- 1Department of Orthopaedics and Sports Medicine, University Medical Center Rotterdam, Rotterdam, Netherlands
- 2Department of Otorhinolaryngology, University Medical Center Rotterdam, Rotterdam, Netherlands
- 3Department of Biomechanical Engineering, Faculty of Mechanical, Maritime and Materials Engineering, Delft University of Technology, Delft, Netherlands
Introduction: Mesenchymal stromal/progenitor cells (MSCs) are promising for cartilage cell-based therapies due to their chondrogenic differentiation capacity. However, MSCs can become senescent during in vitro expansion, a state characterized by stable cell cycle arrest, metabolic alterations, and substantial changes in the gene expression and secretory profile of the cell. In this study, we aimed to investigate how senescence and the senescence-associated secretory phenotype (SASP) affect chondrogenic differentiation of MSCs.
Methods: To study the effect of senescence, we exposed MSCs to gamma irradiation during expansion or during chondrogenic differentiation (the pellet culture). Western blot analysis was used to evaluate MSCs response to the chondrogenic inductor TGF-β.
Results: When senescence was induced during expansion or at day 7 of chondrogenic differentiation, we observed a significant reduction in the cartilage matrix. Interestingly, when senescence was induced at day 14 of differentiation, chondrogenesis was not significantly altered. Moreover, exposing chondrogenic pellets to the medium conditioned by senescent pellets had no significant effect on the expression of anabolic or catabolic cartilage markers, suggesting a neglectable paracrine effect of senescence on cartilage generation in our model. Finally, we show that senescent MSCs showed lower phosphorylated SMAD2 levels after TGFβ1 stimulation than control MSCs.
Conclusion: Overall, these results suggest that the occurrence of senescence in MSCs during expansion or early differentiation could be detrimental for cartilage tissue engineering.
1 Introduction
Articular cartilage is prone to damage and has a limited repair capability. Full-thickness loss of articular cartilage does not spontaneously regenerate and can lead to degenerative joint disease osteoarthritis (OA) (Mankin, 1982; Shapiro et al., 1993). Current treatment methods such as microfracture or autologous chondrocyte graft implantation have limitations and fail to prevent OA progression (Makris et al., 2015). An alternative strategy to repair the damaged cartilage uses mesenchymal stromal/progenitor cells (MSCs). MSCs are progenitor cells that can be isolated from several tissues such as bone marrow, synovial membrane, and adipose tissue and have the capacity to differentiate toward the chondrogenic lineage (Pittenger et al., 1999; Sakaguchi et al., 2005). To obtain enough MSCs to repair a cartilage defect, in vitro expansion is necessary. During extensive expansion, MSCs gradually lose their chondrogenic differentiation capacity (Banfi et al., 2002; Bonab et al., 2006), limiting the applications of these cells. Expansion also triggers cellular senescence, a process leading to an irreversible cell cycle arrest, major metabolic changes, and a senescence-associated secretory phenotype (SASP) (Hayflick and Moorhead, 1961; Hernandez-Segura et al., 2018). SASP factors produced by senescent cells include IL-6, IL-8, IL-1β, TNFα, MMP3, and MMP13 (Philipot et al., 2014; Basisty et al., 2020; Chung et al., 2020). It is known that these SASP factors can hamper tissue regeneration (Josephson et al., 2019), for example, exposure to TNFα and IL-1β during in vitro chondrogenesis limits the chondrogenic differentiation capacity of MSCs (Wehling et al., 2009). In addition, SASP factors such as TNFα and IL-1β are known pro-inflammatory factors contributing to the pathophysiology of OA (Pelletier et al., 1991; Greene and Loeser, 2015). This is further supported by the fact that transplantation of senescent fibroblasts can lead to an OA-like phenotype, including cartilage erosion and delamination of the articular surface (Xu et al., 2017). In addition, the SASP factors such as CCL2, IL-6, IGFBP4, and IGFBP7 have been suggested to contribute to the spread of cellular senescence in MSCs (Severino et al., 2013; Lehmann et al., 2022), known as paracrine senescence (Acosta et al., 2013). It is known that cellular senescence alters the differentiation capacity of MSCs, especially the effects on the osteogenic and adipogenic lineages are studied. Loss of osteogenic and adipogenic potential has been demonstrated in senescent MSCs (Bonab et al., 2006); however, it has also been reported that in late-passaged MSCs, the levels of mineralized matrix decline, while adipocyte differentiation increases (Stenderup et al., 2003; Kim et al., 2012), indicating the complexity of this phenomenon. Moreover, cartilage displays a decline in repair capacity with aging (Im et al., 2006), but little is known about the effect of cellular senescence on the chondrogenic differentiation capacity of MSCs. The aim of this study was, therefore, to determine how cellular senescence and their SASP affect chondrogenesis of MSCs.
2 Materials and methods
2.1 MSC isolation and expansion
MSCs were isolated from iliac crest bone chips that were obtained from patients (9–13 years) undergoing alveolar bone graft surgery, N = 13. The tissue was procured as leftover/waste surgical material, and it was reviewed and deemed exempt from full ethical review after ethical approval by the Erasmus Medical Ethical Committee (MEC-2014-16). These pediatric MSCs have been previously characterized and used in this study because they exhibit a low number of senescent cells at early passages (Knuth et al., 2018; Lehmann et al., 2022). MSCs were isolated by rinsing bone chips twice with 10 mL of alpha-MEM (Gibco, Thermo Fisher Scientific, Waltham, MA, United States) supplemented with 10% fetal calf serum (Thermo Fisher Scientific; selected batch 41Q2047K), 1.5 μg/mL of fungizone (Invitrogen, Thermo Fisher Scientific), 50 μg/mL gentamicin (Invitrogen, Thermo Fisher Scientific), 1 ng/mL FGF2 (R&D Systems, Minneapolis, MN, United States), and 0.1 mM ascorbic acid-2-phosphate (Sigma-Aldrich, Zwijndrecht, Netherlands). The MSCs were plated in T175 flasks, and after 24 h, the non-adherent cells were washed away. MSCs were trypsinized at sub-confluency and reseeded in a density of 2,300 cells/cm2. MSCs between passages 3 and 6 were used for experiments.
2.2 Irradiation of MSCs in a monolayer followed by chondrogenic differentiation
Senescence was induced in the cells by 20 Gy ionizing radiation using an RS320 X-Ray machine (X-Strahl, Camberley, United Kingdom) (Voskamp et al., 2021). MSCs in a monolayer were irradiated in a T175 flask (60%–70% confluency) for 22 min (20 Gy). Then, 24 h post-irradiation, the cells were trypsinized and seeded at a 9,600 cell/cm2 density. Mock-irradiated MSCs were used as non-senescent controls and seeded at 2,300 cells/cm2. Seven days post-irradiation, irradiated and non-irradiated MSCs were trypsinized, mixed (0, 25, 50, 75%, and 100% irradiated versus non-irradiated cells), and centrifuged at 300 g for 8 min to obtain pellets of 2 × 105 cells. To induce chondrogenesis, cell pellets were cultured in a chondrogenic medium, containing a DMEM-HG medium (Invitrogen brand Thermo Fisher Scientific), supplemented with 1% ITS (BD, Franklin Lakes, NJ, United States), 1.5 μg/mL fungizone (Invitrogen brand Thermo Fisher Scientific), 50 μg/mL gentamicin (Invitrogen brand, Thermo Fisher Scientific), 1 mM sodium pyruvate (Invitrogen brand, Thermo Fisher Scientific), 40 μg/mL proline (Sigma-Aldrich), 10 ng/mL TGFβ1 (R&D Systems), 0.1 mM ascorbic acid-2-phosphate (Sigma-Aldrich), and 100 nM dexamethasone (Sigma-Aldrich) for 7, 14, or 21 days. The medium was renewed twice a week.
2.3 Senescence-associated beta-galactosidase staining
To confirm cellular senescence, 7 days post-irradiation, the cells from each donor (N = 5) were trypsinized and seeded in monolayer cultures in triplicates. Subconfluent cells were washed twice with PBS. Next, the cells were fixed with 0.5% glutaraldehyde and 1% formalin in Milli-Q water for 5 min at room temperature. Then, the cells were washed twice with Milli-Q water, and subsequently, the cells were stained with freshly made X-gal solution containing 0.5% X-gal, 5 mM potassium ferricyanide, 5 mM potassium ferrocyanide, 2 mM magnesium chloride, 150 mM sodium chloride, 7 mM citric acid, and 25 mM disodium phosphate incubated for 24 h at 37°C. The cells were counterstained with 1:25 pararosaniline detected by bright field microscopy. Two independent researchers scored at least 100 cells as negative or positive, as previously described (Voskamp et al., 2021).
