- 1Kunming Medical University, Kunming Yunnan, China
- 2Department of Pain Treatment, The First People’s Hospital of Yunnan Province, Kunming Yunnan, China
- 3Department of Sports Medicine, the First Affiliated Hospital of Kunming Medical University, Kunming Yunnan, China
Introduction: Independent augmentation technology based on reinforcing devices has been reported to signifi-cantly reduce the elongation behavior of graft and improve knee stability after anterior cruciate ligament reconstruction (ACLR). Using biodegradable devices could reduce the risk of severe inflammatory reactions due to particle accumulation from foreign bodies. Given the limitations of the mechanical properties of biodegradable materials, partially biodegradable composite devices may offer a compromise strategy.
Methods: Three types of partially absorbable core-sheath sutures, including low-absorbable cord (LA-C), medium-absorbable cord (MA-C) and high-absorbable cord (HA-C), were braided using unabsorbable ultra-high molecular weight polyethylene (UHMWPE) yarn and absorbable polydioxanone (PDO) monofil-ament bundle based on the desired configuration. The feasibility of these partially absorbable cords were verified by biomechanical testing, material degradation testing, and cell experiments, all performed in vitro.
Results: Reinforcement of an 8 mm graft with the cords decreased dynamic elongation by 24%–76%, was positively related to dynamic stiffness, and increased the failure load by 44%–105%, during which LA-C showed maximum enhancement. Human ligament-derived fibroblasts showed good proliferation and vitality on each cord over 2 weeks and aligned themselves in the direction of the fibers, especially the UHMWPE portion.
Discussion: This study supports the potential of partially degradable UHMWPE/PDO cords, particularly LA-C, for graft protection. Nervertheless, a higher proportion of biodegradable material results in lower stiffness, which may impair the protective and lead to mechanical instability during degradation.
1 Introduction
In the United States, it is reported that more than 100,000 ACLRs are performed annually (Montalvo et al., 2019), and primary grafts are autogenous hamstring tendon or bone-patellar tendon-bone complex (Ardern et al., 2011; Vaishya et al., 2015). However, in young and athletic individuals (Wiggins et al., 2016; Zbrojkiewicz et al., 2018), small diameter grafts (Magnussen et al., 2012; Mariscalco et al., 2013), graft ligamentalization (Ménétrey et al., 2008; Claes et al., 2011), accelerated rehabilitation (Beynnon et al., 2011; Culvenor et al., 2018), and accidental injury are all risk factors leading to postoperative graft relaxation or rupture. In short, the mismatch between the graft-loading capacity and load demand in the rehabilitation process leads to adverse results. Improving the mechanical properties of grafts through reinforcement technology may be the solution.
The Kennedy LAD, which appeared in 1980, was proved to significantly enhance the mechanical strength of the patellar tendon graft used for ACLR (Zbrojkiewicz et al., 2018) but was abandoned because of uncertainty regarding its clinical efficacy and complications such as joint effusion and synovitis (Dahlstedt et al., 1990; Batty et al., 2015)]. Although artificial LARS ligament has shown better efficacy and lower complications (Batty et al., 2015), it has also been questioned due to reports of inflammatory reactions from foreign bodies (Sinagra et al., 2018). Recently, reinforcement technology has received renewed attention due to the application of suture tape braided using UHMWPE fibers (Frangie et al., 2020). This high-strength belt could effectively share the graft load in dynamic stretching and play the role of a safety belt to reduce the risk of relaxation (Cook et al., 2017; Bachmaier et al., 2018; Soreide et al., 2019; Smith et al., 2020a; Noonan et al., 2020; Lai et al., 2021; Matava et al., 2021; Bachmaier et al., 2022). In clinical practice, suture tape can significantly improved knee joint function and promoted the return to regular activity after ACLR surgery (Kitchen et al., 2022; von Essen et al., 2022; Parkes et al., 2021; Bodendorfer et al., 2019).
Many studies have demonstrated the effectiveness and necessity of the reinforcement technique in ACLR (EAM et al., 2022). However, given the long-term retention of the reinforcement device in the joint, wear particles generated by non-degradable materials in the long term are potential risk factors for foreign body inflammation (Marieswaran et al., 2018; Sinagra et al., 2018). To achieve biodegradability, researchers have attempted to use materials with long degradation periods, such as polyurethane urea (Peterson et al., 2014), PDO (Buma et al., 2004), and poly-L-lactide/L-lactide-co-glycolide (Dürselen et al., 2003; Dürselen et al., 2006) to manufacture biodegradable reinforcement devices for ACLR. However, negative results and in vivo failure of these devices have been reported (Buma et al., 2004; Dürselen et al., 2006). Therefore, such biodegradable devices cannot be used as reinforcements for ACLR, mainly because of mechanical and structural instability caused by material degradation. In addition, no study has yet described in vitro biomechanical testing to confirm the exact augmentation effect of these devices on grafts.
Inspired by the strategy of composite materials in tissue engineering (Silva et al., 2020), a partially biodegradable reinforcing device may be a solution to balance biodegradability and mechanical requirements. Theoretically, the higher the degradable proportion in the device, the less amount of material is accumulated in vivo, while providing sufficient graft protection. In this study, UHMWPE yarn with superior mechanical strength (Savage et al., 2013) and PDO monofilament with a suitabledegradation period of 6 months (REA et al., 2018) were used to manufacture reinforcing cords with different material configurations. In vitro biomechanical and degradation testing and in vitro cell testing were performed to verify the feasibility of the partially biodegradable reinforcing cord.
2 Materials and methods
2.1 Manufacture of sutures and reinforcing cords
The core-sheath sutures were braided out of PDO bundle (3 monofilaments, USP 7–0 monofilament, Samyong, Korea) and UHMWPE yarn (100 denier, Honeywell, New Jersey, United States ) by a custom 16 spindle braiding machine (Figure 1A), the material configurations of each suture were shown in Table 1. UA-S consisted of UHMWPE sheath and UHMWPE core (Figure 1B), LA-S consisted of UHMWPE sheath and PDO core (Figure 1C), MA-S consisted of UHMWPE/PDO sheath and UHMWPE core (Figure 1D), HA-S consisted of UHMWPE/PDO sheath and PDO core (Figure 1E). As shown in Figure 1F, each single suture was further crocheted into a chain stitch cord by a custom knitting machine. Four reinforcing cords were obtained:, namely, UA-C, LA-C, MA-C, and HA-C. Uniform manufacturing parameters were used between groups in the above two processes.
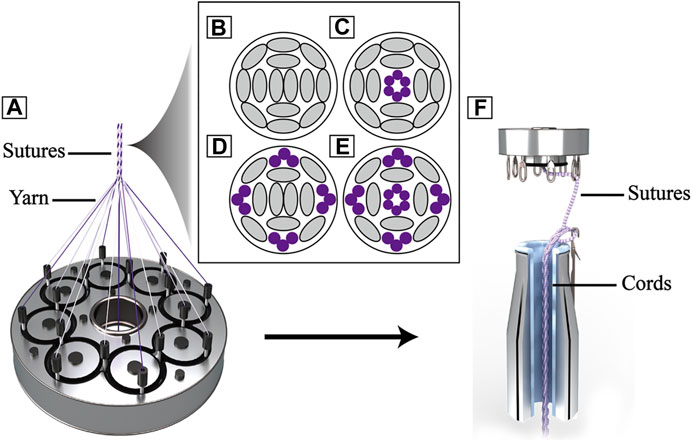
FIGURE 1. Schematic image of reinforcing cords: (A). Schematic of sheath-core sutures braiding; (B), (C), (D), (E) Section schematic of sutures (B): UA-S, (C) LA-S, (D) MA-S, (E) HA-S), grey oval represent UHMWPE yarn, purple circle represent PDO monofilament; (F). Schematic of reinforcing cords weaving.
2.2 Characterization of sutures and cords
Photos of each suture and cord (n = 3) were taken using a depth-of-field microscope (Leica DVM6, Weztla, Germany). The diameter and braiding angle of the sutures and the width and pitch of the cords were measured by ImageJ software (National Institute of Health, Bethesda, MD, United States ), randomly selecting three positions of each photo for measurement and calculating the mean value. The mechanical properties of the sutures and cords (n = 3) were tested by a dynamic machine equipped with 20 kN mechanical units (Instron 8,872, Boston, United States ). Bypassing the suture or cord through the suspension shaft of the upper and lower clamp and tying four half-hitch knots, a loop of 10 cm in length was formed, which was stretched to failure at a speed of 50 mm/min. Data were recorded by Wavematrix software (Instron, Boston, United States ) with a sampling rate of 500 Hz, and stiffness was calculated in the linear region.
