- 1Department of Orthopedics, First Affiliated Hospital of Dalian Medical University, Dalian, China
- 2Key Laboratory of Molecular Mechanism for Repair and Remodeling of Orthopedic Diseases, Dalian, Liaoning, China
- 3Division of Energy Materials (DNL22), Dalian Institute of Chemical Physics, Chinese Academy of Sciences, Dalian, China
The application of three-dimensional printing technology in the medical field has great potential for bone defect repair, especially personalized and biological repair. As a green manufacturing process that does not involve liquefication through heating, low-temperature deposition manufacturing (LDM) is a promising type of rapid prototyping manufacturing and has been widely used to fabricate scaffolds in bone tissue engineering. The scaffolds fabricated by LDM have a multi-scale controllable pore structure and interconnected micropores, which are beneficial for the repair of bone defects. At the same time, different types of cells or bioactive factor can be integrated into three-dimensional structural scaffolds through LDM. Herein, we introduced LDM technology and summarize its applications in bone tissue engineering. We divide the scaffolds into four categories according to the skeleton materials and discuss the performance and limitations of the scaffolds. The ideas presented in this review have prospects in the development and application of LDM scaffolds.
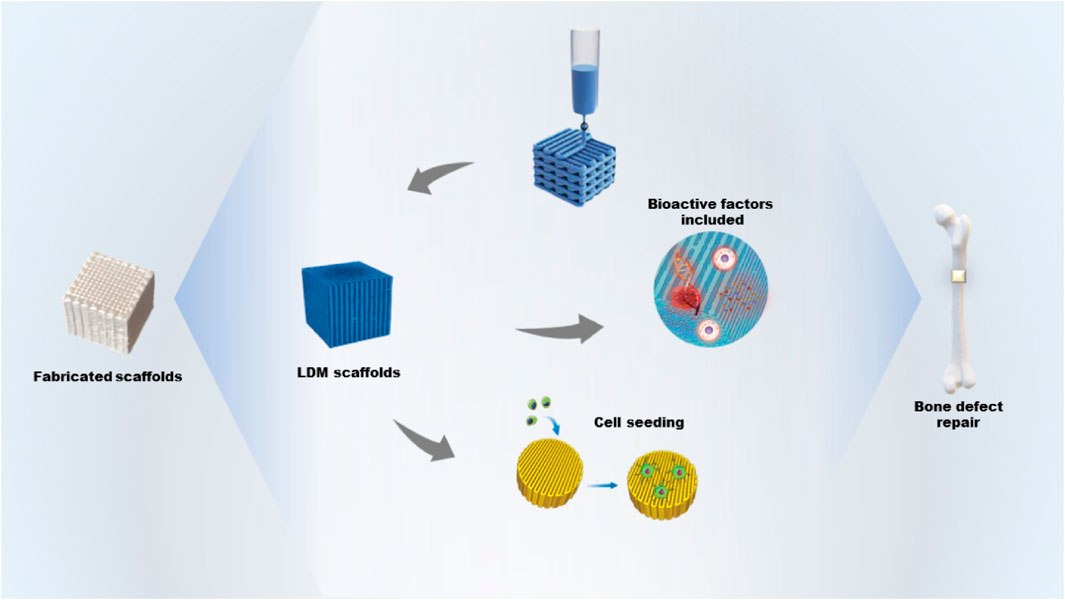
GRAPHICAL ABSTRACT | The fabrication of bone tissue engineering scaffolds including bioactive factors by low-temperature deposition manufacturing and its application for bone defect repair.
1 Introduction
Bone defects, mostly those concerning a young and athletic population, are increasingly receiving attention (Sfeir et al., 2022). In general, bone tissue has a natural capacity to regenerate, which helps the repair of minor injuries. However, large bone defects due to pathological fractures or high-energy injuries present a clinical challenge requiring bone grafting to overcome. Bone tissue engineering, combined scaffolds, seed cells, and cytokines play important roles in bone repair. The ideal bone tissue engineering scaffold should have a suitable surface for cell attachment, a porous structure for vascularization, and a suitable mechanical support (Roseti et al., 2017). Various technologies for fabricating scaffolds with controlled structure and pore size, including rapid prototyping manufacturing (RPM), gas foaming, and electrospinning, have been reported. Representative fabrication methods and materials of bone tissue engineering are given in Table 1. As an innovative material processing approach, RPM scaffolds have been used in preclinical studies (Dadhich et al., 2016; Yang et al., 2017; Dadhich et al., 2021). The materials of RPM can be liquid or solid, and the printing processes using wet materials include inkjet printing, stereolithography, and direct ink writing. With the help of computer-aided design (CAD) tools, RPM offers the possibility of fabricating complex structures through the layer-by layer deposition of inks of various materials (Jones, 2012; Do et al., 2015; Wubneh et al., 2018). Undoubtedly, RPM is important to the future of bone regeneration for its ability to control the geometry and internal porous structures of scaffolds. In 1999, Leu et al. reported rapid freezing prototyping in milestone work (Zhang et al., 1999; Leu et al., 2000). During the fabricating process, water is deposited from a nozzle in a cryogenic atmosphere and rapidly frozen layer by layer. As an extension, RPM is often called low-temperature deposition manufacturing (LDM) when the injection devices extrude materials used for tissue engineering.
LDM was firstly reported in 2002 for the fabrication of bone tissue engineering scaffolds, which is based on traditional fabrication technologies such as direct ink writing (Xiong et al., 2002). The material used in LDM is usually a viscous polymer and the extruder is usually of piston or pneumatic type operating at room temperature (Geven and Grijpma, 2019). In addition, scaffolds can be fabricated on a cold platform or in a freezer layer-by-layer with the solvent then removed by freeze drying (Papastavrou et al., 2018; Qin et al., 2021). Compared with conventional technologies, LDM is combined with a phase separation process and the scaffolds have a hierarchically porous structure from microns to nanometers that is beneficial to cell adhesion and tissue growth (Liu et al., 2017). However, the mechanical properties of the LDM scaffolds are usually slightly weaker than those of traditional scaffolds. In addition, LDM is a kind of green manufacturing because it does not require heating during fabricating (Zhang et al., 2008). LDM scaffolds thus maintain the bioactivities of natural biopolymers, such as gelatin, chitosan and sodium alginate and are often used for bioprinting or printing tissue (Wang et al., 2012; Zafeiris et al., 2021; Zhao et al., 2022). As LDM is a molding method based on material extrusion, it has requirements for the viscosity of the extruded inks. The ability to control the pore size by selecting the ratio of solvent or precursor solution within the specific viscosity range of each material is an obvious advantage of LDM. Inorganic particles such as nano-hydroxyapatite and tricalcium phosphate (TCP) have been widely used for the backbone structure of scaffolds (Wang et al., 2014; He et al., 2016; Cheng et al., 2021). Moreover, synthetic biopolymers, such as poly- (lactic-co-glycolic acid) (PLGA), poly-(L-lactic acid) (PLLA), and polyurethane (PU), have been commonly used in the fabrication of porous bone tissue engineering scaffolds (Wang et al., 2013; Gentile et al., 2014; Zhang et al., 2022; Zhao et al., 2022). Ideal scaffolds can be fabricated by adjusting the properties of the material, the proportions of composite materials or the printing parameters. LDM has been adopted for bone tissue engineering scaffolds for two decades. Herein, we discuss LDM technology and review its applications in the field of bone tissue engineering.
2 Technical process of LDM
2.1 Material preparation and optimization
Typically, the LDM material is fully dissolved in an organic solvent, such as 1,4-dioxane, and the ink is mixed evenly adopting an emulsion stabilizer and ultrasonic technology. Then, the ideal material properties are obtained by adjusting the appropriate mass ratio of the different materials and the mixture is stirred well to form a uniform liquid paste (Lai et al., 2018). The concentration of a material in the solvent affects microstructure of scaffolds (Liu et al., 2007). When the polymeric materials are dissolved at higher concentrations in the solvent, the scaffolds have a smaller pore size and thicker walls of micropores. In contrast, scaffolds with lower concentrations of polymeric materials have larger pores and thinner walls of micropores. Hu et al. (2015) fabricated a pure PLGA scaffold, a PLGA/pearl scaffold with a weight ratio of 5:2, and a PLGA/TCP scaffold with a weight ratio of 5:2 and found that the proportions of the composite materials affected the structure of scaffolds in terms of the continuity of pores. Guo et al. (2017) used PLGA with various lactic acid: glycolic acid molecular weight ratios to demonstrate the dependence of the extrusion process on the polymer composition. They built a statistical model to reveal the correlation and predominant factors that determine printing precision.
Synthetic materials have better mechanical properties whereas natural materials have better biocompatibility in scaffolds (Rahmanian-Schwarz et al., 2014). Unfortunately, no solvent dissolves synthetic and natural materials together in LDM. Gelatin or GelMA hydrogel has a thermo-reversible sol-gel property, having a liquid state at 37°C and a gel state at a temperature lower than 20°C (Luo et al., 2020). They can help combine drugs, essential elements, and other bioactive factors with the scaffold material. GelMA also has a photocrosslinking ability that stabilizes the structures after printing. The bioink then enters a sol-gel state quickly and is transferred to a syringe for subsequent three-dimensional (3D) hybrid printing at low temperature.
2.2 Printing process and improvements
Usually, the structure and pore size of scaffolds determine the cell growth and regeneration of bone tissue. Material properties can be optimized by adjusting the parameters of the LDM system to print more ideal bone tissue engineering scaffolds. The parameters of the LDM system include the proportion of the composite material, the design of the model, the concentration of the material and the working parameters of the devices (Liu et al., 2007; Hu et al., 2015; Bružauskaitė et al., 2016; Wang et al., 2017b; Guo et al., 2017). According to the selection and combination of ink materials, the printer is designed to have a single nozzle or multiple nozzles, and is used with the corresponding syringe (Liu et al., 2009a; Liu et al., 2009b). In the process of LDM, the shape and architecture of scaffolds are controlled through freeform fabrication and the features of microstructure, such as porosity and surface roughness, are realized manufactured through freezing drying (Koski et al., 2018). The dispensing system deposits printing inks in a low-temperature environment, which is a continuous extrusion process in contrast with inkjet processes. The ink-containing syringe extrudes the ink through a micro-nozzle. Compared with other traditional techniques, LDM based on extrusion has an appropriate deposition and printing speed during printing, which facilitates rapid scalability (Johnson and Jia, 2016; Lee et al., 2020). Figure 1 depicts the LDM process of bone tissue engineering scaffolds and the application for bone defect repair. The advantage of this method is the variety of options of printing inks. In bone tissue engineering, various materials containing biologics have been successfully applied for the fabrication of tissue engineering scaffolds (Mehesz et al., 2011; Kronemberger et al., 2022). During printing, the deposited ink is cured to strengthen each layer. As the paste freezes, its particles are expelled from the solidification (freezing) front (Papastavrou et al., 2018). The frozen part is freeze-dried to sublimate the freezing medium and leave a highly porous scaffold.