2.4 Irradiation of chondrogenic pellets and the conditioned medium
To induce cellular senescence in chondrogenic pellets, non-irradiated MSCs were cultured in a chondrogenic medium and renewed twice a week. MSCs in pellets were irradiated at day 7 or 14 of chondrogenic differentiation in a 15-mL tube for 22 min (20 Gy). The chondrogenic medium was renewed 24 h after irradiation; next, the medium was renewed twice a week. Mock-irradiated cells/pellets were used as controls.
To determine the effect of SASP factors on chondrogenic differentiation, we generated two different sets of chondrogenic pellets from the same donor, medium donating pellets from irradiated MSCs and medium recipient pellets from non-irradiated MSCs. To determine the effect at different time points during chondrogenesis, we analyzed the RNA expression of the medium recipient pellets at days 9 and 16. First, to generate the conditioned medium, the medium of the donating pellets was replaced by the DMEM-HG medium supplemented with 1% ITS, 1.5 μg/mL fungizone (Invitrogen brand, Thermo Fisher Scientific), 50 μg/mL gentamicin (Invitrogen brand, Thermo Fisher Scientific), 1 mM sodium pyruvate (Invitrogen brand, Thermo Fisher Scientific), and 40 μg/mL proline 24–48 h before harvesting. The medium from the donating pellets (N = 2) was collected and pooled per donor and time point. To remove the cell debris, the medium was centrifuged at 14,000 g for 1 min. Next, the medium was mixed with the DMEM-HG medium supplemented with 1% ITS, 1.5 μg/mL fungizone (Invitrogen brand, Thermo Fisher Scientific), 50 μg/mL gentamicin (Invitrogen brand, Thermo Fisher Scientific), 1 mM sodium pyruvate (Invitrogen brand, Thermo Fisher Scientific), and 40 μg/mL proline at ratio 3:1, and 0.1 mM ascorbic acid-2-phosphate (Sigma-Aldrich) and 10 ng/mL TGFβ1 were added to the total volume. The conditioned medium mixture was added to non-irradiated recipient MSC pellets for 2 consecutive days, specifically at days 7 and 8 (timepoint 9 days), or at days 14 15 (timepoint 16 days) during chondrogenic differentiation. At days 9 and 16, 24 h after the last addition of the conditioned medium, the medium recipient pellets were lysed in RNA-STAT (Tel-Test, Friendswood, TX, United States) for mRNA expression analysis. Media from the non-irradiated medium, donating MSC pellets using cells from the same donor and at the same time points, were generated and used as a control conditioned media.
2.5 Immunohistochemistry chondrogenic pellets
Pellets were fixed with 3.7% formaldehyde after 7, 14, or 21 days of chondrogenic induction. Next, pellets were embedded in paraffin and sectioned at 6 μm. To detect glycosaminoglycans (GAG), the sections were stained with 0.04% thionine solution. To detect collagen type 2, the sections were first treated with 0.1% pronase (Sigma-Aldrich) in PBS for 30 min at 37°C, followed by 1% hyaluronidase (Sigma-Aldrich) in PBS for 30 min at 37°C. The sections were incubated with 10% normal goat serum (Sigma-Aldrich) and 1% bovine serum albumin (Sigma-Aldrich) in PBS for 30 min, followed by incubation with the collagen type 2 antibody (II-II 6B3, Developmental Studies Hybridoma Bank) for 1 h. Then, the samples were incubated with a biotin-conjugated antibody (HK-325-UM, Biogenex) for 30 min, followed by incubation with alkaline phosphatase-conjugated streptavidin (HK-321-UK, Biogenex) for 30 min. New fuchsin chromogen (B467, Chroma Gesellschaft) was used as a substrate. As a negative control, an IgG1 isotype antibody (X0931, Dako Cytomation) was used. The positive area per pellet was determined using ImageJ software.
2.6 Osteogenic and adipogenic differentiation
To induce osteogenic differentiation, expanded MSCs were trypsinized, seeded at a density of 1.2 × 104 cells/cm2, and cultured in a DMEM HG medium (Gibco brand, Thermo Fisher Scientific) with 10% fetal calf serum (Gibco brand, Thermo Fisher Scientific), 1.5 μg/mL fungizone (Invitrogen brand, Thermo Fisher Scientific), 50 μg/mL gentamicin (Invitrogen brand, Thermo Fisher Scientific), 10 mM β-glycerophosphate (Sigma-Aldrich), 0.1 µM dexamethasone (Sigma-Aldrich), and 0.1 mM ascorbic acid-2-phosphate (Sigma-Aldrich) for 12–21 days. To detect calcium deposits, the cultures were fixed in 3.7% formaldehyde, followed by hydration with Milli-Q water and incubation with 5% silver nitrate solution (von Kossa; Sigma-Aldrich) for 1 h in the presence of bright light. Next, the cultures were washed with distilled water, followed by counterstaining with 0.4% thionine (Sigma-Aldrich). MSCs were used in triplicates (N = 3 donors). To induce adipogenic differentiation, expanded MSCs were trypsinized, seeded in a density of 2 × 104 cells/cm2, and cultured in DMEM HG (Gibco brand, Thermo Fisher Scientific) with 10% fetal calf serum (Gibco brand, Thermo Fisher Scientific), 1.5 μg/mL fungizone (Invitrogen brand, Thermo Fisher Scientific), 50 μg/mL gentamicin (Invitrogen brand, Thermo Fisher Scientific), 1.0 µM dexamethasone (Sigma-Aldrich), 0.2 mM indomethacin (Sigma-Aldrich), 0.01 mg/mL insulin (Sigma-Aldrich), and 0.5 mM 3-isobutyl-l-methylxanthine (Sigma-Aldrich) for 21 days. To detect intracellular lipid accumulation, the cells were fixed in 3.7% formaldehyde, followed by incubation with 0.3% Oil red O solution (Sigma-Aldrich) for 10 min, and then washed with distilled water. MSCs were used in triplicates (N = 3 donors).
2.7 DNA and glycosaminoglycan quantification
Pellets were digested at day 21 of chondrogenic differentiation using 1 mg/mL Proteinase K, 1 mM iodoacetamide, 10 μg/mL Pepstatin A in 50 mM Tris, and 1 mM EDTA buffer (pH 7.6; all Sigma-Aldrich) for 16 h at 56°C, followed by Proteinase K inactivation at 100°C for 10 min. Afterward, to determine the amount of DNA, cell lysates were treated with 0.415 IU heparin and 1.25 µg RNase for 30 min at 37°C, followed by the addition of 30 μL CYQUANT GR solution (Invitrogen). The samples were analyzed using a SpectraMax Gemini plate reader with an excitation of 480 nm and an emission of 520 nm. As a standard, DNA sodium salt from calf thymus (Sigma-Aldrich) was used. To determine the amount of GAG, cell lysates were incubated with 1, 9-dimethylmethylene blue (DMB), as previously described by Farndale et al. (1986), and analyzed with an excitation of 590 nm and 530 nm. The 530:590 nm ratio was used to determine the GAG concentration. As a standard, chondroitin sulfate sodium salt from shark cartilage (Sigma-Aldrich) was used.
2.8 mRNA expression analysis
For both MSCs in pellet cultures and MSCs in monolayer cultures, the medium was renewed 24 h before cell lysis. Pellets were washed twice with PBS, lysed in RNA-STAT (Tel-Test), and manually homogenized. Next, RNA was isolated using chloroform and purified using the RNeasy Micro Kit (QIAGEN, Hilden, Germany), in accordance with the manufacturer’s protocol. MSCs in a monolayer were washed twice with PBS, and RNA was isolated using RLT lysis buffer supplemented with 1% β-mercaptoethanol. Subsequently, RNA was purified using the RNeasy Micro Kit, in accordance with the manufacturer’s protocol. The RevertAid First Strand cDNA Synthesis Kit (Fermentas brand, Thermo Fisher Scientific) was used to reverse transcribe the RNA to cDNA. Next, real-time polymerase chain reactions were performed with SYBR Green (Fermentas brand, Thermo Fisher Scientific) and TaqMan (Applied Biosystems brand, Thermo Fisher Scientific) MasterMix on a CFX96TM PCR machine (Bio-Rad, Hercules, CA, United States) using different primers listed in Table 1. Genes with a housekeeping function are often used as reference genes for qPCR analysis; however, senescent cells often have altered their housekeeping functions (Hernandez-Segura et al., 2019). Therefore, we tested four different housekeeping genes (GAPDH, HPRT1, RPS27A, and ACTB) for each dataset and only used the genes that were stable across the different conditions as a reference. Gene expression levels were calculated using the 2−ΔΔCT formula.
