- 1Department of Radiology & Nuclear Medicine and Molecular Imaging, University Medical Center Groningen, Groningen, Netherlands
- 2Department for Biomedical Sciences, Faculty of Health and Medical Sciences, University of Copenhagen, Copenhagen, Denmark
- 3Department of Surgery, University Medical Center Groningen, Groningen, Netherlands
- 4Department of Radiology, Charité University Medicine Berlin, Berlin, Germany
Stiffness plays a vital role in diagnosing renal fibrosis. However, perfusion influences renal stiffness in various chronic kidney diseases. Therefore, we aimed to characterize the effect of tissue perfusion on renal stiffness and tissue fluidity measured by tomoelastography based on multifrequency magnetic resonance elastography in an ex vivo model. Five porcine kidneys were perfused ex vivo in an MRI-compatible normothermic machine perfusion setup with adjusted blood pressure in the 50/10–160/120 mmHg range. Simultaneously, renal cortical and medullary stiffness and fluidity were obtained by tomoelastography. For the cortex, a statistically significant (p < 0.001) strong positive correlation was observed between both perfusion parameters (blood pressure and resulting flow) and stiffness (r = 0.95, 0.91), as well as fluidity (r = 0.96, 0.92). For the medulla, such significant (p < 0.001) correlations were solely observed between the perfusion parameters and stiffness (r = 0.88, 0.71). Our findings demonstrate a strong perfusion dependency of renal stiffness and fluidity in an ex vivo setup. Moreover, changes in perfusion are rapidly followed by changes in renal mechanical properties—highlighting the sensitivity of tomoelastography to fluid pressure and the potential need for correcting mechanics-derived imaging biomarkers when addressing solid structures in renal tissue.
Introduction
Renal fibrosis, triggered by an initial injury, is the ultimate hallmark of many chronic kidney diseases (CKD) (Liu, 2006; Hewitson, 2012). Regardless of the underlying cause, CKD is characterized by progressive renal scarring that ultimately affects all structures of the kidney as well as renal function. The relentless progression of CKD is postulated to result from a self-perpetuating vicious cycle of fibrosis activated after the initial injury, which can ultimately lead to end-stage renal failure requiring dialysis or kidney transplantation (Fogo, 2007; Anders et al., 2018). This cycle of fibrosis is characterized by the excess accumulation of extracellular matrix causing increased renal stiffness (Wynn and Ramalingam, 2012; Gandhi et al., 2019).
An invasive kidney biopsy is the diagnostic gold standard to measure renal fibrosis (Hysi and Yuen, 2020). Despite the risks of bleeding and sampling bias—at best less than 1% of the kidney is analyzed using this approach (Williams et al., 2012)—the histological evaluation of a kidney biopsy currently depicts the only clinical tool for nephrologists to assess fibrosis (Mansour et al., 2017). Other blood and urine based biomarkers such as serum creatinine (SCr) and albumin excretion rate (AER) are biased by dietary intake and many other factors and are not sufficiently specific to distinguish CKD from other kidney diseases (Lopez-Giacoman, 2015; Greenberg et al., 2018). Hence, there is an urgent need for improved non-invasive diagnostics to quantify renal fibrosis and its consequences in the kidney (Hysi and Yuen, 2020).
Magnetic resonance elastography (MRE) is an advanced quantitative magnetic resonance imaging (MRI) technique that is gaining popularity in diagnosing renal diseases (Venkatesh and Ehman, 2015; Kirpalani et al., 2017). It can detect changes in the viscoelastic properties of soft tissues, which occur during pathological processes (Venkatesh and Ehman, 2015; Kolipaka et al., 2016). Tomoelastography is a recently introduced MRE technique providing shear wave speed and phase angle of the complex shear modulus as surrogate markers of tissue stiffness and fluidity with high spatial fidelity (Tzschätzsch et al., 2016). Tissue stiffness is a measure of the resistance of tissue to deformation when a force is applied. Tissue fluidity reflects the ability of a tissue to deform plastically, to generate friction and absorb mechanical energy during deformation. Tissue fluidity is so named because it ranges from zero (elastic solid) to values found in pure liquids.
In contrast to traditional single-frequency MRE, which is currently used clinically for non-invasive assessment of liver fibrosis, tomoelastography uses wave fields with vibrations of multiple frequencies (Dittmann et al., 2017) being consecutively administered to the tissue. This multifrequency approach improves anatomical resolution, noise robustness, and intra-tissue homogeneity (Tzschätzsch et al., 2016). Previous studies have demonstrated the diagnostic accuracy of tomoelastography to quantify renal stiffness in different cohorts (Marticorena Garcia et al., 2018a; Marticorena Garcia et al., 2019; Marticorena Garcia et al., 2021). Moreover, correlations between renal stiffness in allografts and clinical markers such as glomerular filtration rate (GFR) and resistive index (RI) have been reported (Garcia et al., 2016). Despite these promising results, growing evidence indicates the influence of perfusion on tissue stiffness in addition to fibrosis (Warner et al., 2011; Gennisson et al., 2012; Yin et al., 2017; Gandhi et al., 2019; Brown et al., 2020). Garcia et al. (Marticorena Garcia et al., 2018b) showed increasing stiffness in the ex-vivo porcine renal cortex resulting from rising vascular pressure. However, since in this study, the vascular pressure was artificially altered through water inflow into the renal vein instead of physiological modulation of blood perfusion, the results may change in a more realistic scenario of kidney perfusion. Specifically, the relationship between MRE parameters and amount of renal perfusion needs to be studied in a controlled scenarion to understand and potentially correct perfusion effects on in vivo tomoelastography in the future.
