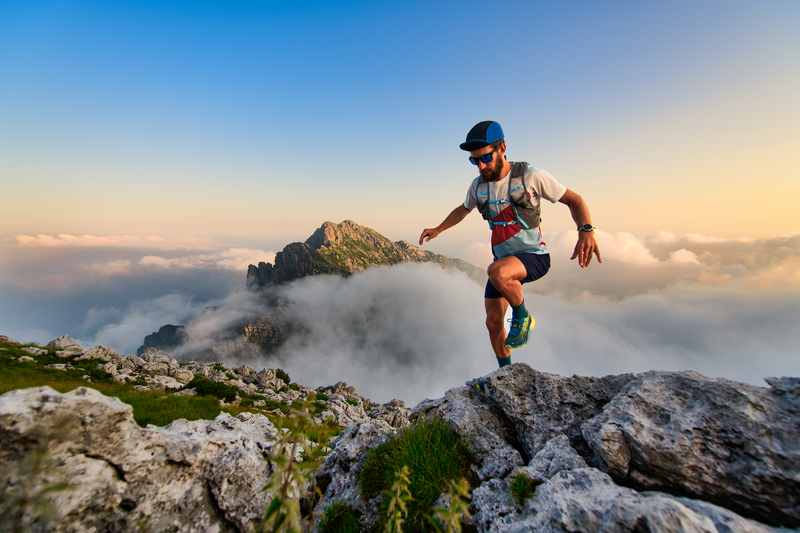
95% of researchers rate our articles as excellent or good
Learn more about the work of our research integrity team to safeguard the quality of each article we publish.
Find out more
MINI REVIEW article
Front. Bioeng. Biotechnol. , 30 May 2023
Sec. Biomaterials
Volume 11 - 2023 | https://doi.org/10.3389/fbioe.2023.1217067
This article is part of the Research Topic Engineered Biomimetic Micro/Nano-Materials for Tissue Regeneration View all 11 articles
In severe or complex cases of peripheral nerve injuries, autologous nerve grafts are the gold standard yielding promising results, but limited availability and donor site morbidity are some of its disadvantages. Although biological or synthetic substitutes are commonly used, clinical outcomes are inconsistent. Biomimetic alternatives derived from allogenic or xenogenic sources offer an attractive off-the-shelf supply, and the key to successful peripheral nerve regeneration focuses on an effective decellularization process. In addition to chemical and enzymatic decellularization protocols, physical processes might offer identical efficiency. In this comprehensive minireview, we summarize recent advances in the physical methods for decellularized nerve xenograft, focusing on the effects of cellular debris clearance and stability of the native architecture of a xenograft. Furthermore, we compare and summarize the advantages and disadvantages, indicating the future challenges and opportunities in developing multidisciplinary processes for decellularized nerve xenograft.
The current gold standard for peripheral nerve repair in segmental defects is autologous nerve grafting (Kalomiri et al., 1994). However, several limitations hamper the clinical practice, such as limited supply, donor site morbidity, and size discrepancy (Hu et al., 2009). Alternative autologous nerve substitutes, such as autologous veins, muscles, and tendons have been utilized with variable outcomes (Ray et al., 2011). Because of the abovementioned drawbacks, tissue engineered nerve grafts (TENG) were developed, aiming to provide a biomimetic scaffold for peripheral nerve regeneration (Houschyar et al., 2016). Although the Food and Drug Administration had approved several off-the-shelf synthetic nerve conduits on the market, but most of them are limited to nerve gaps <3 cm or <0.5 cm in small or large diameter nerves, respectively (de Ruiter et al., 2009). In addition, a recent review does not support the use of currently available TENG over standard nerve repair (Thomson et al., 2022). To achieve a better outcome in longer nerve gaps, nerve allografts are harvested and processed under a commercial decellularization process. Avance®, a mature commercial product which is produced by processing human nerve tissue with a combination of detergent decellularization, chondroitinase CSPG degradation, and gamma-irradiation sterilization, has clinical evidence to overcome up to 70 mm nerve gap in sensory, mixed, and motor nerve repair (Gunn et al., 2010; Safa et al., 2020). However, limited donor sources, high costs, low temperature preservation, and potential immune rejection still remain as clinical concerns for its wide usage.