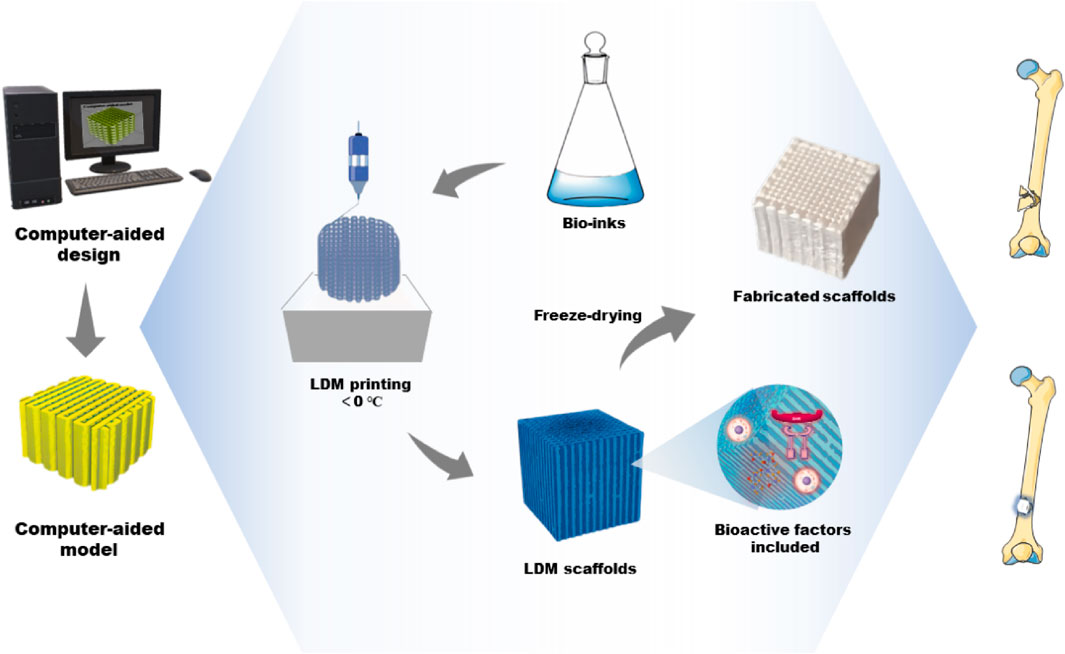
FIGURE 1. The fabrication process of bone tissue engineering scaffolds by LDM and its application for bone defect repair. LDM, low-temperature deposition manufacturing.
The shape and architecture of scaffolds depend on the models designed using computer software (Bružauskaitė et al., 2016). CAD tools offer the possibility to fabricate complex structures and custom scaffolds. In addition, the working parameters of LDM printers need to be adjusted for the fabrication of ideal scaffolds. As an example, the forming platform usually remains below a temperature of 0°C and the temperature of the nozzles must be much higher than that of the platform. Only in this way can the extruded lines be integrated with the previous layer. The extrusion speed and nozzle diameter determine the structure and size of scaffolds (Wang et al., 2017b). In the process of printing, it is necessary to control the appropriate temperature of the platform and nozzle according to the material properties and surrounding environment. At the same time, the temperature, pressure, speed, and other parameters need to be constantly adjusted to ensure the smooth printing of the scaffolds.
3 LDM materials for bone tissue engineering scaffolds
3.1 LDM scaffolds of bio-ceramic materials
Hydroxyapatite (HA) and other related Ca/P-based bio-ceramics have been used in the manufacturing of bone scaffolds for their varying osteoconductive and osteoinductive properties. They not only exist naturally in bone tissue but also have high mechanical strength and biodegradability. Moreover, the biodegradation rates can be optimized by adjusting the molar ratio of Ca and P in the optimized compound. In addition, the inorganic materials can be categorized into silicate-based glasses, borate-based glasses and phosphate-based glasses according to the components of the bioactive glass (Simorgh et al., 2022). The majority of bio-ceramic materials are subjected to a sintering procedure and high temperature treatment to prepare the scaffolds and achieve sufficient mechanical qualities (Baino and Fiume, 2020). As an example, hydrogel, containing active elements is often mixed physically with the scaffold to provide the ceramic material bioactivity (Simorgh et al., 2022). In addition, several studies have used bio-ceramic materials to formulate inks for the preparation of bone tissue engineering scaffolds through LDM. Table 2 gives bone tissue engineering scaffolds based on bio-ceramic materials. We divide the scaffolds with bio-ceramic skeletons into bio-ceramic scaffolds, bio-ceramic scaffolds with natural polymers, and bio-ceramic scaffolds with bioactive factors.
3.1.1 Bio-ceramic scaffolds
Klammert et al. (2010) used binder solution and powder (Mg3(PO4)2) to print scaffolds at room temperature and dried the scaffolds for 24 h. The structures were designed on the basis of stereolithography data and modified with 20% DAHP powder to improve the conversion rate and mechanical reinforcement (Figure 2A). However, they did not conduct in vivo investigations to explore other biological features. Castilho et al. used TCP powder and phosphoric acid solution as a binder to print dense cylindrical and porous scaffolds at room temperature (Castilho et al., 2013). They optimized the computational topology design to better fabricate the scaffolds through LDM and also considered the mechanical properties and permeability. Bolaños et al. printed tailor-made ceramic scaffolds with α/β-TCP and phosphoric acid binder at low temperature (Bolaños et al., 2020). An in vivo experiment using a large animal model showed that the material was degraded and replaced by bone, and the scaffolds had an excellent ability to promote bone formation. At the same time, in vitro experiments showed that porous implants had good mechanical properties.
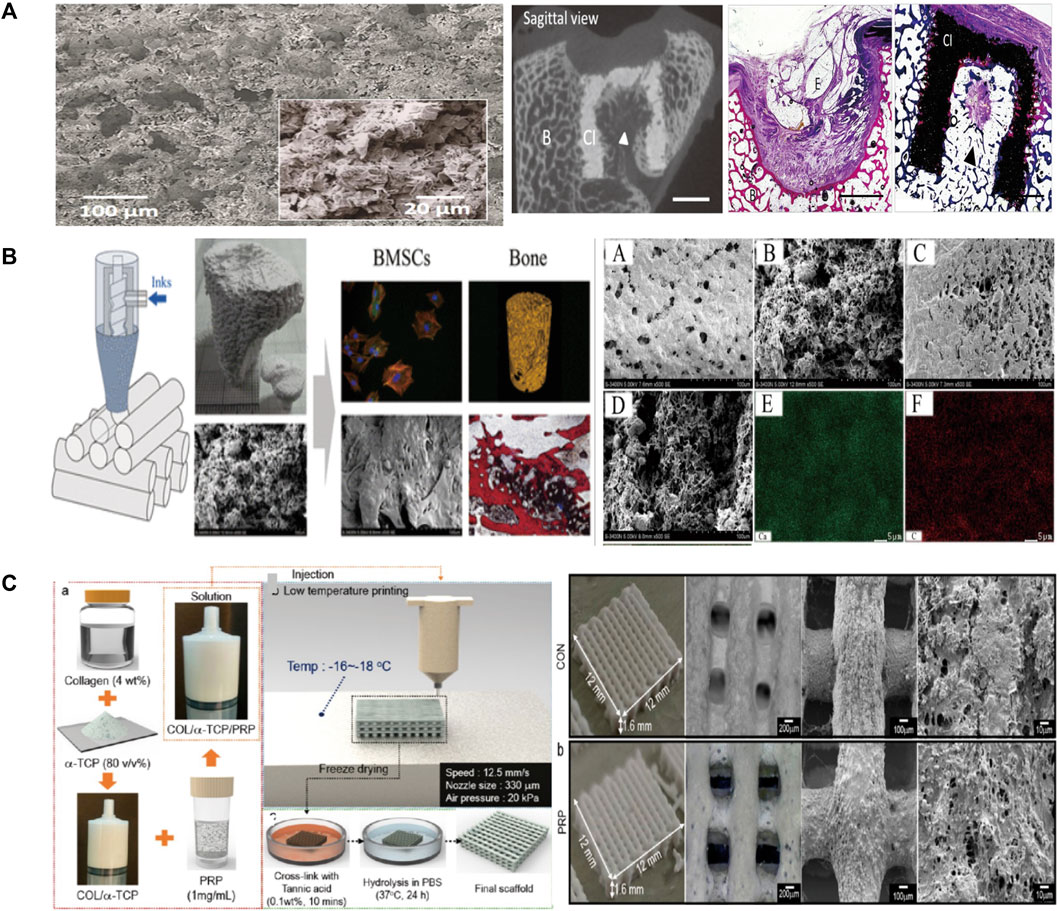
FIGURE 2. (A) Microscopic morphology and in vivo evaluation of the scaffolds. (B) The robocasting fabrication process and SEM images of the surface morphology. Micro-CT 3D reconstruction images and histological analysis of new bone formation around and within the scaffolds. (C) Schematic of the preparation of scaffolds, as well as the macro/micro morphology shown by optical and SEM images. SEM, Scanning electron microscope.