2.9 Western blot
Irradiated MSCs and non-irradiated MSCs in a monolayer were serum starved for 16 h in alpha-MEM (Invitrogen) supplemented with 1% BSA, 1.5 μg/mL fungizone (Invitrogen), and 50 μg/mL gentamicin (Invitrogen). Next, MSCs were stimulated with 0 or 10 ng/mL TGFβ1 for 30 min, and subsequently, the cells were lysed in MPER lysis buffer (Thermo Fisher Scientific) with 1% Halt Protease Inhibitor (Thermo Fisher Scientific) and 1% Halt Phosphatase Inhibitor (Thermo Fisher Scientific). Protein samples, from MSCs and from different donors (N = 3 donors, in triplicates), were separated on a 4%–12% SDS-PAGE gel (Thermo Fisher Scientific) by electrophoresis using an equal amount of protein (5–12 µg) per sample. Proteins were transferred semi-wet from the SDS-PAGE gel on a nitrocellulose membrane (Millipore). The membrane was transferred to a 5% dry milk powder blocking solution in Tris-buffered saline with 0.1% Tween-20 (Millipore Sigma; TBST) for 3 h. Next, the membrane was incubated with the primary monoclonal rabbit antibody against phospho-SMAD2 Ser465/Ser467 (Cell Signaling Technology; 3108S) using a 1:1000 dilution in 5% BSA in TBST overnight at 4°C. Later, the membrane was incubated with a secondary anti-rabbit antibody conjugated with peroxidase (Cell Signaling, 7074S) using a 1:1000 dilution in 5% dry milk powder in TBST for 1.5 h at room temperature. The phospho-SMAD2 signal was detected with the SuperSignal West Pico Complete Rabbit IgG Detection Kit (Thermo Fisher Scientific).
2.10 Data analysis
The Kolmogorov–Smirnov test was used to verify the normal (Gaussian) distribution of all the histology, RNA expression, and Western blot data. For statistical evaluation, a linear mixed model was applied, using the different conditions as fixed parameters and the donors as random factors. The Bonferroni post hoc test was used to correct for multiple comparisons. Data analysis was performed using PSAW statistics 20 software (SPSS Inc., Chicago, IL, United States). Grand means were calculated as the average of each mean calculated per donor. p-values less than 0.05 were considered statistically significant.
3 Results
3.1 Cellular senescence impaired the chondrogenic capacity of MSCs
Cellular senescence was induced in monolayer MSCs by gamma irradiation (20 Gy) and confirmed by an increased mRNA expression of cell-cycle-dependent CDKN2A (6.9-fold) and CDKN1A (4.8-fold), a higher mRNA expression of the SASP-associated gene IL6 (8.6-fold), and a higher percentage of senescence-associated β-galactosidase-positive cells than the mock-treated control MSCs (0 Gy; Figures 1A,B). After 21 days of chondrogenic induction, irradiated MSCs had an impaired capacity to deposit the typical chondrogenic extracellular proteins GAG and COL2 (Figures 1C,D). To determine whether senescent MSCs have an overall reduced differentiation capacity or whether it was specific for the chondrogenic lineage, we assessed their osteogenic and adipogenic differentiation capacity. After adipogenic differentiation, the cells show lipid accumulation and expression of adipogenic genes PPRG and FABP4 in both the irradiated and non-irradiated cells (Figures 1E,F); although for FABP4, a reduced expression was detected compared to control MSCs. After osteogenic differentiation, irradiated and non-irradiated cells show no significant differences in the osteogenic markers RUNX2 and ALPL (Figures 1G,H). Overall, these results indicate that senescent MSCs can differentiate toward the adipogenic and the osteogenic lineage, while a strong negative effect was detected specifically for chondrogenic differentiation.
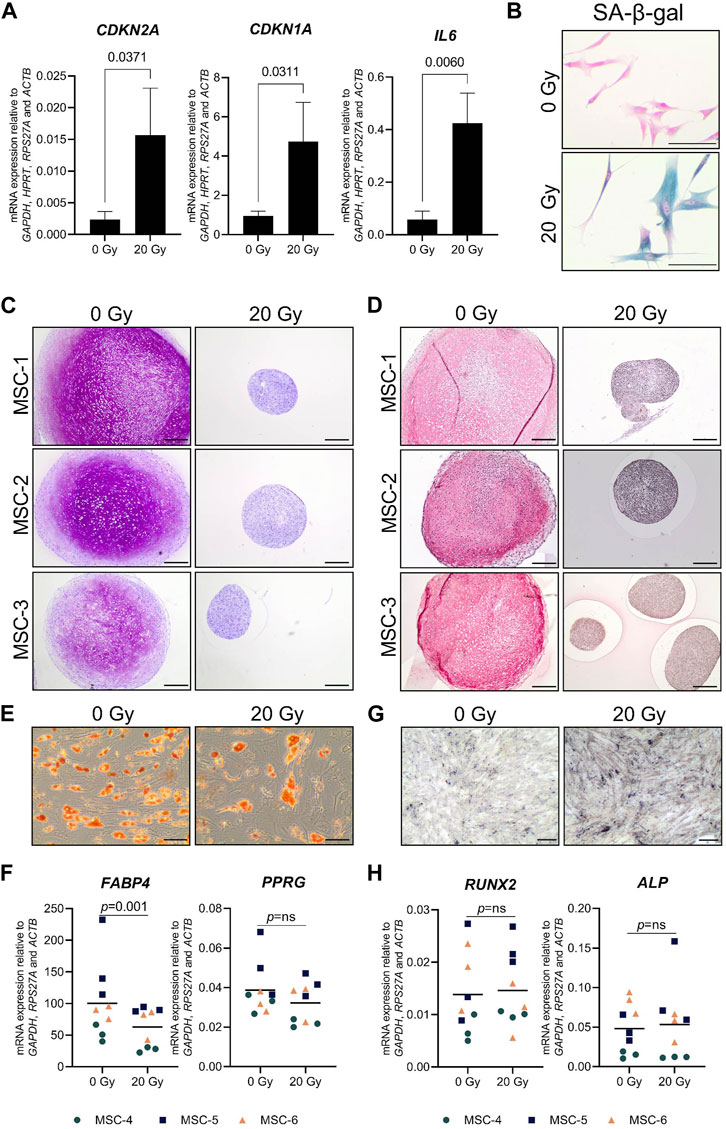
FIGURE 1. Chondrogenic differentiation was impaired in senescent MSCs. MSCs that were gamma-irradiated (20 Gy) or mock-irradiated (0 Gy) after expansion. (A) CDKN2A (P16), CDKN1A (P21), and IL6 mRNA relative to the best housekeeping index (BHI; GAPDH, HPRT, RPS27A, and ACTB). N = 3 donors with 2–3 replicates per donor. Data show grand mean and standard deviation. (B) Representative images of MSCs stained for senescence-associated β-galactosidase (SA-β-gal) activity. The scale bar represents 100 µm. N = 3 donors with 2–3 replicates per donor. (C, D) Representative images of thionine (C) and collagen type 2 (D) staining of pellets of mock-irradiated MSCs that were chondrogenically differentiated for 21 days. The scale bar represents 250 µm. N = 3 donors with three pellets per donor. (E) Representative images of Oil red O staining of mock-irradiated MSCs that were differentiated toward adipogenic lineage for 21 days. The scale bar represents 100 μm; N = 3 donors with three replicates per donor. (F) FABP4 and PPARG mRNA expression relative to the best housekeeping index (BHI; GAPDH, RPS27A, and ACTB) of MSCs that were differentiated toward the adipogenic lineage for 21 days. N = 3 donors with three replicates per donor. (G) Representative images of von Kossa staining of mock-irradiated MSCs that were differentiated toward the osteogenic lineage for 14–21 days. The scale bar represents 200 μm. N = 3 donors with three replicates per donor. (H) RUNX2 and ALP mRNA expression relative to the best housekeeping index (BHI; GAPDH, RPS27A, and ACTB) of MSCs that were differentiated toward the osteogenic lineage for 14–21 days. N = 3 donors with three replicates per donor. Data show individual data points and grand mean. p-values were obtained with the linear mixed model, using the different irradiation conditions as fixed parameters and the donors as random factors; ns = not significant.