Xenotransplantation was developed due to the unlimited availability of sources. In general, fresh xenografts elicit an immune response that causes graft rejection. The presence of non-self-antigenic epitopes triggers the activation of T- and B-lymphocytes. This, in turn, leads to the activation of an immune response mediated by antibodies, ultimately resulting in the rejection of these cells. (Fox et al., 2001; Vadori and Cozzi, 2014; Lopresti et al., 2015). Despite using immunosuppressive drugs, decellularization is an effective approach to utilize the xenogeneic and allogeneic tissues. The cellular components of tissues are the main cause of an adverse host response. To mitigate immune rejection, several decellularization techniques were developed to remove cellular components to reduce immunogenic reactions while preserving native scaffold or extracellular matrix (ECM) microstructure (Rana et al., 2017). Several recent studies reported that acellular nerve xenografts have similar effects on regeneration and immunocompatibility compared with acellular nerve allografts (Zhang et al., 2010; Huang et al., 2015). The concept of Decellularized extracellular matrix (dECM) is established as using various methods (physical, enzymatic, or chemical) to lyse cells and remove the intracellular components from a tissue while preserving the native extracellular components and the cues for cell proliferation and differentiation (Dahl et al., 2003; Lopresti et al., 2015; Kim et al., 2019). Although regeneration can occur in short nerve gaps regardless of an immune response, nerve growth was suppressed in long nerve gaps (Choi and Raisman 2003).
Zhang and Chen summarized several decellularization protocols along with their mechanisms and disadvantages, including physical, chemical, and enzymatic treatments in organ or tissue (Zhang et al., 2022). For nerve growth, a biomimetic neural scaffold is crucial, and thus, limited decellularization methods are applicable. Chemical-based decellularization was widely used in nerve tissues, but the major concern was the possible interruption of nerve growth by the residual chemical agents (Han et al., 2019). Enzymatic treatments provide high specificity for the removal of cellular components; however, they cannot be removed completely that might induce severe distortion of the ECM structure.
This minireview aims to focus on the latest five physical-based decellularization methods for peripheral nerve xenotransplantation (Figure 1). Table 1 summarizes the mechanisms, advantages, and disadvantages of the current physical processing methods.
FIGURE 1. The physical processing methods for tissue decellularization of nerve xenograft, including freezing and thawing cycle, sonication, immersion and agitation, perfusion and supercritical fluids.
This minireview aims to clarify the latest four physical-based decellularization methods for peripheral nerve xenotransplantation (Figure 1). Table 1 summarizes the mechanisms, advantages, and disadvantages of the current physical processing methods.
Freeze–thaw cycles refer to a repetitive freeze-drying process (Zhang et al., 2022). In nerve xenografts, the aim of cold preservation and freeze–thaw cycles is to destroy the nerve cell membranes by inducing the formation of intracellular ice crystals, thereby reducing the immunogenicity of nerve xenografts (Hare et al., 1993; Fox et al., 2005; Philips et al., 2018). Cold preservation and freeze–thaw cycles are easy to manipulate and an initial step in many decellularization protocols, as the protocols can be adjusted according to nerve length and diameter, depending on the laboratory preferences (Lasso and Deleyto, 2017).
