCare TonoVet® rebound tonometer (Icare Finland Oy, Vantaa, Finland) while dogs were in a seated position. Mean perfusion pressure (PP) was calculated as the difference between mean arterial blood pressure and IOP, and vascular pulse amplitude (VPA) as the difference between systolic and diastolic blood pressure.
The left or right eye was randomly selected for every dog to begin measurements for OPA. Dogs were placed in sternal position for pneumotonometer measurements. Ocular surface anesthesia was achieved by administering one drop of proparacaine HCl 0.5% ophthalmic solution (Akorn, Inc., Lake Forest, IL, United States). The Reichert Model 30™ Pneumotonometer (Reichert, Inc., Depew, NY, United States) was used to measure OPA as well as provide additional measurements of IOP, a deviation index, and pulsation rate (Figure 2A). Measurements were recorded by placing the pneumotonometer probe tip on the central cornea following manufacturer guidelines (Figure 2B). The probe tip contains a small (5-mm diameter) fenestrated membrane that permits air to flow through vents in the tip until it conforms to the shape of the cornea. Once the force of pressure being applied to the cornea is equal to the force of pressure in the anterior chamber, the pneumatic sensor charts and records both IOP and an ocular pulse waveform (Figure 2C). Each dog went through this measurement process twice, once to become acclimated to the probe and noise of the machine and a second time for data collection. The data were recorded and printed via the pneumotonometer’s integrated chart strip printout.
FIGURE 2. Equipment and setup for OPA measurements. (A) The Reichert Model 30™ Pneumotonometer. (B) Placement of pneumotonometer probe on central corneal. (C) Chart produced by Reichert Model 30™ Pneumotonometer documenting measurements recorded.
Lastly, dogs received one drop of OptixCare® Plus Eye Lube (CLC MEDICA, Ontario, Canada) and were placed in a seated position for central corneal thickness (CCT) measurements taken via PachPen® handheld pachymeter (Accutome, Inc., Malvern, PA, USA). Dogs were also in a seated position while the axial length (AXL) of the eye was measured via Scanmate A DGH 6000 A-scan (DGH Technology, Inc., Exton, PA, United States).
Archival corneal diameter (CD) was available for 34/101 eyes (34/53 dogs). Measurements occurred using Jameson calipers (Integra Miltex, York, PA, United States). Estimated cumulative IOP (cumIOP) data were available for 81/101 eyes (43/53 dogs): Daily averages of archival diurnal IOPs measured with the iCare TonoVet® rebound tonometer were plotted as a function of time in days. Areas under the curve were calculated to obtain estimates of cumIOPs (mmHg x days). Since IOP measurements were not performed continuously, the cumIOP data are considered estimates.
A separate experiment was performed to determine the repeatability of OPA measurements in dogs. The same experimental setup was used as described for the main experiment. The left or right eye was randomly selected for every dog to begin measurements for OPA. Each of the 18 dogs went through the measurement process twice. The median interval between the first and second measurements was 19 min (range: 6–22 min).
All outcome measures of left and right eyes were averaged for each dog. Data were analyzed by descriptive statistics, analysis of variance (ANOVA), regression analysis, and mixed effects models using R. ANOVA models were used to assess the effect of mutation on OPA and the effect of mutation by age on OPA. A linear model was applied to determine the impact of age on OPA. An analysis of covariance (ANCOVA) was used to analyze the effect of mutation by age on OPA with the following control variables: IOP, PP, AXL, CCT, VPA, CD, and cumIOP. These analyses were performed for all dogs combined as well as by excluding young adult dogs <12 months of age. Possible relationships between variables were evaluated by bivariate correlation analysis for all dogs combined but also separately for normal and ADAMTS10-mutant dogs. Pearson correlation coefficient was calculated between age and AXL for the normal and ADAMTS10-mutant groups. A Bland-Altman analysis was used to interpret the repeatability data.
Intraocular pressure measured by iCare TonoVet® rebound tonometer was used for all the analyses. Even though not the primary purpose of this study, IOPs measured by iCare TonoVet® and Reichert Model 30™ Pneumotonometer were compared with Bland-Altman plots and by regression analysis without averaging left and right eyes (Prism 9; GraphPad Software, Boston, MA).
Ocular pulse amplitude could be easily measured in lightly sedated dogs. Because there was a significant correlation between left and right eyes on OPA (r = 0.42, 95% CI [0.16, 0.63], p = 0.003), outcome measures were averaged for each dog. Testing by ANOVA revealed that OPA was significantly lower in ADAMTS10-mutant dogs (4.1 ± 2.0 mmHg; mean ± standard deviation) compared to normal control dogs (6.5 ± 2.8 mmHg; p < 0.01; Figure 3A). When controlling for the following variables, the mutation effect became even more significant (p < 0.001): age, mutation by age, IOP, cumIOP, PP, AXL, CCT, CD, and gender. With 39 ADAMTS10-mutant and 14 normal dogs, a desired 0.05 type I error, and a power of 0.80 the detectable effect size was considered reasonable with 0.89 standard deviations (SD). When excluding the younger dogs <12 months, the same trend remained, although the difference in OPA was no longer significant (mutant vs. normal: 4.9 ± 2.2 mmHg vs. 6.5 ± 3.0 mmHg; p = 0.05; Figure 3B). In a separate repeatability study, the Bland-Altman plot showed good agreement between first and second OPA measurements (Figure 4).
FIGURE 3. Box plots summarizing the OPAs of ADAMTS10-mutant and normal dogs. (A) The mutant group had a significantly smaller OPA than the normal control group (p < 0.01). (B) When excluding the younger dogs <12 months, the same trend remained, but the difference was no longer significant (p = 0.05). The boxes represent the middle 50% of the data, with the centerline being the median. The bottom and top whiskers show the first and third quartiles, respectively. Outliers are shown as small black dots. The larger black dots inside the boxes represent the means.
FIGURE 4. Repeatability of canine OPA measurements. The Bland-Altman plot compares first and second measurements in a group of 34 eyes of 18 dogs (median time interval: 19 min, range: 6–22 min). The estimated means of the differences between the two measurements are shown by the green horizontal line. The 95% CI is marked by the red lines representing the upper (ULA) and lower (LLA) limits of agreement (mean ± 1.96 SD). Overall, the OPA measurements did not follow any trends and were mainly located within the 95% CI.