Renal normothermic machine perfusion (NMP) is a revolutionary method for evaluating ex vivo kidney graft quality. This technique offers the possibility of creating an isolated near-physiological environment by circulating a perfusion solution at body temperature (35°C–37°C) together with oxygen and nutrients. The potential of NMP-derived markers in assessing the quality of a kidney prior to transplantation has been shown by different research groups (Hosgood et al., 2018; Kaths et al., 2018; Woud et al., 2019; Rigalli et al., 2020). However, due to varying NMP protocols and approaches, it is still unclear which biomarkers during perfusion can reliably indicate the organ’s viability ex vivo (Dittmann et al., 2016).
In this study, we investigated whether blood pressure and flow influence the stiffness and fluidity in the cortex and medulla of ex vivo perfused porcine kidneys. To answer this question, we assessed the shear wave speed and phase angle of the shear modulus in five porcine kidneys, perfused ex vivo in an MRI-compatible NMP circuit with various blood pressures and flows. To the best of our knowledge, this is the first study to evaluate the dependency of perfusion on renal mechanical properties in NMP ex vivo kidneys using tomoelastography.
Materials and methods
Organ procurement and renal NMP
Five viable porcine kidneys were retrieved en bloc 20 min after cardiac arrest from three healthy pigs (Dutch landrace pigs, around 130 kg) in a local slaughterhouse in accordance with all guidelines of the Dutch food safety authority. Subsequently, kidneys were preserved using a pressure-controlled oxygenated hypothermic (1°C–10°C) machine perfusion (O-HMP) (Kidney Assist Transport®, XVIVO, Göteborg, Sweden), primed with cold University of Wisconsin Machine Perfusion Solution (Belzer MPS®, Bridge to Life, Columbia, United States), at a pressure of 25 mmHg. Due to the time interval between organ procurement and MRI scanner availability, the kidneys were preserved using O-HMP for 10 h with an oxygen flow rate of 0.1 L/min, mimicking clinical cold organ preservation.
At least 1 hour prior to the tomoelastography scan, each kidney was separately perfused with a solution composed of isolated autologous red blood cells (RBCs), crystalloids, albumin, creatinine, antibiotics, and electrolyte supplementation at 35°C–37°C in an MRI compatible NMP circuit (see Figure 1). Oxygenation was administered with carbogen (95% O2/5% CO2) at a rate of 0.5 L/min using a clinical-grade oxygenator (Hilite 800 LT, Medos Medizintechnik AG, Stolberg, Germany). The kidney was positioned inside a custom-built organ chamber (LifePort®, Organ Recovery Systems, Itasca, IL, United States) in the isocenter of the MRI scanner and connected to the other perfusion hardware in the scanner’s control room with 7.5 m long polyvinylchloride tubing.
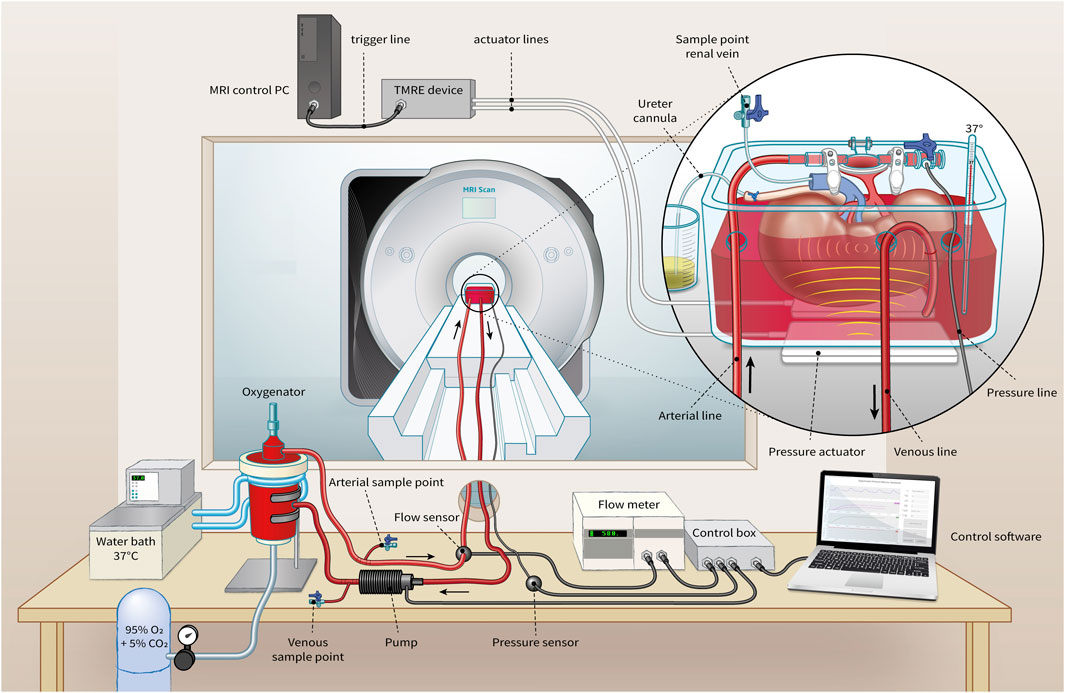
FIGURE 1. Representation of MRI-compatible NMP setup for ex vivo kidney perfusion including Tomoelastography setup. Pressure actuators induce compression wave (yellow) to the organ chamber, evoking shear waves (light red) in the kidney.