3.1.2 Bio-ceramic scaffolds with natural polymers
The brittleness of scaffolds is a main shortcoming of ceramic material. Bio-additives, such as collagen and silk fibroin (SF), are naturally degradable and can be used to optimize the ceramic scaffolds. HA and collagen are often added to the precursor solution to improve the mechanical and clinical properties of the scaffolds (Lin et al., 2016; Salgado et al., 2016). The scaffolds prepared at 4°C by Lin et al. (2016). had an excellent 3D structure and enhanced the osteogenic outcome in rabbit femoral condyle defect models (Figure 2B) (Lin et al., 2016). Huh et al. (2018) prepared bio-ink by mixing gelatin, TCP, and SF loaded the solution into a barrel with the temperature controlled from 25°C to 45°C and printed on the low temperature stage of 10°C (Huh et al., 2018). The scaffold had enhanced mechanical strength and high cellar activity. Liu et al. (2021b) used HA, SF, and gelatin to prepare bio-inks and fabricated scaffolds adopting crosslinking and freeze-drying technologies. The scaffolds were cross-linked under absolute alcohol at room temperature for 24 h. They found that the scaffolds enhanced the compressive modulus in two groups and were conducive to cell proliferation and osteogenesis. Zafeiris et al. (2021) fabricated hydrogels by HA and chitosan and then adopting chemical crosslinking in a natural crosslinking agent to improve mechanical properties. The syringe was prepared with a nozzle tip diameter of 0.41 mm and a printing speed of 0.8–1.5 mm/s to improve the printing accuracy. The 3D printing process was applied at a low temperature of 25°C and the scaffolds were freeze dried to obtain a porous structure by removing the solvent. They obtained mechanical properties close to those of cancellous bone and good cell compatibility. Inzana et al. (2014) increased the phosphoric-acid-based binder solution concentration and dissolved collagen into the binder to maximize mechanical strength and cytocompatibility. This method enhanced the mechanical strength of materials without reducing biocompatibility. They also implanted calcium-phosphate-based scaffolds into murine femoral defect to assess the bone healing performance and osteoconductive.
3.1.3 Bio-ceramic scaffolds with bioactive factors
Another disadvantage of ceramic material is the low retention level of growth factors and drugs. Therefore, bioactive substances such as live cells and growth factors are integrated in bioinks to increase the biological activity and osteogenic ability of bone repair materials. It is not a difficult task to impregnate biological factors into the mixed bioink and the low temperature avoid the risk of thermal degradation of drugs or growth factors. To overcome the shortcomings, Lee et al. designed a new composite with stable structure using CDHA, collagen, and plate-rich plasma (PRP) to enable better growth and differentiation of cells (Lee and Kim, 2018a). They used a nozzle size of 330 μm to print scaffolds at a low temperature of −16°C. Figure 2C (Lee and Kim, 2018a) is a schematic of the preparation of scaffolds and presents the macro/micro-morphology in optical and scanning electron microscopy images. The PRP had several growth factors, and a polyphenol tannic acid was used to control the release of PRP. The result in vitro showed that PRP composited scaffolds have better bone mineralization and cell proliferation, but the efficacy for bone regeneration in vivo remains controversial. Ahlfeld et al. (2018) combined the 3D plotting of calcium phosphate and an alginate-methylcellulose cell-laden blend as model bioink . They printed scaffolds were printed at room temperature using a needle with an inner diameter of 410 µm and a layer thickness of 250 µm. They proposed an osteochondral tissue graft model and evaluated the mechanical properties of the scaffolds. They found that bone morphogenetic protein-2 (BMP-2) promotes osteogenesis and vascular endothelial growth factor promotes angiogenesis. Chen et al. (2020) prepared composite scaffolds loaded with BMP-2 and vascular endothelial growth factor through 3D printing layer by layer at a temperature below 40°C. The nozzle of the printer had a diameter of 0.42 mm and the distance between strands was set at 600 μm to ensure good printing accuracy. They found that the scaffolds had good porosity and osteogenic and angiogenic properties through microcomputed tomography and immunochemical staining. However, the interaction of chitosan with gelatin achieved a compressive strength of only 6 MPa, which is below many clinical requirements. Similarly, Raina et al. formulated a precursor solution by mixing rhBMP-2 and bisphosphonates such as zoledronic acid, which can be used to control the resorption process (Raina et al., 2016). The scaffold loaded with both rhBMP-2 and zoledronic acid showed high bone formation.
3.2 LDM scaffolds of synthetic polymeric materials
Compared with ceramics, polymeric materials have more versatile physicochemical properties that suit clinical applications. Synthetic polymers usually have good processability and mechanical properties and are thus used widely in preparing materials for bone tissue engineering technology (Lutolf and Hubbell, 2005; Rezwan et al., 2006; Amiryaghoubi et al., 2020). Table 3 gives bone tissue engineering scaffolds based on polymeric materials. According to the compositions and functions, we divided the scaffolds with synthetic polymeric skeletons into three categories, namely, synthetic polymeric scaffolds with bioactive factors, synthetic polymeric scaffolds with hydrogels and synthetic polymeric scaffolds with bioactive factors/hydrogels or modified polymers.
3.2.1 Synthetic polymeric scaffolds with bioactive factors
It is often necessary to composite bioactive factors or modify the materials to increase biocompatibility or osteogenic capacity. PLGA is an excellent biomaterial for bone scaffolds with biodegradability and biocompatibility and has good matrix for pearl powder, which can provide bioactivity for PLGA (Zhang et al., 2009). Xu et al. (2010) used LDM technology to combine the advantages of pearl and PLGA and fabricated scaffolds on a platform at a temperature of −40°C. The scaffolds had a porous structure and good biocompatibility, and gene expression and alkaline phosphatase (ALP) activity tests showed osteo-inductive bioactivity more extensive than that of TCP/PLGA scaffolds. Wang et al. (2018) synthesized shape memory PU to fabricate scaffolds adopting LDM technology. The bioink included superparamagnetic iron oxide nanoparticles to promote osteogenic induction and polyethylene oxide or gelatin to improve printing. In their study, the platform was set at −30°C for PU/polyethylene oxide ink and 5°C for PU/gelatin ink. Dimethyl sulfoxide (DMSO) is a solvent that dissolves both polar and nonpolar compounds (Galvao et al., 2014). Guo et al. (2018) incorporated growth factors such as BMP-2 and TGF-β (transforming growth factor-β) into PLGA scaffold using DMSO. They obtained stretchability greater than that of pure PLGA scaffolds. However, DMSO is cytotoxic and needs to be removed completely through evaporation during the preparation. Zhang et al. (2019) adopted LDM technology to fabricate a PLGA scaffold and compounded with decellularized acellular cartilage extracellular matrix through physical–chemical cross-linking. The scaffolds were printed at −20°C and had good biological and mechanical characteristics, but were deficient in the function of recruiting endogenous stem cells. Chen et al. printed scaffolds with acellular cartilage extracellular matrix and waterborne polyurethane (PU) on a platform at temperatures ranging from −25 to −30°C (Chen et al., 2021). Figure 3A (Chen et al., 2021) presents a schematic of adipose-derived stem cell seeding and hematoxylin and eosin staining images of repaired cartilage at 3 and 6 months after operation. The figure shows that the porosity, hydrophilicity, and bioactive components of the scaffolds are improved by adding the extracellular matrix. In view of the photothermal effect and biological activity of magnesium, researchers composited PLGA and shape memory polyurethane (PU) with Mg respectively (Long et al., 2021; Zhang et al., 2022). They constructed the scaffolds adopting LDM technology and verified the good osteogenic performance of the scaffolds. As the thermal-responsive matrix, shape memory PU significantly improved the mechanical properties of the scaffolds and made closer contact with bone tissue.
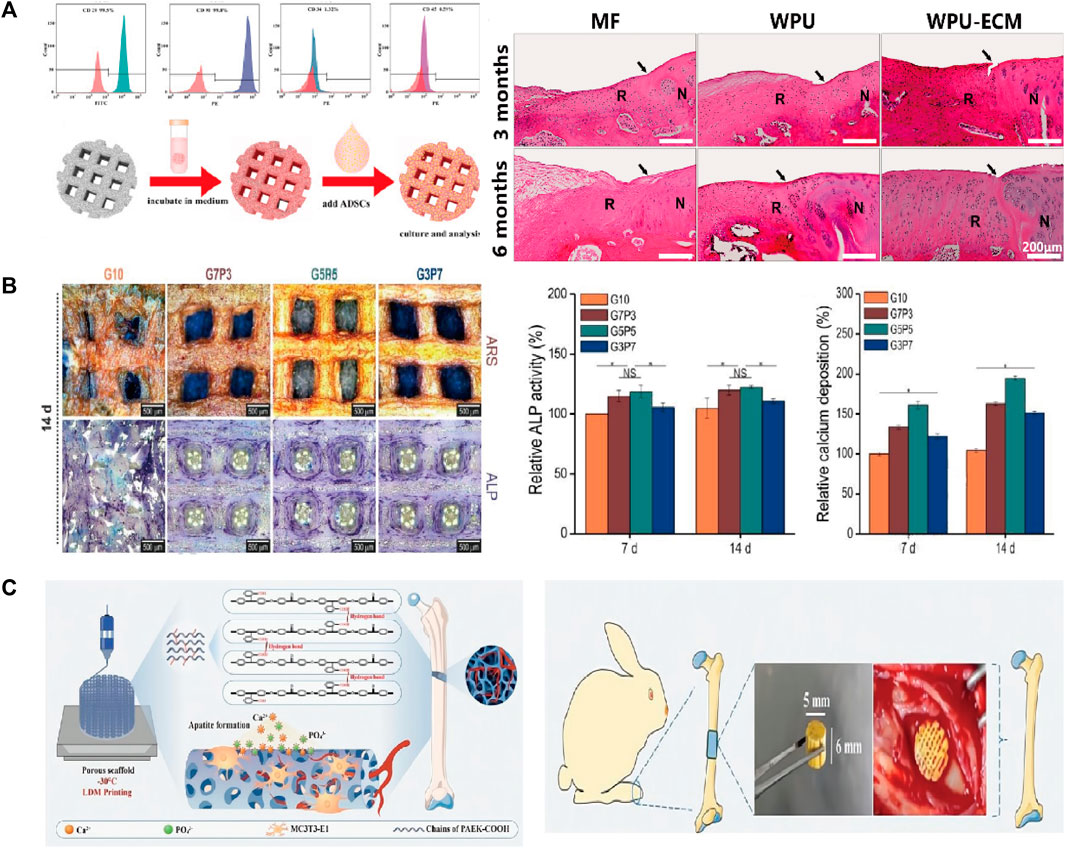
FIGURE 3. (A) Flow cytometric analysis and the schematic diagram of ADSCs seeding. The H&E staining images of the repaired cartilage at 3 and 6 months after cartilage defect creation and scaffold implantation. (B) The optical images of ARS and ALP staining of the scaffolds. The quantitative results of ALP activity and calcium deposition. (C) The hierarchically porous scaffold of PAEK-COOH favoring cellular adhesion and HA mineralization. The scaffold implanted in rabbit femur defects model induced bone formation. ADSCs, Adipose-derived stem cells; ARS, alizarin red S; ALP, alkaline phosphatase; PAEK-COOH, polyaryletherketone with carboxyl groups.