As expected, IOPs were significantly higher in mutant vs. normal dogs (23.0 ± 7.0 mmHg vs. 15.3 ± 3.6 mmHg; p < 0.0001), but PPs were not significantly different despite being smaller in mutant dogs (75.5 ± 20.9 mmHg vs. 88.6 ± 29.7 mmHg; p = 0.18). Axial length was significantly larger in mutant vs. normal dogs (20.9 ± 0.6 mm vs. 19.8 ± 0.8 mm; p = 0.01), but there was no significant difference in mean CCT (666.3 ± 52.0 μm vs. 634.1 ± 24.7 μm; p = 0.74) between mutant and normal dogs. There was no significant difference in CD, regardless of whether young dogs <12 months were included (mutant vs. normal 15.9 ± 0.9 mm vs. 15.3 ± 0.6 mm) or not (mutant vs. normal 16.1 ± 1.0 vs. 15.3 ± 0.6 mm). The difference in AXL remained when young dogs <12 months were excluded (21.2 ± 0.5 mm vs. 20.1 ± 0.6 mm; p < 0.001). We could not detect a significant difference in cumIOP between ADAMTS10-mutant and normal dogs (17,058 ± 14,309 mmHg day vs. 14,981 ± 8,280 mmHg day; p > 0.9).
There was no OPA age effect over all the dogs tested (Figure 5). The age range for normal dogs was 11 years (133 months), and the median was 2.5 years (30 months). The age range for ADAMTS10-mutant dogs was 6 years (73 months), and the median was 3 years (38 months) (Figure 1). When tested over all dogs, we did not find any significant correlations between OPA and these parameters: IOP (p = 0.17), PP (p = 0.34), AXL, (p = 0.62), CCT (p = 0.91), and VPA (p = 0.34) (Figure 6). We did find significant correlations between IOP and PP (r = −0.41; p = 0.01), IOP and AXL (r = 0.43; p = 0.001), and AXL and CCT (r = 0.34; p = 0.02). When analyzing ADAMTS10-mutant dogs alone, we found a significant positive correlation between OPA and IOP (r = 0.61; p = 0.004) (Figure 6). Furthermore, mutant dogs showed significant positive correlations between age and AXL (r = 0.7; p < 0.001), between age and cumIOP (r = 0.97; p < 0.001), and between AXL and cumIOP (r = 0.73; p < 0.001). There were no significant correlations between any of the parameters in the normal control dogs, including age and AXL (Figure 6).
FIGURE 5. Change of OPA with age for ADAMTS10-mutant and normal dogs. Despite the trendlines, there was no detectable age effect on OPA.
FIGURE 6. Summary of correlations. Unshaded numbers show that OPA was not correlated with any of the other parameters when analyzing all dogs together. Significant correlations were found between IOP and PP, IOP and AXL, and AXL and CCT. No significant correlations were found in the normal dog group. The yellow-shaded numbers show the significant correlations in the ADAMTS10-mutant dog group: These were between OPA and IOP, age and AXL, age and cumIOP, and between AXL and cumIOP. Abbreviations: AXL, axial length; CD, corneal diameter; cumIOP, cumulative IOP; IOP, intraocular pressure; OPA, ocular pulse amplitude; CCT, central corneal thickness; PP, perfusion pressure; VPA, vascular pulse amplitude.
Because the iCare TonoVet® is the standard tonometer used in our laboratory and widely used in veterinary clinical practice, we used IOP measurements taken with this instrument for our statistical analyses. However, as part of the study design, IOPs were also measured with the Reichert Model 30™ pneumotonometer immediately before OPA assessment; these readings were not used in our analyses. Nevertheless, Bland-Altman plot and linear regression analysis showed good agreement between the two tonometers with the pneumotonometer overestimating most IOPs compared to the TonoVet® (Figure 7).
FIGURE 7. Comparison of tonometers. (A) Bland-Altman plot comparing Reichert Model 30™ pneumotonometer and iCare TonoVet® rebound tonometer. The estimated means of the differences between the two tonometers are shown by the green horizontal line. The 95% CI is marked by the red lines representing the upper (ULA) and lower (LLA) limits of agreement (mean ± 1.96 SD). Overall, the IOP measurements did not follow any trends and were mainly located within the 95% CI. The estimated means of the differences (±SD) were 6.9 ± 5.1 mmHg. (B) IOP regression analyses between tonometers with linear equation and R2 values. The red line shows strong linear correlation between Reichert Model 30™ pneumotonometer and iCare TonoVet® rebound tonometer with the blue dashed lines marking the 95% prediction intervals. Compared to the black y = x line, the pneumotonometer overestimated most IOPs when compared to the TonoVet® with data points mostly located to the left of the y = x line.
Ocular stiffness may be essential to glaucoma pathophysiology, but the exact role of tissue biomechanics and susceptibility to IOP-related damage is not entirely understood (Park and Komaromy, 2021; Safa et al., 2022). The most commonly used clinical assessments of ocular biomechanics are limited to the cornea and consist of CCT and corneal hysteresis (Gordon et al., 2002; Medeiros et al., 2013; Ayyalasomayajula et al., 2016; Weinreb et al., 2016). Because of their importance in IOP susceptibility, there is a critical need for additional clinical tools that include the biomechanics of the PPS and lamina cribrosa (Downs et al., 2009; Burgoyne, 2011; Safa et al., 2022). Measurement of OPA may be such a tool. The extent of transient IOP fluctuations, such as from saccades, blinking, ocular pulse, or controlled microvolumetric intraocular injections, is related to overall ocular coat stiffness, including corneal and PPS stiffness (Liu and He, 2009; Morris et al., 2013; Beaton et al., 2015; Sturmer and Kniestedt, 2015; Clayson et al., 2017; Sherwood et al., 2019; Turner et al., 2019; Sayah et al., 2020; Ma et al., 2021; Markert et al., 2022). In the current study we demonstrated that compared to normals the OPA is significantly reduced in ADAMTS10-mutant Beagles, a well-established, clinically relevant large animal model of OAG with decreased scleral stiffness and normalized ocular rigidity (Palko et al., 2013; Boote et al., 2016; Palko et al., 2016). We suspect that the softer ADAMTS10-mutant sclera is in part responsible for an improved IOP tolerance with slower progression of ONH atrophy and vision loss in Beagles with ADAMTS10-OAG compared to other glaucoma-affected canine breeds with comparable IOPs (Kuchtey et al., 2011; Park and Komaromy, 2021).