2.3 Biomechanical testing
2.3.1 Graft preparation
Bovine flexor tendons from the hind limbs of adult bovine were selected as grafts for their mechanical and structural properties as they are similar to human hamstring tendons (Donahue et al., 2001). A single tendon was cut to 220 mm in length and trimmed parallel to the direction of collagen fibers until it could be folded into a tripled graft that was 70 mm in length and 8 mm in diameter. Each end of a tendon was passed through an adjustable loop (TightRope RT, Arthrex, Naples, United States ) and folded into a tripled graft. A rip-stop suture was performed with 2# Ethibond suture (Ethicon, New Brunswick, United States ) 5 mm away from both ends (Yoo et al., 2019). The graft was fixed in a preparation station and tensioned with a load of 20 N, then whip-stitched three times at the last 15 mm of both ends. Another rip-stop suture was performed 10 mm away from both ends when the load was increased to 50 N. Grafts were kept moist throughout the preparation process, stored at −20 °C after preparation, and thawed at room temperature for 2 hours before mechanical testing.
2.3.2 Construct fixation and testing protocol
The custom cylindrical clamps contain two adjacent 35 mm long tunnels that can independently accommodate and secure the graft and reinforcing cords. Dynamic mechanical tests were performed on isolated cord groups, graft groups, and cord-reinforced graft groups (n = 3). The fixing process for the above construct was as follows: 1) For the isolated cord groups, the cord bypassed the suspension shaft in the tunnel exits of both clamps and tied four half-hitch knots to form a 100 mm loop; 2) For the graft group, the buttons connected at both ends of the graft were passed through both clamp tunnel exits sequentially and were then flipped. The graft was preloaded with a constant force of 20 N for 1 minute and dynamically stretched 20 times with an applied force between 20 and 80 N at 0.75 Hz (Pilia et al., 2015; Jisa et al., 2016) to simulate intraoperative operation. After completion, the inter-button distance was adjusted to 100 mm and manually pulled alternately to shorten the adjustable loops at both ends to reach a 50 N load; 3) For the cord-reinforced graft groups (Figure 2A), graft fixation followed the same procedure described above, and then the inter-button distance was adjusted to 102 mm. Cord fixation was performed first by bypassing the suspension shaft in the adjacent tunnel and tensioned to 20 N until four half-hitch knots were completed. The inter-button distance was adjusted to 100 mm to loosen the cord, which was then manually pulled alternately to shorten the adjustable loops at both ends to reach a 50 N load.
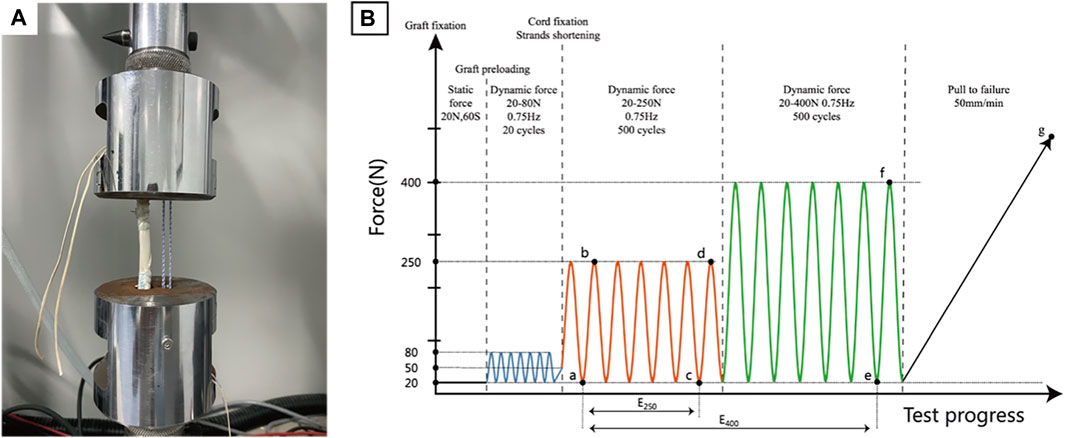
FIGURE 2. Biomechanical testing. (A). Fixation of cord reinforced graft; (B). Testing protocol with points of data analysis.
After fixation, all the above constructs underwent the same dynamic stretching procedure, and 1,000 load controlled tensile cycles were performed at 0.75 Hz. To simulate the in vivo loads of ACL during the early and late rehabilitation stages after ACLR (Toutoungi et al., 2000; Taylor et al., 2013), 250 N and 400 N were set as peak loads for the first 500 cycles and the last 500 cycles, respectively. The valley load level was 20 N. Finally, the construct was pulled to failure at 50 mm/min. The entire test protocol is shown in Figure 2B, and the load-displacement data were recorded with a sampling rate of 500 Hz using Wavematrix software (Instron, Boston, United States ).
2.3.3 Outcome data analysis
Dynamic elongation, dynamic stiffness, and failure load data were recorded and compared. Different letters are used to mark the position in Figure 2B and Δ is the symbol of variation, specifically as follows: valley displacement is the displacement under 20 N load in each cycle, and the valley displacement difference between the initial cycle of the 250 N module and the final cycles of both the 250 N and 400 N modules is dynamic elongation (E250 -Δac, E400 -Δae), namely, the value of plastic deformation or laxity. Dynamic stiffness was calculated in the initial cycle of the 250 N module (SI -Δab) and the final cycles of both load modules (S250 -Δcd, S400 -Δef) by valley and peak values, which could objectively reflect the loading situation of the construct. The failure load was recorded during the pull-to-failure process (LF-Δg), which determined the failure load capacity of the construct. To further evaluate the functional states of the construct, a native ACL reference model was established according to methods reported in the literature (Bachmaier et al., 2018; Noonan et al., 2020), and the displacement at a load of 20 N load was defined as the zero position to facilitate comparison. The load-displacement curves of the final 250 N and 400 N module cycles of the representative sample were input into the natural ACL function model for comparison to determine whether the construct was in a proper, loose, or restricted functional state.
2.4 In vitro degradation
Degradation testing was performed according to ISO standard 13,781 32. A cord sample consisted of an intermediate 20 cm woven section with a 5 cm suture section at both ends and 20 samples per group. After sterilization, the cord was immersed in 10 mL sterile PBS (pH 7.4) and sealed, maintaining the ratio of buffer volume in milliliters to sample mass in grams to within 30–40. Samples were placed on a shaker and swayed at 37 °C, PBS degradation solution was exchanged every 2 weeks, and pH was measured (n = 3).
2.4.1 Scanning electron microscopy
To assess any morphological changes in the material during degradation, a 1 cm suture was cut from the end of the cord. The sample was washed thrice with sterile distilled water and dried overnight in a fume cupboard. After gold coating, three areas of the sample were randomly selected under SEM (HITACHI, Regulus 8,220, Tokyo, Japan), and images were taken at magnifications of×100, ×300, and ×1,000 (n = 1).
2.4.2 Dynamic mechanical testing
At selected time points, the cord was removed from the degradation solution, vacuum dried, and then fixed to the cylindrical clamps described above and secured into a 10 cm loop. The following test procedure was the same as the biomechanical test protocol, including the recording method of dynamic stiffness and failure load (n = 3).
2.5 Cell compatibility
2.5.1 Human ligament-derived fibroblasts culture and transfection
Primary human ligament-derived fibroblasts (hFBs) (iCell, Shanghai, China) were maintained at 37 °C in 5% CO2 Modified Eagle Medium (Sigma-Aldrich, St. Louis, United States ) supplemented with 10% (v/v) fetal bovine serum, 1% (v/v) L-glutamine (Gibco, Carlsbad, United States ) and 1% (v/v) penicillin/streptomycin (Gibco, Carlsbad, United States ), and the media was replaced every third day. During the compatibility experiment, it was found that Dapi could be hardly removed from the cord after staining, so the nucleus could not be clearly observed through the fluorescent signal, and the green fluorescent protein (GFP) gene was transfected into hFBs by lentivirus to make cells spontaneously fluorescent. First, P2 hFBs were implanted in a T25 flask (Corning, Corning, United States ). When the cells had reached approximately 90% confluency overnight, the original medium was replaced with 5 mL media containing 1.2 × 106 TU/mL GFP-Lentivirus (MOI 20) (Genomeditech, Shanghai, China) and 5 μg/mL Polybrene (Solarbio, Beijing, China). The flasks were cultured under standard conditions (37 °C, 5% CO2) for 24 h and then replaced with the standard medium. After 72 h, green fluorescence expression of GFP-hFBs was observed under fluorescent microscope (Nikon, TE 2000, Tokyo, Japan) and cultured in media containing 1 μg/mL Puromycin for further screening.