Blood pressure was controlled through a centrifugal pump (Medos Medizintechnik AG, Stolberg, Germany) using custom-made hard- and software (LabView Software, National Instruments Netherlands B.V., Woerden, the Netherlands). Perfusion pressure was continuously measured in the arterial cannula through four connected pressure lines (Lectro-Cath V-Green PE, Vygon, Ecouen, France) attached to a clinical-grade pressure sensor (TruWave disposable pressure transducer, Edwards Lifesciences, Irvine, CA, United States) positioned in the scanner’s control room at the same height as the kidney. The following systolic/diastolic pressure scenarios were set: 160/120, 110/70, 80/40, and 50/10 mmHg with a pause of 1 minute in between to allow the kidney to adapt to the change in tissue perfusion. Resultant renal flow rates were externally measured on the arterial line with an ultrasonic flow sensor (Transonic® HQXL Flowsensors, Ithaca, IL, United States).
Ex vivo tomoelastography
Tomoelastography was performed utilizing two pneumatic actuators which were placed under the organ chamber. The compressed air-driven actuators induced four vibration frequencies in the range of 40–70 Hz with an increment of 10 Hz and air pressure of 650–800 mbar. All examinations were performed on a 3 T MRI system (Magnetom Prisma; Siemens Healthineers, Erlangen, Germany) using a 20-channel phased-array head coil. The acquisition of the 3-D wave fields was based on the one proposed by Dittmann et al. (Dittmann et al., 2016) using a single-shot, spin-echo planar imaging sequence with flow-compensated motion-encoding gradients (MEG). Eight wave-phase offsets were recorded for each of the three motion directions. In total, 19 paracoronal slices with 2 mm isotropic resolution covering the entire ex vivo kidney were acquired in 4 minutes. Other tomoelasography parameters were: TR = 2,260 ms; TE = 69 ms; FOV of 256 × 256 mm2 (matrix size: 128 × 128) parallel imaging with a GRAPPA factor of 2; MEG frequency = 37.20 Hz, 37.48 Hz, 45.13 Hz, and 74.40 Hz for vibration frequencies of 40 Hz, 50 Hz 60 Hz, and 70 Hz; MEG amplitude = 40 mT/m.
Data processing
Tomoelastography data were post-processed using multifrequency dual elasto-visco inversion programs publicly available at https://bioqic-apps.charite.de(Meyer et al., 2022). Shear wave speed (c in m/s) was retrieved from multifrequency wave-number recovery (k-MDEV) inversion algorithm and the phase angle of the complex shear modulus, φ (range: 0–π/2) from the multifrequency direct inversion (MDEV). Shear wave speed reflects tissue stiffness as it is derived from the real part of wave numbers. Furthermore, φ is an indicator for the solid–fluid behavior of the tissue and is referred to as fluidity for fluid dominated tissue properties (φ > π/4). Pre-smoothing was performed using a Gaussian kernel for both inversion algorithms. Additionally, to suppress low-frequency compression waves, a linear highpass in k-space was employed for k-MDEV and a bandpass Butterworth filter of third order for MDEV. More details concerning the applied inversion methods are given in Streitberger et al. (Streitberger et al., 2014a) for MDEV and Tzschätzsch et al. (Tzschätzsch et al., 2016) for k-MDEV. The resulting elastograms reflecting tissue stiffness (c) as well as tissue fluidity (φ) were visualized with open-source DICOM viewer Horos® (version 3.3.6, https://horosproject.org/). Based on paracoronal anatomical T2-weighted images of the MRE scan, regions of interest (ROIs) were manually drawn in the renal cortex and three different medullary pyramids. Example ROIs are shown in Figure 2.
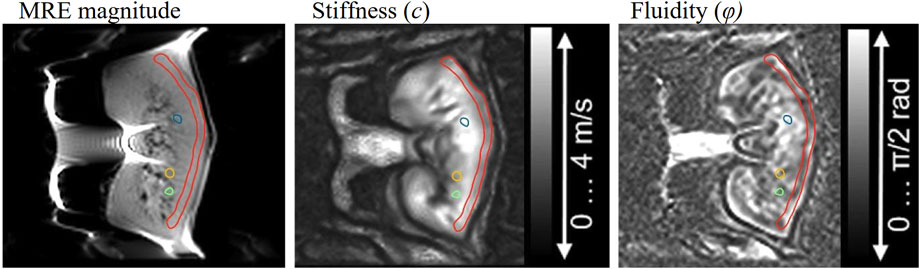
FIGURE 2. Example of cortical (red) and medullary (blue, yellow, green) segmentation for the analysis of stiffness and fluidity.
Statistical analysis
All values are expressed as means ± standard deviations. The Shapiro–Wilk test was used to check the normality assumption for quantitative variables. Pearson’s correlation coefficient was calculated for bivariate correlation to investigate the relationship between mechanical properties (stiffness and fluidity) and perfusion (blood pressure and flow). All statistical analyses and visualizations were performed using OriginPro, (Version 2022b; OriginLab Corporation, Northampton, MA, United States). Statistical significance was assumed when the p-value was <0.01.
Results
Obtained stiffness and fluidity values, as well as tracked blood flow of all ex vivo kidneys for the four adjusted blood pressure scenarios, are summarized in Table 1. Overall, stiffness and fluidity increased with blood pressure in the renal cortex and the medulla. In cortical tissue, wave speed increased by approximately 124% and fluidity by 132%, ranging from 50/10 mmHg to 160/120 mmHg. In contrast, enhancement of the medullary mechanical properties was less pronounced, with an approximate increase of 60% in stiffness and 21% in fluidity.
Figure 3 shows the visually evident effect of increasing blood pressure on the stiffness and fluidity elastograms.