Ocular pulse amplitude is the difference between diastolic and systolic IOP and is created by the pulsatile choroidal blood flow (Dastiridou et al., 2009; Beaton et al., 2015; Sturmer and Kniestedt, 2015). Our conclusions on ADAMTS10-mutant dogs are based on observations in human patients that OPA and ocular rigidity are correlated at controlled IOPs of 15–45 mmHg: increased transient IOP fluctuations, such as OPA, are associated with greater rigidity (Dastiridou et al., 2009). However, the relationship between OPA and ocular rigidity may differ for different eyes because many other factors impact OPA. The OPA measurements using a pneumotonograph were well tolerated and easily performed in lightly sedated dogs, supporting the potential routine clinical application. The reasonable repeatability of the canine OPA measurements demonstrated in a separate experiment further supports their potential clinical usefulness.
Our canine OPA values (normal: 6.5 ± 2.8 mmHg; ADAMTS10-mutant: 4.1 ± 2.0 mmHg) are comparable to the 0.9–7.2-mmHg range in normal and glaucomatous humans; although the mean OPAs in dogs are lower than previously reported in humans (2.2 and 3.0 mmHg, respectively) (Kaufmann et al., 2006; Kac et al., 2011; Sturmer and Kniestedt, 2015). The higher pressure readings by the pneumotonometer could explain this. The obtained data supported our hypothesis that the softer ADAMTS10-mutant sclera may be partly responsible for the significantly lower OPA because it provides less resistance to the pulsatile choroidal blood flow. Our finding of lower OPA in ADAMTS10-mutant dogs is even more significant, considering that their ocular rigidity and OPA should be higher with higher baseline IOPs (Friedenwald, 1937; Dastiridou et al., 2009; Downs, 2015).
While not widely used in clinical settings, OPA was measured previously in humans with different forms of glaucoma; however, IOP susceptibility was not explicitly studied. In most studies, OPA with chronic POAG was not different from normal individuals (Sturmer and Kniestedt, 2015). Some studies showed reduced or elevated OPA in POAG patients (Trew and Smith, 1991a; Trew and Smith, 1991b; Mittag et al., 1994; Schmidt et al., 1997; Kerr et al., 1998; Schmidt et al., 1998; Khan et al., 2002; Kniestedt et al., 2006; Punjabi et al., 2006; Romppainen et al., 2007; Stalmans et al., 2008; Vulsteke et al., 2008; Asejczyk-Widlicka et al., 2014; Katsimpris et al., 2014; Sturmer and Kniestedt, 2015; Milioti et al., 2017). These variations were based on diverse study populations with various degrees of glaucomatous optic neuropathy, different IOP ranges, and the use of IOP-lowering medications (Sturmer and Kniestedt, 2015). In general, OPA increased with progressing POAG, suggesting increased ocular stiffening (Romppainen et al., 2007; Weizer et al., 2007; Kac et al., 2011; Kynigopoulos et al., 2012; Moghimi et al., 2013; Gross et al., 2014; Sturmer and Kniestedt, 2015). Our OPA data supported this: differences between ADAMTS10-mutant and normal dogs became less significant when excluding young dogs before the development of glaucoma. Others have shown increased OPA with decreased glaucoma severity or better IOP control and decreased OPA with progressive ONH atrophy and visual field loss (Agarwal et al., 2003; Weizer et al., 2007; Vulsteke et al., 2008; Sturmer and Kniestedt, 2015).
Controversial results have also been reported when comparing NTG patients to normal: Some found that NTG patients had lower OPA or pulsatile ocular blood flow (POBF) than normal controls (Quaranta et al., 1994; Schmidt et al., 1997; Schwenn et al., 2002). Considering that NTG patients are more susceptible to IOP since they develop glaucomatous optic neuropathy and visual field loss without documented IOP elevation, these findings contradict our results with lower OPA in ADAMTS10-mutant dogs that are more resistant to IOP elevation. Others did not find OPA differences between NTG patients, normals, or chronic POAG-affected individuals (Khan et al., 2002; Kniestedt et al., 2006; Read et al., 2008; Stalmans et al., 2008; Vulsteke et al., 2008). Another study found significantly lower OPA in NTG than in POAG patients, OHT, and normal controls. A logistic regression model was established to identify OPA as an independent risk factor for NTG (Schwenn et al., 2002). OPA and IOP were significantly higher in NTG patients with central visual field loss compared to NTG patients without visual field loss, with a significant correlation between OPA increase and central visual field loss (Lee et al., 2012). OPA measurement could be helpful in the detection and screening of NTG patients, but additional studies are needed for validation.
In contrast to NTG patients, individuals with OHT are more resistant to IOP elevation without developing ONH damage (Weinreb et al., 2016). Studies of OPA in OHT-affected individuals are variable, with some showing higher ocular rigidity compared to POAG patients and others not showing an OPA difference between OHT, POAG, and control groups (Schwenn et al., 2002; Wang et al., 2013). In patients with OHT and chronic POAG, increased OPA was a significant risk factor for visual field loss (Gross et al., 2014).
While we did not include dogs treated with glaucoma medications, a weakness of several referenced human clinical studies is the unknown contribution of glaucoma treatments on the OPA. There are only limited data on the effect of topical glaucoma medications: For example, while the prostaglandin analog latanoprost and the carbonic anhydrase inhibitor dorzolamide HCl were associated with increased POBF, the beta-adrenergic blocker timolol maleate did not affect POBF (Claridge and Smith, 1994; Schmidt et al., 1998; Georgopoulos et al., 2002). Another study showed an OPA-lowering effect of the prostaglandin analogs tafluprost and latanoprost in human patients with NTG and POAG (Park et al., 2015).
The measurement of OPA is a reasonably old method and has been performed by several investigators over the past century on humans and various animal species (Thiel, 1928; Susuki, 1962; Bynke and Krakau, 1964; Lawrence and Schlegel, 1966; Lester, 1966; Bynke, 1968). The reliability of ocular pulse pressure waves measured with a pneumatic tonometer has been previously documented in dogs, confirmed with manometric systems, and compared to humans and rabbits (Tonjum, 1972). The pneumotonography used in this study has been well-established in dogs and is routinely used in our laboratory to measure aqueous humor outflow facility (Gelatt et al., 1996). The pneumotonography tonometer was previously compared to other applanation tonometers in dogs (Gelatt and MacKay, 1998). We have shown an excellent linear correlation between the Reichert Model 30™ Pneumotonometer and the iCare TonoVet® rebound tonometer, with the pneumotonometer reading consistently higher than the rebound tonometer. Because the iCare TonoVet® is the standard tonometer used in our laboratory and widely used in veterinary clinical practice, we used IOP measurements taken with this rebound tonometer for our statistical analyses.