However, studies have shown that the ultrastructure of nerves may be damaged by the freeze–thaw cycles, although the mechanical properties of the nerves are preserved (Osawa et al., 1990; Evans et al., 1998). A slower recovery in rats that received frozen grafts compared with those that received fresh autografts in a 2 cm median-nerve-gap rat model using Beagle dog acellular frozen xenografts indicate that freezing leads to a barren microenvironment for nerve regeneration (Accioli De Vaconcellos et al., 1999). Furthermore, the freeze–thaw process led to nerve xenograft rejection caused by the residual cells and debris (Lu et al., 2009). Despite the easy manipulation of freeze-thaw cycles, they might be responsible for damage to the nerve microstructure and a depleted environment for nerve regeneration.
Perfusion refers to the process of introducing circulating agents through the intrinsic vascular system of organs or tissues. This technique is typically used in larger, thicker tissues or whole organs (Goh et al., 2018). Only one study has reported the use of perfusion decellularization in peripheral nerve repair. Wüthrich and Lese applied perfusion decellularization to surgically procured vascularized porcine sciatic nerves (Wüthrich et al., 2020). A 3D microcomputed tomography imaging showed preserved vasculature and the ECM component. The dissected graft contained more external connective tissues, and the measurable growth factors were detectable at low levels. These results suggest that the biological activity of the graft may be retained and could promote nerve regeneration. An in vitro study revealed the potential for re-endothelialization, but no in vivo study has been conducted yet. These nerve scaffolds can be created for specific lesions and are becoming increasingly available, with the potential to overcome large nerve gaps.
Immersion and agitation refers to the process of submerging tissues into decellularization solutions with constant mechanical agitation (Crapo et al., 2011; Zhang et al., 2022). Compared with perfusion treatment, immersion and agitation is used in processing small, fragile and thin sections of tissues without innate vascular structures (Alshaikh et al., 2019). The efficiency of this method depends on different paraments including agitation intensity, decellularization agent and tissue dimension (Duisit et al., 2018). Immersion and agitation methods of tissue decellularization have been described for a wide variety of tissues, including peripheral nerves (Hudson et al., 2004; Karabekmez et al., 2009; Vasudevan et al., 2014). However, most of the decellularization solutions were chemical, detergent, or enzymatic solutions (Keane et al., 2015). To the best of our knowledge, only Vasudevan et al. reported an immersion and agitation method with detergent-free solution in peripheral nerve field (Vasudevan et al., 2014). In their design, the nerve grafts were immersed with detergent-free solution and were cultured at 37°C with 5% CO2 for 2 weeks under constant agitation, which was performed to initiate Wallerian degeneration in vitro to clear axonal and myelin debris inside the nerves. In the 3.5-cm sciatic nerve transection rat model, nerve regeneration was identical to that of detergent-processed grafts, while the functional nerve regeneration was only observed in detergent-free decellularized grafts at 12 weeks. This method did not significantly affect the ECM surface structure, collagen structure and integrity, mechanical strength, and GAG content, but may cause more damages to tissues due to the limited diffusion of chemical, detergent, or enzymatic decellularization solutions by agitation (Wilson et al., 2016; Simsa et al., 2019; Zhang et al., 2022).
Sonication is a method of rupturing the cell membrane by generating acoustic cavitation bubbles and inducing shear stress effect. It can assist in the penetration of agents by vibration as well as remove cellular debris. It was demonstrated that coupling freeze–thawing with sonication contributes to a cell-free and aseptic xenograft in a shorter time than applying freeze–thawing alone in a rabbit peripheral nerve model (Boriani et al., 2017). Based on these results, they further developed a new method of soaking the nerve tissues in decellularizing solutions, with combination of sonication and freeze–thaw cycles (Bolognesi et al., 2022). This new method was validated through histology and immunohistochemistry, showing its application to decellularized xenografts with similar or better results compared with the Hudson technique (Hudson et al., 2004). However, it was observed that sonication during chemical decellularization did not remove deoxyribonucleic acid (DNA) content but only cellular debris and myelin sheaths (Suss et al., 2022). So far, no study has utilized sonication as a single process for xenograft decellularization.