2.5.2 Cell seeding
The cord was cut into 1 cm sections and placed on a 24-well plate (Corning, Corning, United States ). All cords were sterilized using 70% ethanol for 2 hours. P4-6 hFBs and GFP-hFBs were used for seeding. After counting, a high concentration cell suspension was prepared, and 30 ul (1.5 × 105) was dropped on each sample, which was then placed in a 5% CO2, 37 °C for 2 h to allow cells to attach. Then, the cords were transferred to a fresh 24-well plate, and the gradient number of cells (1 × 104, 2.5 × 104, 5 × 104, 7.5 × 104) were directly implanted into wells for cell attachment analysis and proliferation comparison, then 500 μL media was added to each well.
2.5.3 CCK-8 assay
Cell proliferation was assessed by CCK-8 at selected time points. Cords with hFBs were transferred to a fresh 24-well plate, and media were aspirated from wells implanted with gradient numbers of cells. The wells were rinsed thrice with PBS before adding 500 μL medium containing 10% (v/v) CCK-8 reagent (Dojindo, Kyushu Island, Japan) (n = 3). After 2 h of incubation under standard conditions (37 °C, 5% CO2), 100 µL of CCK-8 medium samples from each well were transferred to 96-well plates (Corning, Corning, United States ) for analysis. The optical density value at 450 nm was measured using a microplate reader (Biorad, Hercules, United States ). The remaining CCK-8 media was removed and replaced with fresh standard media.
2.5.4 Scanning electron microscopy
To assess cell distribution and morphology, samples were observed via SEM. Briefly, cords with hFBs were fixed in 2.5% v/v glutaraldehyde solution for 24 h at room temperature. After being rinsed thrice in PBS, the samples were dehydrated sequentially in a series of graded ethanol concentrations (40%, 50%, 70%, 90%, 100%, v/v) for 10 min each step. Samples were further dehydrated in tertiary butanol for 2 h and vacuum dried overnight. Three areas were randomly observed and photographed on each sample at magnifications of×100, ×500, and ×1,000 after gold-coating (n = 1).
2.5.5 Live/dead assay
Cell viability was assessed by live/dead assay. In brief, because GFP-hFBs already have a green fluorescence signal, samples were incubated in a medium containing only 4.5 μmol/L propidium iodide (Dojindo, Kyushu Island, Japan) for 10 min to stain dead cells. After washing twice in PBS, green and red fluorescence was visualized using confocal microscopy (Nikon T2i, Tokyo, Japan), and a specific thickness layer scan was performed (n = 1). The fluorescence signal synthesis was performed using NIS-Elements Viewer software (Nikon, Tokyo, Japan).
2.6 Statistical analysis
The statistical analysis was performed using GraphPad Prism software version 9 (GraphPad Software, San Diego, United States ). Data in graphs were expressed as mean ± standard mean error. t-Tests and standard ANOVA with Tukey’s multiple comparisons testing were used to examine statistical differences between groups. Results were considered significant for p < 0.05.
3 Results
3.1 Structural and mechanical characteristics of sutures and cords
Four groups of sutures were braided according to different core-sheath material configurations. UA-S was a complete UHMWPE suture (Figure 3A), and UHMWPE/PDO sutures included LA-S, MA-S, and HA-S (Figures 3B–D). Every single suture was crocheted into the corresponding cord (Figures 3E–H). UA-C served as the control group, whereas the UHMWPE/PDO cords were divided into LA-C, MA-C, and HA-C. As shown in Table 2, similar braid angle and pitch were measured in each suture and cord, respectively. Sutures and cords with UHMWPE/PDO sheath showed larger diameter and width, respectively. For sutures, the stiffness was in the 62–142 N/mm range (HA-S > LA-S > MA-S > HA-S) (Figure 4A), whereas the failure loads exceeded 348 N and showed no significant intergroup difference (Figure 4C). For cords, stiffness showed a similar gradient to that of sutures in the 47–151 N/mm range (HA-C > LA-C > MA-C > HA-C) (Figure 4B). The failure load of MA-C (671 N) was significantly lower than those of the other three groups, which exceeded 891 N (Figure 4D).
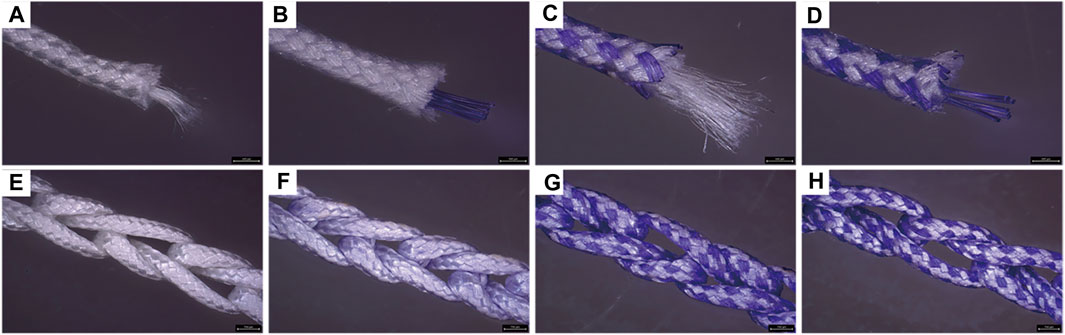
FIGURE 3. Macroscopic picture of sutures and cords. A, B, C, (D) Suture groups (A: UA-S, (B) LA-S, (C) MA-S, (D) HA-S); E, F, G, (H) Cord groups (E: UA-C, (F) LA-C, (G) MA-C, (H) HA-C), Scale bars = 750 μm.
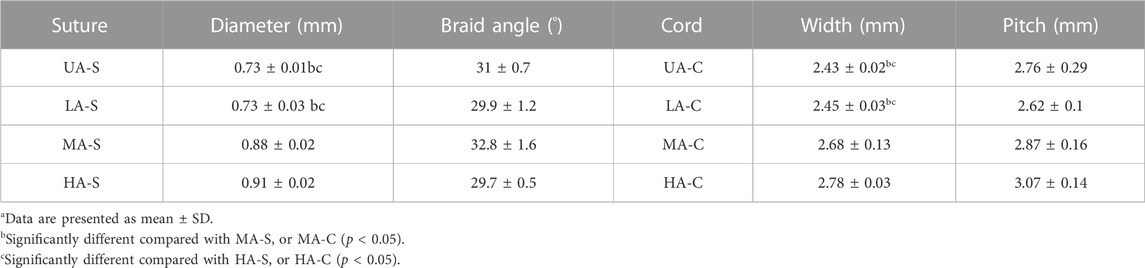
TABLE 2. Structural data of each suture and reinforcing corda.
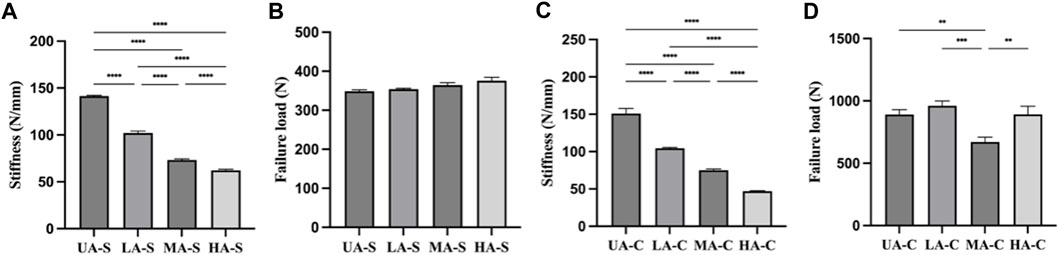
FIGURE 4. Mechanical properties of each sutures and cords: (A). Stiffness of suture; (B). Failure load of suture; (C). Stiffness of cord; (D). Failure load of cord (n = 3) (****p < 0.0001, ***p < 0.001, **p < 0.01).
3.2 Biomechanical testing
Test data for the graft group and each isolated cord group are shown in Table 3. The dynamic elongations (E400) of the three PDO/UHMWPE cords were significantly lower than that of the graft but higher than that of UA-C after the 400 N load module. Dynamic stiffness comprises three representative stiffness data during cyclic stretching (SI, S250, S400), representing the overall stiffness level of the structure. The dynamic stiffness of UA-C was similar to that of the graft, and those of the other three cords were lower than the dynamic stiffness of UA-C to varying degrees. The failure load (LF) of MA-C was significantly lower than that of other cords and graft groups.