3.2 Senescence during early MSC differentiation inhibited cartilage formation
In order to understand whether cellular senescence is affecting chondrogenic differentiation only when induced in specific differentiation stages, we used non-senescent MSCs to generate pellets and triggered senescence by irradiation during chondrogenic differentiation. Specifically, we induced senescence in pellet cultures by gamma irradiation (20 Gy) at 7 (early phase) or 14 (later phase) days of chondrogenesis in a 21-day differentiation protocol. As expected, mock-treated pellets (0 Gy) had an increased GAG deposition over time and the deposition is highest at day 21 of chondrogenic differentiation (p = 0.028 compared to day 7), while pellets treated with 20 Gy at day 7 of culture had an average of 1.6-fold reduction of GAG deposition at day 21 compared to controls (Figure 2A; Supplementary Figure S1; p = 0.035). Immunostaining revealed an overall similar pattern between COL2 and GAG deposition, with a lower COL2 deposition detected at day 21 in day 7-irradiated pellets compared to control pellets (Figure 2B; Supplementary Figure S2; p = 0.010). At the gene expression level, COL2A1 and ACAN significantly increased over time under both irradiated and control conditions, but at day 21, the day 7-irradiated pellets showed a significant reduced expression compared to control (Figure 2C; COL2A1 and ACAN). The transcription factor SOX9 did not strongly increase over time, and its expression was lower in day 7-irradiated pellets than that in control at day 21 (Figure 2C; SOX9). Between days 14 and 21 of chondrogenic differentiation, gene expression of COL2A1, ACAN, and SOX9 remained similar (p = 1.000).
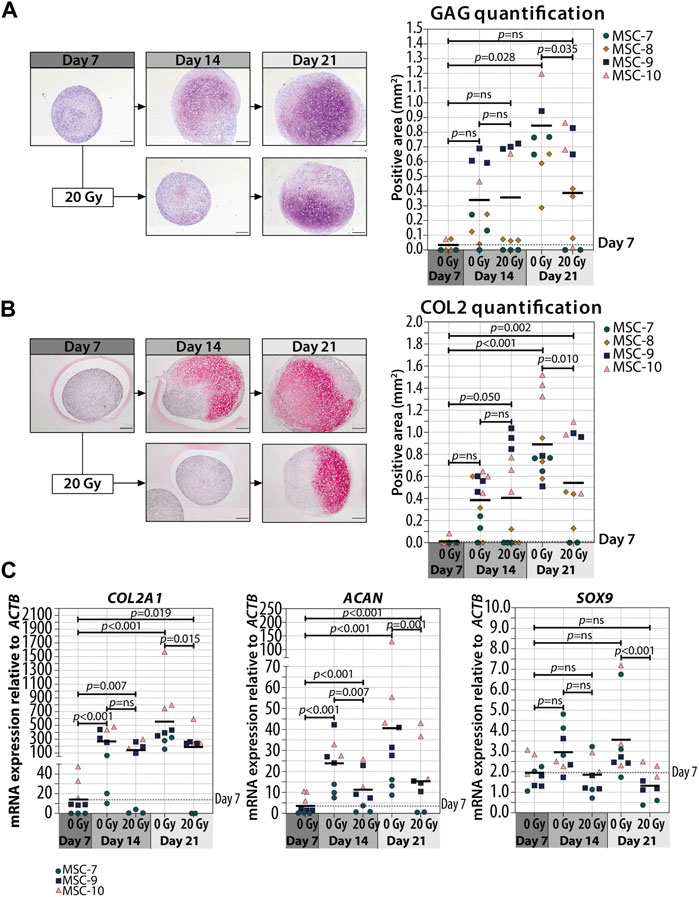
FIGURE 2. Irradiation with 20 Gy at day 7 during MSC differentiation reduced chondrogenic markers at day 21. (A–B; left panels) Representative images of (A) thionine (GAG) and (B) collagen type 2 (COL2) staining of MSC control pellets that were chondrogenically differentiated for 7, 14, and 21 days or MSC pellets that were irradiated at day 7 during chondrogenic differentiation and subsequently differentiated for days 7 or 14. The scale bar represents 200 µm. (A–B; right panels) Quantification of (A) GAG- or (B) COL2-positive area per condition in mm2. N = 4 donors with 1–3 replicates per donor. (C) Gene expression of chondrogenic markers in MSC control pellets that were chondrogenically differentiated for 7, 14, and 21 days or MSC pellets that were irradiated at day 7 during chondrogenic differentiation and subsequently differentiated for 7 and 14 days. Gene expression levels were normalized using ACTB. N = 3 donors with 2–3 replicates per donor. Data show individual data points and grand mean. p-values were obtained with the linear mixed model, using the different irradiation conditions as fixed parameters and the donors as random factors; ns = not significant.
Interestingly, when we irradiated the pellets at day 14, the deposition of GAG and COL2 did not change compared to non-irradiated controls (Figures 3A,B; Supplementary Figure S3). Similarly, the gene expressions of COL2A1, ACAN, and SOX9 at day 21 were comparable between day 14-irradiated pellets and controls (Figure 3C). Overall, these data suggest that the chondrogenesis of MSCs was not negatively influenced by irradiation at day 14. To test whether there was at least an effect on the known hypertrophic tendency of MSCs during chondrogenesis, COL10A1, ALPL, and RUNX2 expressions were analyzed. No differences in COL10A1, ALPL, and RUNX2 expressions were observed between day 14-irradiated and mock-treated pellets (Figure 3D). Although with donor variation, these data suggest that senescence during early differentiation (day 7) inhibits chondrogenic maturation, while senescence during late chondrogenesis (day 14) has no evident effect.
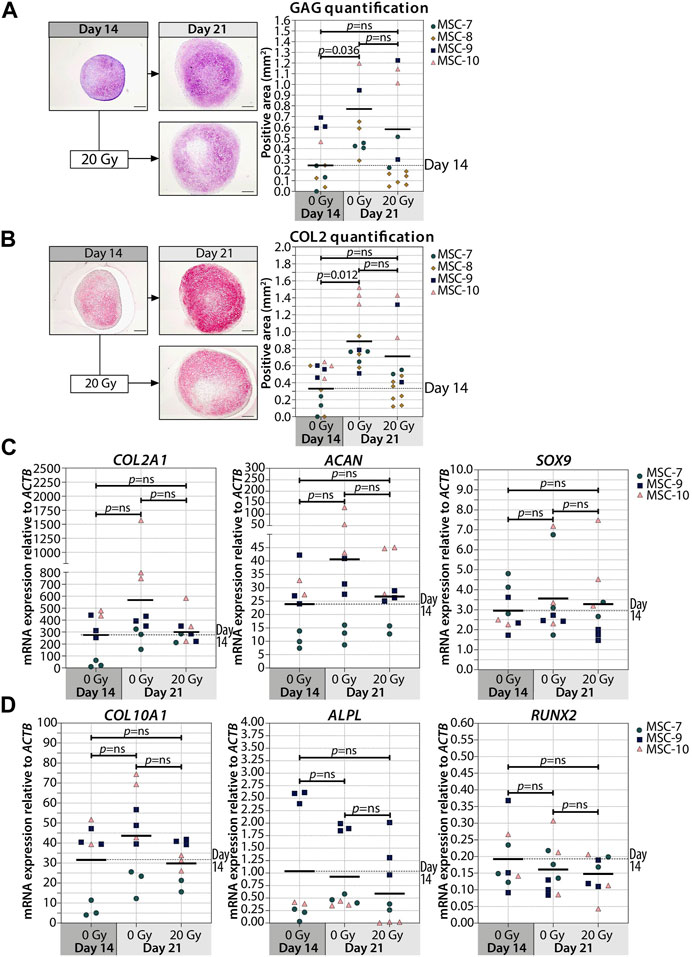
FIGURE 3. Irradiation at day 14 during MSC differentiation did not alter chondrogenic markers at day 21. (A–B; left panels) Representative images of (A) thionine (GAG) and (B) collagen type 2 (COL2) staining of MSC control pellets that were chondrogenically differentiated for 14 and 21 days or MSC pellets that were irradiated at day 14 during chondrogenic differentiation and subsequently differentiated for day 7. The scale bar represents 200 µm. (A-B; right panels) Quantification of (A) GAG- or (B) COL2-positive area per condition in mm2. N = 4 donors with 1–7 replicates per donor. (C, D) Gene expression of (C) chondrogenic markers and (D) hypertrophic markers in MSC control pellets that were chondrogenically differentiated for 14 and 21 days or MSC pellets that were irradiated at day 14 during chondrogenic differentiation and subsequently differentiated for 7 days. Gene expression levels were normalized using ACTB. N = 3 donors with 2–3 replicates per donor. Data show individual data points and grand mean. p-values were obtained with the linear mixed model, using the different irradiation conditions as fixed parameters and the donors as random factors; ns = not significant.