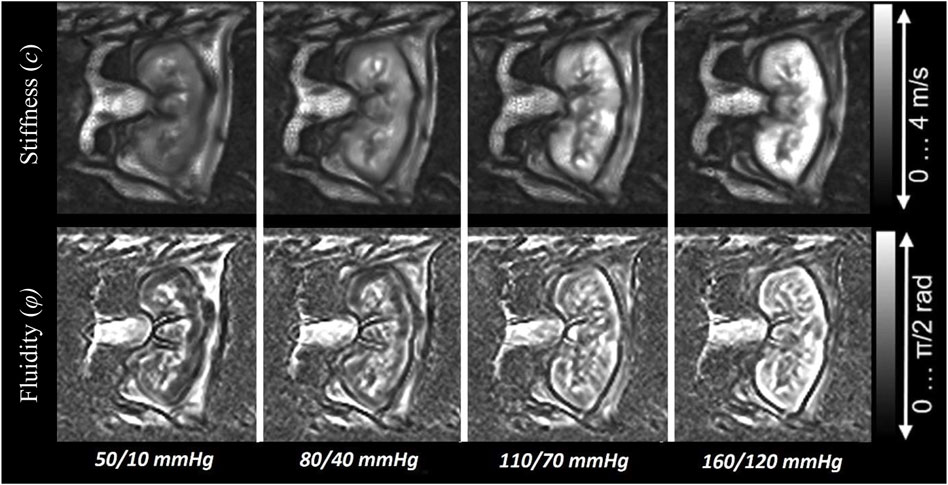
FIGURE 3. Images illustrating the increase of stiffness and fluidity in the ex vivo porcine kidney with rising blood pressure.
Pearson’s correlation was used to compare perfusion markers with observed tomoelastography findings. Figure 4 shows scatterplots and resulting Pearson correlation coefficients between systolic blood pressure/flow and mechanical parameters in the renal cortex and medulla. We found statistically significant high positive correlations between systolic blood pressure and blood flow in cortical stiffness (blood pressure: r = 0.95, p < 0.001; blood flow: r = 0.91, p < 0.001) and fluidity (blood pressure: r = 0.96, p < 0.001; blood flow: r = 0.92, p < 0.001). Likewise, we observed a statistically significant association between medullary stiffness and systolic blood pressure (r = 0.88, p < 0.001) and blood flow (r = 0.71, p < 0.001). On the other hand, there was no statistically significant correlation between medullary fluidity and systolic blood pressure (r = 0.42, p < 0.07) nor blood flow (r = 0.53, p < 0.02). Hence, our results suggest a strong linear relationship between tissue perfusion and renal stiffness as well as cortical fluidity.
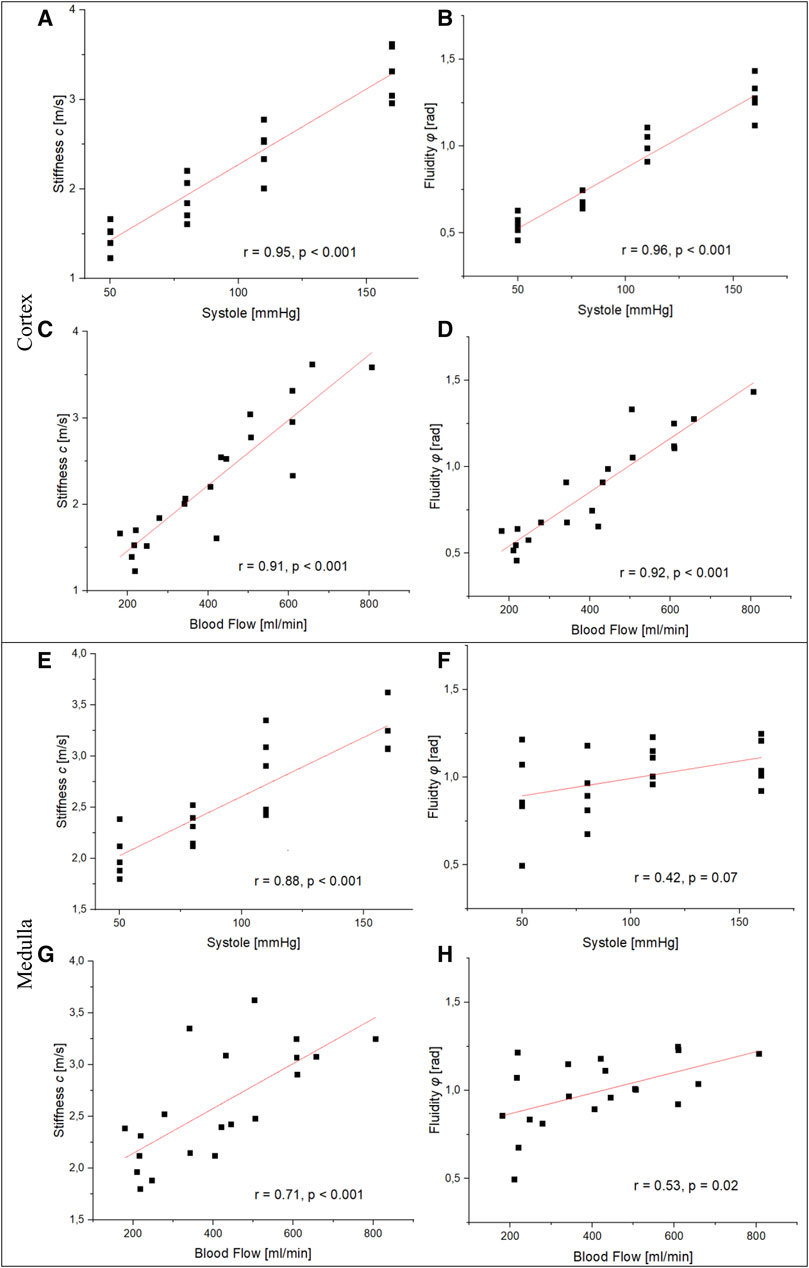
FIGURE 4. Relationship between perfusion markers and the mechanical properties of the renal cortex and medulla. (A, B), (C), and (D) show a statistically significant high positive correlation between blood pressure/flow and stiffness as well as fluidity in the renal cortex. Resemblant significant correlations in the renal medulla were observed between blood pressure/flow and stiffness (E, G). No statistically significant correlation was observed between systolic blood pressure/flow and fluidity (F), (H).