Supercritical fluids are fluids above their critical pressure and temperature that possess characteristics such as low viscosity and high diffusivity. Supercritical carbon dioxide (ScCO2) is an environmentally friendly solvent that is widely used in the field of biomedicine and biomaterials due to its nontoxicity, low cost, and superior disinfection and sterilization abilities (Subramaniam et al., 1997; Casali et al., 2018). It has a critical pressure 7.38 MPa and a critical temperature 31°C. ScCO2 has been shown to remove cells from the tissues while maintaining the ECM structure. However, the exact mechanism by which this occurs is unclear. The hypothesis that high pressure induces cell bursting, as claimed by Topuz et al., has been refuted (Isenschmid et al., 1995; White et al., 2006). It is hypothesized that ScCO2 might induce hypoxia, which has been validated by histological and morphological analyses after successful decellurization of bovine optic nerves using ScCO2 (Topuz et al., 2020). Wei et al. developed a porcine acellular nerve xenograft based on supercritical extraction technology and validated it in a 15-mm rat sciatic nerve model (Wei et al., 2022). A porous nerve basement membrane with a well-preserved 3D structure was observed. Low cytotoxicity was noted in vitro, leading to decreased immune response in vivo. The ScCO2 treatment group was found to be similar to the autologous nerves in terms of regenerated nerve quality, target muscle wet weight regain, and motor function recovery. Moreover, the hybrid detergent plus ScCO2 treatment demonstrated better outcomes in terms of decellularization and defatting compared to ScCO2 alone (Casali et al., 2018).
In recent decades, tissue engineering has been applied in the field of regenerative medicine to peripheral nerve repair, relying on the three main pillars: scaffolds, cells, and growth factors (Carvalho et al., 2019). In scaffolds, preserving the biomimetic microenvironment is essential, whereas in xenografts, the removal of cells is critical to prevent subsequent immune rejection. Gilpin and Yang et al. measured four aspects of the decellularized ECM to assess the quality of decellularization: removal of cells, elimination of genetic material, preservation of the protein content, and retention of the mechanical properties (Gilpin and Yang, 2017). Carpo et al. proposed specific criteria for assessing the efficacy of cell removal: the decellularized ECM must have the following: 1) less than 50 ng double-stranded DNA per mg ECM dry weight, 2) less than 200 bp DNA fragment length, and 3) no visible nuclear material by 4′,6-diamidino-2-phenylindole staining (Crapo et al., 2011).
Chemical and enzymatic approaches are the most widely applied methods for peripheral nerve decellularization, but the possible toxicity of the chemicals and destruction of the ECM proteins are major drawbacks of these methods. Physical treatments like modulating temperature or pressure have been effective, but it comes with limitations because of the natural architecture of nerves. Freeze–thawing is widely used and an important step in all decellularization protocols. However, debris retention and potential microstructure damage might restrict the in vivo therapeutic effects. Perfusion decellularization was developed to overcome long nerve gaps using vascularized porcine sciatic nerves, but the evidence of an in vivo study is lacking. Immersion and agitation decellularization is commonly used but generally with chemical, detergent, and/or enzymatic solutions. Sonication is integrated with other methods to assist in removing cellular debris. However, the clearance of DNA content is questionable. Supercritical fluids show promise with advantages of nontoxicity, low cost, superior disinfection and sterilization abilities, and can solely and effectively remove cells. Pure physical treatment has a limited but acceptable effect in decellularization compared with chemical and enzymatic approaches. Both immersion and agitation and supercritical fluids had shown that identical decellularization efficacy can be achieved as compared to chemical or enzymatic approaches. Further investigations are required to validate the in vivo therapeutic outcomes. With comprehensive understanding of the physical processing methods, multidisciplinary integration of different approaches are expected to elicit accumulative benefits, in terms of reducing immunogenicity and preserving the mechanical properties and microenvironment of native nerve tissue.
