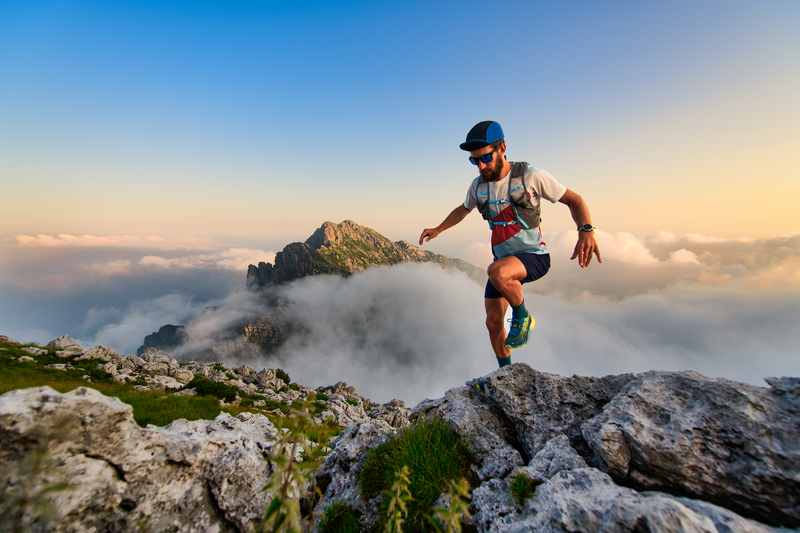
95% of researchers rate our articles as excellent or good
Learn more about the work of our research integrity team to safeguard the quality of each article we publish.
Find out more
ORIGINAL RESEARCH article
Front. Bioeng. Biotechnol. , 23 June 2023
Sec. Bioprocess Engineering
Volume 11 - 2023 | https://doi.org/10.3389/fbioe.2023.1212044
Syngas fermentation processes with acetogens represent a promising process for the reduction of CO2 emissions alongside bulk chemical production. However, to fully realize this potential the thermodynamic limits of acetogens need to be considered when designing a fermentation process. An adjustable supply of H2 as electron donor plays a key role in autotrophic product formation. In this study an anaerobic laboratory scale continuously stirred tank reactor was equipped with an All-in-One electrode allowing for in-situ H2 generation via electrolysis. Furthermore, this system was coupled to online lactate measurements to control the co-culture of a recombinant lactate-producing Acetobacterium woodii strain and a lactate-consuming Clostridium drakei strain to produce caproate. When C. drakei was grown in batch cultivations with lactate as substrate, 1.6 g·L−1 caproate were produced. Furthermore, lactate production of the A. woodii mutant strain could manually be stopped and reinitiated by controlling the electrolysis. Applying this automated process control, lactate production of the A. woodii mutant strain could be halted to achieve a steady lactate concentration. In a co-culture experiment with the A. woodii mutant strain and the C. drakei strain, the automated process control was able to dynamically react to changing lactate concentrations and adjust H2 formation respectively. This study confirms the potential of C. drakei as medium chain fatty acid producer in a lactate-mediated, autotrophic co-cultivation with an engineered A. woodii strain. Moreover, the monitoring and control strategy presented in this study reinforces the case for autotrophically produced lactate as a transfer metabolite in defined co-cultivations for value-added chemical production.
Fermentation processes with syngas have been proposed in the past as a promising technology to reduce the accumulation of carbon dioxide (CO2) in the atmosphere and thus prevent negative effects of rapid climate change (Latif et al., 2014; Bengelsdorf and Dürre, 2017). Among the many microorganisms which can grow using hydrogen (H2), carbon monoxide (CO) and CO2 via hydrogenesis, methanogenesis and acetogenesis (Dürre and Eikmanns, 2015), acetogens show a variety of promising industrial application possibilities (Abubackar et al., 2011; Liew et al., 2016; Liew et al., 2022). Acetobacterium woodii is one of these microorganisms which can use CO2 as sole carbon source, reducing it via the Wood-Ljungdahl pathway to C1 and C2 compounds such as formate, acetate or ethanol (Drake et al., 2008). A. woodii has been studied extensively in the past (Balch et al., 1977; Poehlein et al., 2012; Beck et al., 2020) and is considered to be a model acetogen for sodium bioenergetics (Biegel and Müller, 2010). The main natural product of A. woodii is acetate. It has been genetically accessible since 1994 (Strätz et al., 1994) and recombinant strains have been constructed for the production of C3 compounds like acetone (Hoffmeister et al., 2016) and lactate (Mook et al., 2022). Recently, lactate has drawn special attention for being a promising feed compound to produce higher-value products via chain elongation (Detman et al., 2019; Liu et al., 2020). Caproic acid is an important chemical which can be produced via chain elongation from lactate (Kucek et al., 2016a; Zhu et al., 2017). It has a wide array of applications in the food industry as precursor for aromas (Desbois, 2012), in the cosmetic and pharmaceutical industry and even as possible precursor for biofuels (Cavalcante et al., 2017). Given that the production of caproic acid is currently mainly fossil-based, its sustainable production through fermentation of waste products is gaining increased interest (Wasewar and Shende, 2011). Among the bacteria which can produce caproate are Clostridium kluyveri, Clostridium carboxidivorans, and Clostridium drakei (Wirth and Dürre, 2021), the latter which can also grow on lactate as primary carbon source (Küsel et al., 2000). The production of caproate in C. drakei happens via reverse ß-oxidation. The bcd/hcs gene cluster and its respective enzymes catalyze the elongation of acetyl-CoA to butyryl-CoA and subsequently to hexanoyl-CoA from which caproate is then derived. C. drakei has already been successfully proven to produce caproate from lactate in a co-cultivation with A. woodii (Herzog et al., 2022) and showed great potential for caproate production from CO2.