3.3 Conditioned medium of senescent pellets had no major effect on cartilage formation
Senescent cells can affect their surrounding cells via the secretion of a SASP (Coppé et al., 2010). To investigate whether or not the SASP contributes to reduced cartilage formation in chondrogenic pellets, the conditioned medium of control and senescent pellets during chondrogenic differentiation (days 5 and 6, and days 12 and 13) was generated and added to non-irradiated recipient chondrogenic pellets at day 7 or 14 (Figure 4A). First, we confirmed an increased expression of selected SASP factors IL6 (p < 0.001) and MMP3 (p < 0.001) in the irradiated pellets compared to non-irradiated control pellets (Figure 4B). Next, after exposition to the conditioned media of senescent pellets, we observed that COL2A1, ACAN, SOX9, and COL1A1 expressions were not significantly different compared to the control pellets cultured in control conditioned media (Figure 4C), suggesting that factors secreted from senescent cells during chondrogenesis do not directly alter the expression of chondrogenic genes in recipient pellets under our experimental conditions. To understand whether the absence of changes in the expression of chondrogenic markers was influenced by an altered expression in catabolic genes, we analyzed the expression of MMP13, MMP1, MMP3, and ADAMTS4. Pellets cultured in the conditioned medium of irradiated pellets had similar expression of catabolic genes as pellets cultured in the control conditioned medium at both days 9 and 16 of chondrogenic differentiation (Figure 4C). Overall, these results suggest that the SASP factors produced by senescent cells in the pellets have no major effect at different stages of cartilage formation.
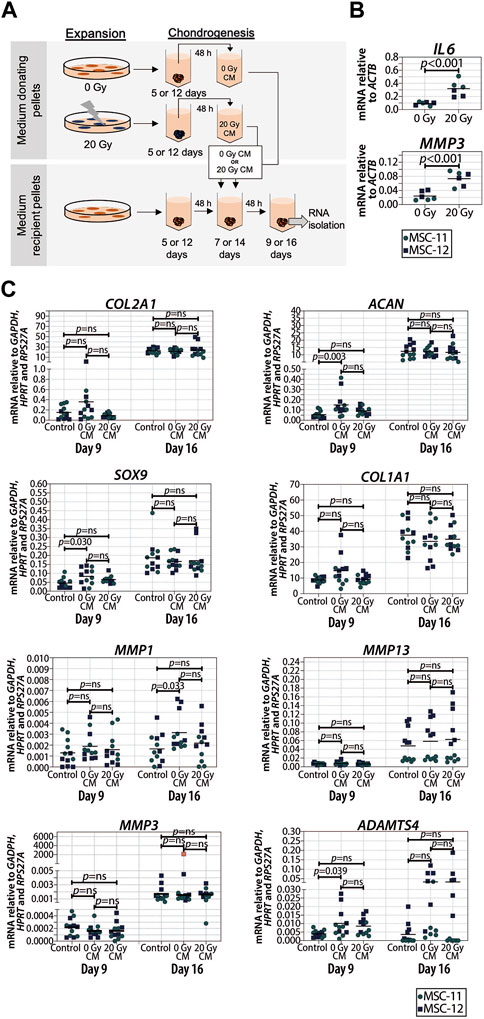
FIGURE 4. Conditioned medium from pellets of senescent MSCs did not alter the expression of chondrogenic genes in recipient non-senescent pellets. (A) Schematic overview of the experimental setup. (B) mRNA expression of day 16 of chondrogenically differentiated pellets from 20 Gy gamma-irradiated (during monolayer expansion) or not irradiated MSCs. N = 2 donors with three replicates per donor. (C) mRNA expression of MSC pellets that were chondrogenically differentiated for 9 or 16 days and treated with the conditioned medium for the last 48 h. The conditioned medium was obtained from chondrogenic pellets from MSCs that were irradiated with 20 Gy or not irradiated during expansion. Gene expression levels were normalized using the best housekeeping index (BHI; GAPDH, HPRT and RPS27A). MMP3 data show one outlier in red. This value was excluded from the statistical analysis. N = 2 donors with six replicates per donor. Data show individual data points and grand mean. p-values were obtained with the linear mixed model, using the different irradiation conditions as fixed parameters and the donors as random factors; ns = not significant.
3.4 The number of senescent cells is associated with a reduced cartilage production
Next, we asked if the observed negative effect on chondrogenesis was dependent on the number of senescent cells present at the moment of pellet formation. To answer these questions, we generated pellets starting with a different ratio of irradiated and non-irradiated cells and we monitored their chondrogenic differentiation capacity. The number of irradiation-induced senescent cells prior to chondrogenic differentiation was indeed associated with a reduced GAG and COL2 deposition (Figures 5A,B; Supplementary Figure S4), and both GAG and DNA contents in chondrogenic pellets were negatively associated with the number of senescent MSCs (Supplementary Figure S4B,C). MSC pellets with 20%–30% senescent MSCs had an average of 42% lower GAG content than pellets with non-irradiated cells (Supplementary Figure S4; p = 0.008), suggesting that a low percentage of senescent cells already have a significant effect on the GAG deposition. MSC pellets with 45%–55% senescent MSCs had, on average, 55% lower GAG/DNA than pellets with non-irradiated cells (Supplementary Figure S4; p = 0.003), indicating that the non-senescent MSCs were still able to deposit GAG in the mixed pellets. Histological analysis clearly showed reduced GAG and COL2 deposition in MSC pellets with 45%–55% senescent MSCs compared to pellets with non-irradiated cells (Figures 5A,B; Supplementary Figure S5). Furthermore, MSC pellets with 45%–55% senescent MSCs had lower expression of COL2A1, SOX9, and ACAN at day 21 of chondrogenic differentiation than non-senescent control MSCs, albeit not statistically significant (Figure 5C). MSC pellets with 70%–80% senescent MSCs had a lower GAG content than MSC pellets with 45%–55% senescent MSCs; however, these pellets still deposited GAG (Supplementary Figure S4). On the other hand, pellets with more than 90% senescent cells did not deposit GAG (Figures 5A,B). These pellets also had a low expression of COL2A1 (97% reduced compared to non-senescent control MSCs; p = 0.013), SOX9 (75% reduced compared to non-senescent control MSCs, p = 0.002), and ACAN (97% reduced compared to non-senescent control MSCs, p = 0.014). No significant differences in the expression of COL1A1 and the catabolic markers MMP13, MMP1, MMP3, and ADAMTS4 were observed between the different conditions (Figures 5D,E). These data may suggest that there is an inverse association between the number of senescent cells and the ability of generating cartilage.
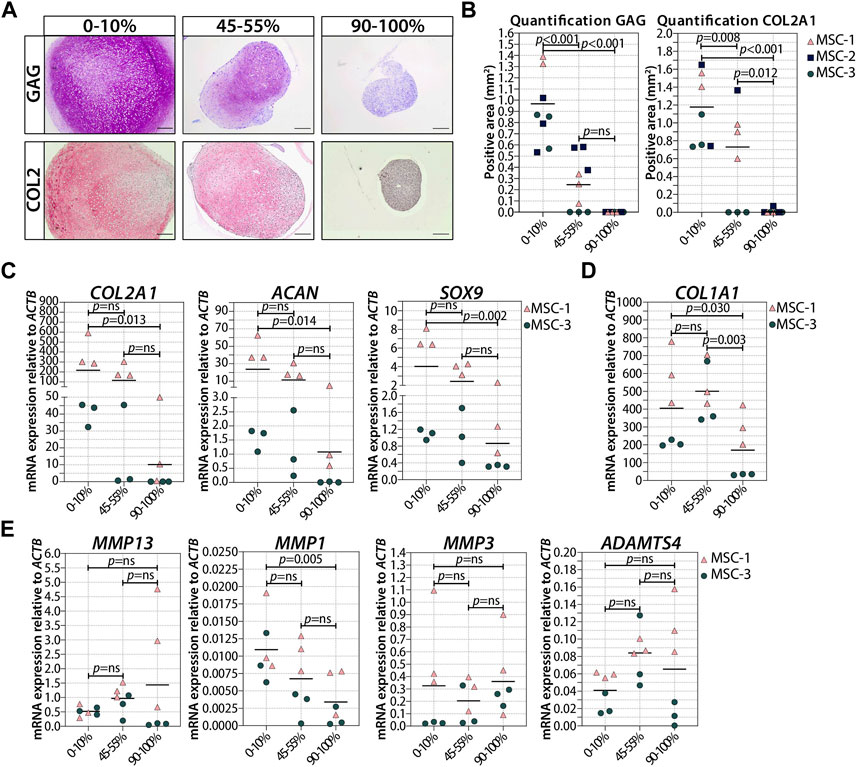
FIGURE 5. Higher ratio of senescent to non-senescent MSC resulted in less cartilage markers. (A, B) Representative images of thionine and collagen type 2 staining of MSCs that were gamma-irradiated during expansion with 0 or 20Gy, mixed, and subsequently chondrogenically differentiated for 21 days. The scale bar represents 200 µm. N = 3 donor with 2–3 pellets per donor. (C–E) mRNA expression of MSC pellets that were gamma-irradiated during expansion with 0 or 20Gy, mixed, and subsequently chondrogenically differentiated for 21 days. Gene expression levels were normalized using ACTB. Data show individual data points and grand mean. p-values were obtained with the linear mixed model, using the experimental conditions as fixed parameters and the donors as random factors; ns = not significant.