M-WH, S-HC, W-LT, K-SH, and T-CC wrote the manuscript, S-CL, JK, and Y-YH conceived the idea of the study, supervised, and revised the manuscript. All authors contributed to the article and approved the submitted version.
This study was supported in part by grants from the National Science and Technology Council (111-2311-B-006-001 and 111-2923-B-006-001-MY2 to Y-YH; 111-2314-B-006-095 to S-HC) and National Cheng Kung University Hospital (NCKUH-11109014 to Y-YH) in Taiwan.
The authors would like to express their sincere gratitude to Ko-Chung Yen and Dar-Jen Hsieh from ACRO biomedical company in Taiwan, for the explanation of terminology and concept to the decellularization process. The research was supported in part by Higher Education Sprout Project, Ministry of Education to the Headquarters of University Advancement at National Cheng Kung University (NCKU).
The authors declare that the research was conducted in the absence of any commercial or financial relationships that could be construed as a potential conflict of interest.
All claims expressed in this article are solely those of the authors and do not necessarily represent those of their affiliated organizations, or those of the publisher, the editors and the reviewers. Any product that may be evaluated in this article, or claim that may be made by its manufacturer, is not guaranteed or endorsed by the publisher.
Accioli De Vaconcellos, Z. A., Duchossoy, Y., Kassar-Duchossoy, L., and Mira, J. C. (1999). Experimental median nerve repair by fresh or frozen nerve autografts and xenografts. Ann. Chir. Main. Memb. Super. 18 (1), 74–84.
Alshaikh, A. B., Padma, A. M., Dehlin, M., Akouri, R., Song, M. J., Brannstrom, M., et al. (2019). Decellularization of the mouse ovary: Comparison of different scaffold generation protocols for future ovarian bioengineering. J. Ovarian Res. 12 (1), 58.
Bolognesi, F., Fazio, N., Boriani, F., Fabbri, V. P., Gravina, D., Pedrini, F. A., et al. (2022). Validation of a cleanroom compliant sonication-based decellularization technique: A new concept in nerve allograft production. Int. J. Mol. Sci. 23 (3).
Boriani, F., Fazio, N., Fotia, C., Savarino, L., Nicoli Aldini, N., Martini, L., et al. (2017). A novel technique for decellularization of allogenic nerves and in vivo study of their use for peripheral nerve reconstruction. J. Biomed. Mater Res. A 105 (8), 2228–2240.
Carvalho, C. R., Oliveira, J. M., and Reis, R. L. (2019). Modern trends for peripheral nerve repair and regeneration: Beyond the hollow nerve guidance conduit. Front. Bioeng. Biotechnol. 7, 337.
Casali, D. M., Handleton, R. M., Shazly, T., and Matthews, M. A. (2018). A novel supercritical CO 2 -based decellularization method for maintaining scaffold hydration and mechanical properties. J. Supercrit. Fluids 131, 72–81.
Crapo, P. M., Gilbert, T. W., and Badylak, S. F. (2011). An overview of tissue and whole organ decellularization processes. Biomaterials 32 (12), 3233–3243.
Dahl, S. L. M., Koh, J., Prabhakar, V., and Niklason, L. E. (2003). Decellularized native and engineered arterial scaffolds for transplantation. Cell Transpl. 12 (6), 659–666.
de Ruiter, G. C., Malessy, M. J., Yaszemski, M. J., Windebank, A. J., and Spinner, R. J. (2009). Designing ideal conduits for peripheral nerve repair. Neurosurg. Focus 26 (2), E5.
Duisit, J., Amiel, H., Orlando, G., Dedriche, A., Behets, C., Gianello, P., et al. (2018). Face graft scaffold production in a rat model. Plast. Reconstr. Surg. 141 (1), 95–103.
Evans, P. J., Mackinnon, S. E., Levi, A. D., Wade, J. A., Hunter, D. A., Nakao, Y., et al. (1998). Cold preserved nerve allografts: Changes in basement membrane, viability, immunogenicity, and regeneration. Muscle Nerve 21 (11), 1507–1522.