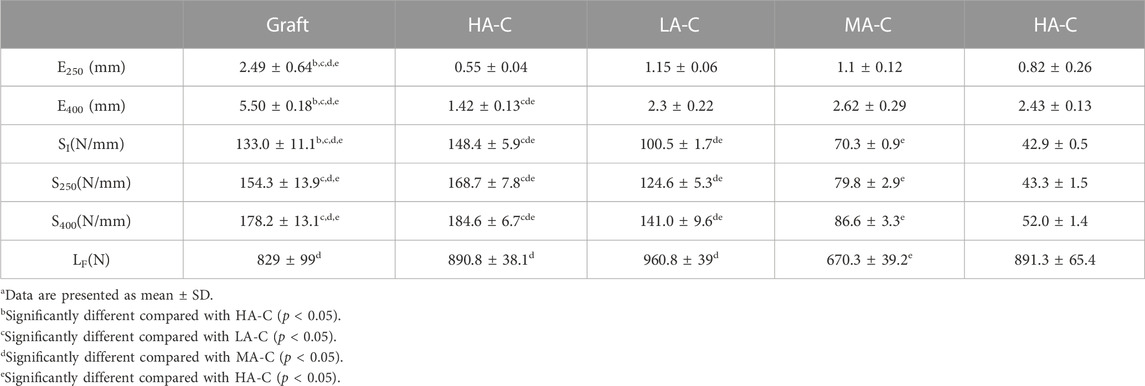
TABLE 3. Biomechanical Behavior of cord Specimensa.
Test data for graft with or without reinforcing cord are shown in Table 4. Compared with the graft group on dynamic elongation, the UA-C, LA-C, MA-C, and HA-C reinforced groups revealed a 69%, 39%, 19%, and 14% decrease in E250, and a 76%, 54%, 38%, and 24% decrease in E400, respectively. Compared to the graft group on dynamic stiffness, the UA-C reinforced group exhibited a 39% increase in SI and a 24% increase in S400. The increased dynamic stiffness in the PDO/UHMWPE cords reinforced groups was less than 7%. However, the UA-C reinforced group had a 17% decrease in S400. Compared to the graft group, the UA-C, LA-C, MA-C, and HA-C reinforced groups showed a 76%, 105%, 60%, and 44% increase in the LF value compared to the graft group, respectively.
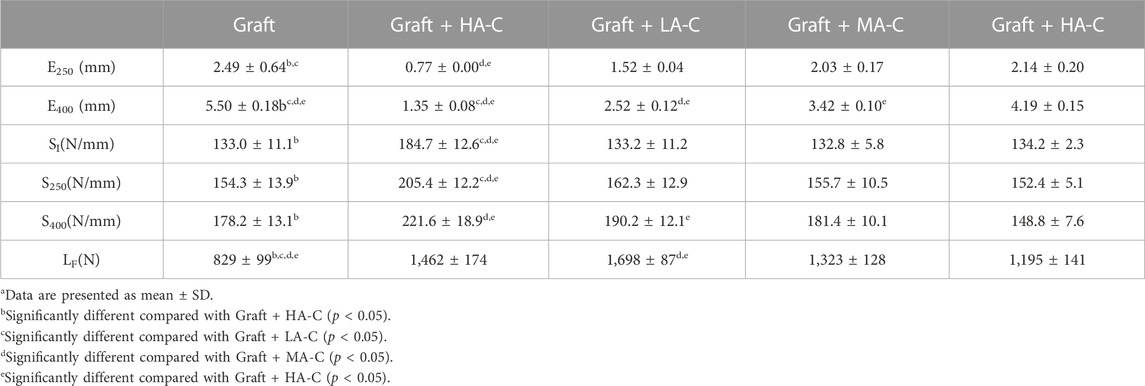
TABLE 4. Biomechanical behavior of graft and Graft + cord specimensa.
The last cycle curves of the 250 N load module from a representative test sample of the graft group and cord reinforced groups were within the defined native ACL function zone (Figure 5A). However, only the UA-C and LA-C reinforced groups were still within this zone after 400 N load module, while the graft group revealed a completely loose state. (Figure 5B).
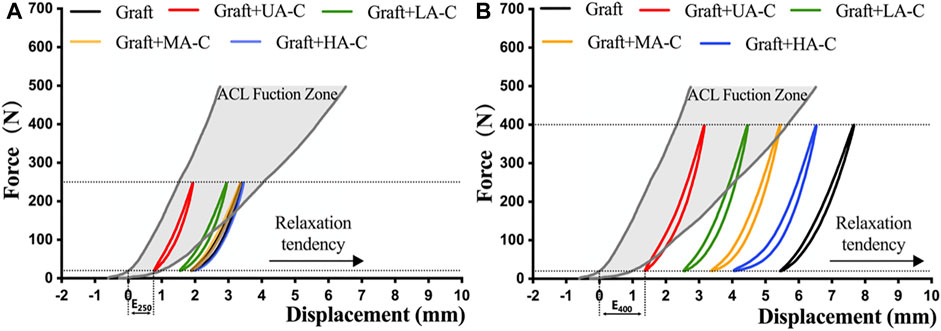
FIGURE 5. Function evaluation of construct after dynamic loading. A and B showing the load-displacement curve of construct at the end of 250 N and 400 N load block respectively, dynamic elongation (E250, E400) and the position relationship between the curve and the native ACL function zone indicate the functional situation of construct.
3.3 Degradation testing
PDO hydrolysate is acidic (Abhari et al., 2017). The degradation solution of three UHMWPE/PDO cords showed a decreasing PH value during the 16-week degradation period, particularly from weeks 8–10, and the degree of reduction was proportional to the ratio of the degradable material. However, the UA-C group remained essentially unchanged. (Figure 6A).
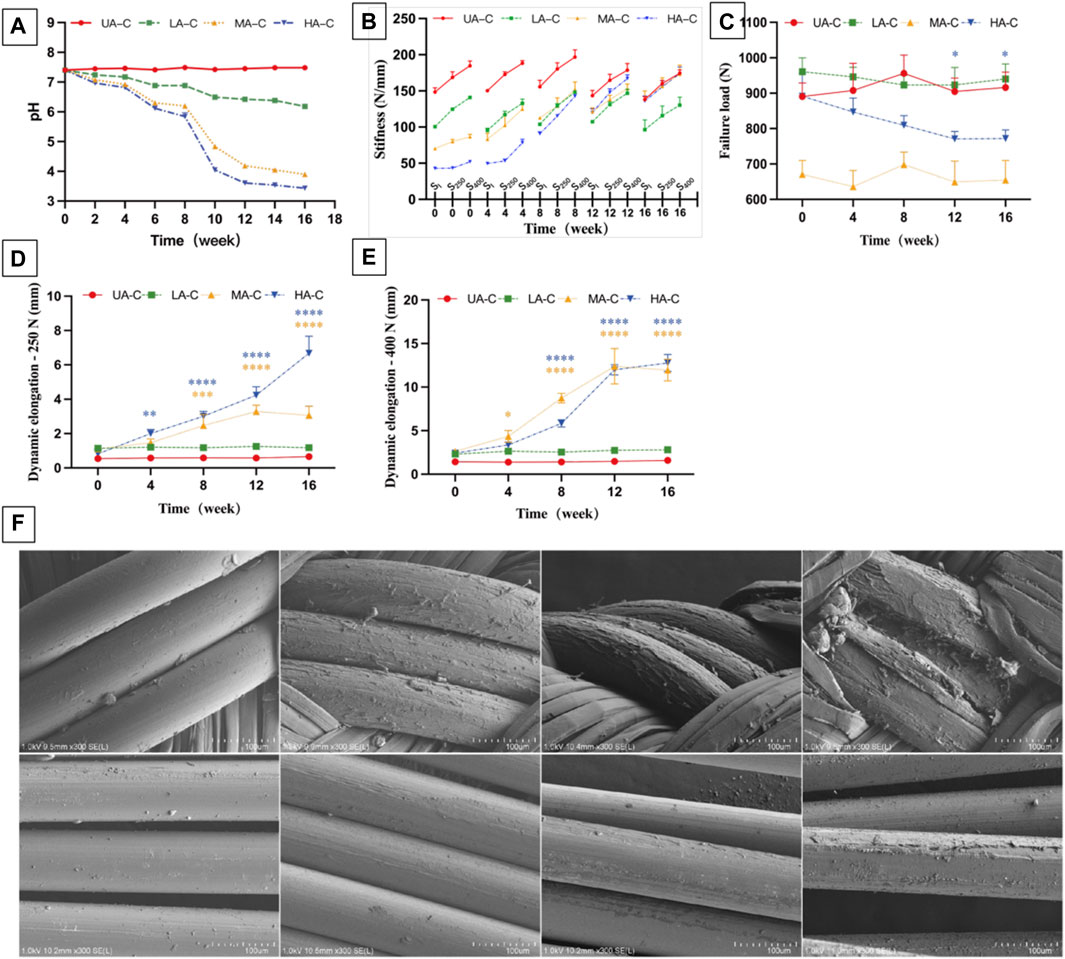
FIGURE 6. Degradation of cord over 16 weeks. (A). PH value of the degradation solution; (B). Dynamic stiffness of cord at different degradation points, SI: stiffness of initial cycle of 250 N load block, S250 and S400: stiffness of last cycle of 250 N and 400 N load block (n = 3); (C). Failure load of cord at different degradation points; (D). Dynamic elongation of cords at different degradation points after 250 N load block (E250); (E). Dynamic elongation of cords at different degradation points after 400 N load block (E400); (F). SEM images of HA-C at different degradation points over 4 moth, Scale bars = 100μm; t-Tests was conducted on dynamic stiffness (S400), failure load and dynamic elongation (E250, E400) between 0w and each degradation point for each group (n = 3), ****p < 0.0001, ***p < 0.001, **p < 0.01, *p < 0.05, different colors represent the corresponding groups.