3.5 Senescent MSCs are less responsive to TGFβ signaling
TGFβ is the main driver of chondrogenesis in MSCs. In order to understand the reason why senescent cells have a reduced capacity to differentiate toward the chondrogenic lineage, we analyzed the TGFβ signaling activation by detecting the pSMAD2 levels in both irradiated MSCs (20 Gy) and control MSCs (0 Gy) upon TGFβ1 stimulation. In the presence of TGFβ1, pSMAD2 levels were higher in non-irradiated control MSCs than those in irradiated MSCs (Figure 6A; +TGFβ; Figure 6B; 6.9-fold, p = 0.020), while no detectable pSMAD2 levels were present in MSCs without TGFβ1 stimulation (Figure 6A; -TGFβ). These data suggest that senescent MSCs are less responsive to TGFβ1, indicating that the reduced chondrogenic potential may be caused by a cell-intrinsic mechanism.
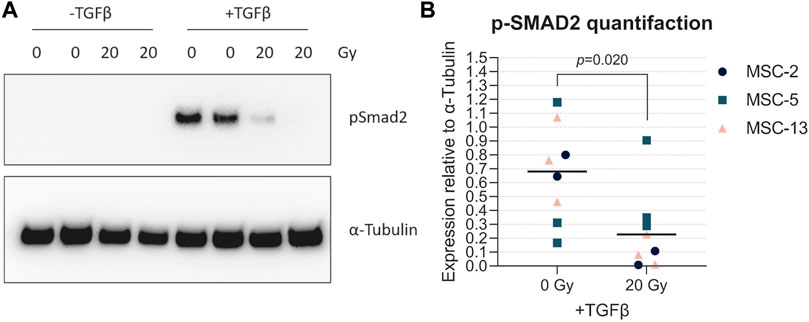
FIGURE 6. Senescent MSCs had low TGFβ-induced phosphorylated SMAD2 levels. (A) Western blot for phosphorylated SMAD2 (p-SMAD2). (B) Quantification of Western blot results relative to α-tubulin. N = 3 donors with 2–3 biological replicates per donor. Data show individual data points and grand mean. p-values were obtained with the linear mixed model, using the different experimental conditions as fixed parameters and the donors as random factors.
4 Discussion
MSCs are promising cells for cartilage tissue regeneration therapies. To obtain reproducible and safe clinical outcomes, it is necessary to understand how the chondrogenic differentiation capacity in MSC populations is regulated. In this study, we demonstrated that cellular senescence impairs the chondrogenic differentiation capacity of MSCs, showed the association between the number of senescent cells at the start of the culture and the reduced chondrogenic differentiation potential, and observed that senescent cells have a reduced ability to respond to TGFβ, the main factor responsible for chondrogenic differentiation of MSCs.
MSCs are a heterogeneous population of cells, and the number of senescent cells varies between MSC cultures from different patients (Schellenberg et al., 2012) and, most importantly, with passaging in vitro (Bonab et al., 2006; Lehmann et al., 2022; Wang et al., 2022). Here, we show for the first time that an increased number of senescent cells contribute to a reduced chondrogenic differentiation potential, indicating that the appearance of cellular senescence can contribute to heterogeneity in chondrogenic differentiation between MSC populations. This may also be linked with our previous observation that different MSC subtypes have a distinct differentiation capacity (Sivasubramaniyan et al., 2018; Sivasubramaniyan et al., 2019). Furthermore, we show that in a mixed population with senescent MSCs, non-senescent MSCs are still able to differentiate toward the chondrogenic lineage and that the secretome of the senescent cells does not grossly influence the differentiation of neighboring non-senescent cells.
Our results suggest that senescent MSCs, while losing their chondrogenic differentiation potential, generally keep their osteogenic and adipogenic differentiation capacity. However, we identified some differences between gene expression and staining, specifically for the osteogenic assay. Although mineral deposition seems slightly increased in irradiated MSCs, gene expression levels for osteogenic markers remain unaffected. This may explain why in the literature there is still no uniformed consensus on the effect of senescence in MSCs, with authors claiming a minimal effect on osteogenic differentiation in late-passaged cells (Bonab et al., 2006), and others claiming upregulation (Wagner et al., 2008) or even downregulation of osteogenic differentiation (Geissler et al., 2012; Despars et al., 2013; Abuna et al., 2016; Hu et al., 2022) with passaging or senescence. This discrepancy might also be linked with the timing of senescence induction during the experiments or could possibly be due to the different ways to induce senescence. Indeed, we and others previously observed different senescence phenotypes depending on the way senescence was induced (Wiley et al., 2016; Voskamp et al., 2021; Wechter et al., 2023), and we cannot exclude that this may have a different impact on MSC differentiation.
In this study, we demonstrated that the effect of irradiation-induced cellular senescence is largest during the early phases of chondrogenic differentiation. It has been shown that proliferation during the early phase of chondrogenesis is essential for proper chondrogenic differentiation (Dexheimer et al., 2012). This indicates that impaired proliferation could be an explanation why MSCs failed to differentiate toward the chondrogenic lineage, specifically when senescence is induced in a monolayer or early during differentiation. Another explanation could be related to the differences we observed in TGFβ signaling pathway activation in senescent MSCs compared to non-senescent MSCs. TGFβ signaling plays an important role in cartilage development and cartilage homeostasis (Thielen et al., 2019). Particularly, in the early phases of re-differentiation, Smad2/3 phosphorylation is essential for chondrogenesis of MSCs (Hellingman et al., 2011) and for re-differentiation of de-differentiated chondrocytes (Narcisi et al., 2012). Here, we demonstrated that senescent MSCs have reduced pSMAD2 levels after TGFβ1 stimulation, compared to non-senescent control MSCs, suggesting that canonical TGFβ signaling is altered in senescent MSCs. However, other non-canonical TGFβ pathways may also be involved in the process of cellular senescence and need further investigations.
It is known that senescent cells can affect the surrounding cells and tissues via their secretome. Previously, it has been shown that implantation of senescent cells can contribute to an OA-like phenotype in mice (Xu et al., 2017). In order to safely use MSCs for cartilage repair strategies, it is crucial to understand whether the SASP factors released by senescence cells can limit chondrogenesis or even contribute to cartilage degeneration. In this study, we found that the conditioned medium of chondrogenic pellets of senescent MSCs had no direct effect on the expression of the chondrogenic (COL2A1, ACAN, and SOX9) or the catabolic (MMP1, MMP13, MMP3, or ADAMTS4) markers in recipient pellet cultures. These data indicate that in our in vitro model, the SASP factors released from senescent MSCs neither have a negative effect on MSC chondrogenesis nor on the matrix degradation processes. Despite the absence of a direct effect on the MSCs exposed to the medium of senescent MSCs, we did find that senescent MSCs in the pellets had higher expression levels of inflammatory factors IL6 and MMP3. The role of IL6 in cartilage tissue is controversial since it has been shown to stimulate both cartilage degeneration and synthesis (Porée et al., 2008; Ryu et al., 2011; Tsuchida et al., 2012; Liao et al., 2022), and MMP3 promotes cartilage loss via degradation of multiple extracellular matrix components (Murphy and Lee, 2005), indicating that the SASP factors released by MSCs could, thus, also contribute to the pathophysiology of OA.
On the other hand, the SASP factors have been shown to be essential for limb regeneration via the recruitment of macrophages (Godwin et al., 2013). The fact that SASP could be beneficial for tissue regeneration is further supported by Biswas et al., showing that the SASP factor IL6 can trigger Myh11+ pericytes to expand and differentiate into chondrocytes and osteoblasts via induction of lymphangiogenesis in bone tissue (Biswas et al., 2023). Although lymphatic vessels are not observed in cartilage tissue, they are present in the subchondral bone (Biswas et al., 2023) and soft tissues around the knee joint (Shi et al., 2014), highlighting the importance to study the effect of the SASP crosstalk between cartilage and the surrounding tissues. In general, more studies specifically focused on the role of individual SASP factors are necessary to better understand their role in cartilage generation and degeneration, as well as possible interventions to counteract these effects.
In this study, we explored how senescence in MSCs affects the chondrogenesis process. We showed that the number of senescent cells in MSC cultures is associated with a reduced chondrogenic differentiation potential. Particularly, senescence in the early phase of chondrogenesis could be detrimental for MSC-based cartilage tissue engineering. Therefore, strategies that prevent or abolish senescence in MSCs could be beneficial for MSC-based cartilage repair.
Data availability statement
The raw data supporting the conclusion of this article will be made available by the authors, without undue reservation.
