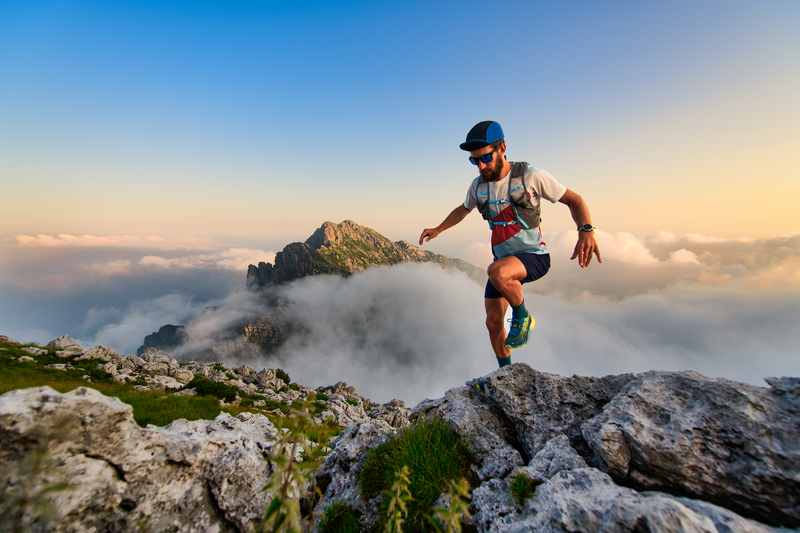
95% of researchers rate our articles as excellent or good
Learn more about the work of our research integrity team to safeguard the quality of each article we publish.
Find out more
REVIEW article
Front. Bioeng. Biotechnol. , 13 September 2023
Sec. Nanobiotechnology
Volume 11 - 2023 | https://doi.org/10.3389/fbioe.2023.1215650
This article is part of the Research Topic Role of Extracellular vesicles (EVs) in pathogenesis, diagnosis, therapeutic delivery, treatment and theranostic applications in Cancer View all 7 articles
Background: In recent years, extracellular vesicles have been recognized as important mediators of intercellular communication through the transfer of active biomolecules (proteins, lipids, and nucleic acids) across the plant and animal kingdoms and have considerable roles in several physiological and pathological mechanisms, showing great promise as new therapeutic strategies for a variety of pathologies.
Methods: In this study, we carefully reviewed the numerous articles published over the last few decades on the general knowledge of extracellular vesicles, their application in the therapy of various pathologies, and their prospects as an approach for the future.
Results: The recent discovery and characterization of extracellular vesicles (EVs) of diverse origins and biogenesis have altered the current paradigm of intercellular communication, opening up new diagnostic and therapeutic perspectives. Research into these EVs released by plant and mammalian cells has revealed their involvement in a number of physiological and pathological mechanisms, such as embryonic development, immune response, tissue regeneration, and cancer. They are also being studied as potential biomarkers for disease diagnosis and vectors for drug delivery.
Conclusion: Nanovesicles represent powerful tools for intercellular communication and the transfer of bioactive molecules. Their molecular composition and functions can vary according to their origin (plant and mammalian), so their formation, composition, and biological roles open the way to therapeutic applications in a variety of pathologies, which is arousing growing interest in the scientific community.
Clinical Trial Registration: ClinicalTrials.gov identifier: NCT03608631
Extracellular vesicles (EVs), also called microparticles, microvesicles, or exosomes, are a heterogeneous group of phospholipids membrane-bound micro-to nano-sized biovesicles derived from eucaryotic and procaryotic cells. EVs are complex membranes composed of lipid bilayers containing mainly proteins and surface receptors (Table 1), protecting the EV content (soluble and genetic material, including miRNAs) from proteases and nucleases (Abels and Breakefield, 2016; Linares et al., 2017; Suharta et al., 2021). These membrane-demarcated particles are produced and released by the cells of all three realms of life and are diverse in their morphology, biogenesis, composition, and biological role (Gill et al., 2019).
The history of their discovery dates from 1868 when Charles Darwin first presented the concept of EVs. His theory was based on the idea that each cell type in the body generates small germs or gemmules (particles), permitting communication and transfer of hereditary information with other cell types. He also suggested that the composition of the granules could be modified by the environment, reflecting the exposure of the organism. Extracellular vesicles have been of increasing interest to researchers for over 50 years. The first time EVs were observed and described by transmission electron microscopy in plants (cotton synergids) was in 1965 by William Jensen; he described them as “single-membraned spheres”, associated morphologically with the multivesicular bodies, which were structures believed at that point to be derived from the terminal portion of the endoplasmic reticulum (Jensen, 1965). Two years later, the extracellular vesicles associated with the multivesicular bodies were described by Halperin and Jensen in carrot cell cultures (Halperin and Jensen, 1967; Potestà et al., 2020; Pinedo et al., 2021), and further classified by Marchant et al., in 1968 as para mural-bodies (lonesome and plasmalemmas). Similar structures of small, spherical “particles” were ultra-structurally identified during the same time in Gram-negative bacteria, where Knox et al. (1966) described them as “extracellular globules” (Knox et al., 1966). In mammalian cells, the discovery of the extracellular vesicles is linked with the work of Chargaff and West (1945) on blood clotting, but the first morphological characterization is attributed to Wolf (1967), describing the extracellular vesicles derived from thrombocytes as dud-like expansions and “platelet dust” (Wolf, 1967; Couch et al., 2021).
They are applied in many fields as participating elements in pathophysiological processes, including those that favor pathological processes, such as atherosclerosis (Gomez et al., 2020) or thrombosis associated with cancer (Lacroix et al., 2019), and as a reflection of a biological event biomarker. EVs carry a large amount of information due to their lipid, protein, and nucleic acid contents. They were quickly considered potential new biomarkers. They are contained in numerous biological fluids, such as blood, pleural fluid, and urine which is also an advantage in the theatre as a biomarker. Some studies also consider them as therapeutic targets. The EVs derived from plants have been examined for their therapeutic activities and their structure and cargo are similar to that of EVs isolated by mammalian cells. In addition, plant-derived EVs include bioactive lipids, proteins, and mRNAs, and can deliver this important cargo to other cells just like mammalian EVs (Ali et al., 2022). More importantly, they compound several advantageous properties such as antioxidant (Dinicola et al., 2014), antibacterial (Hosseini-Giv et al., 2022), anti-inflammatory (Zhang et al., 2016a), anticancer (Chen et al., 2022), and regenerative potential for various diseases (Zhu and He, 2023).
Exosomes also called ectosomes, exovesicles, or exosome-like vesicles are uniformly round, membrane-covered structures (He et al., 2021) with a diameter of 30–150 nm (Figure 1) (Tauro et al., 2012). Exosomes are produced by the constitution of multivesicular bodies and secreted to the extracellular milieu after integration into the plasma membrane (Mathivanan et al., 2010; Żmigrodzka et al., 2016). Exosomes are known for their role in intercellular communication and can transport mRNA, bioactive lipids, and proteins (Rayner and Hennessy, 2013; Robbins and Morelli, 2014) and regulate gene/protein expression. Upon contact, exosomes deliver molecules that can confer new characteristics and/or reproduce recipient cells. They are composed of a certain number of specific proteins, notably the tetraspanin family (CD81, CD82, CD9 and CD63, flotillin, TSG101, and Alix) and heat choc proteins (HSP90, HSP70, HSPA5, CCT2, and HSP60) (Théry et al., 2009; AYokoi et al., 2015; Ciardiello et al., 2016; Ha et al., 2016). Exosomal membranes are composed of sphingomyelin, cholesterol, phosphatidylinositol, ceramide, phosphatidylethanolamine, phosphatidylserine, etc. (Tamkovich et al., 2016).
FIGURE 1. Schematic illustration of the formation of the different EV sub-types. Schematic representation of the liberation of the different types of EVs directly by bourgeoning from the plasma membrane for MPs, by fusing internal multivesicular divisions (MVBs) with the plasma membrane for exosomes, and by bourgeoning from a cell in the process of apoptosis for apoptotic bodies. Adapted from Gurunathan et al. (2019).
Microvesicles (MV) also called microparticles (MP) are vesicles that bud by burgeoning from the plasma membrane and has a size of 50–1,000 nm or even more (Figure 1) (Gould and Raposo, 2013). MV contains essential protein markers including integrins, selectins, and CD40. Their membranes possess cholesterol, diacylglycerol, and phosphatidylserine in greater quantities than exosomes (He et al., 2021). Their constitution is based on its principal mechanisms by a change of membrane phospholipids and the modification of the cytoskeleton of the cell. These MVs then implicate part of the cell’s cytoplasm. They also reflect the state of activation of their cell of origin or its apoptosis (Boireau and Elie-Caille, 2021).
Apoptotic bodies also called apoptosomes are vesicles with the dimension of 1–5 µm delivered by cells in apoptosis (Figure 1) (Zitvogel et al., 1998). They are essentially liberated during cellular apoptosis (Poon et al., 2014). Apoptotic bodies are cleared to assure a “clean” elimination of the cellular content following apoptosis. This content has a strong immunogenic potential, and its release in the extracellular milieu induces local inflammatory reactions. By embedding them in vesicles, the contents of the cell will be eliminated by macrophages that recognize the PS expressed on the apoptotic bodies, thus avoiding any inflammatory responses (Zitvogel et al., 1998). These apoptotic bodies’ function is not limited to the clearance of cellular components since they also participate in intercellular communication.
Extracellular vesicles are distinguished into three groups based on their formation mechanism: exosomes, microvesicles (MV), and apoptotic bodies (AB) (Table 1; Figure 1).
Plant-derived VEs are heterogeneous groups of vesicles containing different functions, mainly multivesicular bodies (MVBs), autophagosomes, vacuoles, and exocyst-positive organelles (EXPOs). The size of plant-derived nanovesicles is generally between 50 and 1,000 nm and varies according to plant origin and isolation technique (see Table 2). Plant-derived extracellular vesicles conduct heterogeneous cargos containing different biomolecules (proteins, small RNAs, lipids, and nucleic acids) and are primarily composed of phosphatidic acid, phosphatidylcholine, di galactosyl diacylglycerol, monogalactosyldiacylglycerol, and phytosterols (Liu et al., 2020a; Kocholata et al., 2022). The group of phospholipids occupies several functions including stability, vesicle liberation, and intercellular communication, and participates in the mechanism of membrane fusion. In addition, phosphatidylcholine and phosphatidylethanolamine are known for their important roles in strengthening therapeutic activities (antioxidant, antipolitic, and anti-inflammatory) (Wang et al., 2014). The presence of lipid composition in the vesicle membrane has an important role in intercellular interactions and in maintaining vesicle stability under physiological and pathological conditions (Xu et al., 2023). However, small RNAs are present in plant-derived nanovesicles that are capable of regulating biological functions, notably inter-kingdom communication to ensure communication between species as has been indicated in Arabidopsis EVs (Zhang et al., 2016b) in the presence of tiny RNAs (ty RNA) with a length of 10–17 nucleotides, long non-coding RNAs (lncRNA), circular RNAs (circRNA), and small RNAs (sRNA) (Cai et al., 2018). RNAs can be identified in the exterior of EVs, where they can be secured by RNA-binding proteins against enzymatic destruction. In addition, PENETRATION1 was identified between the Golgi complex and the plasma membrane and established as a plant-derived nanovesicle (PDNVs) biomarker (Wang et al., 2010). Syntaxins, which are localized in the membrane of the SNARE family were also established as integrated proteins that are implicated in the transport of vesicles inside the cells (Rutter and Innes, 2017). It has been demonstrated that PENETRATION1 does not localize with ARA6, so it indicates that the biogenic pathway of TET8-positive EVs is not the same as that of PEN1-positive EVs. Certain plant tetraspanins, notably Arabidopsis thaliana TETRASPANIN 8 and TETRASPANIN 9 (AtTET8 and AtTET9) are identified explicitly in infection by the fungal pathogen Botrytis cinerea and colocalize with the Arabidopsis MVB marker Rab5-type GTPase ARA6 inside the cell and EVs at fungal infection sites (Ding et al., 2014). In addition, the Exocyst-positive organelle (EXPO) was recently found to combine with the plasma membrane and release Exo70E2-positive cells in the intercellular spaces during live-cell imaging in plants using the immunostaining technique. The Exo70E2 secretion pathway is free of MVB pathways, and EXPO is unaffected by inhibitors of secretion and endocytosis in protoplasts.
Plant-derived nanovesicles generally present as a spherical structure when isolated which could promote cell wall passage. Plant-derived nanovesicle biological compounds, which include proteins, small RNAs, and metabolites, are unique in every case and depend on the original cell (Pegtel and Gould, 2019). These two biomarkers PENETRATION1 and TETRASPANIN 8 of EVs, which are not located in the same places are classified in two categories of plant EVs. Tetraspanins occupy a very important role in biogenesis, especially cargo selection, membrane fusion, and exosome absorption (Colombo et al., 2014). Plant-derived nanovesicles’ biogenesis pathway is similar to the exosome biogenesis pathway in that plant nanovesicles associated with TETRASPANIN 8/TETRASPANIN 9 (TET8/TET9) are produced by multivesicular bodies (MVBs). PENETRATION1 is a plant-derived nanovesicle biomarker found in the plasma membrane (Rutter and Innes, 2017). It has been established that trafficking between the Golgi and the plasma membrane is mediated by PEN1. There is no coincidence between PEN1 and ARA6, which determines that the biogenic pathway of TET8 EVs is not the same as that of PEN1 EVs (He et al., 2021). However, another pathway of EV biogenesis has been observed in the EXPO (EXocyst-Positive Organelles), presented generally as a spherical double-membrane structure, compared to the autophagosome, which has been identified in Arabidopsis. Although they present similarities with autophagosomes, which combine with the plasma membrane and liberate membrane vesicles in the cell wall, these vesicles are considered to be exosomes secreted by EXPO (Wang et al., 2010). Indeed, EXPO can combine with Exo70E2 passing the plasma membrane and thus releasing plant-derived nanovesicles (Figure 2). Plant-derived nanovesicles of RNAs are identified in encapsulated EVs, but they are outside the nanovesicles and can be defended by RNA-binding proteins from enzymatic degradation. As has been proved, ty-RNAs of Arabidopsis thaliana are abundant in EVs in proportion to cellular RNAs (Baldrich et al., 2019), and only siRNAs of the same RNA precursors can be discovered in EVs. RNA-binding proteins (RBPs) appear to have an important role in loading RNA into EV precursors and in the stability of exotic RNAs. Membrane polysomes could be a site for RNA loading into vesicles. Plant-derived nanovesicles are derived from the endocytic pathway when membranes invaginate during late endocytosis to form intraluminal vesicles (ILVs) inside multivesicular bodies (MVBs) (Zhang et al., 2017). ILVs are generated when premature endosomes integrate with the peripheral membrane layer. They encapsulate plant-derived nanovesicles in MVBs, which combine with the plasma membrane to release exosomes. Although the biogenesis of plant-derived nanovesicles has not been well studied, there is evidence for at least three distinct pathways (Figure 2) (Cai et al., 2019; Nemati et al., 2022).
FIGURE 2. Biogenesis pathway of plant-derived nanovesicles. Adapted from Lian et al. (2022).
The types of EVs are MVs and EXOs, and CA has various modes of biogenesis. MVs are derived from bourgeoning from the plasma membrane, while EXOs originate in the endosomal system in the form of intraluminal vesicles (ILVs), which are released when MVBs fuse with the plasma membrane, and apoptotic bodies are essentially liberated by bourgeoning from a cell undergoing apoptosis to apoptotic bodies (Poon et al., 2014) (Figure 3). However, even if the formation of MV and EXO occurs at different cell locations, intracellular mechanisms may be implicated in the formation of the two entities, depending on the cell type (van der Pol et al., 2014). According to the discovery in this study (van der Pol et al., 2014), the results indicated that T lymphocytes were capable of forming EVs at the level of the plasma membrane consisting of the characteristics of EXOs. Then, the intracellular mechanisms implied in the two formations simultaneously alter the possibility of establishing different sub-populations of EVs (Colombo et al., 2014).
FIGURE 3. Biogenesis of microvesicles (A) ARF6: ADP-Ribosylation Factor 6; ESCRT: Endosomal Sorting Complex Required for Transport (van Niel et al., 2018). (B) The molecular machinery of EXO biogenesis: schematization of the different pathways involved in EXO formation involving the ESCRT machinery, tetraspanins, and some lipids. (C) Apoptotic body extrusion appears in the scheme and in sections of conventionally embedded apoptotic cells. Adapted from Colombo et al. (2014).