Fox, A., Mountford, J., Braakhuis, A., and Harrison, L. C. (2001). Innate and adaptive immune responses to nonvascular xenografts: Evidence that macrophages are direct effectors of xenograft rejection. J. Immunol. 166 (3), 2133–2140.
Fox, I. K., Jaramillo, A., Hunter, D. A., Rickman, S. R., Mohanakumar, T., and Mackinnon, S. E. (2005). Prolonged cold-preservation of nerve allografts. Muscle Nerve 31 (1), 59–69.
Gilpin, A., and Yang, Y. (2017). Decellularization strategies for regenerative medicine: From processing techniques to applications. Biomed. Res. Int. 2017, 9831534.
Goh, S. K., Bertera, S., Vaidya, V., Dumpe, S., Barner, S., Mathew, S., et al. (2018). Development of perfusion bioreactor for whole organ engineering - a culture system that enhances cellular engraftment, survival and phenotype of repopulated pancreas. Technology 6 (3-4), 118–134.
Gunn, S., Cosetti, M., and Roland, J. T. (2010). Processed allograft: Novel use in facial nerve repair after resection of a rare racial nerve paraganglioma. Laryngoscope 120 (4), S206.
Han, G. H., Peng, J., Liu, P., Ding, X., Wei, S., Lu, S., et al. (2019). Therapeutic strategies for peripheral nerve injury: Decellularized nerve conduits and schwann cell transplantation. Neural Regen. Res. 14 (8), 1343–1351.
Hare, G. M., Evans, P. J., Mackinnon, S. E., Nakao, Y., Midha, R., Wade, J. A., et al. (1993). Effect of cold preservation on lymphocyte migration into peripheral nerve allografts in sheep. Transplantation 56 (1), 154–162.
Hess, J. R., Brenner, M. J., Fox, I. K., Nichols, C. M., Myckatyn, T. M., Hunter, D. A., et al. (2007). Use of cold-preserved allografts seeded with autologous Schwann cells in the treatment of a long-gap peripheral nerve injury. Plast. Reconstr. Surg. 119 (1), 246–259.
Houschyar, K. S., Momeni, A., Pyles, M. N., Cha, J. Y., Maan, Z. N., Duscher, D., et al. (2016). "The role of current techniques and concepts in peripheral nerve repair." Plast. Surg. Int. 2016, 4175293.
Hu, X., Huang, J., Ye, Z., Xia, L., Li, M., Lv, B., et al. (2009). A novel scaffold with longitudinally oriented microchannels promotes peripheral nerve regeneration. Tissue Eng. Part A 15 (11), 3297–3308.
Huang, H., Xiao, H., Liu, H., Niu, Y., Yan, R., and Hu, M. (2015). A comparative study of acellular nerve xenografts and allografts in repairing rat facial nerve defects. Mol. Med. Rep. 12 (4), 6330–6336.
Hudson, T. W., Liu, S. Y., and Schmidt, C. E. (2004). Engineering an improved acellular nerve graft via optimized chemical processing. Tissue Eng. 10 (9-10), 1346–1358.
Ide, C. (1983). Nerve regeneration and schwann cell basal lamina: Observations of the long-term regeneration. Arch. Histol. Jpn. 46 (2), 243–257.
Ide, C., Tohyama, K., Yokota, R., Nitatori, T., and Onodera, S. (1983). Schwann cell basal lamina and nerve regeneration. Brain Res. 288 (1-2), 61–75.
Isenschmid, A., Marison, I. W., and von Stockar, U. (1995). The influence of pressure and temperature of compressed CO2 on the survival of yeast cells. J. Biotechnol. 39 (3), 229–237.
Jesuraj, N. J., Santosa, K. B., Macewan, M. R., Moore, A. M., Kasukurthi, R., Ray, W. Z., et al. (2014). Schwann cells seeded in acellular nerve grafts improve functional recovery. Muscle Nerve 49 (2), 267–276.