As expected, IOPs were significantly higher in mutant vs. normal dogs because the G661R missense mutation in ADAMTS10 is responsible for OAG (Kuchtey et al., 2011). The IOPs were not high enough to affect PPs significantly, although there was a significant negative correlation between IOP and PP. There were also no significant differences in CCT, but we found a significant correlation between IOP and CCT. In human glaucoma patients, OPA has been previously positively associated with CCT (Weizer et al., 2007). Axial length was significantly larger in our mutant vs. normal dogs, with a significant positive correlation between IOP and AXL, consistent with the chronic IOP increase associated with ADAMTS10-OAG and the development of buphthalmos. The significant linear age-AXL correlation in ADAMTS10-mutant dogs further supported this. Since there was no such correlation in normal dogs, and all enrolled canine eyes were considered adult-size (Tuntivanich et al., 2007), the increase in AXL with age was likely disease-related.
Despite the extensive age range (normal = 11 years; mutant = 6 years), we could not detect a significant OPA age effect in mutant and normal dogs. The trend lines in Figure 5 are likely affected by the outliers. We have previously reported that age was positively associated with complex modulus and negatively associated with loss tangent, suggesting increased stiffness and decreased mechanical damping with age in ADAMTS10-mutant and normal dogs (Palko et al., 2016). This disagreement should be investigated in a future study by comparing OPA and scleral biomechanics in the same canine globes–this was beyond the scope of the current, non-terminal study. We did observe that OPA differences became less significant when excluding young adult dogs <12 months of age.
We found a significant positive correlation between OPA and IOP in ADAMTS10-mutant dogs. This finding is consistent with several human studies that found positive correlations between OPA and IOP in healthy individuals and patients with NTG and OHT (Phillips et al., 1992; Kaufmann et al., 2006; Kim et al., 2013; Sturmer and Kniestedt, 2015).
The remodeling of the sclera is likely more affected by cumIOP rather than a single measurement taken on the procedure day. Therefore, we attempted to include cumIOP as a variable. Because the number of archival IOP data varied between dogs and was not collected continuously, the cumIOP numbers in this study should be considered estimates. The numbers were highly variable and surprisingly not significantly different between mutant and normal dogs, possibly because of the more advanced age of some normal dogs. While we did not find any cumIOP-OPA correlation, age and AXL were positively correlated with cumIOP in ADAMTS10-mutant dogs.
The lack of a significant correlation between OPA and PP is consistent with results from human studies (Sturmer and Kniestedt, 2015). We also did not find a significant correlation between OPA and AXL and between OPA and CD. Previous studies reported significant negative correlations between OPA and AXL, especially in human eyes with high myopia (Kaufmann et al., 2006; Sturmer and Kniestedt, 2015; Yang and Koh, 2015).
We found no significant correlations between OPA and VPA in our dogs. One study in human NTG patients showed a positive correlation between OPA and blood pressure amplitude (Kim et al., 2013). The OPA and blood pressure variances ratio was a potential diagnostic indicator for early human POAG, but the study included only ten normal and 11 glaucoma patients (Robert et al., 2012).
The significant correlation between the two eyes’ OPA justified averaging parameters from the left and right eyes. A previous simulation study showed that using the average of both eyes of the same individual as an analysis unit preserved type I error better than other statistical techniques while maintaining the power (Huang et al., 2018).
In addition to the lack of direct comparison of OPA and scleral biomechanics in the same eyes, our study has several other limitations, including the relatively small sample size compared to most referenced human studies. Because we do not have any clear indication of reduced ocular blood flow in ADAMTS10-mutant dogs, the additional measurement of pulsatile blood flow would have strengthened the main conclusions of our study regarding the OPA-scleral biomechanics relationship. Nevertheless, to our knowledge, this is the first study that evaluates OPA as a potential clinical measure for ocular rigidity in a well-established large animal glaucoma model with well-defined scleral biomechanics. Others proposed a relationship between corneal hysteresis and OPA and their heritability in human subjects (Kaufmann et al., 2006; Carbonaro et al., 2008). Future studies will be strengthened by directly comparing OPA with ex vivo corneal and scleral biomechanical measurements in the same eyes. We are aware of the potential limitations of OPA measurement since it is not only influenced by ocular rigidity but also by other factors, such as the amplitude of the pulsatile blood flow. Nevertheless, the expanded clinical testing of ocular rigidity by including OPA has excellent potential in assessing glaucoma risk relative to IOP.
The raw data supporting the conclusions of this article will be made available by the authors, without undue reservation.
The animal study was approved by Michigan State University Institutional Animal Care and Use Committee (IACUC). The study was conducted in accordance with the local legislation and institutional requirements.
VR: conceptualization, methodology, and writing manuscript; DS: data analysis; SW: data analysis; JK: conceptualization and methodology; AJ: conceptualization and methodology; CH: conceptualization and methodology; AK: conceptualization, writing manuscript, and supervision. All authors contributed to the article and approved the submitted version.
This work was supported by the National Institutes of Health (R01-EY025752, T35-OD016477-19, R01-EY032478), the BrightFocus Foundation (G2022007S), and the Ronald and Sharon Loessel Endowment Fund.
The authors thank Dr. Simon Petersen-Jones, Janice Querubin, Lydia Kapeller, Ava Cabble, and the Michigan State University Campus Animal Resources staff for their technical support.
AK is a consultant and received research funding from Reichert, Inc.
The remaining authors declare that the research was conducted in the absence of any commercial or financial relationships that could be construed as a potential conflict of interest.
All claims expressed in this article are solely those of the authors and do not necessarily represent those of their affiliated organizations, or those of the publisher, the editors and the reviewers. Any product that may be evaluated in this article, or claim that may be made by its manufacturer, is not guaranteed or endorsed by the publisher.