Discussion
Tomoelastography allows early detection of changes in renal stiffness as a result of various kidney pathologies. However, variations in tissue perfusion strongly impact the assessment of renal stiffness (Warner et al., 2011). In the present study, we have characterized the influence of tissue perfusion on stiffness and fluidity in an ex vivo perfused porcine kidney model. Our most remarkable finding is the strong positive correlation between increasing vascular pressure (and consequently blood flow) and stiffness in the cortex and medulla of ex vivo perfused kidneys. Another promising finding was the rapid change in renal tissue stiffness and fluidity after only 1 minute of altered tissue perfusion.
The entire kidney is surrounded by a fibrous capsule composed of tough fibers with minor flexibility. Increasing vascular pressure leads to an increase in renal cortical and medullary stiffness due to the minimal elasticity of the renal capsule. As the kidney is one of the most highly vascularized organs in the body, variations in perfusion will have a greater impact on the mechanical properties than in other organs. This effect may play a major role in MRE of in vivo kidneys to detect early structural changes, as well as to provide valid cut-off values for specific pathologies. It has been discussed that perfusion changes provide the sensitivity to renal MRE to detect glomerulonephritis in patients with preserved renal function (Grossmann et al., 2019). As such, MRE can detect subtle changes in renal tissue softening that may indicate impaired tissue perfusion and reduced fluid pressure at very early stages, making it a sensitive marker for evaluating glomerulonephritis (Marticorena Garcia et al., 2018c; Lang et al., 2019). Otherwise, a correction factor based on blood pressure or blood flow may increase the sensitivity of tomoelastography to solid matrix structures and lead to higher accuracy in inter-study comparisons. Moreover, our findings appear to be similar to the viscoelastic response of the human brain due to altered cerebral perfusion induced by the Valsalva maneuver (Herthum et al., 2021).
During acute kidney injury (AKI), the kidneys undergo functional and structural changes, including inflammation, ischemia, necrosis, and a reduction in regional renal oxygen delivery (Ferenbach and Bonventre, 2016; Hsu and Hsuyuan, 2016; Hatakeyama et al., 2018). AKI and CKD are closely linked and can promote each other (Zhu et al., 2022). In the transition from AKI to CKD, the repair mechanisms in the kidney become maladaptive (Ferenbach and Bonventre, 2016). Instead of fully repairing the damage, the kidneys undergo accelerated fibrosis, which involves excessive collagen deposition and other extracellular matrix components. This fibrotic process contributes to the stiffening of renal tissue and impairs kidney function. However, due to the significant impact of perfusion on renal stiffness, we see an overall renal softening caused by reduced perfusion as a consequence of vessel rarefication. Our results support the theoretical model of renal softening in dysfunctional kidneys due to reduced perfusion, as proposed by Lang et al. (Lang et al., 2019). Additionally, our outcomes underline the potential of flow-based correction factors to unmask renal stiffening attributable to fibrosis.
Renal blood flow is well autoregulated. The kidney distributes about 80% of its blood flow to the cortex and 15% to the outer medulla (Duke, 2011). This difference in perfusion between the cortex and medulla is confirmed by the higher cortical stiffness (124% vs. 60% in the medulla) and fluidity (132% vs. 21%) observed in this study. In addition, our results underline the high elastic modulus—indicating a high resistance to deformation—of the fibrous renal capsule. Although Garcia et al. (Marticorena Garcia et al., 2018b) have also reported increased cortical stiffness with increased perfusion, their results showed a reduction in medullary stiffness with rising venous inflow pressure. This decrease opposes the generally positive correlation between perfusion and stiffness. Nevertheless, it should be noted that their study was conducted on a single non-perfused porcine kidney, hence not under near-physiological conditions. An in vivo study by Gandhi et al. (Gandhi et al., 2020) using single-frequency MRE on healthy subjects found a significant increase in renal stiffness after water intake. These results are consistent with our findings. In addition, the fact that we could directly and reliably measure renal blood pressure corroborates the theory that a higher perfusion pressure in the kidney causes an increase in renal stiffness.
Acquired cortical stiffness (c = 2.43 ± 0.29 m/s) and medullary stiffness (c = 2.85 ± 0.40 m/s) at a blood pressure of 110/70 mmHg—which can be assumed as resting blood pressure—are in line with reported values for in vivo porcine kidneys (Warner et al., 2011) of 2.72 m/s at 120 Hz (shear modulus of 7.4 kPa) for the cortex and 2.79 m/s at 120 Hz (shear modulus of 7.8 kPa) for the medulla. Likewise, cortical stiffness is consistent with previous tomoelastography findings in native human kidneys (c = 2.52 ± 0.11 m/s Marticorena Garcia et al., 2018a, 2.46 ± 0.25 m/s Shahryari et al., 2021). However, we measured higher medullary stiffness compared to the previous study (c = 2.15 m/s ± 0.08 m/s) Marticorena Garcia et al., 2018a. In addition, tissue fluidity, expressed by the phase angle in radians, in the cortex (φ = 0.99 ± 0.09) and medulla (φ = 1.09 ± 0.11) was slightly higher than reported by Streitberger et al. (Streitberger et al., 2014b) (φCortex = 0.83 ± 0.09, φMedulla = 0.89 ± 0.12). We attribute this discrepancy to the different experimental setup, as (Streitberger et al., 2014b) performed an in vivo assessment compared to our ex vivo approach. The difference here could be attributed to the lacking innervation of ex vivo kidney, thus lacking autoregulation of renal blood flow. Especially fluidity could be more directly influenced by vascular interaction when the kidney is not autoregulated (Gandhi et al., 2020; Shahryari et al., 2021). By all means, our study contributes to unraveling factors that aid in detecting renal structural changes.