Exosomes of endosomal origin are confirmed by the analysis of vesicles liberated by immune cells, such as B lymphocytes and dendritic cells (Zitvogel et al., 1998). They are generated by the association of late endosomes with multivesicular bodies (MVBs) which are then released in the extracellular milieu by exocytosis. The constitution of MVBs implies the machinery of the Endosomal Sorting Complex Required for Transport (ESCRT). This machinery is composed of many proteins assembled in four complexes: ESCRT-0, -I, -II, and -III. These complexes have fused proteins such as VSP4, VAT1, ATPase, and TSG101 (Hanson and Cashikar, 2012). In short, before the cascade, we discovered ESCRT-0, which takes care of mobilizing the cargo to the lysosome. It engages ESCRT-I, which makes interaction with ESCRT-II to compose the lipidic vesicle during which the cargos are sequestered. Finally, ESCRT-II engages ESCRT-which takes care of liberating the vesicle in the MVB, acting at the same time with the fifth complex of the machinery: VPS4/VTA1. The formation of EXO independently of the ESCRT complex has been described, implying the synthesis of ceramides. Neutral sphingomyelinase hydrolyzes sphingomyelin to ceramide. This ceramide generates membrane sub-domains that impose a spontaneous negative curvature on the membrane. It has been demonstrated that inhibition of neutral sphingomyelinase 2 (nSMAse-2) prevents ILV bourgeoning in BVMs and EXO liberation by a mechanism independent of the ESCRT machinery (Trajkovic et al., 2008). Exosomes can be detected by different markers, some of which are specific to this type of EV, notably tetraspanin-like membrane proteins (CD9, CD63, CD81, and CD82), or cytosolic proteins involved in endo-lysosomal traffic including Alix, Tsg101, or 14-3-3, major histocompatibility complex (MHC) proteins, heat shock proteins (HSP) chaperones as well as the ESCRT-3 complex binding to the Alix protein (Verderio et al., 2018).
Knowledge of the biogenesis process of MVs produced from healthy cells is more recent (Minciacchi et al., 2015). The biogenesis of MVs results from a remodeling of the cytoskeleton induced by an increase in intracytosolic Ca2+ concentration (Al-Nedawi et al., 2008) (Figure 3A). The increase in Ca2+ stimulates enzymatic machinery providing membrane phospholipid asymmetry, including aminophospholipid translocases (flippases and floppies), scramblases, and calpain. The activation of the enzymatic machinery leads to the externalization of phosphatidylserine (PS) from the inner to the outer leaflet of the plasma membrane. The loss of membrane asymmetry induces an excess of negative charge at the surface of the plastic membrane (Piccin et al., 2007). This excess charge is responsible for membrane curvature and cytoskeletal reorganization favoring MV release (Jimenez et al., 2003; Del Conde et al., 2005). Inhibition of scramblase has been shown to suppress PS externalization in platelets and the formation of procoagulant MVs (Jimenez et al., 2003). However, even when membrane phospholipid asymmetry is maintained, MVs can be formed (Del Conde et al., 2005). These observations indicate that other lipids, and the domains they structure, contribute to the biogenesis of MVs. An important membrane lipid component is cholesterol, which is present in MVs. Depletion of membrane cholesterol has been shown to decrease MV production by THP-1 monocytes (Li et al., 2012). In addition to membrane remodeling and cytoskeletal rearrangement, other regulators are necessary for MV biogenesis. The activity of small GTPases of the RHO family and ROCK (RHO-associated protein kinase), which are important regulators of actin dynamics, induces MV formation in different tumor cells. (McConnell et al., 2009).
Release of MVs requires rupture of the plasma membrane. This mechanism depends on the interaction of actin and myosin with ATP-dependent contraction. (Muralidharan-Chari et al., 2009). In cancer cells, it has been shown that activation of ARF6 (ADP-Ribosylation Factor 6), ADP Ribosylation Factor 1 (ARF1), and small GTP-binding proteins lead to phosphorylation of the myosin side chain of myosin and contraction of actomyosin, permitting MV to detach from the membrane (Nabhan et al., 2012). Another regulator of actin dynamics, the Cdc42 (Cell division control protein 42 homolog), is involved in MV release in HeLa cells; however, the mechanism is unknown (McConnell et al., 2009). In another study, TSG101 (Tumor Susceptibility Gene 101 Protein) and ATPase VPS4, which are mainly involved in the EXO formation via the ESCRT machinery, were reported to be involved in the fission and release of MV (Wehman et al., 2011). The involvement of the ESCRT machinery in MV release has also been demonstrated in C. elegans embryos (Bianco et al., 2005). Another possible pathway for MV release is the activation of the ATP-dependent P2X receptor 7 (P2X purinoceptor 7), which is ATP-dependent. Activation of the receptor results in membrane rearrangement that influences MV release (Bianco et al., 2009). This process is associated with the translocation of acid sphingomyelinase to the plasma membrane, which produces ceramide, favoring membrane bourgeoning and MV liberation. (Reátegui et al., 2018).
Apoptotic bodies are larger than other vesicles and carry particular markers (thrombospondin, complement component 3, and C3B) (Akers et al., 2013; Duijvesz et al., 2015). Apoptotic bodies produce apoptosis-induced cell death (Suárez et al., 2017). They are fragments of the cell formed by blebbing. This phenomenon is produced when ruptures are formed in the actin cortex caused by actomyosin contractions. This creates weak points in this actin cortex. These weak points end up inflating under the hydrostatic pressure of the cell providing bourgeoning called blebs. The actin is then rearranged in these blebs, leading to membrane scission (Suárez et al., 2017). The vesicles are thus liberated into the extracellular milieu (Akers et al., 2013; Suárez et al., 2017). Some smaller vesicles can also be produced during apoptosis (apoptotic vesicles), but the mechanisms that control their production and release are not yet clearly defined (Suárez et al., 2017).
Extracellular vesicles are generally isolated from different biofluids. Given their generosity in terms of size and concentration, and depending on their mode of biogenesis and cellular origin, the separation of these extracellular vesicles is according to their sub-types and sub-populations of EVs, using different isolation techniques or methods (Boireau and Elie-Caille, 2021).
Nowadays, several methods of EV purification exist, but not all of them allow for obtaining the same degree of purification and the same level of concentration of the sample. For this reason, some techniques are called “concentration” techniques, such as differential centrifugation, which allows only a slight purification of the sample even when several rounds of washing are carried out, whereas other techniques are called “purification” techniques because they allow a real separation between the EVs and the soluble proteins of the surrounding medium. At present, many teams are proposing a combination of several methods for purifying EVs. This section will examine the different reference techniques used to isolate EVs as indicated in Figure 4.
FIGURE 4. different methods of isolation of EVs. Adapted from Monguió-Tortajada et al. (2019), Boireau and Elie-Caille (2021), Chhoy et al. (2021).
Ultracentrifugation is the most frequently used method for isolating EVs. This method has been used for studying EVs derived from cell culture supernatants and biological fluids (Campoy et al., 2016; Coughlan et al., 2020). Some advantages of ultracentrifugation are that it can isolate EVs from large volumes of biological fluids, requires a relatively restricted set of reactants and consumables, and has no impact on EVs apart from gravitational force and pipetting (as no chemicals that are likely to interfere with downstream EV analysis are used). To reduce debris caused by co-sedimentation and contamination of preparations by cell lysis products, this phase also includes several sub-phases, firstly by centrifugation at 300–400 × g for 10 min, secondly sedimentation of a large proportion of the cells at 2000 × g to remove cell debris, thirdly at 10,000 × g to eliminate biopolymer aggregates and apoptotic bodies, and finally to obtain contiguous EVs in the supernatant, which are increased by ultracentrifugation at (100,000–200,000 × g) over 2 h.
Differential ultracentrifugation (UC) is a well-known method for isolating EVs in favor of its ease of use and its low character accessibility (Witwer et al., 2013; Pérez-Bermúdez et al., 2017). It is used for separating large EVs (by centrifugation at around 10,000 g) and small EVs (ultracentrifugation at 100,000 g). Differential ultracentrifugation pellets large extracellular vesicles at a slow centrifugation speed and small ones at a high speed (100 000 g). Macromolecules and/or lipoproteins can be co-precipitated with the extracellular vesicles (Blandin and Le Lay, 2020).
Density gradient ultracentrifugation is a classic technique for isolating groups of vesicles based on their density and buoyancy velocity (Cai et al., 2018). This enables them to improve the purification of extracellular vesicles. The flotation of the isolated EVs on a density gradient permits the separation of macromolecular complexes and/or lipoproteins co-precipitated with the EVs. In addition, this method permits excellent results in terms of EV fraction purity and the number of EV proteins and RNA to classical ultracentrifugation and commercial kits (Van Deun et al., 2014). Moreover, it is established that EV preparations isolated from this solution are deprived of microvesicles superior to 200 nm in contrast to EV obtained by other methods (Lobb et al., 2015). At present, density gradient ultracentrifugation is frequently used to isolate microvesicles. However, this method leads to a decrease in the size of EVs; it is complex, tedious, time-consuming (up to 2 days), and requires expensive materials (Lobb et al., 2015; Zeringer et al., 2015).
Diverse techniques have been invented to filter EVs. These include cross-flow filtration (CFT) and centrifugal ultrafiltration (UF). In the last stage, high pressure is used to increase the density of smaller particles, permitting them to pass through the membrane without being blocked by clumps. In addition, UF provides a cut-off point for damage to its membranes due to the weight of the particles. This permits the removal of any contaminants found in extracellular vesicles using TFF. Sequential UF, consisting of a succession of filtration steps with a gradual reduction of the cut-off point, can permit EV isolation and enrichment from complex biological fluids (Boireau and Elie-Caille, 2021).
Size exclusion chromatography is a classical technique for the fractionation of biological entities that establish isolation in proportion to the dimension of the object or its hydrodynamic volume (Boireau and Elie-Caille, 2021). It is used as a porous matrix where EVs smaller than the pore size will be reserved for a long time according to their elution of more advanced large-sized EVs (Blandin and Le Lay, 2020). It is largely applied to the preparation of biopolymers (proteins, polysaccharides, proteoglycans, etc.). As demonstrated, this technique can also be used to separate EVs from protein and lipoprotein complexes in blood plasma and urine (Böing et al., 2014; Muller et al., 2014; Lozano-Ramos et al., 2015; Gámez-Valero et al., 2016), which is difficult, and many other methods have failed (Mathivanan et al., 2012). Gel chromatography is an efficient and rapid technique permitting the isolation of EVs efficiently without loss of high reproducibility. In particular, exosomes have a very large hydrodynamic radius in comparison with proteins, lipoproteins, and protein complexes, and they can be separated very well from these components. However, the size of some of the chylomicrons is similar to that of the isolated vesicles; similarly, EV preparations made in this manner compound lipoproteins but at a much slower rate than that found in the case of other techniques applied to EV isolation (Yamamoto et al., 1970).
Immunocapture or magnetic sorting is a technique that permits the retention of EVs expressing a surface antigen (e.g., CD9, CD63, or CD81) using monoclonal antibodies (directed against this antigen) coupled to magnetic beads retained on a column by magnets. In the absence of a magnetic field, EVs positive with this antigen are then eluted. The isolation of sub-populations of EVs can also be performed by immuno-capture using antibodies specifically directed against antigens carried by EVs, such as tetraspanins (Blandin and Le Lay, 2020). This technique consists of isolating a particular sub-category of EVs from balls coated with an antibody that recognizes the specific protein marker exposed on EV membranes. It can also prevent contamination of isolated EVs by cytoplasmic proteins or RNA (Van Deun et al., 2014).
This is a method that uses polymer solutions with dextran to induce phase separation or extracts that will induce the precipitation (at low-speed centrifugation) of VEs by its surfactant properties, and polyethylene glycol (PEG) retains the macromolecules and other molecular components. The precipitation of EVs by polymer mixtures (Dextran, polyethylene glycol, or PEG) permitting phase separations at a low centrifugation speed and the precipitation of EVs is the basic principle of many commercial kits (e.g., Exo Quick TCTM). These last kits present the advantage of being rapid and easy to use but often lead to the co-precipitation of macromolecular protein complexes, lipoproteins, or immunoglobulins (Blandin and Le Lay, 2020). PEGs of diverse molecular weights have been used for many years for the precipitation of proteins, nucleic acids, viruses, and other small particles (Yamamoto et al., 1970). The technique uses a diminution of and the solvency of elements in super hydrophilic polymer solutions, PEG. The procedure is limited to the combination of polymer solution and sample, incubating and pelleting the EVs by low-speed centrifugation (1,500 × g).
In conclusion, there are other methods for isolating extracellular vesicles, such as microfluidic systems, immunological separation, and microfluidics. This number continues to grow in parallel with technological advances.
At this moment, various methods have been used to isolate mammalian-derived EVs, primarily ultracentrifugation, differential centrifugation, density gradient, and size exclusion chromatography. However, not all these methods are adapted to the evolution of extracellular vesicle production. These methods require several complicated and repetitive centrifugation steps to remove all debris. In addition, these steps are not yet well-detailed in the literature. This observation has permitted some researchers to develop new methods to purify EVs using simple procedures such as those mentioned in the following studies such as isolation of EVs derived from human milk by ultracentrifugation and filtration. For example, separate milk samples by different centrifugations (6,500 × g at 4°C for 30 min and 12,000 × g at 4°C for 1 h) were used to remove debris. Next, skimming was affected using 0.45 and 0.22 μm filters to remove any remaining debris. The filtered supernatant was centrifuged at 135,000× g for 90 min at 4°C to granulate the exosomes (Reif et al., 2020; You et al., 2021). In another study, EVs derived from milk were isolated by differential centrifugation; for density gradient, the milk was centrifuged twice at 3,000 × g, and then the milk supernatant was subjected to differential centrifugation at 5,000 × g and 10,000 × g in new, sterilized SW40 tubes. The supernatant at 10,000 × g was again added to a sucrose gradient (ranging from 2.0 to 0.4 M sucrose) and centrifuged at 192,000 × g for 15–18 h. At the final stage, the samples were collected, combined, and centrifuged at 100,000 × g for 65 min, the supernatant removed, and the EV pellets aliquoted and stored at 80°C (Van Herwijnen et al., 2018a). Bovine milk was isolated by the acetic acid/ultracentrifugation (AA/UC) method. The first skimmed milk was heated for 10 min at 37°C, then mixed with acetic acid, followed by centrifugation at 10,000 g for 10 min at 4°C. The supernatant was filtered through a 0.22 μm membrane and designated as lacto-serum. Lacto-serum was ultracentrifuged at 210,000 g for 70 min at 4°C. An EV pellet was resuspended with phosphate-buffered saline (PBS) for clean-up, and the remaining precipitates were removed by centrifugation at 10,000 g for 5 min at 4° (András and Toborek, 2015). In Table 3 we have summarized several studies on the Isolation of mammalian-derived extracellular vesicles.
There are several methods for the isolation of plant-derived nanovesicles (PDNVs), for example, differential ultracentrifugation (UC) combined with sucrose density gradient centrifugation, PEG precipitation, size exclusion chromatography (SEC), ultra-filtration membrane separation, etc., Nevertheless, in practical terms (extraction rate, extraction time, and purity) none of these methods are excellent, but the combination of several methods seems to be the best possible solution. The advantages and disadvantages of these techniques are established. Differential ultracentrifugation and the extraction method are the most used techniques for isolating nanovesicles of plant origin, then they are purified by density centrifugation on a sucrose gradient. Different types of nanovesicles can be selected based on centrifugation density and sucrose gradient (Yang et al., 2018a). The main stage of the UC step is to prepare the sample including mixing, pressing, or grinding, then plant-derived nanovesicles (by differential ultracentrifugation and sucrose gradient ultracentrifugation) are isolated and purified. In a centrifuge, the supernatant is initially centrifuged at a low speed to remove fiber debris in the plant tissue; in a second step, the centrifugation speed is gradually increased to remove finer particles while preserving plant-derived EVs in the supernatant. Evidently, as the number of centrifugations increases, the speed of centrifugation gradually increases and the duration of centrifugation becomes longer and longer. After three centrifugations at a relatively low speed, the supernatant is maintained. At this point, a centrifugal force of between 100,000 and 150,000 g is selected for the second centrifugation. (Li et al., 2023a). (See Figure 5). In Table 4 we have summarized several studies on the Isolation of Plant-derived extracellular vesicles.
FIGURE 5. (A) Isolation process of nanovesicles derived from a comestible plant (fruit or vegetable) as a first step of sample preparation (mixing, pressing, or grinding). The second step is the isolation and purification of plant-derived nanovesicles (e.g., by differential ultracentrifugation and sucrose gradient ultracentrifugation). (B) The process of isolating EVs from plant leaves. Initial steps to isolate the fluid of apoplastic wash from detached plant leaves. The leaves are then cut using a pair of scissors. The leaves are put into a syringe, then deposited on a piece of transparent adhesive tape and rolled onto the syringe. The taped leaves are then placed in a 50 mL conical tube and collected by centrifugation. Adapted from Li et al. (2023a).