During degradation, the dynamic stiffness of MA-C and HA-C gradually increased and was almost similar to that of UA-C at the end of the experiment (Figure 6B). At week 16, the E250 of MA-C and HA-C increased to 3.06 ± 0.53 mm, and 6.68 ± 1 mm respectively (Figure 6D), and E400 increased to 11.97 ± 1.26 mm and 12.77 ± 0.98 mm (Figure 6E), while the dynamic stiffness and dynamic elongation of UA-C and LA-C remained essentially stable (Figures 6B,D,E). At the end of the experiment, the LF of UA-C decreased slightly, but the other groups showed no significant changes (Figure 6C).
The degradation process of PDO monofilaments in cords is exhibited by the SEM image of HA-C (Figure 6F). The surface of the PDO monofilaments in the sheath gradually changed from smooth to rough and finally cracked at 16 weeks. However, the situation of the core PDO was better, and at 16 weeks, only slight exfoliation occurred. The degradation process of PDO monofilaments at the corresponding parts of LA-C and MA-C was essentially the same as HA-C, and the UHMWPE fiber of all cords had no noticeable change, which is not shown in the picture.
3.4 Cell compatibility
The attachment rate of hFBs to the cord was about 18%–28%, with no significant difference between groups (Figure 7A). Setting the 5 × 104 hFBs implanted wells as control, and the cell proliferation fold of four cord groups showed no significant difference with the control group over 14 days (Figure 7B). On day 7, cells on the UHMWPE yarn distributed in the inter-fiber space and aligned with the fiber direction. Cells on the PDO monofilament tended to wrap around the larger fibers and bridge larger gaps (Figure 7C). The cells proliferated significantly and almost covered the surface of the cords on day 14 and aligned further (Figure 7C).
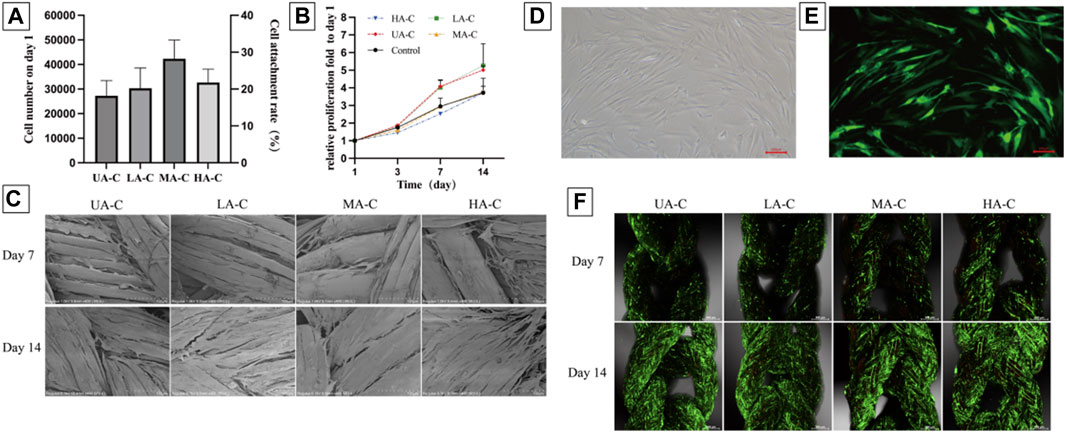
FIGURE 7. Cell attachment, proliferation and vitality on cords, (A). Numbers of cells attached to the materials 24 h after seeding and cell seeding efficiency (n = 3); (B). Relative cell growth on the cords over 14 days (n = 3); (C). SEM images showing cell morphology and spreading on the cords at day 7 and 14 after seeding, scale bars = 100 μm; D-E. Fluorescence images of GFP-hFBs after transfection, scale bars = 100 μm; (F). PI staining showed the vitality of GFP-hFBs on the cord surface at day 7 and 14 after seeding, green fluorescence representing GFP-hFBs and red fluorescence representing dead cells, scale bars = 500 μm.
Green fluorescence of GFP-hFBs can be observed under a fluorescence microscope (Figures 7D,E). From day 7–14, the density of GFP-hFBs increased significantly, and the cells took a long fusiform shape and were densely distributed along the fiber direction on all cords. Few dead cells could be observed at both time points (Figure 7F). The results confirmed that the materials did not significantly affect cell viability.
4 Discussion
Independent suture tape reinforcement can effectively reduce the plastic elongation of graft constructs while enhancing ultimate failure strength (Cook et al., 2017; Bachmaier et al., 2018; Soreide et al., 2019; Smith et al., 2020a; Noonan et al., 2020; Lai et al., 2021; Matava et al., 2021; Bachmaier et al., 2022) and promote the restoration of joint stability and function (Kitchen et al., 2022; von Essen et al., 2022; Parkes et al., 2021; Bodendorfer et al., 2019). However, material debris accumulation may result in long-term foreign body inflammation (Marieswaran et al., 2018; Sinagra et al., 2018). Given the in vivo mechanical and structural instability of biodegradable devices (Dürselen et al., 2003; Buma et al., 2004; Dürselen et al., 2006), a partially degradable composite device could be a compromise solution to balance strength and biodegradability requirements. Both undegradable UHMWPE and biodegradable PDO are commonly used materials for manufacturing surgical sutures. Considering the excellent mechanical properties of the UHMWPE fiber and the suitable degradation period of the PDO monofilament, these two materials were selected to produce three types of core-sheath sutures with different biodegradable proportions according to the selected material configurations. The complete UHMWPE suture served as a control, and each group suture was further crocheted into a cord with the same parameters.
An increasing proportion of PDO would lead to a larger size and lower flexibility for the reinforcing cord; thus, only three available material configurations were selected, but the same manufacturing parameters still resulted in the same braid angle and pitch. Because of the large stiffness gap between the PDO monofilament and the UHMWPE fiber, the adjustment of the material configuration led to the stiffness gradient of the sutures. Stiffness was negatively correlated with the biodegradable ratio. However, sutures with a reduced content of UHMWPE yarn in the sutures can still maintained their failure load. When each suture was crocheted into the cord, the stiffness gradient and range remained unchanged. The failure load showed a more than two-fold increase, except in the case of MA-C (1.8-fold), which may be due to the cutting effect on the UHMWPE/PDO sheath by the UHMWPE core under a specific load (>600 N).
The concept of the reinforcement technique combines the reinforcing device and tendon graft to form a load-sharing system in ACLR. The goal is to reduce graft elongation, which decides the laxity degree of the knee. The mechanical properties of the 8 mm tendon graft used in this study are close to the data in the literature (Noonan et al., 2020). The dynamic stiffness of suture tape was significantly higher than that of the 8 mm graft (Bachmaier et al., 2018; Noonan et al., 2020; Bachmaier et al., 2022). In this study, the dynamic stiffness of UA-C and the graft was similar, and the UHMWPE/PDO cords had lower stiffness than the latter to varying degrees. In addition, a similar failure load and smaller dynamic elongation compared to those of the grafts indicate that the cords have a feasible safety belt function for cords.