ADAMTS10, A disintegrin and metalloproteinase with thrombospondin motifs 10; ANCOVA, analysis of covariance; ANOVA, analysis of variance; AXL, axial length; CCT, central corneal thickness; CD, corneal diameter; cumIOP, cumulative IOP; IOP, intraocular pressure; NTG, normal tension glaucoma; OAG, open-angle glaucoma; OHT, ocular hypertension; OPA, ocular pulse amplitude; ORA, Ocular Response Analyzer®; POAG, primary open-angle glaucoma; POBF, pulsatile ocular blood flow; PP, perfusion pressure; PPS, peripapillary sclera; VPA, vascular pulse amplitude.
Agarwal, H. C., Gupta, V., Sihota, R., and Singh, K. (2003). Pulsatile ocular blood flow among normal subjects and patients with high tension glaucoma. Indian J. Ophthalmol. 51, 133–138.
Asejczyk-Widlicka, M., Krzyzanowska-Berkowska, P., Kowalska, M., and Iskander, D. R. (2014). Clinical utility of spectral analysis of intraocular pressure pulse wave. BMC Ophthalmol. 14, 30. doi:10.1186/1471-2415-14-30
Ayyalasomayajula, A., Park, R. I., Simon, B. R., and Vande Geest, J. P. (2016). A porohyperelastic finite element model of the eye: the influence of stiffness and permeability on intraocular pressure and optic nerve head biomechanics. Comput. Methods Biomech. Biomed. Engin 19, 591–602. doi:10.1080/10255842.2015.1052417
Beaton, L., Mazzaferri, J., Lalonde, F., Hidalgo-Aguirre, M., Descovich, D., Lesk, M. R., et al. (2015). Non-invasive measurement of choroidal volume change and ocular rigidity through automated segmentation of high-speed OCT imaging. Biomed. Opt. Express 6, 1694–1706. doi:10.1364/boe.6.001694
Bellezza, A. J., Hart, R. T., and Burgoyne, C. F. (2000). The optic nerve head as a biomechanical structure: initial finite element modeling. Invest. Ophthalmol. Vis. Sci. 41, 2991–3000.
Boote, C., Palko, J. R., Sorensen, T., Mohammadvali, A., Elsheikh, A., Komaromy, A. M., et al. (2016). Changes in posterior scleral collagen microstructure in canine eyes with an ADAMTS10 mutation. Mol. Vis. 22, 503–517.
Burgoyne, C. F. (2011). A biomechanical paradigm for axonal insult within the optic nerve head in aging and glaucoma. Exp. Eye Res. 93, 120–132. doi:10.1016/j.exer.2010.09.005
Burgoyne, C. F., Downs, J. C., Bellezza, A. J., Suh, J. K., and Hart, R. T. (2005). The optic nerve head as a biomechanical structure: a new paradigm for understanding the role of IOP-related stress and strain in the pathophysiology of glaucomatous optic nerve head damage. Prog. Retin Eye Res. 24, 39–73. doi:10.1016/j.preteyeres.2004.06.001
Bynke, H. G. (1968). Further estimation of the oculosphygmography as a diagnostic method in carotid occlusive disease. Acta Ophthalmol. (Copenh) 46, 792–805. doi:10.1111/j.1755-3768.1968.tb02878.x
Bynke, H. G., and Krakau, C. E. (1964). A handy instrument for oculo-sphygmography with some clinical results. Ophthalmologica 148, 367–373. doi:10.1159/000304708
Carbonaro, F., Andrew, T., Mackey, D. A., Spector, T. D., and Hammond, C. J. (2008). The heritability of corneal hysteresis and ocular pulse amplitude: a twin study. Ophthalmology 115, 1545–1549. doi:10.1016/j.ophtha.2008.02.011
Claridge, K. G., and Smith, S. E. (1994). Diurnal variation in pulsatile ocular blood flow in normal and glaucomatous eyes. Surv. Ophthalmol. 38 (Suppl. l), S198–S205. doi:10.1016/0039-6257(94)90067-1
Clayson, K., Pan, X., Pavlatos, E., Short, R., Morris, H., Hart, R. T., et al. (2017). Corneoscleral stiffening increases IOP spike magnitudes during rapid microvolumetric change in the eye. Exp. Eye Res. 165, 29–34. doi:10.1016/j.exer.2017.08.015
COLLABORATIVE NORMAL-TENSION GLAUCOMA STUDY GROUP (1998a). Comparison of glaucomatous progression between untreated patients with normal-tension glaucoma and patients with therapeutically reduced intraocular pressures. Collaborative Normal-Tension Glaucoma Study Group. Am. J. Ophthalmol. 126, 487–497. doi:10.1016/s0002-9394(98)00223-2
COLLABORATIVE NORMAL-TENSION GLAUCOMA STUDY GROUP (1998b). The effectiveness of intraocular pressure reduction in the treatment of normal-tension glaucoma. Collaborative Normal-Tension Glaucoma Study Group. Am. J. Ophthalmol. 126, 498–505. doi:10.1016/s0002-9394(98)00272-4
Coudrillier, B., Campbell, I. C., Read, A. T., Geraldes, D. M., Vo, N. T., Feola, A., et al. (2016). Effects of peripapillary scleral stiffening on the deformation of the lamina cribrosa. Invest. Ophthalmol. Vis. Sci. 57, 2666–2677. doi:10.1167/iovs.15-18193
Dastiridou, A. I., Ginis, H. S., De Brouwere, D., Tsilimbaris, M. K., and Pallikaris, I. G. (2009). Ocular rigidity, ocular pulse amplitude, and pulsatile ocular blood flow: the effect of intraocular pressure. Invest. Ophthalmol. Vis. Sci. 50, 5718–5722. doi:10.1167/iovs.09-3760
Downs, J. C. (2015). IOP telemetry in the nonhuman primate. Exp. Eye Res. 141, 91–98. doi:10.1016/j.exer.2015.07.015
Downs, J. C., Roberts, M. D., Burgoyne, C. F., and Hart, R. T. (2009). Multiscale finite element modeling of the lamina cribrosa microarchitecture in the eye. Annu. Int. Conf. IEEE Eng. Med. Biol. Soc. 2009, 4277–4280. doi:10.1109/IEMBS.2009.5332755
Friedenwald, J. S. (1937). Contribution to the theory and practice of tonometry. Am. J. Ophthalmol. 20, 985–1024. doi:10.1016/s0002-9394(37)90425-2
Gelatt, K. N., Gum, G. G., Mackay, E. O., and Gelatt, K. J. (1996). Estimations of aqueous humor outflow facility by pneumatonography in normal, genetic carrier and glaucomatous beagles. Veterinary & Comp. Ophthalmol. 6, 148–151.