The biological activities of exosome-type nanovesicles, in their intact natural structural integration with their bioactive cargoes after their simple isolation from plant EVs, diminish pathological situations in species of other reigns and provide multiple therapeutic alternatives (Mu et al., 2014; Dad et al., 2021).
To give an overview of the potential therapeutic application of plant-derived extracellular vesicles (P -EVs) based on plant types, plant comestibles have in recent years been the focus of numerous studies, which have revealed encouraging specificities that explain their availability, biocompatibility, and biodegradability. These EVs could well be necessary for the therapy of many diseases. (Kameli et al., 2021).
More importantly, they consist of several advantageous properties such as Ginger which has various beneficial properties including anti-oxidant (Hung et al., 2017), antibacterial (Dinicola et al., 2014), anti-inflammatory (Rome, 2019), anti-cancer (Vislocky and Fernandez, 2010), and regenerative potential for various diseases (Brahmbhatt et al., 2013). In addition, plant-derived nanovesicles are continents of biomolecules including proteins, drugs, DNA vectors, and siRNAs, and are delivered to target tissues (Nemati et al., 2022). It is for this cause that the biomolecules of plants have gotten much attention from researchers for their potential to improve health and defend against diverse infections.
In recent years, in the field of nanotechnology, nanovesicles derived from edible plants have attracted the attention of scientists for their drug delivery potential, as these particles can deliver hydrophobic and hydrophilic therapeutic agents to disease target sites. We will describe studies that have proven the therapeutic efficacy of nanovesicles derived from edible plants (grape, grapefruit, ginger, lemon, and carrot).
Ginger has intrinsic chemical compositions, such as shoal and gingerol, possessing several health benefits. In addition, multiple studies have indicated the therapeutic effects of ginger, including these studies (Zhang et al., 2016b; Zhu and He, 2023) where the authors have studied the effects of ginger in regeneration. Ginger-derived nanovesicles have demonstrated their role in reducing the expression of secreted hemotoxic protein and influencing the expression of other mitochondrial and cytoplasmic proteins such as heat shock protein, axing, and kinesin for intestinal wound recovery (Kim et al., 2022). Further studies have been interested in the effect of ginger in antitumor activities; the research found that plant-derived miRNAs are capable of crossing species and performing a veritable regulatory role in the human organism (Liu et al., 2017). Furthermore, ginger-derived nanovesicles have been demonstrated to reduce cyclin D1 RNA levels in mice with colorectal cancer (Zhang et al., 2016b). Studies indicate that ginger has hepatoprotective properties against ethanol- and acetaminophen-induced hepatotoxicity of carbon tetrachloride (Zhuang et al., 2015).
Grapefruit-derived nano-vectors (GNVs) presented varieties of therapeutic agents, including chemotherapeutics, DNA expression vectors, siRNAs, and proteins such as antibodies. It has been reported that particles purified from grapefruit indicated high stability and can carry several agents such as curcumin and Zymosan that were functionally active. Grapefruit-derived nanovesicles had similarities in size and structure to mammalian-derived exosomes. They consisted of proteins, lipids, and miRNA and have been assimilated by intestinal macrophages and stem cells. In another study, Wang et al. coated grapefruit-derived nanoparticles (GDNV) rich in active anti-inflammatory leukocyte receptors (IGNV). Using various animal models of inflammation-induced diseases, they have demonstrated that IGNVs target inflamed tumor tissue better than GDNVs. Moreover, this targeting of inflamed tissue was significantly inhibited by blocking LFA-1 or CXCR1/CXCR2 on IGNV membranes. Wang et al. reported that grapefruit-derived nanovesicles could release chemotherapeutic agents, siRNAs, DNA expression vectors, and proteins in different cell types. They also simultaneously administered grapefruit-derived nanovesicles and folic acid, and reported that this strategy significantly increased the efficiency of targeting cells expressing folic acid receptors. They then demonstrated that these nanovesicles improved chemotherapy-induced tumor growth inhibition in CT26 and SW620 cell-derived tumors in mice (Wang et al., 2015).
Nanovesicles derived from Citrus limon (lemon) contain many benefits, including antibacterial, antifungal, anti-inflammatory, anticancer, hepato-regenerative, and cardioprotective activities (Bhavsar et al., 2007; Kim et al., 2012; Riaz et al., 2014; Parhiz et al., 2015; Otang and Afolayan, 2016). The pharmacological potential of citrus lemon is determined by its rich chemical composition such as phenolic acids, coumarins, carboxylic acids, amino acids, and vitamins. In addition, some studies have demonstrated that citrus lemon nanovesicles inhibited the growth of chronic myeloid leukemia (CML) tumors in vivo by specifically reaching tumor sites and activating the TRAIL-mediated apoptosis process. Also, they were studied for their role in reducing tumor growth. They were capable of curbing in vivo tumor development in chronic myeloid leukemia (CML) by targeting tumors, reducing oxidation, and reducing cancer risk (Raimondo et al., 2015). In another study, citrus limon-derived nanovesicles were shown to possess cell growth inhibitory effects, primarily in p53-inactivated CRC cell lines, via the macropinocytosis pathway. The results of this study indicate that p53 inactivation activated macropinocytosis activity and that citrus lemon-derived nanovesicles had a cell growth inhibitory effect via the macropinocytosis pathway (Takakura et al., 2022).
Extracellular vesicles derived from carrot contains phytochemicals, namely, phenolics, carotenoids, polyacetylenes, and ascorbic acid. These chemical products help reduce the risk of cancer and cardiovascular disease because of their antioxidant, anti-inflammatory, plasma lipid-modifying, and anti-tumor properties. The role of polyphenols in the prevention of degenerative diseases, such as cancer, cardiovascular diseases, and neurodegenerative diseases has been reported. Carrot (Daucus carota) is also used in research for its medical effects.
Carrot juice also contains glutathione, an antioxidant that protects against free radicals. It presents potent anti-inflammatory properties that permit the relief of rheumatic and arthritic symptoms (Metzger et al., 2008). A study establishing that carrot-derived nanovesicles have anti-inflammatory and antioxidant effects that can restore glucose tolerance and cardiovascular and liver functions has been assessed in an in vivo model (Poudyal et al., 2010). In another study, EVs derived from carrots (Carex) were studied as a novel biomaterial with antioxidant functions in cardiomyoblastoma and neuroblastoma cells. The results indicated similar properties to EVs, and their antioxidant and apoptotic effects in cardio-myoblasts and neuroblastoma cells were further studied. Carex significantly inhibited ROS production and apoptosis induction; therefore, the antioxidant effect of Carex may be more effective in the early phase of diseases. Carex presented low cytotoxicity in H9C2 cardiomyocytes and SH-SY5Y neuroblastoma cells when high levels of Carex were delivered to cells. In addition, Carex inhibited the reduced expression of antioxidant molecules, including Nrf-2, HO-1, and NQO-1, in both models (Kim and Rhee, 2021).
Grape (Vitis vinifera) nanovesicles and their bioactive compounds have several pharmacological activities such as antioxidation and risk reduction and contain several active components including flavonoids, polyphenols, anthocyanins, proanthocyanidins, procyanidins, and resveratrol, a derivative of stilbene. It has a wide range of pharmacological and therapeutic effects, such as antioxidant, anti-inflammatory, and antimicrobial activities, as well as cardioprotective, hepatoprotective, and neuroprotective effects.
Wang et al. (2013); Zhuang et al., 2016 reported that grape-derived nanovesicles contain microRNA, proteins, and lipids. Although these nanovesicles do not resemble mammalian cell-derived exosomes, their structure and composition are similar to mammalian cell-derived exosomes (Ju et al., 2013). Grape-derived nanovesicles consist of proteins such as aquaporins and HSP70 proteins enriched in phosphatidylethanolamine. A study has indicated that grape-derived nanovesicles present unique transport characteristics and biological functions (Ju et al., 2013; Yu et al., 2020). These nanovesicles can traverse the intestinal mucosal barrier and be taken up by mouse intestinal stem cells, significantly inducing intestinal stem cells through the Wnt/β-catenin pathway (Yu et al., 2020). In addition, nanovesicle grape derivatives can reduce many of the risk factors associated with cancer, cardiovascular health, age-related cognitive diseases, and neurodegenerative diseases. These effects are generally attributed to the function of flavonoids in grapes, antioxidant activity, and increased nitric oxide production (Vislocky and Fernandez, 2010).
In terms of therapeutic applications, the extracellular vesicles released by plant cells possess several therapeutic agents, including drugs, proteins, DNA vectors, and siRNAs, and deliver them to target tissues, making them promising natural resources for modern drug discovery. In addition, they also reduce the risk of various pathologies as mentioned above, including cancer, chronic inflammatory diseases, and others. Because of their drug delivery potential, as these particles can deliver both hydrophobic and hydrophilic therapeutic agents to targeted disease sites, there has been a lot of attention from scientists which shows that extracellular vesicles are positioned as an ideal candidate for therapeutic applications. However, plant-derived nanovesicles can locate intrinsically in target tissues, which is one of the most important characteristics of a targeted delivery system. All aspects of plant-derived nanovesicles have not yet been fully identified and described, as they represent a new concept in the field of nanomedicine. The therapeutic potential of plant-derived nanovesicles has recently been demonstrated in several disease models and has been widely applied in the development of new drugs to treat particular diseases or maintain healthy body functions. A variety of plants have been used for the isolation of therapeutically effective exosomes presenting diverse functionalities. Table 5 lists important plants that have been used to extract plant-derived nanovesicles and their therapeutic applications.
In conclusion, to advance therapeutically, drug nanocarriers require a thorough evaluation of their physicochemical characteristics and communications in various biological environments (Herrmann et al., 2021). While liposomes have been largely evaluated, EVs have indicated unique properties that make them superior for drug delivery systems (Kooijmans et al., 2016). Plant-derived nanovesicles can be used as drug carriers as they present advantageous properties including low immunity, tissue-specific targeting, safety, large-scale production, preferred negative zeta potential values, and the ability to load many biomolecules. In Table 5, we present a summary of another plant-derived nanovesicle for therapeutic use.
We will discuss different studies that attribute various therapeutic functions to mammalian-derived extracellular vesicles, e.g., extracellular vesicles of an endosomal origin that are released by different cell types (neurons) serve to remove unwanted proteins in a drainage system and also serve to intercellularly transport their cargo: a specific set of proteins, RNAs, and lipids. Recently, extracellular vesicles derived from mouse neuroblastomas captured Aβ, resulting in reduced amounts of Aβ, decreased amyloid deposition, and reduced Aβ-induced synaptotoxicity in the hippocampus. This demonstrates the role of neuroblastoma cell extracellular vesicles in Aβ release (Yuyama et al., 2014). Results in another study were also similarly described on neuron-derived EVs (Yuyama et al., 2015). On the other hand, endothelial extracellular vesicles secreted by activated or apoptotic endothelial cells may be capable of participating in both toxic and positive effects of the vascular endothelial response. These effects may include anticoagulation, anti-inflammatory effects, angiogenesis, endothelial survivance, and endothelial regeneration (Dignat-George and Boulanger, 2011). In another study of miRNAs presented in human and porcine milk, EVs focused on a variety of genes that were involved in the regulation of the epithelial barrier and neonatal defense; these miRNAs associated with breast milk EV contributed to the subsequent guided development of the newborn (Van Herwijnen et al., 2018b). However, another study indicated that extracellular vesicles in pig milk also attenuated deoxynivalenol-induced changes in body weight and intestinal epithelial growth in mice. These extracellular vesicles inhibited cell proliferation and the creation of tight junction proteins and reduced deoxynivalenol-induced apoptosis. EVs also increased the expression of miR-181a, miR-30c, miR-365-5p, and miR-769-3p in IPEC-J2 cells, then reduced the expression of their target genes in the p53 pathway, and finally attenuated DON expression by promoting cell proliferation and tight junctions and inhibiting apoptosis to induce lesion (Xie et al., 2020). In addition, a study of cow’s milk-derived extracellular vesicles that reduced primary tumor growth and attenuated the progression of weight loss problems in cancer-related body weight loss was examined using the C-26 colorectal tumor model. Their discoveries highlighted the role of milk-derived extracellular vesicles in interspecies communication and their considerable context-dependent role in regulating cancer progression and metastasis. However, their results also suggested that milk-derived extracellular vesicles possess antiproliferative properties on cancer cells in vitro (Zhuang et al., 2016), as demonstrated in similar studies (Munagala et al., 2016). It has been indicated that peripheral endothelial cell-derived EVs may be involved in the modulation of innate immunity (Palette et al., 2013). In addition, human dermal microvascular endothelial cells have selectively sequestered cytoplasmic RNA degradation mechanisms in exosomes, which could also be involved in gene regulation. It has been established that dendritic cell-derived EVs can be focused to deliver siRNAs to neurons, microglia, and oligodendrocytes in the mouse brain (András and Toborek, 2015; Somiya et al., 2018). In another study, EVs derived from umbilical cord polyvalent mesenchymal stromal cells presented anti-apoptotic, pro-angiogenic, and antifibrotic activities, and immunomodulatory effects; effects similar to those of their source cells (Rohde et al., 2019).
In terms of therapeutic application, after confronting a series of challenges when developing a therapeutic approach including toxicity, safety, target specificity and large-scale production, leading to several targeting strategies for drug delivery in preclinical and clinical settings. Mammalian-derived nanovesicles are promising for clinical applications, both as biomarkers and as therapeutic vectors. As proven by scientists, EVs can be used as therapeutic agents or as drug cargo for reaching a specific target thanks to their membrane protein (Yang et al., 2018b). While the drug is encapsulated in extracellular vesicles, the advantage is that EVs act as a drug carrier, protecting the drug and transmitting it safely to the target site. Numerous studies have indicated that vesicles derived from mesenchymal stem cells (MSCs) appear especially useful for enhancing recovery after various injuries. As has been indicated in mice, injection of MSC-derived EVs suppressed hypoxia-induced inflammation and hypertension (Lee et al., 2012). MSC EVs can exert a neuroprotective effect after a brain lesion (Xin et al., 2012). Similarly, MSC EVs release miR-16 and other molecules to mouse breast cancer cells, exerting a decrease in vascular endothelial growth factor expression and a reduction in tumor growth (Lee et al., 2013). More recently, a study has demonstrated that VEs derived from macrophages or liver sinusoidal cells treated with interferon-α deliver antiviral RNA and proteins to hepatocytes, thereby reducing hepatitis B virus replication (Li et al., 2013). How different natural EVs promote these diverse responses remain to be elucidated. Drug loading into EVs can be achieved by these two strategies, either by directly loading the drug into exosomes or/and by loading the drug to target the mother cell during exosome biogenesis (Mittelbrunn and Sanchez-Madrid, 2010). Moreover, in the case of lipophilic drug switching, the mechanism is relatively simple due to the interaction between the lipid bilayer of EVs and the drug, which occurs via a hydrophobic interaction (Shtam et al., 2013). Such methods as electroporation, incubation, sonication, and thawing have been performed for the exogenous loading of EVs with drugs (Jo et al., 2014).
The use of EVs as drug carriers presents several advantages but must conform to good manufacturing practice (GMP) (Chen et al., 2020). There are several important aspects of nanovesicle GMP. The low large-scale productivity of nanovesicles is one of the major problems encountered in the implementation of nanovesicle-based therapeutics; the main issues to be known about the various isolation methods are the physicochemical and purity properties of exosomes for the high-quality, uniquely shaped nanovesicle community and the standardization of storage requirements. The evolution of the therapeutic potential of nanovesicles by enriching them with therapeutic biomolecules is a simple way of improving their therapeutic potential (Abou-El-Enein et al., 2013). To use nanovesicles as reliable therapeutic agents, scalable manufacturing processes are needed to produce exosomes rapidly, cost-effectively, and reproducibly. In Table 6, we present a summary of another mammalian nanovesicle for therapeutic use.
In this section, we will present the different studies that have been performed on plant and mammalian-derived extracellular vesicles in vivo and in vitro.
Studies that have evaluated plant-derived extracellular vesicles in vivo and in vitro: in vitro studies, researchers observed that Grapefruit-derived nano-vectors have important biocompatibility with little toxicity and apoptosis of macrophages and colon-26 cells in vitro, compared to commercial DC-Chol/DOPE liposome preparation (Nemati et al., 2022). Further in vivo and in vitro tests confirmed that Grapefruit-derived nano-vectors had the potential to regenerate mucus tissue in mice with colitis (Zhang et al., 2016b). Other studies have examined in detail the function of four plant-derived EVs (carrots, grapes, grapefruit, and ginger) on intercell communication. This study was realized in vitro and in vivo, and the results have suggested that these extracellular vesicles can regulate intestinal intercellular communication (Mu et al., 2014).