3.2.2 Synthetic polymeric scaffolds with hydrogels
Hydrogels have strong hydrophilicity, that provides an environment suitable for cell proliferation and differentiation. Therefore, the biological activity and mechanical properties of the scaffold can be improved by compositing hydrogel and polymer. For example, a novel composited PLGA-gelatin/chondroitin/hyaluronate scaffold was fabricated to keep the differentiation of mesenchymal stem cells (MSCs) and improved the regeneration of cartilage (Fan et al., 2006). Kim et al. (2016) proposed a 3D printing method with a low temperature working plate to fabricate scaffolds. They directly printed alginate and collagen layer by layer on a low-temperature (−20°C) cooling plate using a 250-µm printing nozzle and poly (ε-caprolactone) (PCL) as the coating agent. They found that the tensile modulus and osteogenic capacity of the scaffolds were better than those of the pure-hydrogel scaffolds. However, the strong cooling effect of the low-temperature working plate limited the height of the manufactured porous scaffolds, which introduced defects in this LDM process. Similarly, another study reported that adding electrospun PCL fibers into a gelatin hydrogel solution increased the Young’s modulus of the resulting construct (Kai et al., 2012). Another team fabricated scaffolds with gelatin and poly (vinyl alcohol) adopting LDM process (Kim et al., 2018). They explored the best mixture ratio to get the optimal mechanical and biological properties for a working stage temperature ranging from −5 to −40°C. The quantitative results of ALP activity and calcium deposition and optical images of ALP staining of the scaffolds are shown in Figure 3B (Kim et al., 2018). Lee and Kim (2018b) developed scaffolds having a multilayered nanofibrous structure and composited with collagen and pluronic F-127. Geng et al. (2021) fabricated a biodegradable poly (glycerol-co-sebacic acid-co-L-lactic acid-co-polyethylene glycol) scaffold and filled it with gelatin nanofibers. The scaffold promoted bone repair by locally releasing deferoxamine, which is essential for angiogenesis and osteogenesis.
3.2.3 Synthetic polymeric scaffolds with bioactive factors/hydrogels or modified polymers
Zhao et al. dispersed PLLA containing ibuprofen into sodium alginate aqueous solution to prepare the bioink (Zhao et al., 2022). The scaffolds were fabricated at a temperature of −20°C using a 0.41 mm printing nozzle. The plotting pressure was 130 kPa and the extrusion speed was fixed at 3 mm/s. The scaffolds had enhanced mechanical stability, excellent osteogenic activity, and anti-inflammatory activity. In contrast with work that composited bioactive factors and hydrogels, Gao et al. (2022) synthesized an amorphous polyaryletherketone with carboxyl groups (PAEK-COOH). They fabricated scaffolds by LDM technology at a temperature of −30°C, where the porosity was hierarchically controlled and the implanted scaffold induced bone formation in vivo (Figure 3C) (Gao et al., 2022). Compared with other degradable materials, the scaffolds had high mechanical strength that made up for the poor mechanical properties of the low-temperature solution printing method. The electrostatic interaction of carboxyl groups induced HA mineralization and the porous surface further promoted cell adhesion. This research was a breakthrough in the field of bone regeneration and repair of polymeric materials, and research on combining the active factors of PAEK-COOH is currently underway.
3.3 LDM scaffolds of polymer-ceramic materials
High-molecular-weight polymers usually have poor geometric properties, such as a poor pore size and poor porosity and interconnection, which affect the attachment of scaffolds to cells. Generally, the ability of a polymer to support bone conduction is improved by adding ceramic filler to form a polymer–ceramic composite scaffold. Compared with pure polymer, ceramic composites have obvious benefits in terms of cell performance. Table 4 presents bone tissue engineering scaffolds with polymer–ceramic skeletons. We divide the scaffolds into three categories.
3.3.1 Polymer–ceramic scaffolds
The fabrication of PLLA/TCP composite scaffolds for bone tissue engineering was first presented by Xiong et al. (2002) as a new deposition manufacturing method. They mixed PLLA solution and TCP powder and finished the printing process in a low temperature environment of 0°C. The sublimation of dioxane in the freeze-drying procedure created micropores around the printed macropores. Yang et al. (2006) fabricated PLGA/TCP composited scaffolds by LDM and performed cell experiments to observe the mechanical strength, cell affinity, and degradation. Similarly, other PLGA/TCP scaffolds have been fabricated adopting double-nozzle technology and optimizing fabrication parameters to obtain the best mechanical properties and biocompatibility (Kai et al., 2009; Li et al., 2011). Lian et al. (2021) used LDM technology to develop sponge-like PLCL/HA scaffolds that promoted the interactions between MSCs and materials at a temperature of −28°C. In addition, the paracrine function of MSCs on scaffolds was improved, enhancing immunomodulation, angiogenesis, and osteogenic potential. The LDM-printed sponges with hierarchical interconnected pores could promote cell-scaffold interaction and upregulates osteogenic and vasogenic activity via the signaling paths (Figure 4A) (Lian et al., 2021). However, an in vitro cell experiments showed that cell viability on the surface of the scaffolds was time dependent and unaffected by the composited PLGA/nHA (Chen et al., 2013a).
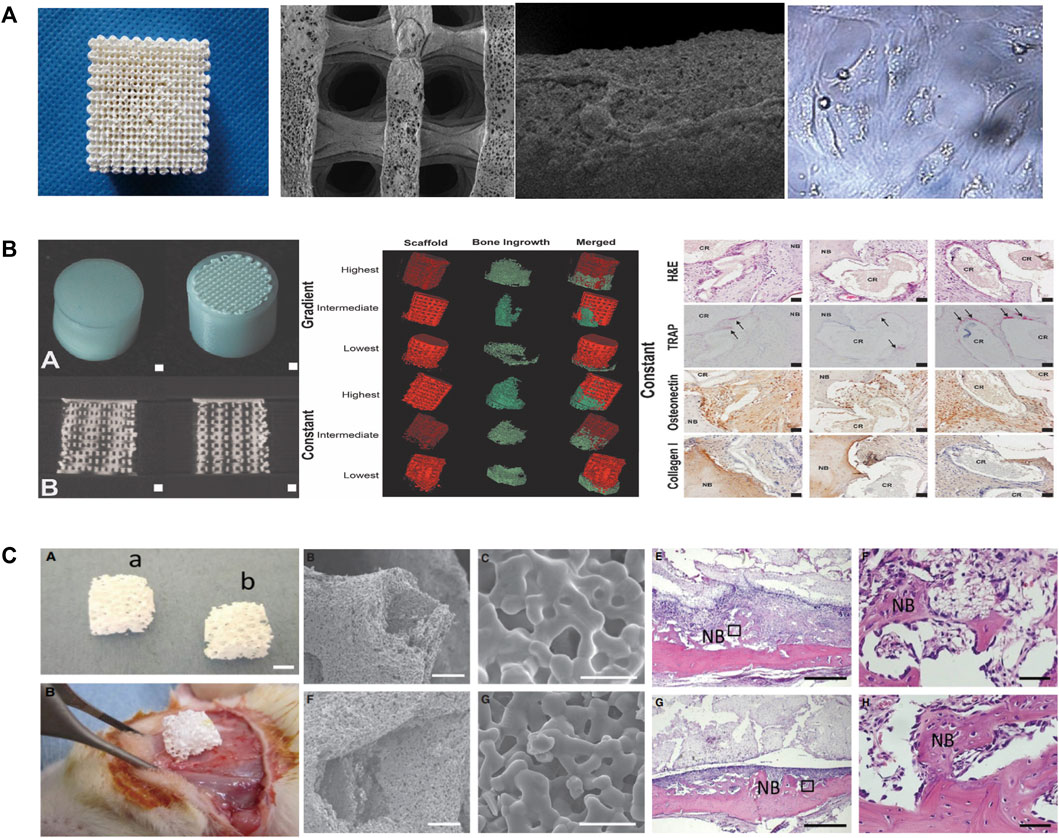
FIGURE 4. (A) Representative of the 3D porous nHA/PLGA composite scaffolds. SEM images of the porous PLGA/n-HA scaffolds and microscopic images of cells. (B) Visualization of the implant and formation of new bones via µCT. H&E, TRAP, osteonectin, and collagen type I staining of decalcified sections of porous structures. (C) Morphological characteristics and in vivo implantation of the scaffolds. The representative histological analysis of new bone formation after implantation. SEM, Scanning electron microscope; LDM, low-temperature deposition manufacturing; H&E, Hematoxylin and eosin; TRAP, Tartrate-resistant acid phosphatase.
3.3.2 Polymer-ceramic scaffolds with hydrogels
Hydrogels and collagen have been used to increase the hydrophilicity of scaffolds composited with ceramics and polymeric materials. For example, PLGA/β-TCP scaffolds prepared by LDM have been wrapped with collagen to improve the hydrophilicity and osteogenic differentiation (Wang et al., 2012; Zhang et al., 2017; Diloksumpan et al., 2020 composited hydrogel and calcium phosphate to prepare the scaffolds at ambient temperature (20°C–25°C) and encased the scaffolds in a PCL shell (Diloksumpan et al., 2020). They found that scaffolds with constant pores had better osteogenic properties than gradient scaffolds and that bone growth was enhanced in only one direction. Figure 4B presents the formation of new bones in scaffolds and the staining of hematoxylin and eosin, tartrate-resistant acidic phosphatase, osteonectin, and collagen type I in decalcified sections of porous structures (Diloksumpan et al., 2020). Similarly, Dou et al. developed a PLGA/HA framework and filled it with gelatin through LDM (Dou et al., 2021). The combination between the scaffolds and the original tissue was closer than that for single-material ones.
3.3.3 Polymer ceramic scaffolds obtained drugs or bioactive factors
Another major advantage of LDM in biomaterial application is the potential of including drugs and growth factors to improve bone healing or resist infection during the creation of polymer–ceramic composites. Dong et al. (2014) proposed a method of using solvent evaporation and low-temperature drying technology to prepare implants, which were composited with poly-DL-lactide, HA, and anti-tuberculosis drugs. Salvianolic acid B (SB) is an active component extracted from danshen and it can improve osteogenesis and angiogenesis (Cui et al., 2012). Lin et al. printed the composited scaffolds composed of PLGA, β-TCP, and SB through LDM at a temperature of −28°C and evaluated the effects on spinal fusion models (Lin et al., 2019). Icariin is another bioactive factor used to producing composite scaffolds. It is compounded into degradable scaffolds fabricated with polymer ceramic composites to provide mechanical support and promote the process of bone regeneration (Lai et al., 2018; Zhang et al., 2021). Chen et al. (2012); Chen et al. (2013b) incorporated BMP-2 and phytomolecule icaritin into PLGA/TCP scaffolds and found that icariin enhanced bone calcium deposition and regeneration.