Our study has several limitations. First, the slight observer-dependency of the manual ROI segmentation that we performed may have affected the quantification of mechanical parameters. This especially holds true for the porcine medulla, which is smaller than a human kidney, making proper delineation challenging. Second, we quantified the total renal blood flow and correlated it to regional stiffness measures of the cortex and medulla. The additional assessment of regional tissue perfusion using MRI perfusion imaging techniques such as dynamic susceptibility contrast (DSC) and arterial spin labeling (ASL) would have enabled a more sophisticated approach to explore the relationship between renal perfusion and stiffness. Thirdly, ex vivo perfused kidneys are abnormally innervated, leading to lacking autoregulation of renal blood flow (Shannon et al., 1998). Hence, high arterial blood pressures are directly transferred to the graft endothelium, making the stiffness of ex vivo kidneys much more closely related to perfusion pressure than native kidneys. As demonstrated by Garcia et al. (Garcia et al., 2016), dysfunctional allografts are softer than their functional counterparts, further highlighting the sensitivity of MRE in detecting changes in renal perfusion. Finally, there were variations in the duration of O-HMP and NMP prior to the tomoelastography scan (1–5.5 h), which might have influenced intrarenal flow distribution.
In conclusion, our study emphasizes the impact of perfusion on the mechanical properties of renal tissue and highlighted the sensitivity of tomoelastography to fluid pressure. Moreover, we observed that renal stiffness and fluidity rapidly change when renal hemodynamics are altered. Therefore, future studies should focus on validating our results in an in vivo setting and determining correction factors for regional tissue perfusion in the renal cortex and medulla.
Data availability statement
The raw data supporting the conclusion of this article will be made available by the authors, without undue reservation.
Ethics statement
Ethical approval was not required for the study involving animals in accordance with the local legislation and institutional requirements because no ethical review and approval is required for the use of such slaughterhouse-derived organs, as organs are obtained after the animal has died in the context of these standard procedures for slaughtering animals for human consumption.
Author contributions
JC was involved in study design, acquisition, analysis, interpretation, and manuscript writing. JC, CP, RA, and MV was involved in acquisiton. IS performed study design and critical revision. RD was involved in critical revision. RB was involved in study design, analysis, interpretation, and critical revision. All authors contributed to the article and approved the submitted version.
Conflict of interest
The authors declare that the research was conducted in the absence of any commercial or financial relationships that could be construed as a potential conflict of interest.
Publisher’s note
All claims expressed in this article are solely those of the authors and do not necessarily represent those of their affiliated organizations, or those of the publisher, the editors and the reviewers. Any product that may be evaluated in this article, or claim that may be made by its manufacturer, is not guaranteed or endorsed by the publisher.
References
Anders, H. J., Huber, T. B., Isermann, B., and Schiffer, M. C. K. D. (2018). CKD in diabetes: diabetic kidney disease versus nondiabetic kidney disease. Nat. Rev. Nephrol. 14, 361–377. doi:10.1038/s41581-018-0001-y
Brown, R. S., Sun, M. R. M., Stillman, I. E., Russell, T. L., Rosas, S. E., and Wei, J. L. (2020). The utility of magnetic resonance imaging for noninvasive evaluation of diabetic nephropathy. Nephrol. Dial. Transpl. 35, 970–978. doi:10.1093/ndt/gfz066
Dittmann, F., Hirsch, S., Tzschätzsch, H., Guo, J., Braun, J., and Sack, I. (2016). In vivo wideband multifrequency MR elastography of the human brain and liver. Magn. Reson Med. 76, 1116–1126. doi:10.1002/mrm.26006
Dittmann, F., Tzschätzsch, H., Hirsch, S., Barnhill, E., Braun, J., Sack, I., et al. (2017). Tomoelastography of the abdomen: tissue mechanical properties of the liver, spleen, kidney, and pancreas from single MR elastography scans at different hydration states. Magn. Reson Med. 78, 976–983. doi:10.1002/mrm.26484
Duke, J. (2011). Renal function and anesthesia. Anesth. Secrets, 308–316. doi:10.1016/B978-0-323-06524-5.00045-3
Ferenbach, D. A., and Bonventre, J. V. (2016). Acute kidney injury and chronic kidney disease: from the laboratory to the clinic. Nephrol. Ther. 12, S41–S48. doi:10.1016/j.nephro.2016.02.005
Fogo, A. B. (2007). Mechanisms of progression of chronic kidney disease. Pediatr. Nephrol. 22, 2011–2022. doi:10.1007/s00467-007-0524-0
Gandhi, D., Kalra, P., Raterman, B., Mo, X., Dong, H., and Kolipaka, A. (2019). Magnetic resonance elastography of kidneys: SE-EPI MRE reproducibility and its comparison to GRE MRE. NMR Biomed. 32, e4141. doi:10.1002/nbm.4141