It was reported that grapefruit-derived nanovesicles were labeled with a lipophilic carbocyanine dye that permitted their in vivo tracking by fluorescence (Zhuang et al., 2016). An in vivo study using diverse inflammation models in mice indicated that leukocyte-coated plant-derived extracellular vesicles (P-EVs) promoted the efficiency of Dox release at inflammatory sites (Li et al., 2018).
Studies that evaluated mammalian-derived extracellular vesicles in vivo and in vitro: In vitro and in vivo studies used to identify TGFβ receptors and mechanisms of action revealed pleiotropic roles for TGFβ in the control of pathophysiological processes. In addition, numerous preclinical results from in vitro cell models and in vivo animal models demonstrate the great potential of antitumor therapies using TGFβ-neutralizing antibodies and ligand traps that block the interaction of TGFβ with its receptors or selective receptor kinase inhibitors for the small molecule TGFβ (Liu et al., 2021). In another study, the effect of siponimod on ocular neovascularization in vivo was evaluated using suture-induced corneal neovascularization in albino rabbits. These results suggest that siponimod does not affect endothelial cell proliferation or metabolic activity but significantly inhibits endothelial cell migration, increases human microvascular endothelial cells’ (HMEC) barrier integrity, and reduces TNF-α-induced barrier disruption (Palette et al., 2013). In another in vivo study, differences in protein cargo and non-coding RNA were identified that differentiated cardio-sphere-derived EV cells from mesenchymal stem cells and reflected differences in the effects of in vivo treatment in vivo (Walravens et al., 2021).
The study of the efficacy of extracellular vesicles for immunogenicity or toxicity in therapy is fundamental to the preclinical development and development of their therapeutic properties. Evaluation of the toxicity and security of EVs in vitro and in vivo will permit the establishment of the dose for future clinical use and the recommendations for safe consumption for human application. An ideal drug delivery system should guarantee non-toxicity and non-immunogenicity without secondary effects both in vitro and in vivo (Zhuang et al., 2015). However, there are still a few tentative discoveries of their cytotoxic effect on living subjects. So far, plant-derived nanovesicles have indicated fascinating biocompatibility due to their natural origin. To evaluate the toxicity of plant-derived nanovesicles’ toxicity, the authors used tumor-targeted Grapefruit-derived nano-vectors (GDNV) and examined whether significant tissue damage occurred in the organs. Because of the effectiveness of GDNV on tumors, GDNV accumulates less in the spleen and liver; this permits the minimization of systemic drug toxicity to normal tissues while improving blood diffusion of delivered drugs. Histological analysis of the heart, liver, spleen, lungs, or kidneys has not demonstrated significant damage compared to the control group, indicating that plant-derived nanovesicles can be applied as nano-drug delivery platforms, improving drug efficacy and reducing potential toxicity (Kim et al., 2022). In a recent study, markers such as pro-inflammatory cytokines and serum levels of liver enzymes, including alanine aminotransferase (ALT) and aspartate aminotransferase (AST), have been evaluated to determine the potential cytotoxic effects of grape-derived nanovesicles in mice. Mice were pretreated with grapefruit-derived nanovesicles and commercially available DOTAP (1,2-diammonium-propane)-DOPE (dioleoyl phosphatidylethanolamine) liposomes.
Loyal-3-trimethylammonium-propane)-DOPE (dioleoyl phosphatidylethanolamine) liposomes and pro-inflammatory cytokines were significantly elevated in liposomes. In addition, the authors found that no increase was recorded in the group of mice treated with Plant Exosome-like Nanovesicles (PELNV). Furthermore, no pathological alterations were observed in histological samples of the liver, kidney, spleen, and lung from mice treated with plant exosome-like nanovesicles (Ding et al., 2014).
In addition, in a study, researchers evaluated the toxicity of milk-derived EVs. The result indicated that no systematic toxicity or immunogenicity was observed. In the experiment, animals were given an intravenous injection containing milk-derived EVs. The results of the blood tests confirmed no damage from these EVs, and no markers of harm or toxicity to the kidneys or liver were found (András and Toborek, 2015). Another study conducted in vitro to evaluate the toxicity of EVs derived from mesenchymal cells and bovine milk indicated that there was no genotoxic response to these two EVs, but a platelet aggregation induced by collagen in a dose-dependent manner was recorded. Another study was conducted in vitro to evaluate the safety of HEK293T cell-derived EVs. Mice were injected intravenously with HEK293T cell-derived EVs. The results indicated no toxic effects, no immune changes, and no alterations in EVs (Rohde et al., 2019).
In effect, plant- and mammal-derived nanovesicles present numerous advantages in terms of biocompatibility, stability, biodistribution, and cellular internalization. However, some challenges related to biosafety and toxicity may also be encountered due to unknown bioactive components of the plant.
Based on the results of the effects of plant-derived nanovesicles, many clinical trials have been initiated to complement the results (Table 7). In another clinical trial (NCT01668849), grape-derived nanovesicles were administered as an anti-inflammatory agent to diminish oral mucositis in head and neck cancer patients undergoing chemotherapy (Xie et al., 2020; Nemati et al., 2022). Trials were conducted after 6–7 weeks of treatment. In addition, another clinical trial (No. NCT01294072) was carried out in 2011 on turmeric-derived nanovesicles for more effective delivery of curcumin to the gut. (Xie et al., 2020). The effect of turmeric-derived nanovesicles on malignant and normal clonal cells and their effect on the immune system were applied to colon cancer patients. This study was developed to investigate the effect of turmeric nanovesicle administration on oral tablets under recruitment conditions (Xie et al., 2020).
There were numerous clinical trials on mammalian-derived extracellular vesicles for therapeutic purposes. The application, dose, number of patients, identification number, and follow-up are indicated. Currently, studies are in clinical trials (listed at www.clinicaltrials.gov).
In a study, the application of mesenchymal stem cell (MSC)-derived exosomes based on siRNA-exosome therapy is used in the treatment of pancreatic cancer patients with KrasG12D mutation via KrasG12D in a phase I clinical trial (ClinicalTrials.gov identifier: NCT03608631). Despite these encouraging results for the application of MSC-derived exosomes as drug vesicles for cancer treatment in the clinic, many challenges remain (Mendt et al., 2018). A combination of ascites-derived exosomes with granulocyte-macrophage colony-stimulating factor (GM-CSF) was tested in a phase I clinical trial for the treatment of advanced colorectal cancer, which was found to be feasible and safe and also capable of eliciting more CTL infiltration in tumor regions (Dai et al., 2008). A study was performed at Sahel University Hospital, Cairo University, to evaluate the effect of consecutive doses of CSM-EV in 20 patients with type 1 diabetes, with a follow-up of 3 months. The results are not yet available (Mendt et al., 2018). In the same hospital, another study recruited 20 patients with chronic renal failure and were administered two doses of CMS-EV from the umbilical cord with follow-up for 1 year and the results are already available (Nassar et al., 2016). Finally, a clinical trial, which involves the injection of MSC-EVs engineered with miR-124 for the treatment of patients after acute ischemic stroke, was approved in Iran (Grange et al., 2019) (see Table 8).
Therapies based on plant- and mammalian-derived extracellular vesicles (EVs) are attracting growing interest as a promising therapeutic approach in various medical fields for the treatment of diverse diseases. The benefits of these devices include their ability to cross biological barriers, enhance drug pharmacokinetics and therapeutic efficacy, and diminish the toxic side effects commonly associated with conventional synthetic nanovesicles. In addition, EVs can be chemically modified to incorporate additional ligands for targeted drug delivery. In addition to their ability to transport biomolecules to other cells, namely, proteins, lipids, and nucleic acids, they are a promising tool for clinical diagnostic and disease prognostic assessments. However, many technological, functional, and safety aspects still need to be taken into account (Li et al., 2023b; Sadeghi et al., 2023).
Targeted drug therapy: EVs can be modified to express specific surface ligands, giving them the ability to selectively target particular cells or tissues. This offers exciting opportunities for targeted drug delivery while at the same time minimizing adverse effects on other healthy tissues. However, the obstacle to EV therapy that needs to be overcome to achieve a therapeutic result is the regulation of EV uptake. Many steps have been taken to improve the factors influencing EV uptake, including cell source selection, cell growth procedures, extraction and purification methods, storage, and routes of administration. Rapid distribution, targeted delivery, and non-targeting of EVs are current challenges that need to be addressed (Claridge et al., 2021; Esmaeili et al., 2022).
Gene therapy: Gene therapy can be applied to treat genetic diseases, control gene expression in particular cells, or trigger specific healing processes. In addition, there is an urgent need for drug delivery vectors capable of efficiently transferring therapeutic cargo to recipient cells while bypassing the cellular barriers created by the development of new genotoxic anti-cancer therapies. Drug delivery methods have been proposed to overcome these restrictions, but their successful clinical application has been thwarted by the occurrence of unanticipated adverse effects and related toxicities (Duan et al., 2021; Jayasinghe et al., 2021).
Immunotherapy: the use of extracellular vesicles has been applied to the field of cancer immunotherapy, and this has made remarkable progress, becoming a real tool in the fight against cancer. Extracellular vesicles were also seen as vectors for molecules capable of activating an immune response and destroying cancer cells. Based on this observation, the new approach is to use extracellular vesicles as a new means against cancer. The immunotherapeutic approach based on extracellular vesicles in the treatment of cancer patients has been demonstrated, even in the case of advanced cancers. The use of extracellular vesicles in immunotherapy does not reveal any qualitative or moral difficulties. Progress in this field will therefore lead to practical results and a new, innovative approach to the fight against cancer. (Giacobino et al., 2021; Marar et al., 2021).
Tissue regeneration: A promising approach in regenerative medicine is the therapeutic use of extracellular vesicles for tissue regeneration. Extracellular vesicles can contain growth factors and proteins essential to the tissue regeneration process. Growth factors can be selectively delivered to target cells via these vesicles, promoting their proliferation and differentiation. This approach is still in the development stage, so it will be some time before it becomes established as a clinical therapy for tissue regeneration. It could 1 day provide new therapeutic alternatives for the treatment of various diseases and conditions associated with tissue damage. (Ju et al., 2022; Zheng et al., 2022).
In recent decades, VEs had capabilities of cell-to-cell communication in prokaryotes and eukaryotes due to their ability to transfer active biomolecules such as proteins, lipids, nucleic acids, and other biologically active substances, and have considerable roles in several physiological and pathological mechanisms. In recent years, extracellular vesicles present numerous benefits in terms of biocompatibility, therapeutic capacity, targeting ability, and cellular uptake. Their low toxicity and immunogenicity make extracellular vesicles an emerging, versatile, and promising biotherapy for a very wide variety of diseases.
In addition, research has indicated that EVs are essential for communication between plants, mammals, and pathogens and that they perform significant roles in a variety of pathologies. However, despite the constant progress of discoveries, complementary studies on extracellular vesicles are still needed in several aspects, especially cellular and molecular biogenesis, functions, and uptake which are not yet well known. In the context of isolation, we remark that particularly for plant-derived EVs, the results obtained in the various studies often vary according to plant derivative, the isolation technique, or the physiological state of the plant, as well as the content of the isolated nanoparticles due to the lack of a standardized isolation protocol. Also, improvements in EV isolation permit the fabrication of high-purity EVs for biomarker discoveries. As new markers of EV subcategories continue to emerge, the capacity to use high-fluorescence microscopy should improve the understanding of EV subcategory biogenesis. In addition, there is a lack of information on plant-derived EV biogenesis, as there are few specific protein markers for EVs, and determining the biological characterization of exosomes needs further investigation, as the surface markers and other characteristic elements of plant exosomes remain uncertain. The application of extracellular vesicles’ therapeutic potential requires further clinical trials to obtain more precise information on results, development of effective isolation, scale-up methodologies, stability, and properties of either plant- or mammalian-derived extracellular vesicles. Although clinical trials on some extracellular vesicles are underway, the regulatory aspects of their use as therapeutic agents are not known. Despite the difficulties and obstacles encountered by researchers in this field, it has been proven that extracellular vesicles have natural therapeutic capacity advantages without toxicity or side effects. Therefore, when targeted and developed with multidisciplinary expertise, EVs can be transformed into reusable therapeutic agents to fight against various pathologies.
The advantage of using nanovesicles is that the efficacy of natural products (plants or mammals) in therapeutic applications as indicated in these studies can be improved by increasing their bioavailability. In systems, nano-distribution can also be used to overcome the limits of therapeutic applications of natural products for several reasons: their capacity to target nanovesicles to specific organs, thus improving selectivity, therapeutic application, efficacy, and security. Nanovesicles passively target pathological sites of action without the addition of specific ligand fragments. The therapeutic efficacy of nanovesicles can reduce side effects because of their properties. Nanovesicles increase the solubility of natural compounds. Nanovesicles can dissolve rapidly in the blood, so they appear to be able to administer small-sized drugs. In addition, it is well established in these studies that the application of nanovesicles in clinical trials is an ideal candidate for the treatment of many diseases. The authors have indicated that several preclinical experiments have confirmed the advantages of nanovesicles for the treatment of numerous pathologies ranging from regenerative medicine to cancers.
Ultimately, we expect that with further research on all the aspects we have just mentioned above on the use of extracellular vesicles, in the next few years, we will probably see an increase in the use of extracellular vesicles both for the diagnosis against more widespread pathologies and as a starting point for the development of new therapies.
IS: writing–Preparation of the original draft. TF: supervision and Validation.
The publication was supported by funds from the National Research Development Projects to Finance Excellence (PFE)-14/2022-2024 granted by the Romanian Ministry of Research and Innovation and the University of Agricultural Sciences and Veterinary Medicine of Cluj/Romania.
The authors declare that the research was conducted in the absence of any commercial or financial relationships that could be construed as a potential conflict of interest.
All claims expressed in this article are solely those of the authors and do not necessarily represent those of their affiliated organizations, or those of the publisher, the editors and the reviewers. Any product that may be evaluated in this article, or claim that may be made by its manufacturer, is not guaranteed or endorsed by the publisher.