Wang et al., 2017a printed scaffolds through LDM with materials composited of Ca-P, PLLA and BMP-2 on a custom-made cryogenic substrate at the temperature of −30°C. The scaffolds had well biological activity and sustained release of Ca2+ and BMP-2. Another type of diphasic magnetic scaffold was fabricated by LDM with PLGA, collagen, nano-hydroxyapatite, and Fe2O3 using a low-temperature rapid prototyping instrument at −4°C (Huang et al., 2018). It had good biocompatibility and matched better to the structure of cartilage or subchondral bones. Yoshida et al. (2015) fabricated PLGA/β-TCP scaffolds combined with fibroblast growth factor-2, which are showed good bioeffect for bone augmentation. There are other works on the loading of active factors, such as platelet-rich fibrin, magnesium, cucurbitacin B, osteogenic peptide, arginine-glycine-aspartic acid (RGD) and CaO2 microspheres, on scaffold materials to increase osteogenesis (Wei et al., 2015; Song et al., 2018; Lai et al., 2019; Cheng et al., 2021; Lai et al., 2022; Wang et al., 2022). It is worth mentioning that the platelet-rich fibrin facilitates hemostasis and the secretion of growth factors while degrading fibrin (Song et al., 2018). Magnesium provides mechanical properties and biodegradability for polymer-ceramic scaffolds (Lai et al., 2019). Scaffolds fabricated at −30°C had 3D porous structures and enhanced the new bone formation within the tunnel after implantation (Figure 4C) (Lai et al., 2019). Wang et al. (2022) fabricated scaffolds on a receiving platform at a temperature of −10°C and showed that CaO2 improves the expression of transcription factors by releasing O2 and thus promotes osteogenesis. RGD-containing peptides have the ability for modulation of cell adhesion and differentiation (Li et al., 2017).
3.4 Others
There are scaffolds that are constructed without compositing a ceramic or polymer framework. Table 5 presents bone tissue engineering scaffolds based on other materials. Lode et al. (2016) dispersed high-density collagen to stabilize structures in LDM and adopted chemical crosslinking with 1-ethyl-3-(3-dimethylaminopropyl) carbodiimide. Zhang et al. (2007) prepared scaffolds comprising collagen/chitosan/BMP-7 materials and implanted them into defects of mandible. Similarly, Reed et al. (2016) developed an acellular scaffold with chitosan/alginate and controlled the structure of the scaffold adopting LDM technology to improve cell influx and distribution. Lee et al. (2018) used decellularized extracellular matrix) to induce cellular activities and SF to achieve proper mechanical strength. The scaffolds were fabricated on a low-temperature (−40°C) stage using a 300-μm nozzle at a speed of 10 mm s−1. 3D-printed scaffolds have been fabricated with gelatin and SF, platelets, or polyols adopting crosslinking and freeze-drying technologies (Zhu et al., 2016; Liu et al., 2021a). He et al. (2021) combined natural modified protein technologies with LDM to fabricate scaffolds of BMP-2 and Human Bata Defensin-3 (hBD3). The scaffolds could realize bone induction through BMP-2 and antibacterial properties through hBD3. Karamat-Ullah et al. (2021) developed the silica-SF bio-ink to print scaffolds by LDM. The antibacterial peptide was covalently linked to SF and had effective bactericidal ability.
4 Conclusion and challenges
In this article, we introduced the technical process of LDM and reviewed the applications of this technology to bone tissue engineering scaffolds. As a green manufacturing method, LDM not only controls the pore size of the scaffolds through the use of CAD tools, but also preserves the activity of biological factors. However, there remain many challenges in the LDM of scaffolds. First, the mechanical strength of a scaffold fabricated by LDM is commonly low. Although composited materials improve the mechanical properties, the strength requirements of load-bearing bone are not met. In addition, it is difficult to select a solvent that dissolves both synthetic and natural polymers. Few solvents have been used up to the point and it is undeniable that the benefits of this technology are underutilized. Secondly, it is difficult to balance the degradation of scaffold materials with the regeneration of new bone tissue. There is no doubt that such osteogenesis is not ideal when the degradation rate of scaffolds is faster than the regeneration rate of new bone. Furthermore, the majority of the degradation products are acidic and thus mildly toxic or even toxic to the human body. In addition, a suitable substrate temperature and requirements of the printing platform are not clear. In brief, materials printed on cold plates and freezers form ice crystals in different orientations, which control the microstructures of porous scaffolds. Developing a more suitable low temperature printing system is one of the future research directions.
5 Prospects
Bone regeneration is not just a simple process of bone formation and resorption. It is a multi-system including the musculoskeletal system and immune system (Zheng et al., 2021). In addition, the combination of antimicrobial drugs and biomaterials can effectively control bone infection and improve prognosis while promoting bone repair (Hu et al., 2021). In contrast to traditional manufacturing techniques, 3D printing, particularly LDM, has the advantage in that it can produce tissue engineering scaffolds with customized forms, bioactivity, porosity, and mechanical properties. One approach of bioprinting involves injecting cell-rich hydrogels into scaffolds, which has drawbacks including an uneven cell distribution and an injection pressure that affects cell activity and scaffold porosity. An exceptional benefit of LDM is the ability to fabricate scaffolds with linked macro-pores and micro-pores while consistently allowing the integration of biomolecules, such as live cells, into the scaffolds. In addition, LDM allows the customization of scaffolds through changing the nozzles and combining various RPM techniques.
An ideal bone tissue engineering scaffold fabricated by LDM has the following properties. The scaffolds must, first and foremost, be of high mechanical quality to suit the demands of bone tissue, particularly the stability required by load-bearing bone. Second, the material should have strong cytocompatibility and a capacity for bone conduction, vascularization, nervousness, and disintegration, among other good biological qualities. Third, the interfacial repair of complicated bone defects depends on the creation of multilayer gradient scaffolds made of bone, cartilage, and soft tissue. LDM is a promising RPM technique that promises the fabrication of ideal scaffolds and is expected to become indispensable in bone tissue engineering. The next step is to investigate various solvent systems for blending different materials or bioactive components and to develop an ideal printing workstation. Bioinks with optimal properties have been prepared by adjusting parameters to obtain a balance of mechanical properties and biological activity in scaffolds. In future work, composite scaffolds with near-ideal levels should be further prepared with LDM technology to promote the regeneration of bone tissue and surrounding tissues and ultimately realize multi-organ bioprinting.
Author contributions
TS and JW: paper writing and data collection; HH, XL, WZ, and JZ: data collection; HW and ZL: supervision and paper revision. All authors contributed to the article and approved the submitted version.
Funding
This study was supported by the Science and Technology Innovation Foundation of Dalian (2022JJ12SN045) and the Natural Science Foundation of Liaoning Province (2022-MS-322). The funders had no role in the study design, data collection and analysis, decision to publish, or preparation of the manuscript.
Acknowledgments
We thank Liwen Bianji (Edanz) (www.liwenbianji.cn) for editing the language of a draft of this manuscript. We would like to thank all the participants in the studies.
Conflict of interest
The authors declare that the research was conducted in the absence of any commercial or financial relationships that could be construed as a potential conflict of interest.
Publisher’s note
All claims expressed in this article are solely those of the authors and do not necessarily represent those of their affiliated organizations, or those of the publisher, the editors and the reviewers. Any product that may be evaluated in this article, or claim that may be made by its manufacturer, is not guaranteed or endorsed by the publisher.