While syngas fermentation processes could contribute to the reduction of CO2 by reusing this waste component as primary carbon-source for the production of industrial chemicals, they also require H2 as reduction equivalent (Wood, 1991). H2 demand added up to 94 Mt worldwide in 2021 and is still almost entirely produced from fossil sources, mainly coal and natural gas. The associated CO2 emissions of H2 production added up to 900 Mt in 2021. H2 will be a critical element in the energy transition and an important technology towards a climate friendly industry. It is estimated that in 2030 only 24 Mt could be produced from low-emission sources (IEA, 2022). This implies that for the near future the great majority of H2 will be produced from fossil sources. To ensure a true low-emission syngas fermentation, H2 would have to either be produced from gasification of biomass (Abubackar et al., 2011) or via electrolysis powered by renewable energy. A promising technology to produce H2 directly in the bioreactor is the All-in-One (AiO) electrode (Utesch and Zeng, 2018). This highly flexible electrode can be inserted in any standard bioreactor to apply in situ electrolysis during the running fermentation (Utesch and Zeng, 2018). This AiO-electrode has been successfully used for the control and optimization of electricity-aided microbial production of 1,3-propanediol and lipids (Utesch et al., 2019; Arbter et al., 2022). Powering this electrode with electricity from renewable sources could also turn the syngas fermentation into a true low-emission process.
The aim of this work was the characterization of C. drakei growing on lactate as carbon source in a stirred-tank bioreactor and proving the feasibility of a lactate based-process control. First, we identified the lactate consumption and caproate production rates of C. drakei grown on lactate. Furthermore, we designed and programmed a lactate-based process control which can be applied in any lactate producing fermentation. Applying this to the co-culture process of C. drakei and A. woodii (Herzog et al., 2022) the lactate production rate of A. woodii can be adjusted to the maximum lactate consumption rate of C. drakei determined through a dynamic operation of the AiO-electrode. This control should minimize H2 production to the required minimum and prevent an excess waste of energy and H2. In times of high energy prices, minimizing energy costs plays a major role in economic feasibility of a new process.
Construction of A. woodii [PbgaL_ldhD_NFP] is described in Mook et al. (2022) while the C. drakei wild-type strain was obtained from the German Collection of Microorganisms (DSMZ 12750). Cultivation medium for both strains was prepared according to Herzog et al. (2022). For C. drakei cultivations, 10 g·L−1 DL-lactate was added as carbon source to the medium. Pre-cultures of A. woodii [PbgaL_ldhD_NFP] were cultivated heterotrophically using fructose (2 g·L−1) at 30°C in non-agitated anaerobic serum bottles. C. drakei pre-cultures were cultivated under the same conditions with the exception of using a 75:25 ratio (v/v) of lactate and fructose. The pre-cultures were incubated for 30–33 h until reaching an optical density at 600 nm (OD600) of 3.3 ± 0.4 for C. drakei and 2.0 ± 0.9 for A. woodii [PbgaL_ldhD_NFP].