Abels, E. R., and Breakefield, X. O. (2016). Introduction to extracellular vesicles: biogenesis, RNA cargo selection, content, release, and uptake. Cell. Mol. Neurobiol. 36 (3), 301–312. View at: Publisher Site | Google Scholar. doi:10.1007/s10571-016-0366-z
Abou-El-Enein, M., Römhild, A., Kaiser, D., Beier, C., Bauer, G., Volk, H-D., et al. (2013). Good manufacturing practices (GMP) manufacturing of advanced therapy medicinal products: A novel tailored model for optimizing performance and estimating costs. Cytotherapy 15, 362–383. Publisher's website |PubMed | Google Scholar |. doi:10.1016/j.jcyt.2012.09.006
Abraham, A. M., Wiemann, S., Ambreen, G., Zhou, J., Engelhardt, K., Brüßler, J., et al. (2022). Cucumber-derived exosome-like vesicles and plant crystals for improved dermal drug delivery. Pharmaceutics 14, 476. [PMC free article] [PubMed] [CrossRef] [Google Scholar] [Ref list]. doi:10.3390/pharmaceutics14030476
Akers, J. C., Gonda, D., Kim, R., Carter, B. S., and Chen, C. C. (2013). Biogenesis of extracellular vesicles (EV): exosomes, microvesicles, retrovirus-like vesicles, and apoptotic bodies. J. Neurooncol 113 (1), 1–11. Epub 2013 Mar 2. PMID: 23456661; PMCID: PMC5533094.Publisher's pub med |Google Scholar. doi:10.1007/s11060-013-1084-8
Al-Nedawi, K., Meehan, B., Micallef, J., Lhotak, V., May, L., Guha, A., et al. (2008). Intercellular transfer of the oncogenic receptor EGFRvIII by microvesicles derived from tumour cells. Nat. Cell Biol. 10 (5), 619–624. Erratum in: Nat Cell Biol. 2008 Jun;10(6):752. PMID: 18425114. Publisher's pub med |Google Scholar. doi:10.1038/ncb1725
Ali, N. B., Abdull Razis, A. F., Ooi, J., Chan, K. W., Ismail, N., and Foo, J. B. (2022). Theragnostic applications of mammal and plant-derived extracellular vesicles: latest findings, current technologies, and prospects. Molecules 27 (12), 3941. ; PMCID: PMC9228370. See on: Publisher's website PubMed | Google Scholar. doi:10.3390/molecules27123941
Alvarez-Erviti, L., Seow, Y., Yin, H., Betts, C., Lakhal, S., and Wood, M. J. (2011). Delivery of siRNA to the mouse brain by systemic injection of targeted exosomes. Nat. Biotechnol. 29, 341–345. PubMed | CrossRef |Google Scholar. doi:10.1038/nbt.1807
Aminzadeh, M. A., Fournier, M., Akhmerov, A., Jones-Ungerleider, K. C., Valle, J. B., and Marbán, E. (2021). Casein-Enhanced uptake and disease-modifying bioactivity of ingested extracellular vesicles. J. Extracell. Vesicles 10, e12045. [CrossRef]. doi:10.1002/jev2.12045
András, I. E., and Toborek, M. (2015). Extracellular vesicles of the blood-brain barrier. Tissue Barriers 4 (1), e1131804. PMID: 27141419; PMCID: PMC4836554. Publisher's website |PubMed | Google Scholar | CrossRef. doi:10.1080/21688370.2015.1131804
Ayokoi, A., Yoshioka, Y., and Ochiya, T. (2015). Towards the realization of clinical extracellular vesicle diagnostics: challenges and opportunities. Expert Rev. Mol. Diagn 15 (12), 1555–1566. See on: Publisher's website PubMed | Google Scholar. doi:10.1586/14737159.2015.1104249
Baldrich, P., Rutter, B. D., Karimi, H. Z., Podicheti, R., Meyers, B. C., and Innes, R. W. (2019). Plant extracellular vesicles contain diverse small RNA species and are enriched in 10- to 17-nucleotide "tiny" RNAs. Plant Cell 31, 315–324. doi:10.1105/tpc.18.00872
Berger, E., Colosetti, P., Jalabert, A., Meugnier, E., Wiklander, O. P. B., Jouhet, J., et al. (2020). Use of nanovesicles from orange juice to reverse diet-induced gut modifications in diet-induced obese mice. Mol. Ther. Methods Clin. Dev. 18, 880–892. PMID: 32953937; PMCID: PMC7481887. See on: Publisher's website |PubMed | Google Scholar. doi:10.1016/j.omtm.2020.08.009
Bhavsar, S. K., Joshi, P., Shah, M. B., and Santani, D. D. (2007). Investigation into hepatoprotective activity ofCitrus limon. Pharm. Biol. 45, 303–311. [Google Scholar] [CrossRef]. doi:10.1080/13880200701214995
Bianco, F., Perrotta, C., Novellino, L., Francolini, M., Riganti, L., Menna, E., et al. (2009). Acid sphingomyelinase activity triggers microparticle release from glial cells. EMBO J. 28 (8), 1043–1054. Epub 2009 Mar 19. Erratum in: EMBO J. 2009 May 6;28(9):1374. Publisher's pub med |Google Scholar. doi:10.1038/emboj.2009.45
Bianco, F., Pravettoni, E., Colombo, A., Schenk, U., Möller, T., Matteoli, M., et al. (2005). Astrocyte-derived ATP induces vesicle shedding and IL-1 beta release from microglia. J. Immunol. 174 (11), 7268–7277. PMID: 15905573. Publisher's pub med |Google Scholar. doi:10.4049/jimmunol.174.11.7268
Blandin, A., and Le Lay, S. (2020). Exosomes, extracellular vesicles and interorgan dialogue. Med. Metabolic Dis. 14 (3), 250–257. ISSN 1957-2557. doi.org/Publisher's website | Sciencedirect. doi:10.1016/j.mmm.2020.01.013
Böing, A. N., van der Pol, E., Grootemaat, A. E., Coumans, F. A., Sturk, A., and Nieuwland, R. (2014). Single-step isolation of extracellular vesicles by size-exclusion chromatography. J. Extracell. Vesicles 3, 23430. PMID: 25279113; PMCID: PMC4159761. Publisher's website |PubMed | Google Scholar | web science. doi:10.3402/jev.v3.23430
Boireau, W., and Elie-Caille, C. (2021). Extracellular vesicles: definition, isolation and characterization. Med. Sci. Paris. 37 (12), 1092–1100. French Epub 2021 Dec 20. PMID: 34928211. See on: Publisher's website PubMed | Google Scholar. doi:10.1051/medsci/2021201
Bokka, R., Ramos, A. P., Fiume, I., Manno, M., Raccosta, S., Turiák, L., et al. (2020). Biomanufacturing of tomato-derived nanovesicles. Foods 9 (12), 1852. PMID: 33322632; PMCID: PMC7764365. See on: Publisher's website |PubMed | Google Scholar. doi:10.3390/foods9121852
Brahmbhatt, M., Gundala, S. R., Asif, G., Shamsi, S. A., and Aneja, R. (2013). Ginger phytochemicals exhibit synergy to inhibit prostate cancer cell proliferation. Nutr. Cancer 65 (2), 263–272. PMID: 23441614; PMCID: PMC3925258. Publisher's website |PubMed | Google Scholar. doi:10.1080/01635581.2013.749925
Budai, M. M., Varga, A., Milesz, S., Tőzsér, J., and Benkő, S. (2013). Aloe vera downregulates LPS-induced inflammatory cytokine production and expression of NLRP3 inflammasome in human macrophages. Mol. Immunol. 56 (4), 471–479. Epub 2013 Aug 1. PMID: 23911403. [PubMed] [CrossRef] [Google Scholar] [Liste de référence]. doi:10.1016/j.molimm.2013.05.005
Burke, J., Kolhe, R., Hunter, M., Isales, C., Hamrick, M., and Fulzele, S. (2016). Stem cell-derived exosomes: A potential alternative therapeutic agent in orthopaedics. Stem Cells Int. 2016, 1–6. [CrossRef] [PubMed]. doi:10.1155/2016/5802529
Cai, Q., He, B., and Jin, H. (2019). A safe ride in extracellular vesicles - small RNA trafficking between plant hosts and pathogens. Curr. Opin. Plant Biol. 52, 140–148. Epub 2019 Oct 23. PMID: 31654843. Publisher's Pub med |Google Scholar |web science. doi:10.1016/j.pbi.2019.09.001
Cai, Q., Qiao, L., Wang, M., He, B., Lin, F. M., Palmquist, J., et al. (2018). Plants send small RNAs in extracellular vesicles tofungal pathogen to silence virulence genes. Science 360, 1126–1129. free article |PubMed |CrossRef |Google Scholar. doi:10.1126/science.aar4142
Campoy, I., Lanau, L., Altadill, T., Sequeiros, T., Cabrera, S., Cubo-Abert, M., et al. (2016). Exosome-like vesicles in uterine aspirates: A comparison of ultracentrifugation-based isolation protocols. J. Transl. Med. 14 (1), 180. article no. 180, 2016. See on: Publisher's website | Google Scholar. doi:10.1186/s12967-016-0935-4
Cao, M., Yan, H., Han, X., Weng, L., Wei, Q., Sun, X., et al. (2019). Ginseng-derived nanoparticles alter macrophage polarization to inhibit melanoma growth. J. Immunother. Cancer 7 (1), 326. PMID: 31775862; PMCID: PMC6882204. See on: Publisher's website |PubMed | Google Scholar. doi:10.1186/s40425-019-0817-4
Caruso, S., and Poon, I. K. H. (2018). Apoptotic cell-derived extracellular vesicles: more than just debris. Front. Immunol. 9, 1486. PMID: 30002658; PMCID: PMC6031707.Publisher's pub med |Google Scholar |web science. doi:10.3389/fimmu.2018.01486
Chen, J., Tan, Q., Yang, Z., and Jin, Y. (2022). Engineered extracellular vesicles: potentials in cancer combination therapy. J. Nanobiotechnol 20, 132. See on: Publisher's website PubMed | Google Scholar. doi:10.1186/s12951-022-01330-y
Chen, X., Zhou, Y., and Yu, J. (2019). Exosome-like nanoparticles from ginger rhizomes inhibited NLRP3 inflammasome activation. Mol. Pharm. 16 (6), 2690–2699. Epub 2019 Apr 30. PMID: 31038962. Publisher's website |PubMed. doi:10.1021/acs.molpharmaceut.9b00246
Chen, Y-S., Lin, E-Y., Chiou, T-W., and Harn, H-J. (2020). Exosomes in clinical trial and their production in compliance with good manufacturing practice. Tzu Chi Med. J. 32, 113–120. Publisher's website |PubMed | Google Scholar |. doi:10.4103/tcmj.tcmj_182_19
Chhoy, P., Brown, C. W., Amante, J. J., and Mercurio, A. M. (2021). Protocol for the separation of extracellular vesicles by ultracentrifugation from in vitro cell culture models. Star. Protoc. 2 (1), 100303. PMID: 33554138; PMCID: PMC7848770. doi:10.1016/j.xpro.2021.100303
Cianciaruso, C., Phelps, E. A., Pasquier, M., Hamelin, R., Demurtas, D., Ahmed, M. A., et al. (2017). Primary human and rat β-cells release the intracellular autoantigens GAD65, IA-2, and proinsulin in exosomes together with cytokine-induced enhancers of immunity. Diabetes 66, 460–473. [CrossRef] [PubMed]. doi:10.2337/db16-0671
Ciardiello, C., Cavallini, L., Spinelli, C., Yang, J., Reis-Sobreiro, M., de Candia, P., et al. (2016). Focus on extracellular vesicles: new Frontiers of cell-to-cell communication in cancer. Int. J. Mol. Sci. 17 (2), 175. See on: Publisher's website PubMed | Google Scholar. doi:10.3390/ijms17020175
Claridge, B., Lozano, J., Poh, Q. H., and Greening, D. W. (2021). Development of extracellular vesicle therapeutics: challenges, considerations, and opportunities. Front. Cell Dev. Biol. 9, 734720. PMID: 34616741; PMCID: PMC8488228. doi:10.3389/fcell.2021.734720
Colombo, M., Raposo, G., and Théry, C. (2014). Biogenesis, secretion, and intercellular interactions of exosomes and other extracellular vesicles. Annu. Rev. Cell Dev. Biol. 30, 255–289. Epub 2014 Aug 21. PMID: 25288114. Publisher's pub med |Google Scholar |web science. doi:10.1146/annurev-cellbio-101512-122326
Couch, Y., Buzàs, E. I., Di Vizio, D., Gho, Y. S., Harrison, P., Hill, A. F., et al. (2021). A brief history of nearly EV-erything - the rise and rise of extracellular vesicles. J. Extracell. Vesicles 10 (14), e12144. PMID: 34919343; PMCID: PMC8681215. See on: Publisher's website PubMed | Google Scholar. doi:10.1002/jev2.12144
Coughlan, C., Bruce, K. D., Burgy, O., Boyd, T. D., Michel, C. R., Garcia-Perez, J. E., et al. (2020). Exosome isolation by ultracentrifugation and precipitation and techniques for downstream analyses. Curr. Protoc. Cell Biol. 88 (1), e110. PMID: 32633898; PMCID: PMC8088761. PMID: 27305142; PMCID: PMC4909321. View at: Publisher Site pub med | Google Scholar. doi:10.1002/cpcb.110
Dad, H. A., Gu, T. W., Zhu, A. Q., Huang, L. Q., and Peng, L. H. (2021). Plant exosome-like nanovesicles: emerging therapeutics and drug delivery nanoplatforms. Mol. Ther. 29 (1), 13–31. Epub 2020 Dec 3. PMID: 33278566; PMCID: PMC7791080. Publisher's website |PubMed | Google Scholar. doi:10.1016/j.ymthe.2020.11.030
Dai, S., Wei, D., Wu, Z., Zhou, X., Wei, X., Huang, H., et al. (2008). Phase I clinical trial of autologous ascites-derived exosomes combined with GM-CSF for colorectal cancer. Mol. Ther. 16, 782–790. [PMC free article] [PubMed] [CrossRef] [Google Scholar]. doi:10.1038/mt.2008.1
Del Conde, I., Shrimpton, C. N., Thiagarajan, P., and López, J. A. (2005). Tissue-factor-bearing microvesicles arise from lipid rafts and fuse with activated platelets to initiate coagulation. Blood 106 (5), 1604–1611. Epub 2005 Mar 1. PMID: 15741221. Publisher's pub med |Google Scholar. doi:10.1182/blood-2004-03-1095
Deng, Z., Rong, Y., Teng, Y., Mu, J., Zhuang, X., Tseng, M., et al. (2017). Broccoli-derived nanoparticle inhibits mouse colitis by activating dendritic cell AMP-activated protein kinase. Mol. Ther. 25, 1641–1654. [PMC free article] [PubMed] [CrossRef] [Google Scholar] [Ref list]. doi:10.1016/j.ymthe.2017.01.025
Dignat-George, F., and Boulanger, C. M. (2011). The many faces of endothelial microparticles. Arterioscler. Thromb. Vasc. Biol. 31 (1), 27–33. [PMID:21160065;Crossref], [PubMed], [Web of Science ®], [Google Scholar]. doi:10.1161/ATVBAHA.110.218123
Ding, Y., Wang, J., Lai, J. H. C., Chan, V. H. L., Wang, X. F., Cai, Y., et al. (2014). Exo70E2 is essential for exocyst subunit recruitment and EXPO formation in both plants and animals. Mol. Biol. Cell 25, 412–426. doi:10.1091/mbc.e13-10-0586
Dinicola, S., Cucina, A., Antonacci, D., and Bizzarri, M. (2014). Anticancer effects of grape seed extract on human cancers: A review. J. Carcinog. Mutagen. Publisher's website | Google Scholar. doi:10.4172/2157-2518.S8-005
Duan, L., Xu, L., Xu, X., Qin, Z., Zhou, X., Xiao, Y., et al. (2021). Exosome-mediated delivery of gene vectors for gene therapy. Nanoscale 13 (3), 1387–1397. Publisher's website |PubMed | Google Scholar |. doi:10.1039/d0nr07622h
Duijvesz, D., Versluis, C. Y., van der Fels, C. A., Vredenbregt-van den Berg, M. S., Leivo, J., Peltola, M. T., et al. (2015). Immuno-based detection of extracellular vesicles in urine as diagnostic marker for prostate cancer: extracellular vesicles as marker for PCa. Int. J. Cancer 137 (12), 2869–2878. Epub 2015 Jul 16. PMID: 26139298. Publisher's Pub med |Google Scholar. doi:10.1002/ijc.29664
Esmaeili, A., Alini, M., Baghaban Eslaminejad, M., and Hosseini, S. (2022). Engineering strategies for customizing extracellular vesicle uptake in a therapeutic context. Stem Cell Res. Ther. 13, 129. Publisher's website |PubMed | Google Scholar |. doi:10.1186/s13287-022-02806-2
Fu, B., Ma, H., and Liu, D. (2020). Extracellular vesicles function as bioactive molecular transmitters in the mammalian oviduct: an inspiration for optimizing in vitro culture systems and improving delivery of exogenous nucleic acids during preimplantation embryonic development. Int. J. Mol. Sci. 21, 2189. Publisher's website| Google Scholar. doi:10.3390/ijms21062189
Gámez-Valero, A., Monguió-Tortajada, M., Carreras-Planella, L., Franquesa, M., Beyer, K., and Borràs, F. E. (2016). Size-Exclusion Chromatography-based isolation minimally alters Extracellular Vesicles' characteristics compared to precipitating agents. Sci. Rep. 6, 33641. PMID: 27640641; PMCID: PMC5027519. See on: Publisher's website |PubMed | Google Scholar | web science. doi:10.1038/srep33641
Garaeva, L., Kamyshinsky, R., Kil, Y., Varfolomeeva, E., Verlov, N., Komarova, E., et al. (2021). Delivery of functional exogenous proteins by plant-derived vesicles to human cells in vitro. Sci. Rep. 11 (1), 6489. PMID: 33753795; PMCID: PMC7985202. Publisher's website |PubMed | Google Scholar. doi:10.1038/s41598-021-85833-y
Giacobino, C., Canta, M., Fornaguera, C., Borrós, S., and Cauda, V. (2021). Extracellular vesicles and their current role in cancer immunotherapy. Cancers 13, 2280. Publisher's website |PubMed | Google Scholar |. doi:10.3390/cancers13092280