Kaizawa, Y., Kakinoki, R., Ikeguchi, R., Ohta, S., Noguchi, T., Takeuchi, H., et al. (2017). A nerve conduit containing a vascular bundle and implanted with bone marrow stromal cells and decellularized allogenic nerve matrix. Cell Transpl. 26 (2), 215–228.
Kalomiri, D. E., Soucacos, P. N., and Beris, A. E. (1994). Nerve grafting in peripheral nerve microsurgery of the upper extremity. Microsurgery 15 (7), 506–511.
Karabekmez, F. E., Duymaz, A., and Moran, S. L. (2009). Early clinical outcomes with the use of decellularized nerve allograft for repair of sensory defects within the hand. Hand (N Y) 4 (3), 245–249.
Keane, T. J., Swinehart, I. T., and Badylak, S. F. (2015). Methods of tissue decellularization used for preparation of biologic scaffolds and in vivo relevance. Methods 84, 25–34.
Kim, Y. S., Majid, M., Melchiorri, A. J., and Mikos, A. G. (2019). Applications of decellularized extracellular matrix in bone and cartilage tissue engineering. Bioeng. Transl. Med. 4 (1), 83–95.
Lasso, J. M., and Deleyto, E. (2017). Current status in peripheral nerve xenotransplantation. Xenotransplantation - New Insights.
Lopresti, S. T., and Brown, B. N. (2015). “Chapter 4 - host response to naturally derived biomaterials,” in Host response to biomaterials (Oxford: Academic Press), 53–79.
Lu, L. J., Sun, J. B., Liu, Z. G., Gong, X., Cui, J. L., and Sun, X. G. (2009). "Immune responses following mouse peripheral nerve xenotransplantation in rats." J. Biomed. Biotechnol. 2009, 412598.
Osawa, T., Tohyama, K., and Ide, C. (1990). Allogeneic nerve grafts in the rat, with special reference to the role of Schwann cell basal laminae in nerve regeneration. J. Neurocytol. 19 (6), 833–849.
Philips, C., Cornelissen, M., and Carriel, V. (2018). Evaluation methods as quality control in the generation of decellularized peripheral nerve allografts. J. Neural Eng. 15 (2), 021003.
Rana, D., Zreiqat, H., Benkirane-Jessel, N., Ramakrishna, S., and Ramalingam, M. (2017). Development of decellularized scaffolds for stem cell-driven tissue engineering. J. Tissue Eng. Regen. Med. 11 (4), 942–965.
Ray, W. Z., Kale, S. S., Kasukurthi, R., Papp, E. M., Johnson, P. J., Santosa, K. B., et al. (2011). Effect of cold nerve allograft preservation on antigen presentation and rejection. J. Neurosurg. 114 (1), 256–262.
Safa, B., Jain, S., Desai, M. J., Greenberg, J. A., Niacaris, T. R., Nydick, J. A., et al. (2020). Peripheral nerve repair throughout the body with processed nerve allografts: Results from a large multicenter study. Microsurgery 40 (5), 527–537.
Simsa, R., Vila, X. M., Salzer, E., Teuschl, A., Jenndahl, L., Bergh, N., et al. (2019). Effect of fluid dynamics on decellularization efficacy and mechanical properties of blood vessels. PLoS One 14 (8), 0220743.
Subramaniam, B., Rajewski, R. A., and Snavely, K. (1997). Pharmaceutical processing with supercritical carbon dioxide. J. Pharm. Sci. 86 (8), 885–890.
Suss, P. H., Ribeiro, V. S. T., Motooka, C. E., de Melo, L. C., and Tuon, F. F. (2022). Comparative study of decellularization techniques to obtain natural extracellular matrix scaffolds of human peripheral-nerve allografts. Cell Tissue Bank. 23 (3), 511–520.