References
Ahlfeld, T., Doberenz, F., Kilian, D., Vater, C., Korn, P., Lauer, G., et al. (2018). Bioprinting of mineralized constructs utilizing multichannel plotting of a self-setting calcium phosphate cement and a cell-laden bioink. Biofabrication 10, 045002. doi:10.1088/1758-5090/aad36d
Amiryaghoubi, N., Fathi, M., Pesyan, N. N., Samiei, M., Barar, J., and Omidi, Y. (2020). Bioactive polymeric scaffolds for osteogenic repair and bone regenerative medicine. Med. Res. Rev. 40, 1833–1870. doi:10.1002/med.21672
Baino, F., and Fiume, E. (2020). 3D printing of hierarchical scaffolds based on mesoporous bioactive glasses (MBGs)-Fundamentals and applications. Mater. (Basel) 13, 1688. doi:10.3390/ma13071688
Bolaños, R. V., Castilho, M., De Grauw, J., Cokelaere, S., Plomp, S., Groll, J., et al. (2020). Long-term in vivo performance of low-temperature 3D-printed bioceramics in an equine model. ACS Biomater. Sci. Eng. 6, 1681–1689. doi:10.1021/acsbiomaterials.9b01819
Bružauskaitė, I., Bironaitė, D., Bagdonas, E., and Bernotienė, E. (2016). Scaffolds and cells for tissue regeneration: Different scaffold pore sizes—different cell effects. Cytotechnology 68, 355–369. doi:10.1007/s10616-015-9895-4
Castilho, M., Dias, M., Gbureck, U., Groll, J., Fernandes, P., Pires, I., et al. (2013). Fabrication of computationally designed scaffolds by low temperature 3D printing. Biofabrication 5, 035012. doi:10.1088/1758-5082/5/3/035012
Chen, L., Zhu, W.-M., Fei, Z.-Q., Chen, J.-L., Xiong, J.-Y., Zhang, J.-F., et al. (2013a). The study on biocompatibility of porous nHA/PLGA composite scaffolds for tissue engineering with rabbit chondrocytes in vitro. BioMed Res. Int. 2013, 1–6. doi:10.1155/2013/412745
Chen, M., Li, Y., Liu, S., Feng, Z., Wang, H., Yang, D., et al. (2021). Hierarchical macro-microporous WPU-ECM scaffolds combined with microfracture promote in situ articular cartilage regeneration in rabbits. Bioact. Mater 6, 1932–1944. doi:10.1016/j.bioactmat.2020.12.009
Chen, S. H., Lei, M., Xie, X. H., Zheng, L. Z., Yao, D., Wang, X. L., et al. (2013b). PLGA/TCP composite scaffold incorporating bioactive phytomolecule icaritin for enhancement of bone defect repair in rabbits. Acta Biomater. 9, 6711–6722. doi:10.1016/j.actbio.2013.01.024
Chen, S. H., Wang, X. L., Xie, X. H., Zheng, L. Z., Yao, D., Wang, D. P., et al. (2012). Comparative study of osteogenic potential of a composite scaffold incorporating either endogenous bone morphogenetic protein-2 or exogenous phytomolecule icaritin: An in vitro efficacy study. Acta Biomater. 8, 3128–3137. doi:10.1016/j.actbio.2012.04.030
Chen, S., Shi, Y., Zhang, X., and Ma, J. (2020). Evaluation of BMP-2 and VEGF loaded 3D printed hydroxyapatite composite scaffolds with enhanced osteogenic capacity in vitro and in vivo. Mater Sci. Eng. C Mater Biol. Appl. 112, 110893. doi:10.1016/j.msec.2020.110893
Cheng, W. X., Liu, Y. Z., Meng, X. B., Zheng, Z. T., Li, L. L., Ke, L. Q., et al. (2021). PLGA/β-TCP composite scaffold incorporating cucurbitacin B promotes bone regeneration by inducing angiogenesis. J. Orthop. Transl. 31, 41–51. doi:10.1016/j.jot.2021.10.002
Cui, L., Li, T., Liu, Y., Zhou, L., Li, P., Xu, B., et al. (2012). Salvianolic acid B prevents bone loss in prednisone-treated rats through stimulation of osteogenesis and bone marrow angiogenesis. PLoS One 7, e34647. doi:10.1371/journal.pone.0034647
Dadhich, P., Das, B., Pal, P., Srivas, P. K., Dutta, J., Ray, S., et al. (2016). A simple approach for an eggshell-based 3D-printed osteoinductive multiphasic calcium phosphate scaffold. ACS Appl. Mater Interfaces 8, 11910–11924. doi:10.1021/acsami.5b11981
Dadhich, P., Srivas, P. K., Das, B., Pal, P., Dutta, J., Maity, P., et al. (2021). Direct 3D printing of seashell precursor toward engineering a multiphasic calcium phosphate bone graft. ACS Biomater. Sci. Eng. 7, 3806–3820. doi:10.1021/acsbiomaterials.1c00303
Diloksumpan, P., Bolaños, R. V., Cokelaere, S., Pouran, B., De Grauw, J., Van Rijen, M., et al. (2020). Orthotopic bone regeneration within 3D printed bioceramic scaffolds with region-dependent porosity gradients in an equine model. Adv. Healthc. Mater 9, e1901807. doi:10.1002/adhm.201901807
Do, A. V., Khorsand, B., Geary, S. M., and Salem, A. K. (2015). 3D printing of scaffolds for tissue regeneration applications. Adv. Healthc. Mater 4, 1742–1762. doi:10.1002/adhm.201500168
Dong, J., Zhang, S., Liu, H., Li, X., Liu, Y., and Du, Y. (2014). Novel alternative therapy for spinal tuberculosis during surgery: Reconstructing with anti-tuberculosis bioactivity implants. Expert Opin. Drug Deliv. 11, 299–305. doi:10.1517/17425247.2014.872625
Dou, Y., Huang, J., Xia, X., Wei, J., Zou, Q., Zuo, Y., et al. (2021). A hierarchical scaffold with a highly pore-interconnective 3D printed PLGA/n-HA framework and an extracellular matrix like gelatin network filler for bone regeneration. J. Mater Chem. B 9, 4488–4501. doi:10.1039/d1tb00662b
Fan, H., Hu, Y., Zhang, C., Li, X., Lv, R., Qin, L., et al. (2006). Cartilage regeneration using mesenchymal stem cells and a PLGA–gelatin/chondroitin/hyaluronate hybrid scaffold. Biomaterials 27, 4573–4580. doi:10.1016/j.biomaterials.2006.04.013
Galvao, J., Davis, B., Tilley, M., Normando, E., Duchen, M. R., and Cordeiro, M. F. (2014). Unexpected low-dose toxicity of the universal solvent DMSO. Faseb J. 28, 1317–1330. doi:10.1096/fj.13-235440
Gao, X., Wang, H., Luan, S., and Zhou, G. (2022). Low-temperature printed hierarchically porous induced-biomineralization polyaryletherketone scaffold for bone tissue engineering. Adv. Healthc. Mater 11, e2200977. doi:10.1002/adhm.202200977
Geng, M., Zhang, Q., Gu, J., Yang, J., Du, H., Jia, Y., et al. (2021). Construction of a nanofiber network within 3D printed scaffolds for vascularized bone regeneration. Biomater. Sci. 9, 2631–2646. doi:10.1039/d0bm02058c
Gentile, P., Chiono, V., Carmagnola, I., and Hatton, P. V. (2014). An overview of poly(lactic-co-glycolic) acid (PLGA)-based biomaterials for bone tissue engineering. Int. J. Mol. Sci. 15, 3640–3659. doi:10.3390/ijms15033640
Geven, M. A., and Grijpma, D. W. (2019). Additive manufacturing of composite structures for the restoration of bone tissue. Multifunct. Mater. 2, 024003. doi:10.1088/2399-7532/ab201f
Guo, T., Holzberg, T. R., Lim, C. G., Gao, F., Gargava, A., Trachtenberg, J. E., et al. (2017). 3D printing PLGA: A quantitative examination of the effects of polymer composition and printing parameters on print resolution. Biofabrication 9, 024101. doi:10.1088/1758-5090/aa6370
Guo, T., Lim, C., Noshin, M., Ringel, J. P., and Fisher, J. P. (2018). 3D printing bioactive PLGA scaffolds using DMSO as a removable solvent. Bioprinting 10, e00038. doi:10.1016/j.bprint.2018.e00038
He, S., Lin, K. F., Sun, Z., Song, Y., Zhao, Y. N., Wang, Z., et al. (2016). Effects of nano-hydroxyapatite/poly(DL-lactic-co-glycolic acid) microsphere-based composite scaffolds on repair of bone defects: Evaluating the role of nano-hydroxyapatite content. Artif. Organs 40, E128–E135. doi:10.1111/aor.12741
He, W., Wei, D., Zhang, J., Huang, X., He, D., Liu, B., et al. (2021). Novel bone repairing scaffold consisting of bone morphogenetic Protein-2 and human Beta Defensin-3. J. Biol. Eng. 15, 5. doi:10.1186/s13036-021-00258-5
Hu, T., Xu, M.-E., Yan, M., Guo, M., Shi, R., and Zhou, Q.-Q. (2015). Low-temperature deposition manufacturing and property of polylactic-co-glycolic acid composite scaffolds at different ratios. Chin. J. Tissue Eng. Res. 19, 1805. doi:10.3969/j.issn.2095-4344.2015.12.001
Hu, Y., Li, X., Zhang, Q., Gu, Z., Luo, Y., Guo, J., et al. (2021). Exosome-guided bone targeted delivery of Antagomir-188 as an anabolic therapy for bone loss. Bioact. Mater 6, 2905–2913. doi:10.1016/j.bioactmat.2021.02.014
Huang, J., Liu, W., Liang, Y., Li, L., Duan, L., Chen, J., et al. (2018). Preparation and biocompatibility of diphasic magnetic nanocomposite scaffold. Mater Sci. Eng. C Mater Biol. Appl. 87, 70–77. doi:10.1016/j.msec.2018.02.003
Huh, J., Lee, J., Kim, W., Yeo, M., and Kim, G. (2018). Preparation and characterization of gelatin/α-TCP/SF biocomposite scaffold for bone tissue regeneration. Int. J. Biol. Macromol. 110, 488–496. doi:10.1016/j.ijbiomac.2017.09.030
Inzana, J. A., Olvera, D., Fuller, S. M., Kelly, J. P., Graeve, O. A., Schwarz, E. M., et al. (2014). 3D printing of composite calcium phosphate and collagen scaffolds for bone regeneration. Biomaterials 35, 4026–4034. doi:10.1016/j.biomaterials.2014.01.064
Johnson, B. N., and Jia, X. (2016). 3D printed nerve guidance channels: Computer-aided control of geometry, physical cues, biological supplements and gradients. Neural Regen. Res. 11, 1568–1569. doi:10.4103/1673-5374.193230
Jones, N. (2012). Science in three dimensions: The print revolution. Nature 487, 22–23. doi:10.1038/487022a
Kai, D., Prabhakaran, M. P., Stahl, B., Eblenkamp, M., Wintermantel, E., and Ramakrishna, S. (2012). Mechanical properties and in vitro behavior of nanofiber-hydrogel composites for tissue engineering applications. Nanotechnology 23, 095705. doi:10.1088/0957-4484/23/9/095705
Kai, H., Wang, X., Madhukar, K. S., Qin, L., Yan, Y., Zhang, R., et al. (2009). Fabrication of a two-level tumor bone repair biomaterial based on a rapid prototyping technique. Biofabrication 1, 025003. doi:10.1088/1758-5082/1/2/025003