Gill, S., Catchpole, R., and et Forterre, P. (2019). Extracellular membrane vesicles in the three domains of life and beyond. Microbiol. FEMS. Rév. 43, 273–303. See on: Publisher's website PubMed | Google Scholar. doi:10.1093/femsre/fuy042
Gomez, I., Ward, B., Souilhol, C., Recarti, C., Ariaans, M., Johnston, J., et al. (2020). Neutrophil microvesicles drive atherosclerosis by delivering miR-155 to atheroprone endothelium. Nat. Commun. 11 (1), 214. PMID: 31924781; PMCID: PMC6954269. See on: Publisher's website PubMed | Google Scholar. doi:10.1038/s41467-019-14043-y
Gould, S. J., and Raposo, G. (2013). As we wait: coping with an imperfect nomenclature for extracellular vesicles. J. Extracell. Vesicles 2, 20389. Voir sur : Site de l’éditeur | Google Scholar. doi:10.3402/jev.v2i0.20389
Grange, C., Skovronova, R., Marabese, F., and Bussolati, B. (2019). Stem cell-derived extracellular vesicles and kidney regeneration. Cells 8, 1240. [PMC free article] [PubMed] [CrossRef] [Google Scholar]. doi:10.3390/cells8101240
Gurunathan, S., Kang, M. H., Jeyaraj, M., Qasim, M., and Kim, J. H. (2019). Review of the isolation, characterization, biological function, and multifarious therapeutic approaches of exosomes. Cells 8 (4), 307. doi:10.3390/cells8040307
Ha, D., Yang, N., and Nadithe, V. (2016). Exosomes as therapeutic drug carriers and delivery vehicles across biological membranes: current perspectives and future challenges. Acta Pharm. Sin. B 6 (4), 287–296. Epub 2016 Mar 8. PMID: 27471669; PMCID: PMC4951582. See on: Publisher's website PubMed | Google Scholar. doi:10.1016/j.apsb.2016.02.001
Halperin, W., and Jensen, W. A. (1967). Ultrastructural changes during growth and embryogenesis in carrot cell cultures. J. Ultrastruct. Res. 18 (3), 428–443. PMID: 6025110. See on: Publisher's website PubMed | Google Scholar. doi:10.1016/s0022-5320(67)80128-x
Hanson, P. I., and Cashikar, A. (2012). Multivesicular body morphogenesis. Annu. Rev. Cell Dev. Biol. 28, 337–362. Epub 2012 Jul 20. PMID: 22831642. Publisher's pub med |Google Scholar. doi:10.1146/annurev-cellbio-092910-154152
He, B., Cai, Q., Qiao, L., Huang, C. Y., Wang, S., Miao, W., et al. (2021). RNA-binding proteins contribute to small RNA loading in plant extracellular vesicles. Nat. Plants 7, 342–352. doi:10.1038/s41477-021-00863-8
Herrmann, I. K., Wood, M. J. A., and Fuhrmann, G. (2021). Extracellular vesicles as a next-generation drug delivery platform. Nat. Nanotechnol. 16, 748–759. doi:10.1038/s41565-021-00931-2
Hosseini-Giv, N., Basas, A., Hicks, C., El-Omar, E., El-Assaad, F., and Hosseini-Beheshti, E. (2022). Bacterial extracellular vesicles and their novel therapeutic applications in health and cancer. Front. Cell Infect. Microbiol. 12, 962216. PMID: 36439225; PMCID: PMC9691856. See on: Publisher's website PubMed | Google Scholar. doi:10.3389/fcimb.2022.962216
Hristov, M., Erl, W., Linder, S., and Weber, P. C. (2004). Apoptotic bodies from endothelial cells enhance the number and initiate the differentiation of human endothelial progenitor cells in vitro. Blood 104, 2761–2766. PubMed |CrossRef |Google Scholar. doi:10.1182/blood-2003-10-3614
Hung, W. L., Suh, J. H., and Wang, Y. (2017). Chemistry and health effects of furanocoumarins in grapefruit. J. Food Drug Anal. 25 (1), 71–83. Epub 2016 Dec 6. PMID: 28911545; PMCID: PMC9333421. Publisher's website |PubMed | Google Scholar. doi:10.1016/j.jfda.2016.11.008
Jayachandran, M., Miller, V. M., Heit, J. A., and Owen, W. G. (2012). Methodology for isolation, identification, and characterization of microvesicles in peripheral blood. J. Immunol. Methods 375, 207–214. PMC Open Article| PubMed |CrossRef |Google Scholar. doi:10.1016/j.jim.2011.10.012
Jayasinghe, M. K., Tan, M., Peng, B., Yang, Y., Sethi, G., Pirisinu, M., et al. (2021). New approaches in extracellular vesicle engineering for improving the efficacy of anti-cancer therapies. Semin. Cancer Biol. 74, 62–78. Epub 2021 Feb 17. PMID: 33609665. Publisher's website |PubMed | Google Scholar |. doi:10.1016/j.semcancer.2021.02.010
Jensen, William A. (1965). The ultrastructure and histochemistry of the synergids of cotton. Am. J. Bot. 52 (3), 238–256. See on: Publisher's website PubMed | Google Scholar. doi:10.1002/j.1537-2197.1965.tb06781.x
Jimenez, J. J., Jy, W., Mauro, L. M., Soderland, C., Horstman, L. L., and Ahn, Y. S. (2003). Endothelial cells release phenotypically and quantitatively distinct microparticles in activation and apoptosis. Thromb. Res. 109 (4), 175–180. PMID: 12757771. Publisher's pub med |Google Scholar. doi:10.1016/s0049-3848(03)00064-1
Jo, W., Jeong, D., Kim, J., Cho, S., Jang, S. C., Han, C., et al. (2014). Microfluidic fabrication of cell-derived nanovesicles as endogenous RNA carriers. Lab. Chip 14, 1261–1269. Publisher's website |PubMed | Google Scholar |. doi:10.1039/c3lc50993a
Ju, S., Mu, J., Dokland, T., Zhuang, X., Wang, Q., Jiang, H., et al. (2013). Grape exosome-like nanoparticles induce intestinal stem cells and protect mice from DSS-induced colitis. Mol. Ther. 21 (7), 1345–1357. Epub 2013 Jun 11. PMID: 23752315; PMCID: PMC3702113. Publisher's website |PubMed | Google Scholar. doi:10.1038/mt.2013.64
Ju, Y., Hu, Y., Yang, P., Xie, X., and Fang, B. (2022). Extracellular vesicle-loaded hydrogels for tissue repair and regeneration. Mater Today Bio 18, 100522. PMID: 36593913; PMCID: PMC9803958. doi:10.1016/j.mtbio.2022.100522
Kameli, N., Dragojlovic-Kerkache, A., Savelkoul, P., and Stassen, F. R. (2021). Plant-derived extracellular vesicles: current findings, challenges, and future applications. Membr. (Basel) 11 (6), 411. PMID: 34072600; PMCID: PMC8226527. Publisher's website |PubMed | Google Scholar. doi:10.3390/membranes11060411
Kim, D. K., and Rhee, W. J. (2021). Antioxidative effects of carrot-derived nanovesicles in cardiomyoblast and neuroblastoma cells. Pharmaceutics 13 (8), 1203. PMCID: PMC8400689. See on: Publisher's website |PubMed | Google Scholar. doi:10.3390/pharmaceutics13081203
Kim, J., Li, S., Zhang, S., and Wang, J. (2022). Plant-derived exosome-like nanoparticles and their therapeutic activities. Asian J. Pharm. Sci. 17 (1), 53–69. Epub 2021 Jul 10. PMID: 35261644; PMCID: PMC8888139. Publisher's website |PubMed | Google Scholar | web science. doi:10.1016/j.ajps.2021.05.006
Kim, J., Jayaprakasha, G. K., Uckoo, R. M., and Patil, B. S. (2012). Evaluation of chemopreventive and cytotoxic effect of lemon seed extracts on human breast cancer (MCF-7) cells. Food Chem. Toxicol. 50, 423–430. [Google Scholar] [CrossRef] [PubMed]. doi:10.1016/j.fct.2011.10.057
Kim, K., Yoo, H. J., Jung, J. H., Lee, R., Hyun, J. K., Park, J. H., et al. (2020). Cytotoxic effects of plant sap-derived extracellular vesicles on various tumor cell types. J. Funct. Biomater. 11 (2), 22. PMID: 32252412; PMCID: PMC7353476. See on: Publisher's website |PubMed | Google Scholar | Web science. doi:10.3390/jfb11020022
Knox, K. W., Vesk, M., and Work, E. (1966). Relation between excreted lipopolysaccharide complexes and surface structures of a lysine-limited culture of Escherichia coli. J. Bacteriol. 92 (4), 1206–1217. PMID: 4959044; PMCID: PMC276396. See on: Publisher's website PubMed | Google Scholar. doi:10.1128/jb.92.4.1206-1217.1966
Kobayashi-Sun, J., Yamamori, S., Kondo, M., Kuroda, J., Ikegame, M., Suzuki, N., et al. (2020). Uptake of osteoblast-derived extracellular vesicles promotes the differentiation of osteoclasts in the zebrafish scale. Commun. Biol. 3, 190. [CrossRef]. doi:10.1038/s42003-020-0925-1
Kocholata, M., Maly, J., Martinec, J., and Auer Malinska, H. (2022). Plant extracellular vesicles and their potential in human health research, the practical approach. Physiol. Res. 71 (3), 327–339. PMID: 35904344; PMCID: PMC9470093. doi:10.33549/physiolres.934886
Kooijmans, S., Fliervoet, L., Van Der Meel, R., Fens, M., Heijnen, H., van Bergen en Henegouwen, P. M. P., et al. (2016). PEGylated and targeted extra-cellular vesicles display enhanced cell specificity and circulation time. J. Control. Release 224, 77–85. Publisher's website |PubMed | Google Scholar |. doi:10.1016/j.jconrel.2016.01.009
Lacroix, R., Vallier, L., Bonifay, A., Simoncini, S., Mege, D., Aubert, M., et al. (2019). Microvesicles and cancer associated thrombosis. Semin. Thromb. Hemost. 45 (6), 593–603. Epub 2019 Aug 20. See on: Publisher's website PubMed | Google Scholar. doi:10.1055/s-0039-1693476
Lakhter, A. J., and Sims, E. K. (2015). Minireview: emerging roles for extracellular vesicles in diabetes and related metabolic disorders. Mol. Endocrinol. 29 (11), 1535–1548. Epub 2015 Sep 22. PMID: 26393296; PMCID: PMC4627606. See on: Publisher's website PubMed. doi:10.1210/me.2015-1206
Lee, C., Mitsialis, S. A., Aslam, M., Vitali, S. H., Vergadi, E., Konstantinou, G., et al. (2012). Exosomes mediate the cytoprotective action of mesenchymal stromal cells on hypoxia-induced pulmonary hypertension. Circulation 126, 2601–2611. Publisher's website |PubMed | Google Scholar |. doi:10.1161/circulationaha.112.114173
Lee, J. K., Park, S. R., Jung, B. K., Jeon, Y. K., Lee, Y. S., Kim, M. K., et al. (2013). Exosomes derived from mesenchymal stem cells suppress angiogenesis by down-regulating VEGF expression in breast cancer cells. PLOS ONE 8, e84256. Publisher's website |PubMed | Google Scholar |. doi:10.1371/journal.pone.0084256
Lee, R., Ko, H. J., Kim, K., Sohn, Y., Min, S. Y., Kim, J. A., et al. (2019). Anti-melanogenic effects of extracellular vesicles derived from plant leaves and stems in mouse melanoma cells and human healthy skin. J. Extracell. Vesicles 9 (1), 1703480. PMCID: PMC6968621. Publisher's website |PubMed | Google Scholar. doi:10.1080/20013078.2019.1703480
Li, A., Li, D., Gu, Y., Liu, R., Tang, X., Zhao, Y., et al. (2023a). Plant-derived nanovesicles: further exploration of biomedical function and application potential. Acta Pharm. Sin. B. See on: Publisher's website |PubMed | Google Scholar. doi:10.1016/j.apsb.2022.12.0222211-3835
Li, B., Antonyak, M. A., Zhang, J., and Cerione, R. A. (2012). RhoA triggers a specific signaling pathway that generates transforming microvesicles in cancer cells. Oncogene 31 (45), 4740–4749. Epub 2012 Jan 23. PMID: 22266864; PMCID: PMC3607381. Publisher's pub med |Google Scholar. doi:10.1038/onc.2011.636
Li, G., Chen, T., Dahlman, J., Eniola-Adefeso, L., Ghiran, I. C., Kurre, P., et al. (2023b). Current challenges and future directions for engineering extracellular vesicles for heart, lung, blood and sleep diseases. J. Extracell. Vesicles 12 (2), e12305. Erratum in: J Extracell Vesicles. 2023 Mar;12(3):e12314. PMID: 36775986; PMCID: PMC9923045. doi:10.1002/jev2.12305
Li, J., Liu, K., Liu, Y., Xu, Y., Zhang, F., Yang, H., et al. (2013). Exosomes mediate the cell-to-cell transmission of IFN-α-induced antiviral activity. Nat. Immunol. 14, 793–803. doi:10.1038/ni.2647
Li, Z., Wang, H., Yin, H., Bennett, C., Zhang, H. G., and Guo, P. (2018). Arrowtail RNA for ligand display on ginger exosome-like nanovesicles to systemic deliver siRNA for cancer suppression. Sci. Rep. 8 (1), 14644. PMID: 30279553; PMCID: PMC6168523. Publisher's website |PubMed | Google Scholar. doi:10.1038/s41598-018-32953-7
Lian, M. Q., Chng, W. H., Liang, J., Yeo, H. Q., Lee, C. K., Belaid, M., et al. (2022). Plant-derived extracellular vesicles: recent advancements and current challenges on their use for biomedical applications. J. Extracell. Vesicles 11 (12), e12283. PMID: 36519808; PMCID: PMC9753580. See on: Publisher's website PubMed. doi:10.1002/jev2.12283
Linares, R., Tan, S., Gounou, C., and Brisson, A. R. (2017). Imaging and quantification of extracellular vesicles by transmission electron microscopy. Methods Mol. Biol. 1545, 43–54. PMID: 27943206. See on: Publisher's website PubMed | Google Scholar. doi:10.1007/978-1-4939-6728-5_4
Liu, N. J., Bao, J. J., Wang, L. J., and Chen, X. Y. (2020a). Arabidopsis leaf extracellular vesicles in wound-induced jasmonate accumulation. Plant Signal Behav. 15, 1833142–1833145. doi:10.1080/15592324.2020.1833142
Liu, S., Ren, J., and Ten Dijke, P. (2021). Targeting TGFβ signal transduction for cancer therapy. Signal Transduct. target Ther. 6 (1), 8. PMID: 33414388; PMCID: PMC7791126.Publisher's website| Google Scholar. doi:10.1038/s41392-020-00436-9
Liu, Y., Wu, S., Koo, Y., Yang, A., Dai, Y., Khant, H., et al. (2020b). Characterization of and isolation methods for plant leaf nanovesicles and small extracellular vesicles. Nanomedicine 29, 102271. Epub 2020 Jul 21. PMID: 32702466. See on: Publisher's website |PubMed | Google Scholar | Web science. doi:10.1016/j.nano.2020.102271
Liu, Y. C., Chen, W. L., Kung, W. H., and Huang, H. D. (2017). Plant miRNAs found in human circulating system provide evidences of cross kingdom RNAi. BMC Genomics 18 (2), 112. PMID: 28361700; PMCID: PMC5374554. Publisher's website |PubMed | Google Scholar. doi:10.1186/s12864-017-3502-3
Lobb, R. J., Becker, M., Wen Wen, S., Wong, C. S. F., Wiegmans, A. P., Leimgruber, A., et al. (2015). Optimized exosome isolation protocol for cell culture supernatant and human plasma. J. Extracell. Vesicles 4, 27031. See on: Article ID 27031Publisher's website |PubMed | Google Scholar. doi:10.3402/jev.v4.27031
Lozano-Ramos, I., Bancu, I., Oliveira-Tercero, A., Armengol, M. P., Menezes-Neto, A., Del Portillo, H. A., et al. (2015). Size-exclusion chromatography-based enrichment of extracellular vesicles from urine samples. J. Extracell. Vesicles 4, 27369. PMID: 26025625; PMCID: PMC4449362. Publisher's website |PubMed | Google Scholar. doi:10.3402/jev.v4.27369
Marar, C., Starich, B., and Wirtz, D. (2021). Extracellular vesicles in immunomodulation and tumor progression. Nat. Immunol. 22, 560–570. Publisher's website |PubMed | Google Scholar |. doi:10.1038/s41590-021-00899-0
Mathivanan, S., Fahner, C. J., Reid, G. E., and Simpson, R. J. (2012). ExoCarta 2012: database of exosomal proteins, RNA and lipids. Nucleic Acids Res. 40, D1241–D1244. PMC free article |PubMed |CrossRef |Google Scholar. doi:10.1093/nar/gkr828
Mathivanan, S., Ji, H., and Simpson, R. J. (2010). Exosomes: extracellular organelles important in intercellular communication. J. Proteomics 73 (10), 1907–1920. Epub 2010 Jul 1. See on: Publisher's website PubMed | Google Scholar. doi:10.1016/j.jprot.2010.06.006
McConnell, R. E., Higginbotham, J. N., Shifrin, D. A., Tabb, D. L., Coffey, R. J., and Tyska, M. J. (2009). The enterocyte microvillus is a vesicle-generating organelle. J. Cell Biol. 185 (7), 1285–1298. PMID: 19564407; PMCID: PMC2712962. Publisher's pub med. doi:10.1083/jcb.200902147
Mendt, M., Kamerkar, S., Sugimoto, H., McAndrews, K. M., Wu, C. C., Gagea, M., et al. (2018). Generation and testing of clinical-grade exosomes for pancreatic cancer. JCI. Perspicacité 3, e99263. [PMC free article] [PubMed] [CrossRef] [Google Scholar]. doi:10.1172/jci.insight.99263
Metzger, B. T., Barnes, D. M., and Reed, J. D. (2008). Purple carrot (Daucus carota L.) polyacetylenes decrease lipopolysaccharide-induced expression of inflammatory proteins in macrophage and endothelial cells. J. Agric. Food Chem. 56 (10), 3554–3560. Epub 2008 Apr 24. PMID: 18433135. Publisher's website |PubMed | Google Scholar. doi:10.1021/jf073494t