Ethics statement
The studies involving humans were approved by the Erasmus Medical Ethical Committee (MEC-2014-16). The studies were conducted in accordance with the local legislation and institutional requirements. The human samples used in this study were acquired from a by- product of routine care or industry. Written informed consent for participation was not required from the participants or the participants’ legal guardians/next of kin in accordance with the national legislation and institutional requirements.
Author contributions
Study conception, design, analysis, and final approval of the article: CV, WK, GV, and RN. Drafting of the article: CV, GO, and RN. All authors contributed to the article and approved the submitted version.
Funding
This research was financially supported by the Dutch Arthritis Society (ReumaNederland; 16-1-201) and by a TTW Perspectief grant from NWO (William Hunter Revisited; P15-23). This study is part of the Medical Delta RegMed4D program.
Conflict of interest
The authors declare that the research was conducted in the absence of any commercial or financial relationships that could be construed as a potential conflict of interest.
Publisher’s note
All claims expressed in this article are solely those of the authors and do not necessarily represent those of their affiliated organizations, or those of the publisher, the editors, and the reviewers. Any product that may be evaluated in this article, or claim that may be made by its manufacturer, is not guaranteed or endorsed by the publisher.
Supplementary material
The Supplementary Material for this article can be found online at: https://www.frontiersin.org/articles/10.3389/fbioe.2023.1241338/full#supplementary-material
References
Abuna, R. P., Stringhetta-Garcia, C. T., Fiori, L. P., Dornelles, R. C., Rosa, A. L., and Beloti, M. M. (2016). Aging impairs osteoblast differentiation of mesenchymal stem cells grown on titanium by favoring adipogenesis. J. Appl. Oral Sci. 24, 376–382. doi:10.1590/1678-775720160037
Acosta, J. C., Banito, A., Wuestefeld, T., Georgilis, A., Janich, P., Morton, J. P., et al. (2013). A complex secretory program orchestrated by the inflammasome controls paracrine senescence. Nat. Cell. Biol. 15, 978–990. doi:10.1038/ncb2784
Banfi, A., Bianchi, G., Notaro, R., Luzzatto, L., Cancedda, R., and Quarto, R. (2002). Replicative aging and gene expression in long-term cultures of human bone marrow stromal cells. Tissue Eng. 8, 901–910. doi:10.1089/107632702320934001
Basisty, N., Kale, A., Jeon, O. H., Kuehnemann, C., Payne, T., Rao, C., et al. (2020). A proteomic atlas of senescence-associated secretomes for aging biomarker development. PLoS Biol. 18, e3000599. doi:10.1371/journal.pbio.3000599
Biswas, L., Chen, J., De Angelis, J., Singh, A., Owen-Woods, C., Ding, Z., et al. (2023). Lymphatic vessels in bone support regeneration after injury. Cell. 186, 382–397 e24. doi:10.1016/j.cell.2022.12.031
Bonab, M. M., Alimoghaddam, K., Talebian, F., Ghaffari, S. H., Ghavamzadeh, A., and Nikbin, B. (2006). Aging of mesenchymal stem cell in vitro. BMC Cell. Biol. 7, 14. doi:10.1186/1471-2121-7-14
Chung, Y. P., Chen, Y. W., Weng, T. I., Yang, R. S., and Liu, S. H. (2020). Arsenic induces human chondrocyte senescence and accelerates rat articular cartilage aging. Arch. Toxicol. 94, 89–101. doi:10.1007/s00204-019-02607-2
Coppé, J. P., Desprez, P. Y., Krtolica, A., and Campisi, J. (2010). The senescence-associated secretory phenotype: The dark side of tumor suppression. Annu. Rev. Pathol. 5, 99–118. doi:10.1146/annurev-pathol-121808-102144
Despars, G., Carbonneau, C. L., Bardeau, P., Coutu, D. L., and Beauséjour, C. M. (2013). Loss of the osteogenic differentiation potential during senescence is limited to bone progenitor cells and is dependent on p53. PLoS One 8, e73206. doi:10.1371/journal.pone.0073206
Dexheimer, V., Frank, S., and Richter, W. (2012). Proliferation as a requirement for in vitro chondrogenesis of human mesenchymal stem cells. Stem Cells Dev. 21, 2160–2169. doi:10.1089/scd.2011.0670
Farndale, R. W., Buttle, D. J., and Barrett, A. J. (1986). Improved quantitation and discrimination of sulphated glycosaminoglycans by use of dimethylmethylene blue. Biochim. Biophys. Acta 883, 173–177. doi:10.1016/0304-4165(86)90306-5
Geissler, S., Textor, M., Kühnisch, J., Könnig, D., Klein, O., Ode, A., et al. (2012). Functional comparison of chronological and in vitro aging: Differential role of the cytoskeleton and mitochondria in mesenchymal stromal cells. PLoS One 7, e52700. doi:10.1371/journal.pone.0052700
Godwin, J. W., Pinto, A. R., and Rosenthal, N. A. (2013). Macrophages are required for adult salamander limb regeneration. Proc. Natl. Acad. Sci. U. S. A. 110, 9415–9420. doi:10.1073/pnas.1300290110
Greene, M. A., and Loeser, R. F. (2015). Aging-related inflammation in osteoarthritis. Osteoarthr. Cartil. 23, 1966–1971. doi:10.1016/j.joca.2015.01.008
Hayflick, L., and Moorhead, P. S. (1961). The serial cultivation of human diploid cell strains. Exp. Cell. Res. 25, 585–621. doi:10.1016/0014-4827(61)90192-6
Hellingman, C. A., Davidson, E. N., Koevoet, W., Vitters, E. L., Van Den Berg, W. B., Van Osch, G. J., et al. (2011). Smad signaling determines chondrogenic differentiation of bone-marrow-derived mesenchymal stem cells: Inhibition of smad1/5/8P prevents terminal differentiation and calcification. Tissue Eng. Part A 17, 1157–1167. doi:10.1089/ten.tea.2010.0043
Hernandez-Segura, A., Nehme, J., and Demaria, M. (2018). Hallmarks of cellular senescence. Trends Cell. Biol. 28, 436–453. doi:10.1016/j.tcb.2018.02.001
Hernandez-Segura, A., Rubingh, R., and Demaria, M. (2019). Identification of stable senescence-associated reference genes. Aging Cell. 18, e12911. doi:10.1111/acel.12911
Hu, M., Xing, L., Zhang, L., Liu, F., Wang, S., Xie, Y., et al. (2022). NAP1L2 drives mesenchymal stem cell senescence and suppresses osteogenic differentiation. Aging Cell. 21, e13551. doi:10.1111/acel.13551
Im, G. I., Jung, N. H., and Tae, S. K. (2006). Chondrogenic differentiation of mesenchymal stem cells isolated from patients in late adulthood: The optimal conditions of growth factors. Tissue Eng. 12, 527–536. doi:10.1089/ten.2006.12.527
Josephson, A. M., Bradaschia-Correa, V., Lee, S., Leclerc, K., Patel, K. S., Muinos Lopez, E., et al. (2019). Age-related inflammation triggers skeletal stem/progenitor cell dysfunction. Proc. Natl. Acad. Sci. U. S. A. 116, 6995–7004. doi:10.1073/pnas.1810692116
Kim, M., Kim, C., Choi, Y. S., Kim, M., Park, C., and Suh, Y. (2012). Age-related alterations in mesenchymal stem cells related to shift in differentiation from osteogenic to adipogenic potential: Implication to age-associated bone diseases and defects. Mech. Ageing Dev. 133, 215–225. doi:10.1016/j.mad.2012.03.014
Knuth, C. A., Kiernan, C. H., Palomares Cabeza, V., Lehmann, J., Witte-Bouma, J., Ten Berge, D., et al. (2018). Isolating pediatric mesenchymal stem cells with enhanced expansion and differentiation capabilities. Tissue Eng. Part C Methods 24, 313–321. doi:10.1089/ten.tec.2018.0031
Lehmann, J., Narcisi, R., Franceschini, N., Chatzivasileiou, D., Boer, C. G., Koevoet, W., et al. (2022). WNT/beta-catenin signalling interrupts a senescence-induction cascade in human mesenchymal stem cells that restricts their expansion. Cell. Mol. Life Sci. 79, 82. doi:10.1007/s00018-021-04035-x
Liao, Y., Ren, Y., Luo, X., Mirando, A. J., Long, J. T., Leinroth, A., et al. (2022). Interleukin-6 signaling mediates cartilage degradation and pain in posttraumatic osteoarthritis in a sex-specific manner. Sci. Signal 15, eabn7082. doi:10.1126/scisignal.abn7082