Karamat-Ullah, N., Demidov, Y., Schramm, M., Grumme, D., Auer, J., Bohr, C., et al. (2021). 3D printing of antibacterial, biocompatible, and biomimetic hybrid aerogel-based scaffolds with hierarchical porosities via integrating antibacterial peptide-modified silk fibroin with silica nanostructure. ACS Biomater. Sci. Eng. 7, 4545–4556. doi:10.1021/acsbiomaterials.1c00483
Kim, H., Yang, G. H., Choi, C. H., Cho, Y. S., and Kim, G. (2018). Gelatin/PVA scaffolds fabricated using a 3D-printing process employed with a low-temperature plate for hard tissue regeneration: Fabrication and characterizations. Int. J. Biol. Macromol. 120, 119–127. doi:10.1016/j.ijbiomac.2018.07.159
Kim, W., Lee, H., Kim, Y., Choi, C. H., Lee, D., Hwang, H., et al. (2016). Versatile design of hydrogel-based scaffolds with manipulated pore structure for hard-tissue regeneration. Biomed. Mater 11, 055002. doi:10.1088/1748-6041/11/5/055002
Klammert, U., Vorndran, E., Reuther, T., Müller, F. A., Zorn, K., and Gbureck, U. (2010). Low temperature fabrication of magnesium phosphate cement scaffolds by 3D powder printing. J. Mater Sci. Mater Med. 21, 2947–2953. doi:10.1007/s10856-010-4148-8
Koski, C., Onuike, B., Bandyopadhyay, A., and Bose, S. (2018). Starch-hydroxyapatite composite bone scaffold fabrication utilizing a slurry extrusion-based solid freeform fabricator. Addit. Manuf. 24, 47–59. doi:10.1016/j.addma.2018.08.030
Kronemberger, G. S., Miranda, G., Silva, T. I. G., Gonçalves, R. M., Granjeiro, J. M., and Baptista, L. S. (2022). Large-scale, automated production of adipose-derived stem cell spheroids for 3D bioprinting. J. Vis. Exp. 1, 3430. doi:10.3791/63430
Lai, J., Wang, C., Liu, J., Chen, S., Liu, C., Huang, X., et al. (2022). Low temperature hybrid 3D printing of hierarchically porous bone tissue engineering scaffolds within situdelivery of osteogenic peptide and mesenchymal stem cells. Biofabrication 14, 045006. doi:10.1088/1758-5090/ac84b0
Lai, Y., Cao, H., Wang, X., Chen, S., Zhang, M., Wang, N., et al. (2018). Porous composite scaffold incorporating osteogenic phytomolecule icariin for promoting skeletal regeneration in challenging osteonecrotic bone in rabbits. Biomaterials 153, 1–13. doi:10.1016/j.biomaterials.2017.10.025
Lai, Y., Li, Y., Cao, H., Long, J., Wang, X., Li, L., et al. (2019). Osteogenic magnesium incorporated into PLGA/TCP porous scaffold by 3D printing for repairing challenging bone defect. Biomaterials 197, 207–219. doi:10.1016/j.biomaterials.2019.01.013
Lee, H., Yang, G. H., Kim, M., Lee, J., Huh, J., and Kim, G. (2018). Fabrication of micro/nanoporous collagen/dECM/silk-fibroin biocomposite scaffolds using a low temperature 3D printing process for bone tissue regeneration. Mater Sci. Eng. C Mater Biol. Appl. 84, 140–147. doi:10.1016/j.msec.2017.11.013
Lee, J., and Kim, G. (2018a). Calcium-deficient hydroxyapatite/collagen/platelet-rich plasma scaffold with controlled release function for hard tissue regeneration. ACS Biomater. Sci. Eng. 4, 278–289. doi:10.1021/acsbiomaterials.7b00640
Lee, J., and Kim, G. (2018b). Three-dimensional hierarchical nanofibrous collagen scaffold fabricated using fibrillated collagen and pluronic F-127 for regenerating bone tissue. ACS Appl. Mater Interfaces 10, 35801–35811. doi:10.1021/acsami.8b14088
Lee, S. C., Gillispie, G., Prim, P., and Lee, S. J. (2020). Physical and chemical factors influencing the printability of hydrogel-based extrusion bioinks. Chem. Rev. 120, 10834–10886. doi:10.1021/acs.chemrev.0c00015
Leu, M.-C., Zhang, W., and Sui, G. J. C. A. (2000). An experimental and analytical study of ice part fabrication with rapid freeze prototyping. CIRP Ann. 49, 147–150. doi:10.1016/S0007-8506(07)62916-3
Li, J., Tan, L., Liu, X., Cui, Z., Yang, X., Yeung, K. W. K., et al. (2017). Balancing bacteria-osteoblast competition through selective physical puncture and biofunctionalization of ZnO/Polydopamine/Arginine-Glycine-Aspartic acid-cysteine nanorods. ACS Nano 11, 11250–11263. doi:10.1021/acsnano.7b05620
Li, J., Zhang, L., Lv, S., Li, S., Wang, N., and Zhang, Z. (2011). Fabrication of individual scaffolds based on a patient-specific alveolar bone defect model. J. Biotechnol. 151, 87–93. doi:10.1016/j.jbiotec.2010.10.080
Lian, M., Sun, B., Han, Y., Yu, B., Xin, W., Xu, R., et al. (2021). A low-temperature-printed hierarchical porous sponge-like scaffold that promotes cell-material interaction and modulates paracrine activity of MSCs for vascularized bone regeneration. Biomaterials 274, 120841. doi:10.1016/j.biomaterials.2021.120841
Lin, K. F., He, S., Song, Y., Wang, C. M., Gao, Y., Li, J. Q., et al. (2016). Low-temperature additive manufacturing of biomimic three-dimensional hydroxyapatite/collagen scaffolds for bone regeneration. ACS Appl. Mater Interfaces 8, 6905–6916. doi:10.1021/acsami.6b00815
Lin, S., Cui, L., Chen, G., Huang, J., Yang, Y., Zou, K., et al. (2019). PLGA/β-TCP composite scaffold incorporating salvianolic acid B promotes bone fusion by angiogenesis and osteogenesis in a rat spinal fusion model. Biomaterials 196, 109–121. doi:10.1016/j.biomaterials.2018.04.004
Liu, C., Bai, Z., Lin, J., Jiang, K., Huang, S., Zheng, W., et al. (2021a). 3D printing silk-gelatin-propanediol scaffold with enhanced osteogenesis properties through p-Smad1/5/8 activated Runx2 pathway. J. Biomater. Sci. Polym. Ed. 32, 1515–1529. doi:10.1080/09205063.2021.1912977
Liu, H., Qiu, L., Liu, H., Li, F., Fan, Y., Meng, L., et al. (2021b). Effects of fiber cross-angle structures on the mechanical property of 3D printed scaffolds and performance of seeded mc3t3-E1 cells. ACS Omega 6, 33665–33675. doi:10.1021/acsomega.1c04672
Liu, L., Xiong, Z., Yan, Y., Hu, Y., Zhang, R., and Wang, S. (2007). Porous morphology, porosity, mechanical properties of poly(α-hydroxy acid)–tricalcium phosphate composite scaffolds fabricated by low-temperature deposition. J. Biomed. Mater Res. A 82, 618–629. doi:10.1002/jbm.a.31177
Liu, L., Xiong, Z., Yan, Y., Zhang, R., Wang, X., and Jin, L. (2009a). Multinozzle low-temperature deposition system for construction of gradient tissue engineering scaffolds. J. Biomed. Mater Res. B Appl. Biomater. 88, 254–263. doi:10.1002/jbm.b.31176
Liu, L., Xiong, Z., Zhang, R., Jin, L., and Yan, Y. (2009b). A novel osteochondral scaffold fabricated via multi-nozzle low-temperature deposition manufacturing. J. Bioact. Compatible Polym. 24, 18–30. doi:10.1177/0883911509102347
Liu, W., Wang, D., Huang, J., Wei, Y., Xiong, J., Zhu, W., et al. (2017). Low-temperature deposition manufacturing: A novel and promising rapid prototyping technology for the fabrication of tissue-engineered scaffold. Mater Sci. Eng. C Mater Biol. Appl. 70, 976–982. doi:10.1016/j.msec.2016.04.014
Lode, A., Meyer, M., Brüggemeier, S., Paul, B., Baltzer, H., Schröpfer, M., et al. (2016). Additive manufacturing of collagen scaffolds by three-dimensional plotting of highly viscous dispersions. Biofabrication 8, 015015. doi:10.1088/1758-5090/8/1/015015
Long, J., Zhang, W., Chen, Y., Teng, B., Liu, B., Li, H., et al. (2021). Multifunctional magnesium incorporated scaffolds by 3D-Printing for comprehensive postsurgical management of osteosarcoma. Biomaterials 275, 120950. doi:10.1016/j.biomaterials.2021.120950
Luo, C., Xie, R., Zhang, J., Liu, Y., Li, Z., Zhang, Y., et al. (2020). Low-temperature three-dimensional printing of tissue cartilage engineered with gelatin methacrylamide. Tissue Eng. Part C Methods 26, 306–316. doi:10.1089/ten.tec.2020.0053
Lutolf, M. P., and Hubbell, J. A. (2005). Synthetic biomaterials as instructive extracellular microenvironments for morphogenesis in tissue engineering. Nat. Biotechnol. 23, 47–55. doi:10.1038/nbt1055
Mehesz, A. N., Brown, J., Hajdu, Z., Beaver, W., Da Silva, J. V., Visconti, R. P., et al. (2011). Scalable robotic biofabrication of tissue spheroids. Biofabrication 3, 025002. doi:10.1088/1758-5082/3/2/025002
Papastavrou, E., Breedon, P., and Fairhurst, D. (2018). Low-temperature deposition modeling of β-TCP scaffolds with controlled bimodal porosity. Methods Mol. Biol. 1758, 41–54. doi:10.1007/978-1-4939-7741-3_4
Qin, K., Parisi, C., and Fernandes, F. M. (2021). Recent advances in ice templating: From biomimetic composites to cell culture scaffolds and tissue engineering. J. Mater Chem. B 9, 889–907. doi:10.1039/d0tb02506b
Rahmanian-Schwarz, A., Held, M., Knoeller, T., Stachon, S., Schmidt, T., Schaller, H. E., et al. (2014). In vivo biocompatibility and biodegradation of a novel thin and mechanically stable collagen scaffold. J. Biomed. Mater Res. A 102, 1173–1179. doi:10.1002/jbm.a.34793
Raina, D. B., Isaksson, H., Teotia, A. K., Lidgren, L., Tägil, M., and Kumar, A. (2016). Biocomposite macroporous cryogels as potential carrier scaffolds for bone active agents augmenting bone regeneration. J. Control Release 235, 365–378. doi:10.1016/j.jconrel.2016.05.061
Reed, S., Lau, G., Delattre, B., Lopez, D. D., Tomsia, A. P., and Wu, B. M. (2016). Macro- and micro-designed chitosan-alginate scaffold architecture by three-dimensional printing and directional freezing. Biofabrication 8, 015003. doi:10.1088/1758-5090/8/1/015003
Rezwan, K., Chen, Q. Z., Blaker, J. J., and Boccaccini, A. R. (2006). Biodegradable and bioactive porous polymer/inorganic composite scaffolds for bone tissue engineering. Biomaterials 27, 3413–3431. doi:10.1016/j.biomaterials.2006.01.039