Gandhi, D., Kalra, P., Raterman, B., Mo, X., Dong, H., and Kolipaka, A. (2020). Magnetic resonance elastography-derived stiffness of the kidneys and its correlation with water perfusion. NMR Biomed. 33, e4237. doi:10.1002/nbm.4237
Garcia, S. R. M., Fischer, T., Dürr, M., Gültekin, E., Braun, J., Sack, I., et al. (2016). Multifrequency magnetic resonance elastography for the assessment of renal allograft function. Investig. Radiol. 51, 591–595. doi:10.1097/rli.0000000000000271
Gennisson, J. L., Grenier, N., Combe, C., and Tanter, M. (2012). Supersonic shear wave elastography of in vivo pig kidney: influence of blood pressure, urinary pressure and tissue anisotropy. Ultrasound Med. Biol. 38, 1559–1567. doi:10.1016/j.ultrasmedbio.2012.04.013
Greenberg, J. H., Kakajiwala, A., Parikh, C. R., and Furth, S. (2018). Emerging biomarkers of chronic kidney disease in children. Pediatr. Nephrol. 33, 925–933. doi:10.1007/s00467-017-3701-9
Grossmann, M., Tzschätzsch, H., Lang, S. T., Guo, J., Bruns, A., Dürr, M., et al. (2019). US time-harmonic elastography for the early detection of glomerulonephritis. Radiology 292, 676–684. doi:10.1148/radiol.2019182574
Hatakeyama, Y., Horino, T., Nagata, K., Matsumoto, T., Terada, Y., and Okuhara, Y. (2018). Transition from acute kidney injury to chronic kidney disease: a single-centre cohort study. Clin. Exp. Nephrol. 22, 1281–1293. doi:10.1007/s10157-018-1571-5
Herthum, H., Shahryari, M., Tzschätzsch, H., Schrank, F., Warmuth, C., Görner, S., et al. (2021). Real-time multifrequency MR elastography of the human brain reveals rapid changes in viscoelasticity in response to the Valsalva maneuver. Front. Bioeng. Biotechnol. 9, 1–12. doi:10.3389/fbioe.2021.666456
Hewitson, T. D. (2012). Fibrosis in the kidney: is a problem shared a problem halved? Fibrogenes. Tissue Repair 5, S14–S15. doi:10.1186/1755-1536-5-s1-s14
Hosgood, S. A., Thompson, E., Moore, T., Wilson, C. H., and Nicholson, M. L. (2018). Normothermic machine perfusion for the assessment and transplantation of declined human kidneys from donation after circulatory death donors. Br. J. Surg. 105, 388–394. doi:10.1002/bjs.10733
Hsu, R. K., and Hsuyuan, C. (2016). THE ROLE OF ACUTE KIDNEY INJURY IN CHRONIC KIDNEY DISEASE. Semin. Nephrol. 36, 283–292. doi:10.1016/j.semnephrol.2016.05.005
Hysi, E., and Yuen, D. A. (2020). Imaging of renal fibrosis. Curr. Opin. Nephrol. Hypertens. 29, 599–607. doi:10.1097/mnh.0000000000000650
Kaths, J. M., Hamar, M., Echeverri, J., Linares, I., Urbanellis, P., Cen, J. Y., et al. (2018). Normothermic ex vivo kidney perfusion for graft quality assessment prior to transplantation. Am. J. Transpl. 18, 580–589. doi:10.1111/ajt.14491
Kirpalani, A., Hashim, E., Leung, G., Kim, J. K., Krizova, A., Jothy, S., et al. (2017). Magnetic resonance elastography to assess fibrosis in kidney allografts. Clin. J. Am. Soc. Nephrol. 12, 1671–1679. doi:10.2215/cjn.01830217
Kolipaka, A., Illapani, V. S. P., Kenyhercz, W., Dowell, J. D., Go, M. R., Starr, J. E., et al. (2016). Quantification of abdominal aortic aneurysm stiffness using magnetic resonance elastography and its comparison to aneurysm diameter. J. Vasc. Surg. 64, 966–974. doi:10.1016/j.jvs.2016.03.426
Lang, S. T., Guo, J., Bruns, A., Dürr, M., Braun, J., Hamm, B., et al. (2019). Multiparametric quantitative MRI for the detection of IgA nephropathy using tomoelastography, DWI, and BOLD imaging. Investig. Radiol. 54, 669–674. doi:10.1097/rli.0000000000000585
Liu, Y. (2006). Renal fibrosis: new insights into the pathogenesis and therapeutics. Kidney Int. 69, 213–217. doi:10.1038/sj.ki.5000054
Lopez-Giacoman, S. (2015). Biomarkers in chronic kidney disease, from kidney function to kidney damage. World J. Nephrol. 4, 57. doi:10.5527/wjn.v4.i1.57
Mansour, S. G., Puthumana, J., Coca, S. G., Gentry, M., and Parikh, C. R. (2017). Biomarkers for the detection of renal fibrosis and prediction of renal outcomes: a systematic review. BMC Nephrol. 18, 72. doi:10.1186/s12882-017-0490-0
Marticorena Garcia, S. R., Grossmann, M., Bruns, A., Dürr, M., Tzschätzsch, H., Hamm, B., et al. (2019). Tomoelastography paired with T2∗ magnetic resonance imaging detects lupus nephritis with normal renal function. Investig. Radiol. 54, 89–97. doi:10.1097/rli.0000000000000511
Marticorena Garcia, S. R., Grossmann, M., Lang, S. T., Tzschätzsch, H., Dittmann, F., Hamm, B., et al. (2018a). Tomoelastography of the native kidney: regional variation and physiological effects on in vivo renal stiffness. Magn. Reson Med. 79, 2126–2134. doi:10.1002/mrm.26892
Marticorena Garcia, S. R., Grossmann, M., Lang, S. T., Tzschätzsch, H., Dittmann, F., Hamm, B., et al. (2018b). Tomoelastography of the native kidney: regional variation and physiological effects on in vivo renal stiffness. Magn. Reson Med. 79, 2126–2134. doi:10.1002/mrm.26892