Gelatt, K. N., and Mackay, E. O. (1998). Distribution of intraocular pressure in dogs. Vet. Ophthalmol. 1, 109–114. doi:10.1046/j.1463-5224.1998.00024.x
Georgopoulos, G. T., Diestelhorst, M., Fisher, R., Ruokonen, P., and Krieglstein, G. K. (2002). The short-term effect of latanoprost on intraocular pressure and pulsatile ocular blood flow. Acta Ophthalmol. Scand. 80, 54–58. doi:10.1034/j.1600-0420.2002.800111.x
Gordon, M. O., Beiser, J. A., Brandt, J. D., Heuer, D. K., Higginbotham, E. J., Johnson, C. A., et al. (2002). The Ocular Hypertension Treatment Study: baseline factors that predict the onset of primary open-angle glaucoma. Arch. Ophthalmol. 120, 714–720. doi:10.1001/archopht.120.6.714
Gross, S., Gugleta, K., Turksever, C., Ledolter, A., Kochkorov, A., Flammer, J., et al. (2014). Analysis of risk factors for long-term glaucomatous damage development. Klin. Monbl Augenheilkd 231, 335–339. doi:10.1055/s-0034-1368222
Hopkins, A. A., Murphy, R., Irnaten, M., Wallace, D. M., Quill, B., and O’Brien, C. (2020). The role of lamina cribrosa tissue stiffness and fibrosis as fundamental biomechanical drivers of pathological glaucoma cupping. Am. J. Physiol. Cell Physiol. 319, C611–C623. doi:10.1152/ajpcell.00054.2020
Huang, J., Huang, J., Chen, Y., and Ying, G. S. (2018). Evaluation of approaches to analyzing continuous correlated eye data when sample size is small. Ophthalmic Epidemiol. 25, 45–54. doi:10.1080/09286586.2017.1339809
Jonas, J. B., Aung, T., Bourne, R. R., Bron, A. M., Ritch, R., and Panda-Jonas, S. (2017). Glaucoma. Lancet 390, 2183–2193. doi:10.1016/s0140-6736(17)31469-1
Kac, M. J., Solari, H. P., Velarde, G. C., Brazuna, R., Cardoso, G. P., and Ventura, M. P. (2011). Ocular pulse amplitude in patients with asymmetric primary open-angle glaucoma. Curr. Eye Res. 36, 727–732. doi:10.3109/02713683.2011.584652
Katsimpris, J. M., Theoulakis, P. E., Papadopoulos, G. E., Katsimpris, A., Lepidas, J., and Petropoulos, I. K. (2014). Ocular pulse amplitude measurement using pascal dynamic contour tonometer in glaucoma patients. Klin. Monbl Augenheilkd 231, 363–367. doi:10.1055/s-0034-1368220
Kaufmann, C., Bachmann, L. M., Robert, Y. C., and Thiel, M. A. (2006). Ocular pulse amplitude in healthy subjects as measured by dynamic contour tonometry. Arch. Ophthalmol. 124, 1104–1108. doi:10.1001/archopht.124.8.1104
Kerr, J., Nelson, P., and O’Brien, C. (1998). A comparison of ocular blood flow in untreated primary open-angle glaucoma and ocular hypertension. Am. J. Ophthalmol. 126, 42–51. doi:10.1016/s0002-9394(98)00074-9
Khan, J. C., Hughes, E. H., Tom, B. D., and Diamond, J. P. (2002). Pulsatile ocular blood flow: the effect of the Valsalva manoeuvre in open angle and normal tension glaucoma: a case report and prospective study. Br. J. Ophthalmol. 86, 1089–1092. doi:10.1136/bjo.86.10.1089
Kim, Y. J., Lee, K. S., Lee, J. R., Na, J. H., Choi, J., Han, S., et al. (2013). Ocular pulse amplitude as a dynamic parameter and its relationship with 24-h intraocular pressure and blood pressure in glaucoma. Exp. Eye Res. 115, 65–72. doi:10.1016/j.exer.2013.06.010
Kniestedt, C., Lin, S., Choe, J., Nee, M., Bostrom, A., Sturmer, J., et al. (2006). Correlation between intraocular pressure, central corneal thickness, stage of glaucoma, and demographic patient data: prospective analysis of biophysical parameters in tertiary glaucoma practice populations. J. Glaucoma 15, 91–97. doi:10.1097/00061198-200604000-00003
Koz, O. G., Turkcu, M. F., Yarangumeli, A., Koz, C., and Kural, G. (2009). Normotensive glaucoma and risk factors in normotensive eyes with pseudoexfoliation syndrome. J. Glaucoma 18, 684–688. doi:10.1097/ijg.0b013e31819c4311
Kuchtey, J., Olson, L. M., Rinkoski, T., Mackay, E. O., Iverson, T. M., Gelatt, K. N., et al. (2011). Mapping of the disease locus and identification of ADAMTS10 as a candidate gene in a canine model of primary open angle glaucoma. PLoS Genet. 7, e1001306. doi:10.1371/journal.pgen.1001306
Kynigopoulos, M., Tzamalis, A., Ntampos, K., and Schlote, T. (2012). Decreased ocular pulse amplitude associated with functional and structural damage in open-angle glaucoma. Eur. J. Ophthalmol. 22, 111–116. doi:10.5301/ejo.5000043
Lawrence, C., and Schlegel, W. A. (1966). Ophthalmic pulse studies. I. Influence of intraocular pressure. Invest. Ophthalmol. 5, 515–525.