Minciacchi, V. R., Freeman, M. R., and Di Vizio, D. (2015). Extracellular vesicles in cancer: exosomes, microvesicles and the emerging role of large oncosomes. Semin. Cell Dev. Biol. 40, 41–51. Epub 2015 Feb 23. PMID: 25721812; PMCID: PMC4747631. Publisher's Pub med |Google Scholar. doi:10.1016/j.semcdb.2015.02.010
Mittelbrunn, M., and Sanchez-Madrid, F. (2010). Intercellular communication: diverse structures for exchange ´ of genetic information. Nat. Rev. Mol. Cell Biol. 13, 328–335. doi:10.1038/nrm3335
Monguió-Tortajada, M., Gálvez-Montón, C., Bayes-Genis, A., Roura, S., and Borràs, F. E. (2019). Extracellular vesicle isolation methods: rising impact of size-exclusion chromatography. Cell. Mol. Life Sci. 76, 2369–2382. See on: Publisher's website PubMed. doi:10.1007/s00018-019-03071-y
Mu, J., Zhuang, X., Wang, Q., Jiang, H., Deng, Z. B., Wang, B., et al. (2014). Interspecies communication between plant and mouse gut host cells through edible plant derived exosome-like nanoparticles. Mol. Nutr. Food Res. 58 (7), 1561–1573. Epub 2014 May 19. Erratum in: Mol Nutr Food Res. 2016 Apr;60(4):964. PMID: 24842810; PMCID: PMC4851829. Publisher's website |PubMed | Google Scholar. doi:10.1002/mnfr.201300729
Muller, L., Hong, C. S., Stolz, D. B., Watkins, S. C., and Whiteside, T. L. (2014). Isolation of biologically-active exosomes from human plasma. J. Immunol. Methods 411, 55–65. Epub 2014 Jun 18. PMID: 24952243; PMCID: PMC4260336. Publisher's website |PubMed. doi:10.1016/j.jim.2014.06.007
Munagala, R., Aqil, F., Jeyabalan, J., and Gupta, R. C. (2016). Bovine milk derived exosomes for drug delivery. Cancer Lett. 371, 48–61. CAS article PubMed Google Scholar. doi:10.1016/j.canlet.2015.10.020
Muralidharan-Chari, V., Clancy, J., Plou, C., Romao, M., Chavrier, P., Raposo, G., et al. (2009). ARF6-regulated shedding of tumor cell-derived plasma membrane microvesicles. Curr. Biol. 19 (22), 1875–1885. Epub 2009 Nov 5. PMID: 19896381; PMCID: PMC3150487. Publisher's pub med |Google Scholar. doi:10.1016/j.cub.2009.09.059
Nabhan, J. F., Hu, R., Oh, R. S., Cohen, S. N., and Lu, Q. (2012). Formation and release of arrestin domain-containing protein 1-mediated microvesicles (ARMMs) at plasma membrane by recruitment of TSG101 protein. Proc. Natl. Acad. Sci. U. S. A. 109 (11), 4146–4151. Epub 2012 Feb 6. PMID: 22315426; PMCID: PMC3306724. Publisher's pub med |Google Scholar. doi:10.1073/pnas.1200448109
Nassar, W., El-Ansary, M., Sabry, D., Mostafa, M. A., Fayad, T., Kotb, E., et al. (2016). Umbilical cord mesenchymal stem cells derived extracellular vesicles can safely ameliorate the progression of chronic kidney diseases. Biomater. Res. 20, 21. [Google Scholar] [CrossRef] [PubMed] [Green Version]. doi:10.1186/s40824-016-0068-0
Nemati, M., Singh, B., Mir, R. A., Nemati, M., Babaei, A., Ahmadi, M., et al. (2022). Plant-derived extracellular vesicles: A novel nanomedicine approach with advantages and challenges. Cell Commun. Signal 20 (1), 69. PMID: 35606749; PMCID: PMC9128143. Publisher's pub med |Google Scholar |web science. doi:10.1186/s12964-022-00889-1
O'Brien, K., Breyne, K., Ughetto, S., Laurent, L. C., and Breakefield, X. O. (2020). RNA delivery by extracellular vesicles in mammalian cells and its applications. Nat. Rev. Mol. Cell Biol. 21, 585–606. [CrossRef]. doi:10.1038/s41580-020-0251-y
Otang, W. M., and Afolayan, A. J. (2016). Antimicrobial and antioxidant efficacy of Citrus limon L. peel extracts used for skin diseases by Xhosa tribe of Amathole District, Eastern Cape, South Africa. S. Afr. J. Bot. 102, 46–49. [Google Scholar] [CrossRef]. doi:10.1016/j.sajb.2015.08.005
Palette, N., Sirois, I., Bell, C., Hanafi, L. A., Hamelin, K., Dieudé, M., et al. (2013). A comprehensive characterization of membrane vesicles released by autophagic human endothelial cells. Proteomics 13 (7), 1108–1120. PMID:23436686[Crossref], [PubMed], [Web of Science ], [Google Scholar]. doi:10.1002/pmic.201200531
Parhiz, H., Roohbakhsh, A., Soltani, F., Rezaee, R., and Iranshahi, M. (2015). Antioxidant and anti-inflammatory properties of the citrus flavonoids hesperidin and hesperetin: an updated review of their molecular mechanisms and experimental models: hesperidin and hesperetin as antioxidant and anti-inflammatory agents. Phyther. Res. 29, 323–331. [Google Scholar] [CrossRef] [PubMed]. doi:10.1002/ptr.5256
PCOS (2021). Plant exosomes and patients diagnosed with polycystic ovary syndrome (PCOS) 17-full text view-ClinicalTrials.gov. Available online: https://clinicaltrials.gov/ct2/show/NCT03493984 (Accessed on May 6, 2021).
Pegtel, D. M., and Gould, S. J. (2019). Exosomes. Annu. Rev. Biochem. 88, 487–514. Publisher's pub med |Google Scholar |web science. doi:10.1146/annurev-biochem-013118-111902
Pérez-Bermúdez, P., Blesa, J., Soriano, J. M., and Marcilla, A. (2017). Extracellular vesicles in food: experimental evidence of their secretion in grape fruits. Eur. J. Pharm. Sci. 98, 40–50. Epub 2016 Sep 21. PMID: 27664331. Publisher's website PubMed |Google Scholar. doi:10.1016/j.ejps.2016.09.022
Perut, F., Roncuzzi, L., Avnet, S., Massa, A., Zini, N., Sabbadini, S., et al. (2021). Strawberry-derived exosome-like nanoparticles prevent oxidative stress in human mesenchymal stromal cells. Biomolecules 11 (1), 87. PMID: 33445656; PMCID: PMC7828105. Publisher's website |PubMed | Google Scholar. doi:10.3390/biom11010087
Piccin, A., Murphy, W. G., and Smith, O. P. (2007). Circulating microparticles: pathophysiology and clinical implications. Blood Rev. 21 (3), 157–171. Epub 2006 Nov 22. PMID: 17118501. Publisher's pub med |Google Scholar. doi:10.1016/j.blre.2006.09.001
Pinedo, M., de la Canal, L., and Lousa, M. (2021). A call for rigor and standardization in plant extracellular vesicle research. J. Extracell. Vesicles 10, 1–8. 49. Baldrich P, Rutter BD, Karimi HZ, Podicheti R, Meyers BC, Innes RW. Plant extracellular vesicles contain diverse small RNA species and are enriched in 10- to 17-nucleotide "Tiny" RNAs. Plant Cell 2019;31:315-324. 10.1105/tpc.18.00872. doi:10.1002/jev2.12048
Poon, I. K., Lucas, C. D., Rossi, A. G., and Ravichandran, K. S. (2014). Apoptotic cell clearance: basic biology and therapeutic potential. Nat. Rev. Immunol. 14 (3), 166–180. See on: Publisher's website PubMed | Google Scholar. doi:10.1038/nri3607
Potestà, M., Roglia, V., Fanelli, M., Pietrobono, E., Gismondi, A., Vumbaca, S., et al. (2020). Effect of microvesicles from Moringa oleifera containing miRNA on proliferation and apoptosis in tumor cell lines. Cell Death Discov. 6, 43–17. doi:10.1038/s41420-020-0271-6
Poudyal, H., Panchal, S., and Brown, L. (2010). Comparison of purple carrot juice and β-carotene in a high-carbohydrate, high-fat diet-fed rat model of the metabolic syndrome. Br. J. Nutr. 104 (9), 1322–1332. Epub 2010 Jul 12. PMID: 20619064. Publisher's website |PubMed | Google Scholar. doi:10.1017/S0007114510002308
Raimondo, S., Naselli, F., Fontana, S., Monteleone, F., Lo Dico, A., Saieva, L., et al. (2015). Citrus limon-derived nanovesicles inhibit cancer cell proliferation and suppress CML xenograft growth by inducing TRAIL-mediated cell death. Oncotarget 6 (23), 19514–19527. PMID: 26098775; PMCID: PMC4637302. Publisher's website |PubMed | Google Scholar |CrossRef. doi:10.18632/oncotarget.4004
Rayner, K. J., and Hennessy, E. J. (2013). Extracellular communication via microRNA: lipid particles have a new message. J. Lipid Res. 54 (5), 1174–1181. Epub 2013 Mar 15. PMID: 23505318; PMCID: PMC3622315. See on: Publisher's website PubMed | Google Scholar. doi:10.1194/jlr.R034991
Reátegui, E., van der Vos, K. E., Lai, C. P., Zeinali, M., Atai, N. A., Aldikacti, B., et al. (2018). Engineered nanointerfaces for microfluidic isolation and molecular profiling of tumor-specific extracellular vesicles. Nat. Commun. 9 (1), 175. PMID: 29330365; PMCID: PMC5766611. Publisher's pub med |Google Scholar | web science. doi:10.1038/s41467-017-02261-1
Redman, R. (2012). Ability of the edible plant exosome to prevent oral mucositis associated with chemoradiotherapy treatment of head and neck cancer. Bethesda, MD: United States National Library of Medicine. Clin. Trials. gov [Internet] Publisher's website| Google Scholar Available from: https://clinicaltrials.gov/ct2/show/NCT01668849. Clinical Trials. Gov identifier: NCT01668849.
Reif, S., Elbaum-Shiff, Y., Koroukhov, N., Shilo, I., Musseri, M., and Golan-Gerstl, R. (2020). Cow and human milk-derived exosomes ameliorate colitis in DSS murine model. Nutriments 12 (9), 2589. PMID : 32858892; Numéro PMCID : PMC7551078. See on: Publisher's website |PubMed | Google Scholar | web science. doi:10.3390/nu12092589
Riaz, A., Khan, R. A., Mirza, T., Mustansir, T., and Ahmed, M. (2014). In vitro/in vivo effect of Citrus limon (L. Burm. f.) juice on blood parameters, coagulation and anticoagulation factors in rabbits. Pak. J. Pharm. Sci. 27, 907–915. [Google Scholar] [PubMed].
Robbins, P. D., and Morelli, A. E. (2014). Regulation of immune responses by extracellular vesicles. Nat. Rev. Immunol. 14 (3), 195–208. ; PMCID: PMC4350779. See on: Publisher's website PubMed | Google Scholar. doi:10.1038/nri3622
Rohde, E. V. A., Pachler, K., and Gimona, M. (2019). Manufacturing and characterization of extracellular vesicles from umbilical cord–derived mesenchymal stromal cells for clinical testing. Cytotherapy 21, 581–592. Publisher's website| Google Scholar | CrossRef. doi:10.1016/j.jcyt.2018.12.006
Rome, S. (2019). Biological properties of plant-derived extracellular vesicles. Food Funct. 10 (2), 529–538. Publisher's website |PubMed | Google Scholar. doi:10.1039/c8fo02295j
Rutter, B. D., and Innes, R. W. (2017). Extracellular vesicles isolated from the leaf apoplast carry stress-response proteins. Plant Physiol. 173 (1), 728–741. Epub 2016 Nov 8. PMID: 27837092; PMCID: PMC5210723. See on: Publisher's pub med |Google Scholar. doi:10.1104/pp.16.01253
Sadeghi, S., Tehrani, F. R., Tahmasebi, S., Shafiee, A., and Hashemi, S. M. (2023). Exosome engineering in cell therapy and drug delivery. Inflammopharmacology 31 (1), 145–169. Epub 2023 Jan 7. PMID: 36609717; PMCID: PMC9823267. doi:10.1007/s10787-022-01115-7
Shtam, T. A., Kovalev, R. A., Varfolomeeva, E. Y., Makarov, E. M., Kil, Y. V., and Filatov, M. V. (2013). Exosomes are natural carriers of exogenous siRNA to human cells in vitro. Cell Commun. Signal 11, 88. Publisher's website |PubMed | Google Scholar |. doi:10.1186/1478-811x-11-88
Somiya, M., Yoshioka, Y., and Ochiya, T. (2018). Biocompatibility of highly purified bovine milk- derived extracellular vesicles. J. Extracell. Vesicles 7, 1440132. Online], [Web of Science ®], [Google Scholar]. doi:10.1080/20013078.2018.1440132
Suárez, H., Gámez-Valero, A., Reyes, R., López-Martín, S., Rodríguez, M. J., Carrascosa, J. L., et al. (2017). A bead-assisted flow cytometry method for the semi-quantitative analysis of Extracellular Vesicles. Sci. Rep. 7 (1), 11271. PMID: 28900146; PMCID: PMC5595788. Publisher's pub med |Google Scholar. doi:10.1038/s41598-017-11249-2
Suharta, S., Barlian, A., Hidajah, A. C., Notobroto, H. B., Ana, I. D., Indariani, S., et al. (2021). Plant-derived exosome-like nanoparticles: A concise review on its extraction methods, content, bioactivities, and potential as functional food ingredient. J. Food Sci. 86 (7), 2838–2850. Epub 2021 Jun 20. PMID: 34151426. See on: Publisher's website PubMed | Google Scholar. doi:10.1111/1750-3841.15787
Suresh, A. P., Kalarikkal, S. P., Pullareddy, B., and Sundaram, G. M. (2021). Low pH-based method to increase the yield of plant-derived nanoparticles from fresh ginger rhizomes. ACS Omega 6 (27), 17635–17641. PMID: 34278148; PMCID: PMC8280662. See on: Publisher's website |PubMed | Google Scholar. doi:10.1021/acsomega.1c02162
Takakura, H., Nakao, T., Narita, T., Horinaka, M., Nakao-Ise, Y., Yamamoto, T., et al. (2022). Citrus limon L.-Derived nanovesicles show an inhibitory effect on cell growth in p53-inactivated colorectal cancer cells via the macropinocytosis pathway. Biomédicaments 10, 1352. doi:10.3390/biomedicines10061352
Tamkovich, S. N., Tutanov, O. S., and Laktionov, P. P. (2016). Exosomes: generation, structure, transport, biological activity, and diagnostic application. Biol. Membr. 33 (3), 163–173. | Google Scholar. doi:10.1134/S1990747816020112
Tauro, B. J., Greening, D. W., Mathias, R. A., Ji, H., Mathivanan, S., Scott, A. M., et al. (2012). Comparison of ultracentrifugation, density gradient separation, and immunoaffinity capture methods for isolating human colon cancer cell line LIM1863-derived exosomes. Methods (San Diego, Calif.) 56 (2), 293–304. Publisher's website PubMed | Google Scholar. doi:10.1016/j.ymeth.2012.01.002
Teng, Y., Ren, Y., Sayed, M., Hu, X., Lei, C., Kumar, A., et al. (2018). Plant-derived exosomal MicroRNAs shape the gut microbiota. Cell Host Microbe 24 (5), 637–652.e8. Epub 2018 Oct 25. PMID: 30449315; PMCID: PMC6746408. Publisher's website |PubMed | Google Scholar. doi:10.1016/j.chom.2018.10.001
Théry, C., Ostrowski, M., and Segura, E. (2009). Membrane vesicles as conveyors of immune responses. Nat. Rev. Immunol. 9 (8), 581–593. . PMID: 19498381. See on: Publisher's website PubMed | Google Scholar. doi:10.1038/nri2567
Trajkovic, K., Hsu, C., Chiantia, S., Rajendran, L., Wenzel, D., Wieland, F., et al. (2008). Ceramide triggers budding of exosome vesicles into multivesicular endosomes. Science 319 (5867), 1244–1247. PMID: 18309083. Publisher's Pub med |Google Scholar. doi:10.1126/science.1153124
Trentini, M., Zanotti, F., Tiengo, E., Camponogara, F., Degasperi, M., Licastro, D., et al. (2022). An apple a day keeps the doctor away: potential role of miRNA 146 on macrophages treated with exosomes derived from apples. Biomedicines 10, 415. [PMC free article] [PubMed] [CrossRef] [Google Scholar] [Ref list]. doi:10.3390/biomedicines10020415
van der Pol, E., Coumans, F. A., Grootemaat, A. E., Gardiner, C., Sargent, I. L., Harrison, P., et al. (2014). Particle size distribution of exosomes and microvesicles determined by transmission electron microscopy, flow cytometry, nanoparticle tracking analysis, and resistive pulse sensing. J. Thromb. Haemost. 12 (7), 1182–1192. Epub 2014 Jun 19. PMID: 24818656. Publisher's pub med |Google Scholar. doi:10.1111/jth.12602
Van Deun, J., Mestdagh, P., Sormunen, R., Cocquyt, V., Vermaelen, K., Vandesompele, J., et al. (2014). The impact of disparate isolation methods for extracellular vesicles on downstream RNA profiling. J. Extracell. Vesicles (JEV) 3 (1), 24858. Article ID 24858 See on: Publisher's website | Google Scholar. doi:10.3402/jev.v3.24858
Van Herwijnen, M. J. C., Driedonks, T. A. P., Snoek, B. L., Kroon, A. M. T., Kleinjan, M., Jorritsma, R., et al. (2018a). Abundantly present miRNAs in milk-derived extracellular vesicles are conserved between mammals. Avant Nutr. 5, 81. PMID : 30280098; PMCID : PMC6153340. See on: Publisher's website |PubMed | Google Scholar | web science. doi:10.3389/fnut.2018.00081
Van Herwijnen, M. J. C., Driedonks, T. A. P., Snoek, B. L., Kroon, A. M. T., Kleinjan, M., Jorritsma, R., et al. (2018b). Abundantly present MicroRNAs in milk-derived extracellular vesicles are conserved between mammals. Front. Nutr. 5, 1–6. [CrossRef].