Makris, E. A., Gomoll, A. H., Malizos, K. N., Hu, J. C., and Athanasiou, K. A. (2015). Repair and tissue engineering techniques for articular cartilage. Nat. Rev. Rheumatol. 11, 21–34. doi:10.1038/nrrheum.2014.157
Mankin, H. J. (1982). The response of articular cartilage to mechanical injury. J. Bone Jt. Surg. Am. 64, 460–466. doi:10.2106/00004623-198264030-00022
Murphy, G., and Lee, M. H. (2005). What are the roles of metalloproteinases in cartilage and bone damage? Ann. Rheum. Dis. 64 (4), iv44–7. doi:10.1136/ard.2005.042465
Narcisi, R., Signorile, L., Verhaar, J. A., Giannoni, P., and Van Osch, G. J. (2012). TGFβ inhibition during expansion phase increases the chondrogenic re-differentiation capacity of human articular chondrocytes. Osteoarthr. Cartil. 20, 1152–1160. doi:10.1016/j.joca.2012.06.010
Pelletier, J. P., Roughley, P. J., Dibattista, J. A., Mccollum, R., and Martel-Pelletier, J. (1991). Are cytokines involved in osteoarthritic pathophysiology? Semin. Arthritis Rheum. 20, 12–25. doi:10.1016/0049-0172(91)90024-t
Philipot, D., Guérit, D., Platano, D., Chuchana, P., Olivotto, E., Espinoza, F., et al. (2014). p16INK4a and its regulator miR-24 link senescence and chondrocyte terminal differentiation-associated matrix remodeling in osteoarthritis. Arthritis Res. Ther. 16, R58. doi:10.1186/ar4494
Pittenger, M. F., Mackay, A. M., Beck, S. C., Jaiswal, R. K., Douglas, R., Mosca, J. D., et al. (1999). Multilineage potential of adult human mesenchymal stem cells. Science 284, 143–147. doi:10.1126/science.284.5411.143
Porée, B., Kypriotou, M., Chadjichristos, C., Beauchef, G., Renard, E., Legendre, F., et al. (2008). Interleukin-6 (IL-6) and/or soluble IL-6 receptor down-regulation of human type II collagen gene expression in articular chondrocytes requires a decrease of Sp1.Sp3 ratio and of the binding activity of both factors to the COL2A1 promoter. J. Biol. Chem. 283, 4850–4865. doi:10.1074/jbc.m706387200
Ryu, J. H., Yang, S., Shin, Y., Rhee, J., Chun, C. H., and Chun, J. S. (2011). Interleukin-6 plays an essential role in hypoxia-inducible factor 2α-induced experimental osteoarthritic cartilage destruction in mice. Arthritis Rheum. 63, 2732–2743. doi:10.1002/art.30451
Sakaguchi, Y., Sekiya, I., Yagishita, K., and Muneta, T. (2005). Comparison of human stem cells derived from various mesenchymal tissues: Superiority of synovium as a cell source. Arthritis Rheum. 52, 2521–2529. doi:10.1002/art.21212
Schellenberg, A., Stiehl, T., Horn, P., Joussen, S., Pallua, N., Ho, A. D., et al. (2012). Population dynamics of mesenchymal stromal cells during culture expansion. Cytotherapy 14, 401–411. doi:10.3109/14653249.2011.640669
Severino, V., Alessio, N., Farina, A., Sandomenico, A., Cipollaro, M., Peluso, G., et al. (2013). Insulin-like growth factor binding proteins 4 and 7 released by senescent cells promote premature senescence in mesenchymal stem cells. Cell. Death Dis. 4, e911. doi:10.1038/cddis.2013.445
Shapiro, F., Koide, S., and Glimcher, M. J. (1993). Cell origin and differentiation in the repair of full-thickness defects of articular cartilage. J. Bone Jt. Surg. Am. 75, 532–553. doi:10.2106/00004623-199304000-00009
Shi, J., Liang, Q., Zuscik, M., Shen, J., Chen, D., Xu, H., et al. (2014). Distribution and alteration of lymphatic vessels in knee joints of normal and osteoarthritic mice. Arthritis Rheumatol. 66, 657–666. doi:10.1002/art.38278
Sivasubramaniyan, K., Ilas, D. C., Harichandan, A., Bos, P. K., Santos, D. L., De Zwart, P., et al. (2018). Bone marrow-harvesting technique influences functional heterogeneity of mesenchymal stem/stromal cells and cartilage regeneration. Am. J. Sports Med. 46, 3521–3531. doi:10.1177/0363546518804807
Sivasubramaniyan, K., Koevoet, W., Hakimiyan, A. A., Sande, M., Farrell, E., Hoogduijn, M. J., et al. (2019). Cell-surface markers identify tissue resident multipotential stem/stromal cell subsets in synovial intimal and sub-intimal compartments with distinct chondrogenic properties. Osteoarthr. Cartil. 27, 1831–1840. doi:10.1016/j.joca.2019.08.006
Stenderup, K., Justesen, J., Clausen, C., and Kassem, M. (2003). Aging is associated with decreased maximal life span and accelerated senescence of bone marrow stromal cells. Bone 33, 919–926. doi:10.1016/j.bone.2003.07.005
Thielen, N. G. M., Van Der Kraan, P. M., and Van, C. A. A. M. (2019). TGFβ/BMP signaling pathway in cartilage homeostasis. Cells 8, 969. doi:10.3390/cells8090969
Tsuchida, A. I., Beekhuizen, M., Rutgers, M., Van Osch, G. J., Bekkers, J. E., Bot, A. G., et al. (2012). Interleukin-6 is elevated in synovial fluid of patients with focal cartilage defects and stimulates cartilage matrix production in an in vitro regeneration model. Arthritis Res. Ther. 14, R262. doi:10.1186/ar4107
Voskamp, C., Anderson, L. A., Koevoet, W. J., Barnhoorn, S., Mastroberardino, P. G., Van Osch, G. J., et al. (2021). TWIST1 controls cellular senescence and energy metabolism in mesenchymal stem cells. Eur. Cell. Mater 42, 401–414. doi:10.22203/ecm.v042a25
Wagner, W., Horn, P., Castoldi, M., Diehlmann, A., Bork, S., Saffrich, R., et al. (2008). Replicative senescence of mesenchymal stem cells: A continuous and organized process. PLoS One 3, e2213. doi:10.1371/journal.pone.0002213
Wang, N., He, Y., Liu, S., Makarcyzk, M. J., Lei, G., Chang, A., et al. (2022). Engineering osteoarthritic cartilage model through differentiating senescent human mesenchymal stem cells for testing disease-modifying drugs. Sci. China Life Sci. 65, 309–327. doi:10.1007/s11427-021-1933-7
Wechter, N., Rossi, M., Anerillas, C., Tsitsipatis, D., Piao, Y., Fan, J., et al. (2023). Single-cell transcriptomic analysis uncovers diverse and dynamic senescent cell populations. Aging (Albany NY) 15, 2824–2851. doi:10.18632/aging.204666
Wehling, N., Palmer, G. D., Pilapil, C., Liu, F., Wells, J. W., Müller, P. E., et al. (2009). Interleukin-1β and tumor necrosis factor α inhibit chondrogenesis by human mesenchymal stem cells through NF-κB-dependent pathways. Arthritis Rheum. 60, 801–812. doi:10.1002/art.24352
Wiley, C. D., Velarde, M. C., Lecot, P., Liu, S., Sarnoski, E. A., Freund, A., et al. (2016). Mitochondrial dysfunction induces senescence with a distinct secretory phenotype. Cell. Metab. 23, 303–314. doi:10.1016/j.cmet.2015.11.011
Keywords: senescence, cartilage, MSC, chondrogenesis, SASP, regenerative medicine, tissue engineering, TGFβ
Citation: Voskamp C, Koevoet WJLM, Van Osch GJVM and Narcisi R (2023) Senescence during early differentiation reduced the chondrogenic differentiation capacity of mesenchymal progenitor cells. Front. Bioeng. Biotechnol. 11:1241338. doi: 10.3389/fbioe.2023.1241338
Received: 16 June 2023; Accepted: 25 July 2023;
Published: 07 August 2023.
Edited by:
J. Mary Murphy, University of Galway, IrelandReviewed by:
Gina Lisignoli, Rizzoli Orthopedic Institute (IRCCS), ItalyAnjali P. Kusumbe, University of Oxford, United Kingdom
Copyright © 2023 Voskamp, Koevoet, Van Osch and Narcisi. This is an open-access article distributed under the terms of the Creative Commons Attribution License (CC BY). The use, distribution or reproduction in other forums is permitted, provided the original author(s) and the copyright owner(s) are credited and that the original publication in this journal is cited, in accordance with accepted academic practice. No use, distribution or reproduction is permitted which does not comply with these terms.
*Correspondence: Roberto Narcisi, ci5uYXJjaXNpQGVyYXNtdXNtYy5ubA==; Gerjo J. V. M. Van Osch, Zy52YW5vc2NoQGVyYXNtdXNtYy5ubA==
†These authors have contributed equally to this work and share last authorship