Lee, M., Cho, E. H., Lew, H. M., and Ahn, J. (2012). Relationship between ocular pulse amplitude and glaucomatous central visual field defect in normal-tension glaucoma. J. Glaucoma 21, 596–600. doi:10.1097/ijg.0b013e31824cfbf7
Lester, H. A. (1966). Ocular oscillometry in cerebrovascular disease. Arch. Ophthalmol. 76, 391–398. doi:10.1001/archopht.1966.03850010393018
Liu, J., and He, X. (2009). Corneal stiffness affects IOP elevation during rapid volume change in the eye. Invest. Ophthalmol. Vis. Sci. 50, 2224–2229. doi:10.1167/iovs.08-2365
Markert, J. E., Turner, D. C., Jasien, J. V., Nyankerh, C. N. A., Samuels, B. C., and Downs, J. C. (2022). Ocular pulse amplitude correlates with ocular rigidity at native IOP despite the variability in intraocular pulse volume with each heartbeat. Transl. Vis. Sci. Technol. 11, 6. doi:10.1167/tvst.11.9.6
Ma, Y., Moroi, S. E., and Roberts, C. J. (2021). Non-invasive clinical measurement of ocular rigidity and comparison to biomechanical and morphological parameters in glaucomatous and healthy subjects. Front. Med. (Lausanne) 8, 701997. doi:10.3389/fmed.2021.701997
Medeiros, F. A., Meira-Freitas, D., Lisboa, R., Kuang, T. M., Zangwill, L. M., and Weinreb, R. N. (2013). Corneal hysteresis as a risk factor for glaucoma progression: a prospective longitudinal study. Ophthalmology 120, 1533–1540. doi:10.1016/j.ophtha.2013.01.032
Milioti, G., Langenbucher, A., Seitz, B., and Low, U. (2017). Effect of morphological and functional parameters on ocular pulse amplitudes: an analysis in ocular hypertension and different types of glaucoma. Klin. Monbl Augenheilkd 234, 223–230. doi:10.1055/s-0042-101350
Mittag, T. W., Serle, J., Schumer, R., Brodie, S., Stegman, D., Schmidt, K. G., et al. (1994). Studies of the ocular pulse in primates. Surv. Ophthalmol. 38 (Suppl. l), S183–S190. doi:10.1016/0039-6257(94)90065-5
Moghimi, S., Torabi, H., Fakhraie, G., Nassiri, N., and Mohammadi, M. (2013). Dynamic contour tonometry in primary open angle glaucoma and pseudoexfoliation glaucoma: factors associated with intraocular pressure and ocular pulse amplitude. Middle East Afr. J. Ophthalmol. 20, 158–162. doi:10.4103/0974-9233.110606
Morris, H. J., Tang, J., Cruz Perez, B., Pan, X., Hart, R. T., Weber, P. A., et al. (2013). Correlation between biomechanical responses of posterior sclera and IOP elevations during micro intraocular volume change. Invest. Ophthalmol. Vis. Sci. 54, 7215–7222. doi:10.1167/iovs.13-12441
Palko, J. R., Iwabe, S., Pan, X., Agarwal, G., Komaromy, A. M., and Liu, J. (2013). Biomechanical properties and correlation with collagen solubility profile in the posterior sclera of canine eyes with an ADAMTS10 mutation. Invest. Ophthalmol. Vis. Sci. 54, 2685–2695. doi:10.1167/iovs.12-10621
Palko, J. R., Morris, H. J., Pan, X., Harman, C. D., Koehl, K. L., Gelatt, K. N., et al. (2016). Influence of age on ocular biomechanical properties in a canine glaucoma model with ADAMTS10 mutation. PLoS One 11, e0156466. doi:10.1371/journal.pone.0156466
Park, S. A., and Komaromy, A. M. (2021). Biomechanics of the optic nerve head and sclera in canine glaucoma: a brief review. Vet. Ophthalmol. 24, 316–325. doi:10.1111/vop.12923
Park, S. H., Yoo, S. H., and Ha, S. J. (2015). Comparison of ocular pulse amplitude-lowering effects of tafluprost and latanoprost by dynamic contour tonometry. J. Ocul. Pharmacol. Ther. 31, 617–622. doi:10.1089/jop.2014.0122
Phillips, C. I., Tsukahara, S., Hosaka, O., and Adams, W. (1992). Ocular pulsation correlates with ocular tension: the choroid as piston for an aqueous pump? Ophthalmic Res. 24, 338–343. doi:10.1159/000267190
Punjabi, O. S., Ho, H. K., Kniestedt, C., Bostrom, A. G., Stamper, R. L., and Lin, S. C. (2006). Intraocular pressure and ocular pulse amplitude comparisons in different types of glaucoma using dynamic contour tonometry. Curr. Eye Res. 31, 851–862. doi:10.1080/02713680600899887
Quaranta, L., Manni, G., Donato, F., and Bucci, M. G. (1994). The effect of increased intraocular pressure on pulsatile ocular blood flow in low tension glaucoma. Surv. Ophthalmol. 38, S177–S182. doi:10.1016/0039-6257(94)90064-7
Quigley, H. A., and Broman, A. T. (2006). The number of people with glaucoma worldwide in 2010 and 2020. Br. J. Ophthalmol. 90, 262–267. doi:10.1136/bjo.2005.081224
Read, S. A., Collins, M. J., and Iskander, D. R. (2008). Diurnal variation of axial length, intraocular pressure, and anterior eye biometrics. Invest. Ophthalmol. Vis. Sci. 49, 2911–2918. doi:10.1167/iovs.08-1833
Robert, Y. C., Wild, A., Kessels, A. G., Backes, W. H., Zollinger, A., and Bachmann, L. M. (2012). Discrimination of healthy and glaucomatous eyes based on the ocular pulse amplitude: a diagnostic case-control study. Ophthalmic Res. 48, 1–5. doi:10.1159/000334616
Romppainen, T., Kniestedt, C., Bachmann, L. M., and Sturmer, J. (2007). Ocular pulse amplitude: a new biometrical parameter for the diagnose of glaucoma? Ophthalmologe 104, 230–235. doi:10.1007/s00347-006-1467-8
Safa, B. N., Wong, C. A., Ha, J., and Ethier, C. R. (2022). Glaucoma and biomechanics. Curr. Opin. Ophthalmol. 33, 80–90. doi:10.1097/icu.0000000000000829
Sayah, D. N., Mazzaferri, J., Ghesquiere, P., Duval, R., Rezende, F., Costantino, S., et al. (2020). Non-invasive in vivo measurement of ocular rigidity: clinical validation, repeatability and method improvement. Exp. Eye Res. 190, 107831. doi:10.1016/j.exer.2019.107831
Schmidt, K. G., Ruckmann, A. V., Mittag, T. W., Hessemer, V., and Pillunat, L. E. (1997). Reduced ocular pulse amplitude in low tension glaucoma is independent of vasospasm. Eye (Lond) 11 (Pt 4), 485–488. doi:10.1038/eye.1997.131
Schmidt, K. G., Von Ruckmann, A., and Pillunat, L. E. (1998). Topical carbonic anhydrase inhibition increases ocular pulse amplitude in high tension primary open angle glaucoma. Br. J. Ophthalmol. 82, 758–762. doi:10.1136/bjo.82.7.758
Schwenn, O., Troost, R., Vogel, A., Grus, F., Beck, S., and Pfeiffer, N. (2002). Ocular pulse amplitude in patients with open angle glaucoma, normal tension glaucoma, and ocular hypertension. Br. J. Ophthalmol. 86, 981–984. doi:10.1136/bjo.86.9.981
Sherwood, J. M., Boazak, E. M., Feola, A. J., Parker, K., Ethier, C. R., and Overby, D. R. (2019). Measurement of ocular compliance using iPerfusion. Front. Bioeng. Biotechnol. 7, 276. doi:10.3389/fbioe.2019.00276
Sommer, A., Tielsch, J. M., Katz, J., Quigley, H. A., Gottsch, J. D., Javitt, J., et al. (1991). Relationship between intraocular pressure and primary open angle glaucoma among white and black Americans. The Baltimore Eye Survey. Arch. Ophthalmol. 109, 1090–1095. doi:10.1001/archopht.1991.01080080050026
Stalmans, I., Harris, A., Vanbellinghen, V., Zeyen, T., and Siesky, B. (2008). Ocular pulse amplitude in normal tension and primary open angle glaucoma. J. Glaucoma 17, 403–407. doi:10.1097/ijg.0b013e31815c5f2c
Sturmer, J. P., and Kniestedt, C. (2015). Role of ocular pulse amplitude in glaucoma. Klin. Monbl Augenheilkd 232, 162–168. doi:10.1055/s-0034-1396232
Thiel, R. (1928). Hornhautpulsation, Blutdruck und Augendruck. Ber. Dtsch. Ophthal Ges. 47, 198–206.