Regarding biomechanical results, the reinforcement effects of UHMWPE/PDO cords on the graft were mainly reflected in the reduction of dynamic elongation and the increase of failure load. In addition, the dynamic stiffness of the structure was determined by both the graft and the cords. The UHMWPE/PDO reinforced group differed from the UA-C reinforced group that showed similar dynamic stiffness to the graft group in the test environment of this study, which indicated that the graft was the dominant stabilizer in this state. Finally, it is worth noting that the HA-C reinforced group showed lower stiffness at the end of cyclic stretching than the graft group, which may be due to its limited protective effect. HA-C becomes the dominant stabilizer when the graft reaches a specific elongation, and the stiffness of the construction converges on the cord.
In this study, load and displacement data of native ACLs during walking activities were used to establish a functional model to more intuitively evaluate whether the biomechanical behavior of reconstructed ACLs restores native ACL function. Compared with the 250 N load module, the cord showed a more prominent protective effect after the 400 N load module because tendency for laxity of the reinforced graft groups was significantly inhibited compared to the graft group, and the degree of significance was positively correlated with the cord stiffness. Finally, only UA-C and LA-C reinforced grafts remained in the functional area after dynamic tensile testing.
If ACLR was enhanced by a device containing degradable components, changes in mechanical properties and structure caused by material degradation should be considered. According to the pH value of the degradation solution and SEM images, a rapid degradation phase began at week 8. The UHMWPE fibers crushed PDO monofilaments hydrolyzed in the sheath during cyclic stretching, resulting in the elongation of the MA-C and HA-C. After a 250 N load module, MA-C elongation was less than HA-C. Nonetheless, this difference was not evident after applying a 400 N load module, and it appears that the UHMWPE core could limit cord elongation under a smaller load. The decreasing braiding angle and increasing weaving pitch during cord elongation led to greater dynamic stiffness. The dynamic stiffness of MA-C and HA-C gradually increased to match that of the UA-C from weeks 8–16, which was consistent with the time point of significant change in mechanical properties of different PDO devices after 9 weeks of in vitro degradation reported in the literature (Zilberman et al., 2005; Li et al., 2014; Loskot et al., 2022). In addition, the incorporation of a few UHMWPE yarns can also guarantee the mechanical strength of the device because even HA-C, with the highest PDO proportion, merely showed a slight decrease in the failure load at the end of the degradation period. However, the stiffer and longer cord still lost its protective effect. For ACLR, the ideal protection time is to last until graft ligamentization reaches the maturation phase, which begins 3 months after surgery in animal studies (Scheffler et al., 2008; Claes et al., 2011), and the mechanical strength of the graft will continue to increase during this phase (Goradia et al., 2000; Weiler et al., 2001; Buma et al., 2004). Research on the ligamentization process of human tendon grafts after surgery is more complex than animal experiments, which are generally thought to take longer (Falconiero et al., 1998; Sánchez et al., 2010). In this way, the mechanical properties and structural stability of UHMWPE/PDO cords are less durable except LA-C; hence, so materials with a longer degradation period may be needed to enhance the stability of the device when pursuing a higher biodegradable ratio.
In vivo studies have reported that UHMWPE (Smith et al., 2019; Smith et al., 2020b)and PDO (Buma et al., 2004; Dürselen et al., 2006) have good joint biocompatibility. However, given the close contact between reinforcing devices and tendon grafts undergoing continuous ligamentalization in joints, human ligament-derived fibroblasts were used for cytocompatibility testing. The low cell seeding rate was related to the hydrophobicity of the material and the gaps between fibers in the woven structures (Savić et al., 2021). Cell proliferation showed an approximately 3.7–5.3-fold increase in cell numbers over 2 weeks, while well cell vitality was observed. UHMWPE yarn with a smaller fiber diameter and inter-fiber gap showed better guidance and support for cell morphology and arrangement. It has been found in tissue engineering studies that aligned morphological features of fibers play an important role in the induction and maintenance of fibroblast morphology and phenotype (Olvera et al., 2017). Hence, the gene expression profile of cells is worth further research in this type of study.
This study demonstrated the feasibilityof a partially biodegradable reinforcing device for assisted ACLR through physical and biological assessments. A composite material scheme was adopted, and four types of cords were manufactured by a two-step process according to the planned material configuration and parameters, namely, one UHMWPE cord and three UHMWPE/PDO cords. The proportion of PDO in the cord is inversely related to its dynamic stiffness, while the latter is positively related to the reduction of graft elongation. Therefore, the stiffer cord provided better protection against elongation. During degradation, all the cords maintained their failure load. Only LA-C showed the same stable dynamic stiffness and elongation as that of UA-C, while material degradation in the sheath resulted in the other two UHMWPE/PDO cords growing longer and stiffer during dynamic stretching. Cells showed good proliferation and vitality on all cords and were aligned parallel to the fiber.
5 Conclusion
Overall, this study supports the potential of partially degradable UHMWPE/PDO cords, particularly LA-C, in internal bracing technology for graft protection. Nevertheless, a higher content of biodegradable material results in lower stiffness may impair the protective effect and lead to mechanical instability during early degradation.
Data availability statement
The original contributions presented in the study are included in the article/supplementary material, further inquiries can be directed to the corresponding author.
Ethics statement
The animal studies were approved by Animal Ethics Committee, Kunming Medical University. The studies were conducted in accordance with the local legislation and institutional requirements. Written informed consent was obtained from the owners for the participation of their animals in this study.
Author contributions
Conceptualization and design, YL; data statistics, GC; data collection, YY; statistical analysis, GW; writing- original draft preparation, FX; All authors contributed to the article and approved the submitted version.
Funding
Yunnan Province Major Science and Technology Special Plan Projects (No. 202102AA100015) Yunnan Province Clinical Center for Bone and Joint Diseases (No. ZX2019-03-04) Kunming Medical University joint project (No. 202301AY070001-078) Technological innovation talents of Yunnan Province (No. 202105AD160018).
Conflict of interest
The authors declare that the research was conducted in the absence of any commercial or financial relationships that could be construed as a potential conflict of interest.
Publisher’s note
All claims expressed in this article are solely those of the authors and do not necessarily represent those of their affiliated organizations, or those of the publisher, the editors and the reviewers. Any product that may be evaluated in this article, or claim that may be made by its manufacturer, is not guaranteed or endorsed by the publisher.