Roseti, L., Parisi, V., Petretta, M., Cavallo, C., Desando, G., Bartolotti, I., et al. (2017). Scaffolds for bone tissue engineering: State of the art and new perspectives. Mater Sci. Eng. C Mater Biol. Appl. 78, 1246–1262. doi:10.1016/j.msec.2017.05.017
Salgado, C. L., Grenho, L., Fernandes, M. H., Colaço, B. J., and Monteiro, F. J. (2016). Biodegradation, biocompatibility, and osteoconduction evaluation of collagen-nanohydroxyapatite cryogels for bone tissue regeneration. J. Biomed. Mater Res. A 104, 57–70. doi:10.1002/jbm.a.35540
Sfeir, J. G., Drake, M. T., Khosla, S., and Farr, J. N. (2022). Skeletal aging. Skelet. Aging. Mayo Clin. Proc. 97, 1194–1208. doi:10.1016/j.mayocp.2022.03.011
Simorgh, S., Alasvand, N., Khodadadi, M., Ghobadi, F., Malekzadeh Kebria, M., Brouki Milan, P., et al. (2022). Additive manufacturing of bioactive glass biomaterials. Methods 208, 75–91. doi:10.1016/j.ymeth.2022.10.010
Song, Y., Lin, K., He, S., Wang, C., Zhang, S., Li, D., et al. (2018). Nano-biphasic calcium phosphate/polyvinyl alcohol composites with enhanced bioactivity for bone repair via low-temperature three-dimensional printing and loading with platelet-rich fibrin. Int. J. Nanomedicine 13, 505–523. doi:10.2147/ijn.s152105
Wang, C., Meng, G., Zhang, L., Xiong, Z., and Liu, J. (2012). Physical properties and biocompatibility of a core-sheath structure composite scaffold for bone tissue engineering in vitro. J. Biomed. Biotechnol. 2012, 579141–579149. doi:10.1155/2012/579141
Wang, C., Zhao, Q., and Wang, M. (2017a). Cryogenic 3D printing for producing hierarchical porous and rhBMP-2-loaded Ca-P/PLLA nanocomposite scaffolds for bone tissue engineering. Biofabrication 9, 025031. doi:10.1088/1758-5090/aa71c9
Wang, D. X., He, Y., Bi, L., Qu, Z. H., Zou, J. W., Pan, Z., et al. (2013). Enhancing the bioactivity of Poly(lactic-co-glycolic acid) scaffold with a nano-hydroxyapatite coating for the treatment of segmental bone defect in a rabbit model. Int. J. Nanomedicine 8, 1855–1865. doi:10.2147/ijn.s43706
Wang, F., Zhang, Y. C., Zhou, H., Guo, Y. C., and Su, X. X. (2014). Evaluation of in vitro and in vivo osteogenic differentiation of nano-hydroxyapatite/chitosan/poly(lactide-co-glycolide) scaffolds with human umbilical cord mesenchymal stem cells. J. Biomed. Mater Res. A 102, 760–768. doi:10.1002/jbm.a.34747
Wang, X., Rijff, B. L., and Khang, G. (2017b). A building-block approach to 3D printing a multichannel, organ-regenerative scaffold. J. Tissue Eng. Regen. Med. 11, 1403–1411. doi:10.1002/term.2038
Wang, Y. J., Jeng, U. S., and Hsu, S. H. (2018). Biodegradable water-based polyurethane shape memory elastomers for bone tissue engineering. ACS Biomater. Sci. Eng. 4, 1397–1406. doi:10.1021/acsbiomaterials.8b00091
Wang, Y., Xie, C., Zhang, Z., Liu, H., Xu, H., Peng, Z., et al. (2022). 3D printed integrated bionic oxygenated scaffold for bone regeneration. ACS Appl. Mater Interfaces 14, 29506–29520. doi:10.1021/acsami.2c04378
Wei, X., He, K., Yu, S., Zhao, W., Xing, G., Liu, Y., et al. (2015). RGD peptide-modified poly(lactide-co-glycolide)/<I>β</I>-Tricalcium phosphate scaffolds increase bone formation after transplantation in a rabbit model. J. Biomaterials Tissue Eng. 5, 378–386. doi:10.1166/jbt.2015.1330
Wubneh, A., Tsekoura, E. K., Ayranci, C., and Uludağ, H. (2018). Current state of fabrication technologies and materials for bone tissue engineering. Acta Biomater. 80, 1–30. doi:10.1016/j.actbio.2018.09.031
Xiong, Z., Yan, Y., Wang, S., Zhang, R., and Zhang, C. J. S. M. (2002). Fabrication of porous scaffolds for bone tissue engineering via low-temperature deposition. Screen. Mat. 46, 771–776. doi:10.1016/s1359-6462(02)00071-4
Xu, M., Li, Y., Suo, H., Yan, Y., Liu, L., Wang, Q., et al. (2010). Fabricating a pearl/PLGA composite scaffold by the low-temperature deposition manufacturing technique for bone tissue engineering. Biofabrication 2, 025002. doi:10.1088/1758-5082/2/2/025002
Yang, F., Cui, W., Xiong, Z., Liu, L., Bei, J., and Wang, S. (2006). Poly (l, l-lactide-co-glycolide)/tricalcium phosphate composite scaffold and its various changes during degradation in vitro. Polym. Degrad. Stab. 91, 3065–3073. doi:10.1016/j.polymdegradstab.2006.08.008
Yang, T., Hu, Y., Wang, C., and Binks, B. P. (2017). Fabrication of hierarchical macroporous biocompatible scaffolds by combining pickering high internal phase emulsion templates with three-dimensional printing. ACS Appl. Mater Interfaces 9, 22950–22958. doi:10.1021/acsami.7b05012
Yoshida, T., Miyaji, H., Otani, K., Inoue, K., Nakane, K., Nishimura, H., et al. (2015). Bone augmentation using a highly porous PLGA/β-TCP scaffold containing fibroblast growth factor-2. J. Periodontal Res. 50, 265–273. doi:10.1111/jre.12206
Zafeiris, K., Brasinika, D., Karatza, A., Koumoulos, E., Karoussis, I. K., Kyriakidou, K., et al. (2021). Additive manufacturing of hydroxyapatite-chitosan-genipin composite scaffolds for bone tissue engineering applications. Mater Sci. Eng. C Mater Biol. Appl. 119, 111639. doi:10.1016/j.msec.2020.111639
Zhang, B., Shen, S., Xian, H., Dai, Y., Guo, W., Li, X., et al. (2019). Fabrication of poly (lactic-co-glycolic acid)/decellularized articular cartilage extracellular matrix scaffold by three-dimensional printing technology and investigating its physicochemical properties. Zhongguo Xiu Fu Chong Jian Wai Ke Za Zhi 33, 1011–1018. doi:10.7507/1002-1892.201901082
Zhang, J. T., Zhang, S. S., Liu, C. G., Kankala, R. K., Chen, A. Z., and Wang, S. B. (2021). Low-temperature extrusion-based 3D printing of icariin-laden scaffolds for osteogenesis enrichment. Regen. Ther. 16, 53–62. doi:10.1016/j.reth.2021.01.001
Zhang, P., Hong, Z., Yu, T., Chen, X., and Jing, X. (2009). In vivo mineralization and osteogenesis of nanocomposite scaffold of poly(lactide-co-glycolide) and hydroxyapatite surface-grafted with poly(L-lactide). Biomaterials 30, 58–70. doi:10.1016/j.biomaterials.2008.08.041
Zhang, R., Yan, Y., and Lin, F. (2008). Low temperature rapid prototyping (LT-RP) and green manufacturing. Manuf. Technol. Mach. Tool 4, 71–75.
Zhang, T., Zhang, H., Zhang, L., Jia, S., Liu, J., Xiong, Z., et al. (2017). Biomimetic design and fabrication of multilayered osteochondral scaffolds by low-temperature deposition manufacturing and thermal-induced phase-separation techniques. Biofabrication 9, 025021. doi:10.1088/1758-5090/aa7078
Zhang, W., Leu, M. C., Ji, Z., and Yan, Y. (1999). Rapid freezing prototyping with water. Mater. Des. 20, 139–145. doi:10.1016/S0261-3069(99)00020-5
Zhang, Y., Li, C., Zhang, W., Deng, J., Nie, Y., Du, X., et al. (2022). 3D-printed NIR-responsive shape memory polyurethane/magnesium scaffolds with tight-contact for robust bone regeneration. Bioact. Mater 16, 218–231. doi:10.1016/j.bioactmat.2021.12.032
Zhang, Y., Song, J., Shi, B., Wang, Y., Chen, X., Huang, C., et al. (2007). Combination of scaffold and adenovirus vectors expressing bone morphogenetic protein-7 for alveolar bone regeneration at dental implant defects. Biomaterials 28, 4635–4642. doi:10.1016/j.biomaterials.2007.07.009
Zhao, L., Chen, S., Xie, C., Liang, Q., Xu, D., Chen, W., et al. (2022). The fabrication of multifunctional sodium alginate scaffold incorporating ibuprofen-loaded modified PLLA microspheres based on cryogenic 3D printing. J. Biomater. Sci. Polym. Ed. 33, 1269–1288. doi:10.1080/09205063.2022.2049059
Zheng, K., Niu, W., Lei, B., and Boccaccini, A. R. (2021). Immunomodulatory bioactive glasses for tissue regeneration. Acta Biomater. 133, 168–186. doi:10.1016/j.actbio.2021.08.023
Zhu, W., Xu, C., Ma, B. P., Zheng, Z. B., Li, Y. L., Ma, Q., et al. (2016). Three-dimensional printed scaffolds with gelatin and platelets enhance in vitro preosteoblast growth behavior and the sustained-release effect of growth factors. Chin. Med. J. Engl. 129, 2576–2581. doi:10.4103/0366-6999.192770
Keywords: three-dimensional printing, low-temperature deposition manufacturing, rapid prototyping manufacturing, bone tissue engineering, scaffold
Citation: Sun T, Wang J, Huang H, Liu X, Zhang J, Zhang W, Wang H and Li Z (2023) Low-temperature deposition manufacturing technology: a novel 3D printing method for bone scaffolds. Front. Bioeng. Biotechnol. 11:1222102. doi: 10.3389/fbioe.2023.1222102
Received: 13 May 2023; Accepted: 01 August 2023;
Published: 09 August 2023.
Edited by:
Antonella Motta, University of Trento, ItalyReviewed by:
Valentin Mateev, Technical University, Sofia, BulgariaFrancesco Baino, Polytechnic University of Turin, Italy
Copyright © 2023 Sun, Wang, Huang, Liu, Zhang, Zhang, Wang and Li. This is an open-access article distributed under the terms of the Creative Commons Attribution License (CC BY). The use, distribution or reproduction in other forums is permitted, provided the original author(s) and the copyright owner(s) are credited and that the original publication in this journal is cited, in accordance with accepted academic practice. No use, distribution or reproduction is permitted which does not comply with these terms.
*Correspondence: Honghua Wang, d2FuZ2hoMjJAZGljcC5hYy5jbg==; Zhonghai Li, bGl6aG9uZ2hhaXNwaW5lQDEyNi5jb20=
†These authors have contributed equally to this work and share first authorship