Tonjum, A. M. (1972). Studies on the ocular pulse pressure wave in human, dog, and rabbit eyes with a pneumatic tonometer. Acta Ophthalmol. (Copenh) 50, 677–688. doi:10.1111/j.1755-3768.1972.tb06608.x
Trew, D. R., and Smith, S. E. (1991a). Postural studies in pulsatile ocular blood flow: I. Ocular hypertension and normotension. Br. J. Ophthalmol. 75, 66–70. doi:10.1136/bjo.75.2.66
Trew, D. R., and Smith, S. E. (1991b). Postural studies in pulsatile ocular blood flow: II. Chronic open angle glaucoma. Br. J. Ophthalmol. 75, 71–75. doi:10.1136/bjo.75.2.71
Tuntivanich, N., Petersen-Jones, S. M., Steibel, J. P., Johnson, C., and Forcier, J. Q. (2007). Postnatal development of canine axial globe length measured by B-scan ultrasonography. Vet. Ophthalmol. 10, 2–5. doi:10.1111/j.1463-5224.2007.00481.x
Turner, D. C., Edmiston, A. M., Zohner, Y. E., Byrne, K. J., Seigfreid, W. P., Girkin, C. A., et al. (2019). Transient intraocular pressure fluctuations: source, magnitude, frequency, and associated mechanical energy. Invest. Ophthalmol. Vis. Sci. 60, 2572–2582. doi:10.1167/iovs.19-26600
Vulsteke, C., Stalmans, I., Fieuws, S., and Zeyen, T. (2008). Correlation between ocular pulse amplitude measured by dynamic contour tonometer and visual field defects. Graefes Arch. Clin. Exp. Ophthalmol. 246, 559–565. doi:10.1007/s00417-007-0706-2
Wang, J., Freeman, E. E., Descovich, D., Harasymowycz, P. J., Kamdeu Fansi, A., Li, G., et al. (2013). Estimation of ocular rigidity in glaucoma using ocular pulse amplitude and pulsatile choroidal blood flow. Invest. Ophthalmol. Vis. Sci. 54, 1706–1711. doi:10.1167/iovs.12-9841
Weinreb, R. N., Aung, T., and Medeiros, F. A. (2014). The pathophysiology and treatment of glaucoma: a review. JAMA 311, 1901–1911. doi:10.1001/jama.2014.3192
Weinreb, R. N., Leung, C. K., Crowston, J. G., Medeiros, F. A., Friedman, D. S., Wiggs, J. L., et al. (2016). Primary open-angle glaucoma. Nat. Rev. Dis. Prim. 2, 16067. doi:10.1038/nrdp.2016.67
Weizer, J. S., Asrani, S., Stinnett, S. S., and Herndon, L. W. (2007). The clinical utility of dynamic contour tonometry and ocular pulse amplitude. J. Glaucoma 16, 700–703. doi:10.1097/ijg.0b013e31806ab2fe
Keywords: ADAMTS10, dog, glaucoma, intraocular pressure (IOP), ocular pulse amplitude (OPA), ocular rigidity
Citation: Raphtis VA, Sharma D, Wang S, Kim JY, Jacobson AL, Harman CD and Komáromy AM (2023) Ocular pulse amplitude (OPA) in canine ADAMTS10-open-angle glaucoma (ADAMTS10-OAG). Front. Bioeng. Biotechnol. 11:1242166. doi: 10.3389/fbioe.2023.1242166
Received: 18 June 2023; Accepted: 27 November 2023;
Published: 07 December 2023.
Edited by:
Matthew A. Reilly, The Ohio State University, United StatesReviewed by:
Joel Palko, West Virginia State University, United StatesCopyright © 2023 Raphtis, Sharma, Wang, Kim, Jacobson, Harman and Komáromy. This is an open-access article distributed under the terms of the Creative Commons Attribution License (CC BY). The use, distribution or reproduction in other forums is permitted, provided the original author(s) and the copyright owner(s) are credited and that the original publication in this journal is cited, in accordance with accepted academic practice. No use, distribution or reproduction is permitted which does not comply with these terms.
*Correspondence: András M. Komáromy, a29tYXJvbXlAbXN1LmVkdQ==
Disclaimer: All claims expressed in this article are solely those of the authors and do not necessarily represent those of their affiliated organizations, or those of the publisher, the editors and the reviewers. Any product that may be evaluated in this article or claim that may be made by its manufacturer is not guaranteed or endorsed by the publisher.
Research integrity at Frontiers
Learn more about the work of our research integrity team to safeguard the quality of each article we publish.