Marticorena Garcia, S. R., Guo, J., Dürr, M., Denecke, T., Hamm, B., Sack, I., et al. (2018c). Comparison of ultrasound shear wave elastography with magnetic resonance elastography and renal microvascular flow in the assessment of chronic renal allograft dysfunction. Acta Radiol. 59, 1139–1145. doi:10.1177/0284185117748488
Marticorena Garcia, S. R., Althoff, C. E., Dürr, M., Halleck, F., Budde, K., Grittner, U., et al. (2021). Tomoelastography for longitudinal monitoring of viscoelasticity changes in the liver and in renal allografts after direct-acting antiviral treatment in 15 kidney transplant recipients with chronic hcv infection. J. Clin. Med. 10, 1–15. doi:10.3390/jcm10030510
Meyer, T, Marticorena Garcia, S., Tzschätzsch, H., Herthum, H., Shahryari, M., Stencel, L., et al. (2022). Comparison of inversion methods in MR elastography: an open-access pipeline for processing multifrequency shear-wave data and demonstration in a phantom, human kidneys, and brain. Magn Reson Med 88(4), 1840-1850. doi:10.1002/mrm.29320
Rigalli, J. P., Barros, E. R., Sommers, V., Bindels, R. J. M., and Hoenderop, J. G. J. (2020). Novel aspects of extracellular vesicles in the regulation of renal physiological and pathophysiological processes. Front. Cell. Dev. Biol. 8, 244. doi:10.3389/fcell.2020.00244
Shahryari, M., Meyer, T., Warmuth, C., Herthum, H., Bertalan, G., Tzschätzsch, H., et al. (2021). Reduction of breathing artifacts in multifrequency magnetic resonance elastography of the abdomen. Magn. Reson Med. 85, 1962–1973. doi:10.1002/mrm.28558
Shannon, J. L., Headland, R., MacIver, A. G., Ferryman, S. R., Barber, P. C., and Howie, A. J. (1998). Studies on the innervation of human renal allografts. J. Pathol. 186, 109–115. doi:10.1002/(sici)1096-9896(199809)186:1<109::aid-path134>3.0.co;2-n
Streitberger, K. J., Guo, J., Tzschätzsch, H., Hirsch, S., Fischer, T., Braun, J., et al. (2014b). High-resolution mechanical imaging of the kidney. J. Biomech. 47, 639–644. doi:10.1016/j.jbiomech.2013.11.051
Streitberger, K. J., Reiss-Zimmermann, M., Freimann, F. B., Bayerl, S., Guo, J., Arlt, F., et al. (2014a). High-resolution mechanical imaging of glioblastoma by multifrequency magnetic resonance elastography. PLoS One 9, e110588. doi:10.1371/journal.pone.0110588
Tzschätzsch, H., Guo, J., Dittmann, F., Hirsch, S., Barnhill, E., Jöhrens, K., et al. (2016). Tomoelastography by multifrequency wave number recovery from time-harmonic propagating shear waves. Med. Image Anal. 30, 1–10. doi:10.1016/j.media.2016.01.001
Venkatesh, S. K., and Ehman, R. L. (2015). Magnetic resonance elastography of abdomen. Abdom. Imaging 40, 745–759. doi:10.1007/s00261-014-0315-6
Warner, L., Yin, M., Glaser, K. J., Woollard, J. A., Carrascal, C. A., Korsmo, M. J., et al. (2011). Noninvasive in vivo assessment of renal tissue elasticity during graded renal ischemia using MR elastography. Investig. Radiol. 46, 509–514. doi:10.1097/rli.0b013e3182183a95
Williams, W. W., Taheri, D., Tolkoff-Rubin, N., and Colvin, R. B. (2012). Clinical role of the renal transplant biopsy. Nat. Rev. Nephrol. 8, 110–121. doi:10.1038/nrneph.2011.213
Woud, W. W., Merino, A., Hoogduijn, M. J., Boer, K., van den Hoogen, M. W. F., Baan, C. C., et al. (2019). Nanoparticle release by extended criteria donor kidneys during normothermic machine perfusion. Transplantation 103, e110–e111. doi:10.1097/tp.0000000000002642
Wynn, T. A., and Ramalingam, T. R. (2012). Mechanisms of fibrosis: therapeutic translation for fibrotic disease. Nat. Med. 18, 1028–1040. doi:10.1038/nm.2807
Yin, M., Glaser, K. J., Manduca, A., Mounajjed, T., Malhi, H., Simonetto, D. A., et al. (2017). Distinguishing between hepatic inflammation and fibrosis with MR elastography. Radiology 284, 694–705. doi:10.1148/radiol.2017160622
Keywords: magnetic resonance elastography, tomoelastography, stiffness, fluidity, ex vivo kidney, tissue perfusion
Citation: Castelein J, Pamplona C, Armstrong Junior R, Vidal dos Santos M, Sack I, Dierckx R, Moers C and Borra R (2023) Effects of kidney perfusion on renal stiffness and tissue fluidity measured with tomoelastography in an MRI-compatible ex vivo model. Front. Bioeng. Biotechnol. 11:1236949. doi: 10.3389/fbioe.2023.1236949
Received: 08 June 2023; Accepted: 27 October 2023;
Published: 09 November 2023.
Edited by:
Gabriele Ciasca, Catholic University of the Sacred Heart, ItalyReviewed by:
Peter R. Corridon, Khalifa University, United Arab EmiratesYuan Feng, Shanghai Jiao Tong University, China
Riccardo Di Santo, Catholic University of the Sacred Heart, Rome, Italy
Copyright © 2023 Castelein, Pamplona, Armstrong Junior, Vidal dos Santos, Sack, Dierckx, Moers and Borra. This is an open-access article distributed under the terms of the Creative Commons Attribution License (CC BY). The use, distribution or reproduction in other forums is permitted, provided the original author(s) and the copyright owner(s) are credited and that the original publication in this journal is cited, in accordance with accepted academic practice. No use, distribution or reproduction is permitted which does not comply with these terms.
*Correspondence: Johannes Castelein, ai5jYXN0ZWxlaW5AdW1jZy5ubA==