References
Abhari, R. E., Martins, J. A., Morris, H. L., Mouthuy, P. A., and Carr, A. (2017). Synthetic sutures: clinical evaluation and future developments. J. Biomater. Appl. 32 (3), 410–421. doi:10.1177/0885328217720641
Ardern, C. L., Webster, K. E., Taylor, N. F., and Feller, J. A. (2011). Return to sport following anterior cruciate ligament reconstruction surgery: A systematic review and meta-analysis of the state of play. Br. J. Sports Med. 45 (7), 596–606. doi:10.1136/bjsm.2010.076364
Bachmaier, S., Smith, P. A., Argintar, E. H., Chahla, J., Higgins, L. D., and Wijdicks, C. A. (2022). Independent suture augmentation with all-inside anterior cruciate ligament reconstruction reduces peak loads on soft-tissue graft. A biomechanical full-construct study. Arthroscopy 38 (1), 88–98. doi:10.1016/j.arthro.2021.09.032
Bachmaier, S., Smith, P. A., Bley, J., and Wijdicks, C. A. (2018). Independent suture tape reinforcement of small and standard diameter grafts for anterior cruciate ligament reconstruction: A biomechanical full construct model. Arthroscopy 34 (2), 490–499. doi:10.1016/j.arthro.2017.10.037
Batty, L. M., Norsworthy, C. J., Lash, N. J., Wasiak, J., Richmond, A. K., and Feller, J. A. (2015). Synthetic devices for reconstructive surgery of the cruciate ligaments: A systematic review. Arthroscopy 31 (5), 957–968. doi:10.1016/j.arthro.2014.11.032
Beynnon, B. D., Johnson, R. J., Naud, S., Fleming, B. C., Abate, J. A., Brattbakk, B., et al. (2011). Accelerated versus nonaccelerated rehabilitation after anterior cruciate ligament reconstruction: A prospective, randomized, double-blind investigation evaluating knee joint laxity using roentgen stereophotogrammetric analysis. Am. J. Sports Med. 39 (12), 2536–2548. doi:10.1177/0363546511422349
Bodendorfer, B. M., Michaelson, E. M., Shu, H. T., Apseloff, N. A., Spratt, J. D., Nolton, E. C., et al. (2019). Suture augmented versus standard anterior cruciate ligament reconstruction: A matched comparative analysis. Arthroscopy 35 (7), 2114–2122. doi:10.1016/j.arthro.2019.01.054
Buma, P., Kok, H. J., Blankevoort, L., Kuijpers, W., Huiskes, R., and Van Kampen, A. (2004). Augmentation in anterior cruciate ligament reconstruction-a histological and biomechanical study on goats. Int. Orthop. 28 (2), 91–96. doi:10.1007/s00264-003-0515-0
Claes, S., Verdonk, P., Forsyth, R., and Bellemans, J. (2011). The "ligamentization" process in anterior cruciate ligament reconstruction: what happens to the human graft? A systematic review of the literature. Am. J. Sports Med. 39 (11), 2476–2483. doi:10.1177/0363546511402662
Cook, J. L., Smith, P., Stannard, J. P., Kuroki, K., Bozynski, C., Cook, C., et al. (2017). A canine arthroscopic anterior cruciate ligament reconstruction model for study of synthetic augmentation of tendon allografts. J. Knee Surg. 30 (7), 704–711. doi:10.1055/s-0036-1597618
Culvenor, A. G., Patterson, B. E., Guermazi, A., Morris, H. G., Whitehead, T. S., and Crossley, K. M. (2018). Accelerated return to sport after anterior cruciate ligament reconstruction and early knee osteoarthritis features at 1 Year: an exploratory study. Pm R. 10 (4), 349–356. doi:10.1016/j.pmrj.2017.09.005
Dahlstedt, L., Dalén, N., and Jonsson, U. (1990). Goretex prosthetic ligament vs. Kennedy ligament augmentation device in anterior cruciate ligament reconstruction. A prospective randomized 3-year follow-up of 41 cases. Acta Orthop. Scand. 61 (3), 217–224. doi:10.3109/17453679008993504
Donahue, T. L., Gregersen, C., Hull, M. L., and Howell, S. M. (2001). Comparison of viscoelastic, structural, and material properties of double-looped anterior cruciate ligament grafts made from bovine digital extensor and human hamstring tendons. J. Biomech. Eng. 123 (2), 162–169. doi:10.1115/1.1351889
Dürselen, L., Dauner, M., Hierlemann, H., Planck, H., Ignatius, A., and Claes, L. E. (2003). Control of material stiffness during degradation for constructs made of absorbable polymer fibers. J. Biomed. Mater Res. B Appl. Biomater. 67 (2), 697–701. doi:10.1002/jbm.b.10054
Dürselen, L., Häfner, M., Ignatius, A., Kraft, K., Hollstein, E., Pokar, S., et al. (2006). Biological response to a new composite polymer augmentation device used for cruciate ligament reconstruction. J. Biomed. Mater Res. B Appl. Biomater. 76 (2), 265–272. doi:10.1002/jbm.b.30381
Eam, C., Huntington, L. S., and Tulloch, S. (2022). Suture tape augmentation of anterior cruciate ligament reconstruction increases biomechanical stability: A scoping review of biomechanical, animal, and clinical studies. Arthroscopy 38 (6), 2073–2089. doi:10.1016/j.arthro.2021.12.036
Falconiero, R. P., DiStefano, V. J., and Cook, T. M. (1998). Revascularization and ligamentization of autogenous anterior cruciate ligament grafts in humans. Arthroscopy 14 (2), 197–205. doi:10.1016/s0749-8063(98)70041-6
Frangie, R., Warth, R. J., and Harner, C. D. (2020). Editorial commentary: will suture tape augmentation prove to Be the answer to anterior cruciate ligament graft remodeling, ultimate strength, and safe return to play? Arthroscopy 36 (2), 490–491. doi:10.1016/j.arthro.2019.11.129
Goradia, V. K., Rochat, M. C., Grana, W. A., Rohrer, M. D., and Prasad, H. S. (2000). Tendon-to-bone healing of a semitendinosus tendon autograft used for ACL reconstruction in a sheep model. Am. J. Knee Surg. 13 (3), 143–151.
Jisa, K. A., Williams, B. T., Jaglowski, J. R., Turnbull, T. L., LaPrade, R. F., and Wijdicks, C. A. (2016). Lack of consensus regarding pretensioning and preconditioning protocols for soft tissue graft reconstruction of the anterior cruciate ligament. Knee Surg. Sports Traumatol. Arthrosc. 24 (9), 2884–2891. doi:10.1007/s00167-015-3530-y
Kitchen, B. T., Mitchell, B. C., Cognetti, D. J., Siow, M. Y., Howard, R., Carroll, A. N., et al. (2022). Outcomes after hamstring ACL reconstruction with suture tape reinforcement in adolescent athletes. Orthop. J. Sports Med. 10 (4), 232596712210855. doi:10.1177/23259671221085577
Lai, V. J., Reynolds, A. W., Kindya, M., Konicek, J., and Akhavan, S. (2021). The use of suture augmentation for graft protection in ACL reconstruction: A biomechanical study in porcine knees. Arthrosc. Sports Med. Rehabil. 3 (1), e57–e63. doi:10.1016/j.asmr.2020.08.009
Li, G., Li, Y., Lan, P., Li, J., Zhao, Z., He, X., et al. (2014). Biodegradable weft-knitted intestinal stents: fabrication and physical changes investigation in vitro degradation. J. Biomed. Mater Res. A 102 (4), 982–990. doi:10.1002/jbm.a.34759
Loskot, J., Jezbera, D., Zmrhalová, Z. O., Nalezinková, M., Alferi, D., Lelkes, K., et al. (2022). A complex in vitro degradation study on polydioxanone biliary stents during a clinically relevant period with the focus on Raman spectroscopy validation. Polym. (Basel) 14 (5), 938. doi:10.3390/polym14050938
Magnussen, R. A., Lawrence, J. T., West, R. L., Toth, A. P., Taylor, D. C., and Garrett, W. E. (2012). Graft size and patient age are predictors of early revision after anterior cruciate ligament reconstruction with hamstring autograft. Arthroscopy 28 (4), 526–531. doi:10.1016/j.arthro.2011.11.024
Marieswaran, M., Jain, I., Garg, B., Sharma, V., and Kalyanasundaram, D. (2018). A review on Biomechanics of anterior cruciate ligament and materials for reconstruction. Appl. Bionics Biomech. 2018, 1–14. doi:10.1155/2018/4657824
Mariscalco, M. W., Flanigan, D. C., Mitchell, J., Pedroza, A. D., Jones, M. H., Andrish, J. T., et al. (2013). The influence of hamstring autograft size on patient-reported outcomes and risk of revision after anterior cruciate ligament reconstruction: A multicenter orthopaedic outcomes network (MOON) cohort study. Arthroscopy 29 (12), 1948–1953. doi:10.1016/j.arthro.2013.08.025
Matava, M. J., Koscso, J., Melara, L., and Bogunovic, L. (2021). Suture tape augmentation improves the biomechanical performance of bone-patellar tendon-bone grafts used for anterior cruciate ligament reconstruction. Arthroscopy 37 (11), 3335–3343. doi:10.1016/j.arthro.2021.04.053
Ménétrey, J., Duthon, V. B., Laumonier, T., and Fritschy, D. (2008). Biological failure" of the anterior cruciate ligament graft. Knee Surg. Sports Traumatol. Arthrosc. 16 (3), 224–231. doi:10.1007/s00167-007-0474-x