van Niel, G., D'Angelo, G., and Raposo, G. (2018). Shedding light on the cell biology of extracellular vesicles. Nat. Rev. Mol. Cell Biol. 19 (4), 213–228. Epub 2018 Jan 17. PMID: 29339798. Publisher's pub med |Google Scholar. doi:10.1038/nrm.2017.125
Verderio, C., Gabrielli, M., and Giussani, P. (2018). Role of sphingolipids in the biogenesis and biological activity of extracellular vesicles. J. Lipid Res. 59 (8), 1325–1340. Epub 2018 May 31. PMID: 29853528; PMCID: PMC6071771. Publisher's pub med |Google Scholar |web science. doi:10.1194/jlr.R083915
Vislocky, L. M., and Fernandez, M. L. (2010). Biomedical effects of grape products. Nutr. Rev. 68 (11), 656–670. Publisher's website |PubMed | Google Scholar. doi:10.1111/j.1753-4887.2010.00335.x
Walravens, A. S., Smolgovsky, S., Li, L., Kelly, L., Antes, T., Peck, K., et al. (2021). Mechanistic and therapeutic distinctions between cardiosphere-derived cell and mesenchymal stem cell extracellular vesicle non-coding RNA. Sci. Rep. 11, 8666. Publisher's website| Google Scholar. doi:10.1038/s41598-021-87939-9
Wang, B., Zhuang, X., Deng, Z. B., Jiang, H., Mu, J., Wang, Q., et al. (2014). Targeted drug delivery to intestinal macrophages by bioactive nanovesicles released from grapefruit. Mol. Ther. 22, 522–534. doi:10.1038/mt.2013.190
Wang, J. A., Ding, Y., Wang, J. Q., Hillmer, S., Miao, Y. S., Lo, S. W., et al. (2010). EXPO, an exocyst-positive organelle distinct from multivesicular endosomes and autophagosomes, mediates cytosol to cell wall exocytosis in Arabidopsis and tobacco cells. Plant Cell 22, 4009–4030. doi:10.1105/tpc.110.080697
Wang, Q., Zhuang, X., Mu, J., Deng, Z. B., Jiang, H., Zhang, L., et al. (2013). Delivery of therapeutic agents by nanoparticles made of grapefruit-derived lipids. Nat. Commun. 4, 1867. Erratum in: Nat Commun. 2013;4:2358. Zhang, Lifeng [added]. Erratum in: Nat Commun. 2016;7:11347. PMID: 23695661; PMCID: PMC4396627. Publisher's website |PubMed | Google Scholar. doi:10.1038/ncomms2886
Wang, Q. L., Ren, Y., Mu, J. Y., Egilmez, N. K., Zhuang, X. Y., Deng, Z. B., et al. (2015). Grapefruit-derived nanovectors utilize an activated leukocyte tra-ficking pathway to deliver therapeutic agents to inflammatory tumor sites. Cancer Res. 75, 25290. PMID:25883092 Publisher's website |PubMed | Google Scholar. doi:10.1158/0-0504872.CAN-14-3095
Wehman, A. M., Poggioli, C., Schweinsberg, P., Grant, B. D., and Nance, J. (2011). The P4-ATPase TAT-5 inhibits the budding of extracellular vesicles in C. elegans embryos. Curr. Biol. 21 (23), 1951–1959. Epub 2011 Nov 17. PMID: 22100064; PMCID: PMC3237752. Publisher's pub med |Google Scholar. doi:10.1016/j.cub.2011.10.040
Witwer, K. W., Buzás, E. I., Bemis, L. T., Bora, A., Lässer, C., Lötvall, J., et al. (2013). Standardization of sample collection, isolation and analysis methods in extracellular vesicle research. J. Extracell. Vesicles. 2, 20360. PMID: 24009894; PMCID: PMC3760646. Publisher's website |PubMed |Google Scholar. doi:10.3402/jev.v2i0.20360
Wolf, P. (1967). The nature and significance of platelet products in human plasma. Br. J. Haematol. 13 (3), 269–288. PMID: 6025241. See on: Publisher's website PubMed | Google Scholar. doi:10.1111/j.1365-2141.1967.tb08741.x
Wu, K., Xing, F., Wu, S. Y., and Watabe, K. (2017). Extracellular vesicles as emerging targets in cancer: recent development from bench to bedside. Biochim. Biophys. Acta Rev. Cancer 1868 (2), 538–563. Epub 2017 Oct 18. PMID: 29054476; PMCID: PMC5675795. Publisher's website |PubMed | Google Scholar | CrossRef. doi:10.1016/j.bbcan.2017.10.001
Xiao, J., Feng, S., Wang, X., Long, K., Luo, Y., Wang, Y., et al. (2018). Identification of exosome-like nanoparticle-derived microRNAs from 11 edible fruits and vegetables. PeerJ 6, e5186. [PMC free article] [PubMed] [CrossRef] [Google Scholar] [Ref list]. doi:10.7717/peerj.5186
Xie, M. Y., Chen, T., Xi, Q. Y., Hou, L. J., Luo, J. Y., Zeng, B., et al. (2020). Porcine milk exosome miRNAs protect intestinal epithelial cells against deoxynivalenol-induced damage. Biochem. Pharmacol. 175, 113898. Epub 2020 Mar 4. PMID: 32145262. Publisher's website |PubMed | Google Scholar | CrossRef. doi:10.1016/j.bcp.2020.113898
Xin, H., Li, Y., Buller, B., Katakowski, M., Zhang, Y., Wang, X., et al. (2012). Exosome-mediated transfer of miR-133b from multipotent mesenchymal stromal cells to neural cells contributes to neurite outgrowth. Stem Cells 30, 1556–1564. Publisher's website |PubMed | Google Scholar |. doi:10.1002/stem.1129
Xu, Z., Xu, Y., Zhang, K., Liu, Y., Liang, Q., Thakur, A., et al. (2023). Plant-derived extracellular vesicles (PDEVs) in nanomedicine for human disease and therapeutic modalities. J. Nanobiotechnol 21, 114. free article |PubMed |CrossRef |Google Scholar. doi:10.1186/s12951-023-01858-7
Yamamoto, K. R., Alberts, B. M., Benzinger, R., Lawhorne, L., and Treiber, G. (1970). Rapid bacteriophage sedimentation in the presence of polyethylene glycol and its application to large-scale virus purification. Virology 40 (3), 734–744. PMID: 4908735. Publisher's website |PubMed | Google Scholar. doi:10.1016/0042-6822(70)90218-7
Yang, C., Zhang, M., and Merlin, D. (2018a). Advances in plant-derived edible nanoparticle-based lipid nano-drug delivery systems as therapeutic nanomedicines. J. Mater Chem. B 6 (9), 1312–1321. Epub 2018 Jan 29. See on: Publisher's website |PubMed | Google Scholar |CrossRef. doi:10.1039/C7TB03207B
Yang, Y., Hong, Y., Cho, E., Kim, G. B., and Kim, I. S. (2018b). Extracellular vesicles as a platform for membrane-associated therapeutic protein delivery. J. Extracell. Vesicles 7, 1440131. [CrossRef]. doi:10.1080/20013078.2018.1440131
You, J. Y., Kang, S. J., and Rhee, W. J. (2021). Isolation of cabbage exosome-like nanovesicles and investigation of their biological activities in human cells. Bioact. Mater 6 (12), 4321–4332. PMID: 33997509; PMCID: PMC8105599. See on: Publisher's website |PubMed | Google Scholar. doi:10.1016/j.bioactmat.2021.04.023
Yu, L., Deng, Z., Liu, L., Zhang, W., and Wang, C. (2020). Plant-derived nanovesicles: A novel form of nanomedicine. Front. Bioeng. Biotechnol. 8, 584391. PMID: 33154966; PMCID: PMC7591720. Publisher's website |PubMed | Google Scholar | web science. doi:10.3389/fbioe.2020.584391
Yuyama, K., Sun, H., Sakai, S., Mitsutake, S., Okada, M., Tahara, H., et al. (2014). Decreased amyloid-β pathologies by intracerebral loading of glycosphingolipid-enriched exosomes in alzheimer model mice. J. Biol. Chem. 289 (35), 24488–24498. EPUB 2014 Jul 18. PMID: 25037226; PMCID: PMC4148874. doi:10.1074/jbc.M114.577213
Yuyama, K., Sun, H., Usuki, S., Sakai, S., Hanamatsu, H., Mioka, T., et al. (2015). A potential function for neuronal exosomes: sequestering intracerebral amyloid-β peptide. FEBS Lett. 589, 84–88. [PMID:25436414;Crossref], [PubMed], [Web of Science ®], [Google Scholar]. doi:10.1016/j.febslet.2014.11.027
Zeringer, E., Barta, T., Li, M., and Vlassov, A. V. (2015). Strategies for isolation of exosomes. Cold Spring Harb. Protoc. 2015 (4), pdb.top074476–323. See on: Publisher's website |PubMed | Google Scholar. doi:10.1101/pdb.top074476
Zhang, J., Khan, S. A., Heckel, D. G., and Bock, R. (2017). Next-generation insect-resistant plants: RNAi-mediated crop protection. Trends Biotechnol. 35, 871–882. doi:10.1016/j.tibtech.2017.04.009
Zhang, M., Viennois, E., Prasad, M., Zhang, Y., Wang, L., Zhang, Z., et al. (2016b). Edible ginger-derived nanoparticles: A novel therapeutic approach for the prevention and treatment of inflammatory bowel disease and colitis-associated cancer. Biomaterials 101, 321–340. Epub 2016 Jun 9. PMID: 27318094; PMCID: PMC4921206. Publisher's website |PubMed | Google Scholar. doi:10.1016/j.biomaterials.2016.06.018
Zhang, M., Viennois, E., Xu, C., and Merlin, D. (2016a). Plant-derived edible nanoparticles as a new therapeutic approach against diseases. Tissue Barriers 4 (2), e1134415. PMCID: PMC4910829. See on: Publisher's website PubMed | Google Scholar. doi:10.1080/21688370.2015.1134415
Zheng, D., Ruan, H., Chen, W., Zhang, Y., Cui, W., Chen, H., et al. (2022). Advances in extracellular vesicle functionalization strategies for tissue regeneration. Bioact. Mater 25, 500–526. PMID: 37056271; PMCID: PMC10087114. doi:10.1016/j.bioactmat.2022.07.022
Zhu, H., and He, W. (2023). Ginger: A representative material of herb-derived exosome-like nanoparticles. Front. nut 10, 1223349. PMID: 37521414; PMCID: PMC10374224. Publisher's website |PubMed | Google Scholar. doi:10.3389/fnut.2023.1223349
Zhuang, X., Deng, Z. B., Mu, J., Zhang, L., Yan, J., Miller, D., et al. (2015). Ginger-derived nanoparticles protect against alcohol-induced liver damage. J. Extracell. Vesicles 4, 28713. PMID: 26610593; PMCID: PMC4662062. Publisher's website |PubMed | Google Scholar. doi:10.3402/jev.v4.28713
Zhuang, X., Teng, Y., Samykutty, A., Mu, J., Deng, Z., Zhang, L., et al. (2016). Grapefruit-derived nanovectors delivering therapeutic miR17 through an intranasal route inhibit brain tumor progression. Mol. Ther. 24, 96–105. Publisher's website |PubMed | Google Scholar. doi:10.1038/mt.2015.188
Zitvogel, L., Regnault, A., Lozier, A., Wolfers, J., Flament, C., Tenza, D., et al. (1998). Eradication of established murine tumors using a novel cell-free vaccine: dendritic cell-derived exosomes. Nat. Med. 4 (5), 594–600. PMID: 9585234. Publisher's pub med |Google Scholar. doi:10.1038/nm0598-594
Żmigrodzka, M., Guzera, M., Miśkiewicz, A., Jagielski, D., and Winnicka, A. (2016). The biology of extracellular vesicles with focus on platelet microparticles and their role in cancer development and progression. Tumour Biol. 37 (11), 14391–14401. Epub 2016 Sep 15. PMID: 27629289; PMCID: PMC5126185. See on: Publisher's website PubMed | Google Scholar. doi:10.1007/s13277-016-5358-6
Keywords: extracellular vesicle, therapeutics, microvesicles, nanovesicles, exosomes, apoptotic bodies, plant, mammal
Citation: Sall IM and Flaviu TA (2023) Plant and mammalian-derived extracellular vesicles: a new therapeutic approach for the future. Front. Bioeng. Biotechnol. 11:1215650. doi: 10.3389/fbioe.2023.1215650
Received: 02 May 2023; Accepted: 16 August 2023;
Published: 13 September 2023.
Edited by:
Reza Shahbazi, Indiana University Bloomington, United StatesReviewed by:
Danyang Li, Sun Yat-Sen University, ChinaCopyright © 2023 Sall and Flaviu. This is an open-access article distributed under the terms of the Creative Commons Attribution License (CC BY). The use, distribution or reproduction in other forums is permitted, provided the original author(s) and the copyright owner(s) are credited and that the original publication in this journal is cited, in accordance with accepted academic practice. No use, distribution or reproduction is permitted which does not comply with these terms.
*Correspondence: Tabaran Alexandru Flaviu, YWxleGFuZHJ1LnRhYmFyYW5AdXNhbXZjbHVqLnJv
Disclaimer: All claims expressed in this article are solely those of the authors and do not necessarily represent those of their affiliated organizations, or those of the publisher, the editors and the reviewers. Any product that may be evaluated in this article or claim that may be made by its manufacturer is not guaranteed or endorsed by the publisher.
Research integrity at Frontiers
Learn more about the work of our research integrity team to safeguard the quality of each article we publish.