Thomson, S. E., Ng, N. Y., Riehle, M. O., Kingham, P. J., Dahlin, L. B., Wiberg, M., et al. (2022). Bioengineered nerve conduits and wraps for peripheral nerve repair of the upper limb. Cochrane Database Syst. Rev. 12 (12), CD012574.
Topuz, B., Günal, G., Guler, S., and Aydin, H. M. (2020). Use of supercritical CO(2) in soft tissue decellularization. Methods Cell Biol. 157, 49–79.
Vadori, M., and Cozzi, E. (2014). Immunological challenges and therapies in xenotransplantation. Cold Spring Harb. Perspect. Med. 4 (4), a015578.
Vasudevan, S., Huang, J., Botterman, B., Matloub, H. S., Keefer, E., and Cheng, J. (2014). Detergent-free decellularized nerve grafts for long-gap peripheral nerve reconstruction. Plast. Reconstr. Surg. Glob. Open 2 (8), 201.
Wei, S., Hu, Q., Ma, J., Dai, X., Sun, Y., Han, G., et al. (2022). Acellular nerve xenografts based on supercritical extraction technology for repairing long-distance sciatic nerve defects in rats. Bioact. Mater. 18, 300–320.
White, A., Burns, D., and Christensen, T. W. (2006). Effective terminal sterilization using supercritical carbon dioxide. J. Biotechnol. 123 (4), 504–515.
Wilson, S. L., Sidney, L. E., Dunphy, S. E., Dua, H. S., and Hopkinson, A. (2016). Corneal decellularization: A method of recycling unsuitable donor tissue for clinical translation? Curr. Eye Res. 41 (6), 769–782.
Wüthrich, T., Lese, I., Haberthür, D., Zubler, C., Hlushchuk, R., Hewer, E., et al. (2020). Development of vascularized nerve scaffold using perfusion-decellularization and recellularization. Mater. Sci. Eng. C 117, 111311.
Zhang, X., Chen, X., Hong, H., Hu, R., Liu, J., and Liu, C. (2022). Decellularized extracellular matrix scaffolds: Recent trends and emerging strategies in tissue engineering. Bioact. Mater 10, 15–31.
Keywords: decellularized nerve xenograft, peripheral nerve regeneration, physical processing, freeze-thaw, perfusion, immersion and agitation, sonication, supercritical fluids
Citation: Hsu M-W, Chen S-H, Tseng W-L, Hung K-S, Chung T-C, Lin S-C, Koo J and Hsueh Y-Y (2023) Physical processing for decellularized nerve xenograft in peripheral nerve regeneration. Front. Bioeng. Biotechnol. 11:1217067. doi: 10.3389/fbioe.2023.1217067
Received: 04 May 2023; Accepted: 23 May 2023;
Published: 30 May 2023.
Edited by:
Yawei Du, Shanghai Jiao Tong University, ChinaReviewed by:
Junjie Li, Soochow University, ChinaCopyright © 2023 Hsu, Chen, Tseng, Hung, Chung, Lin, Koo and Hsueh. This is an open-access article distributed under the terms of the Creative Commons Attribution License (CC BY). The use, distribution or reproduction in other forums is permitted, provided the original author(s) and the copyright owner(s) are credited and that the original publication in this journal is cited, in accordance with accepted academic practice. No use, distribution or reproduction is permitted which does not comply with these terms.
*Correspondence: Yuan-Yu Hsueh, eXloc3VlaEBtYWlsLm5ja3UuZWR1LnR3
Disclaimer: All claims expressed in this article are solely those of the authors and do not necessarily represent those of their affiliated organizations, or those of the publisher, the editors and the reviewers. Any product that may be evaluated in this article or claim that may be made by its manufacturer is not guaranteed or endorsed by the publisher.
Research integrity at Frontiers
Learn more about the work of our research integrity team to safeguard the quality of each article we publish.