Montalvo, A. M., Schneider, D. K., Yut, L., Webster, K. E., Beynnon, B., Kocher, M. S., et al. (2019). What's my risk of sustaining an ACL injury while playing sports?" A systematic review with meta-analysis. Br. J. Sports Med. 53 (16), 1003–1012. doi:10.1136/bjsports-2016-096274
Noonan, B. C., Bachmaier, S., Wijdicks, C. A., and Bedi, A. (2020). Independent suture tape reinforcement of tripled smaller-diameter and quadrupled grafts for anterior cruciate ligament reconstruction with tibial screw fixation: A biomechanical full construct model. Arthroscopy 36 (2), 481–489. doi:10.1016/j.arthro.2019.06.036
Olvera, D., Sathy, B. N., Carroll, S. F., and Kelly, D. J. (2017). Modulating microfibrillar alignment and growth factor stimulation to regulate mesenchymal stem cell differentiation. Acta Biomater. 64, 148–160. Disclaimer/Publisher’s Note: The statements, opinions and data contained in all publications are solely those of the individual author(s) and contributor(s) and not of MDPI and/or the editor(s). MDPI and/or the editor(s) disclaim responsibility for any injury to people or property resulting from any ideas, methods, instructions or products referred to in the content. doi:10.1016/j.actbio.2017.10.010
Parkes, C. W., Leland, D. P., Levy, B. A., Stuart, M. J., Camp, C. L., Saris, D. B., et al. (2021). Hamstring autograft anterior cruciate ligament reconstruction using an all-inside technique with and without independent suture tape reinforcement. Arthroscopy 37 (2), 609–616. doi:10.1016/j.arthro.2020.09.002
Peterson, L., Eklund, U., Engström, B., Forssblad, M., Saartok, T., and Valentin, A. (2014). Long-term results of a randomized study on anterior cruciate ligament reconstruction with or without a synthetic degradable augmentation device to support the autograft. Knee Surg. Sports Traumatol. Arthrosc. 22 (9), 2109–2120. doi:10.1007/s00167-013-2636-3
Pilia, M., Murray, M., Guda, T., Heckman, M., and Appleford, M. (2015). Pretensioning of soft tissue grafts in anterior cruciate ligament reconstruction. Orthopedics 38 (7), e582–e587. doi:10.3928/01477447-20150701-55
Rea, A., Pam, A., Av, B., Jes, B., Cpb, A., and Ajc, A. (2018). Using an industrial braiding machine to upscale the production and modulate the design of electrospun medical yarns. Polym. Test. 69, 188–198. doi:10.1016/j.polymertesting.2018.05.014
Sánchez, M., Anitua, E., Azofra, J., Prado, R., Muruzabal, F., and Andia, I. (2010). Ligamentization of tendon grafts treated with an endogenous preparation rich in growth factors: gross morphology and histology. Arthroscopy 26 (4), 470–480. doi:10.1016/j.arthro.2009.08.019
Savage, E., Hurren, C. J., Slader, S., Khan, L. A., Sutti, A., and Page, R. S. (2013). Bending and abrasion fatigue of common suture materials used in arthroscopic and open orthopedic surgery. J. Orthop. Res. 31 (1), 132–138. doi:10.1002/jor.22185
Savić, L., Augustyniak, E. M., Kastensson, A., Snelling, S., Abhari, R. E., Baldwin, M., et al. (2021). Early development of a polycaprolactone electrospun augment for anterior cruciate ligament reconstruction. Mater Sci. Eng. C Mater Biol. Appl. 129, 112414. doi:10.1016/j.msec.2021.112414
Scheffler, S. U., Unterhauser, F. N., and Weiler, A. (2008). Graft remodeling and ligamentization after cruciate ligament reconstruction. Knee Surg. Sports Traumatol. Arthrosc. 16 (9), 834–842. doi:10.1007/s00167-008-0560-8
Silva, M., Ferreira, F. N., Alves, N. M., and Paiva, M. C. (2020). Biodegradable polymer nanocomposites for ligament/tendon tissue engineering. J. Nanobiotechnology 18 (1), 23. doi:10.1186/s12951-019-0556-1
Sinagra, Z. P., Kop, A., Pabbruwe, M., Parry, J., and Clark, G. (2018). Foreign body reaction associated with artificial LARS ligaments: A retrieval study. Orthop. J. Sports Med. 6 (12), 232596711881160. doi:10.1177/2325967118811604
Smith, P. A., Bozynski, C. C., Kuroki, K., Henrich, S. M., Wijdicks, C. A., and Cook, J. L. (2019). Intra-Articular biocompatibility of multistranded, long-chain polyethylene suture tape in a canine ACL model. J. Knee Surg. 32 (6), 525–531. doi:10.1055/s-0038-1655765
Smith, P. A., Bradley, J. P., Konicek, J., Bley, J. A., and Wijdicks, C. A. (2020a). Independent suture tape internal brace reinforcement of bone-patellar tendon-bone allografts: biomechanical assessment in a full-ACL reconstruction laboratory model. J. Knee Surg. 33 (10), 1047–1054. doi:10.1055/s-0039-1692649
Smith, P. A., Stannard, J. P., Bozynski, C. C., Kuroki, K., Cook, C. R., and Cook, J. L. (2020b). Patellar bone-tendon-bone autografts versus quadriceps tendon allograft with synthetic augmentation in a canine model. J. Knee Surg. 33 (12), 1256–1266. doi:10.1055/s-0039-1695040
Soreide, E., Denbeigh, J. M., Lewallen, E. A., Thaler, R., Xu, W., Berglund, L., et al. (2019). In vivo assessment of high-molecular-weight polyethylene core suture tape for intra-articular ligament reconstruction: an animal study. Bone Jt. J. 101-b (10), 1238–1247. doi:10.1302/0301-620x.101b10.bjj-2018-1282.r2
Taylor, K. A., Cutcliffe, H. C., Queen, R. M., Utturkar, G., Spritzer, C., Garrett, W., et al. (2013). In vivo measurement of ACL length and relative strain during walking. J. Biomech. 46 (3), 478–483. doi:10.1016/j.jbiomech.2012.10.031
Toutoungi, D. E., Lu, T. W., Leardini, A., Catani, F., and O'Connor, J. J. (2000). Cruciate ligament forces in the human knee during rehabilitation exercises. Clin. Biomech. (Bristol, Avon) 15 (3), 176–187. doi:10.1016/s0268-0033(99)00063-7
Vaishya, R., Agarwal, A. K., Ingole, S., and Vijay, V. (2015). Current trends in anterior cruciate ligament reconstruction: A review. Cureus 7 (11), e378. doi:10.7759/cureus.378
von Essen, C., Sarakatsianos, V., Cristiani, R., and Stålman, A. (2022). Suture tape reinforcement of hamstring tendon graft reduces postoperative knee laxity after primary ACL reconstruction. J. Exp. Orthop. 9 (1), 20. doi:10.1186/s40634-022-00454-2
Weiler, A., Peters, G., Mäurer, J., Unterhauser, F. N., and Südkamp, N. P. (2001). Biomechanical properties and vascularity of an anterior cruciate ligament graft can be predicted by contrast-enhanced magnetic resonance imaging: A two-year study in Sheep<sup/>. Am. J. Sports Med. 29 (6), 751–761. doi:10.1177/03635465010290061401
Wiggins, A. J., Grandhi, R. K., Schneider, D. K., Stanfield, D., Webster, K. E., and Myer, G. D. (2016). Risk of secondary injury in younger athletes after anterior cruciate ligament reconstruction: A systematic review and meta-analysis. Am. J. Sports Med. 44 (7), 1861–1876. doi:10.1177/0363546515621554
Yoo, J. S., Lee, S. J., Jang, J. E., Jang, Y., Kim, C., and In, Y. (2019). Biomechanical comparison of different tendon suturing techniques for three-stranded all-inside anterior cruciate ligament grafts. Orthop. Traumatol. Surg. Res. 105 (6), 1101–1106. doi:10.1016/j.otsr.2019.06.007
Zbrojkiewicz, D., Vertullo, C., and Grayson, J. E. (2018). Increasing rates of anterior cruciate ligament reconstruction in young Australians, 2000-2015. Med. J. Aust. 208 (8), 354–358. doi:10.5694/mja17.00974
Keywords: ACL reconstruction, augmentation technique, reinforcing cord, polydioxanone, ultra-high molecular weight polyethylene
Citation: Xu F, Li Y, Yu Y, Wang G and Cai G (2023) Evaluation of biomechanical properties and biocompatibility: are partially absorbable cords eligible for anterior cruciate ligament reconstruction?. Front. Bioeng. Biotechnol. 11:1216937. doi: 10.3389/fbioe.2023.1216937
Received: 04 May 2023; Accepted: 05 September 2023;
Published: 02 October 2023.
Edited by:
Bernardo Innocenti, Université libre de Bruxelles, BelgiumReviewed by:
Rene Verdonk, Ghent University, BelgiumDong Jiang, Peking University Third Hospital, China
Copyright © 2023 Xu, Li, Yu, Wang and Cai. This is an open-access article distributed under the terms of the Creative Commons Attribution License (CC BY). The use, distribution or reproduction in other forums is permitted, provided the original author(s) and the copyright owner(s) are credited and that the original publication in this journal is cited, in accordance with accepted academic practice. No use, distribution or reproduction is permitted which does not comply with these terms.
*Correspondence: Guofeng Cai, MTE0NzUwNzQ2OEBxcS5jb20=
†These authors share first authorship