Batch fermentations were carried out in a 2.0 L stirred-tank bioreactor (KSF 2000, Bioengineering AG, Wald, Switzerland) with a working volume of 1.4 L. The medium was sterilized in situ at 121°C for 20 min before each fermentation. Then, the cultivation medium was degassed with N2 to ensure anaerobic conditions. An oxygen reduction potential (ORP) sensor was installed to ensure an ORP value lower than −280 mV prior to inoculation. After degassing, the reactor was sparged constantly with CO2 at a gas flow rate of 0.9 L·h−1 with an open tube L-sparger. A. woodii [PbgaL_ldhD_NFP] cultures were agitated with three Rushton disk turbines (d = 40 mm) at a stirrer speed of 800 rpm (P V−1 = 2.4 W·L−1), while C. drakei culture were agitated at 210 rpm (P V−1 = 0.04 W·L−1). The temperature was controlled at 30°C and pH was measured via a pH sensor and controlled at pH 7.0 ± 0.2 by the addition of a 5 M KOH solution. H2 for the fermentations with A. woodii [PbgaL_ldhD_NFP] was supplied by the AiO-electrode via in situ electrolysis. The rod-shaped electrode consists of a platinized titan mesh as working electrode (platin coating thickness d = 2.5 µm, coating density ρ = 50 g·m-2) on the outside where H2 is produced and which is in contact with the fermentation medium. The working electrode is separated from the counter electrode by a ceramic separator, creating a counter electrode chamber with an exhaust duct at the top. This enables the produced O2 to leave the bioreactor without interfering with the cultivation medium. The working electrode surface is 75 cm2 while the counter electrode surface is 14 cm2 (Utesch and Zeng, 2018). A scheme of the AiO-electrode is shown in the Supplementary Figure S1. The AiO-electrode was operated without reference electrode to maintain a constant current of 600 mA (j = 8 mA·cm−2, Ecell = 4.2 ± 0.3 V) by a power supply (2231A-30-3; Keithley, Solon, OH, United States). C. drakei cultivations were conducted without the AiO-electrode. The OD600 of the pre-culture was used to calculate the necessary inoculation volume to start the fermentations to an OD600 of 0.15 for A. woodii [PbgaL_ldhD_NFP] and 0.2 for C. drakei respectively. For A. woodii [PbgaL_ldhD_NFP] cultivations, the production of lactate was induced with the addition of 0.3 g·L−1 lactose. Induction was conducted when the batch culture had reached an OD600 of 0.48 ± 0.13. The online lactate measurement was carried out with a TRACE C2 Control (TRACE Analytics GmbH, Braunschweig, Germany) using a dialysis probe with a membrane for low lactate concentrations. The automated current adjustment for H2 production by the AiO-electrode was regulated by a LabVIEW script containing a proportional-integral-derivate controller (PID) algorithm. The process variable lactate concentration (g·L−1) was transmitted with 4-20 mA via an analog digital converter (USB-6001; National Instruments Corp., Austin, TX, United States) to the host computer. The control variable was the electrical current (A) applied to the AiO-electrode by the power supply via RS232 communication. The set point was the desired lactate concentration. The derivative value of the controller was set to 0 while proportional gain was set to 0.01 and the integral time to 2 min with a sampling time of 10 ms. For co-cultivations, A. woodii [PbgaL_ldhD_NFP] was inoculated first into the bioreactor and grown under the same conditions as in the pure culture experiments (T = 30°C; pH = 7.0; P V−1 = 2.4 W·L−1; FCO2 = 0.9 L·h−1; IAiO = 600 mA; V0 = 1.4 L) until 0.4 g·L−1 of lactate were produced. The automated process control was programmed to switch off the AiO-electrode at this lactate concentration. Subsequently, 90 mL of a C. drakei pre-culture were added to the bioreactor. Cell growth was determined with OD600 measurements (photospectrometry), while lactate, acetate, butyrate and formate concentrations were determined via HPLC. The exhaust gas composition and flow were monitored constantly with a mass flow meter (EL-FLOW prestige, Bronkhorst High-Tech B.V., Ruurlo, Netherlands) and a mass spectrometer (Omnistar GDS 300, Pfeiffer Vacuum GmbH, Asslar, Germany). All analytical methods are described in detail in Herzog et al. (2022). The experimental plan of the fermentations conducted in this study is shown in Table 1.
C. drakei was cultivated in a stirred tank reactor as described in Chapter 2.2 with lactate being the sole substrate. The cultivation was reproduced (n = 2) and average values with standard deviation are mentioned in this section. Representative data of only one fermentation are shown in Figure 1 (refer to Supplementary Figure S2 in the additional dataset). C. drakei cells grew with a growth rate of 0.04 h−1 in the first 15 h to an OD600 of 0.39 ± 0.12 and subsequently entered stationary phase. This phase lasted 16 h where the average OD600 added up to 0.41 ± 0.03. Afterwards, the C. drakei culture entered a second growth phase with µ = 0.04 h−1 which lasted for 20 h reaching an OD600 of 0.93 ± 0.08 after 49 h of fermentation time. Subsequently, the culture was stationary for the remaining 17 h of the process at a constant OD600 of 0.96 ± 0.04. Lactate concentrations in the medium started decreasing with the start of the fermentation. In the first 21 h, lactate was consumed at a rate of 0.06 g·L−1 h−1, reducing the initial concentration of 10.7 g·L−1 by 11% to 9.5 g·L−1. Then, at the end of the first stationary growth phase, the lactate consumption rate almost doubled to 0.11 g·L−1 h−1 and during the second exponential growth phase it reached a maximum value of 0.24 g·L−1 h−1. After 66 h of fermentation time, lactate concentration decreased to 0.13 ± 0.18 g·L−1. Acetate was produced throughout the fermentation at a constant rate of 0.06 g·L−1 h−1 and reached a final concentration of 4.53 ± 0.53 g·L−1. Butyrate was produced at a similar rate as acetate during the first 15 h. Afterwards, the production rate increased to 0.15 g·L−1 h−1, reaching a peak concentration of 1.57 ± 0.65 g·L−1 after 21 h of fermentation time. In the following 8 h, butyrate concentration decreased to 1.26 ± 0.27 g·L−1 due to reassimilation, before increasing again with a production rate of 0.10 g·L−1 h−1 until 47 h fermentation time. The concentration then increased further to a maximum of 3.14 ± 0.08 g·L−1 after 64 h with a reduced rate of 0.04 g·L−1 h−1. Caproate concentrations were detected in the medium after 23 h of fermentation. The caproate production rate added up to 0.01 g·L−1 h−1 in the first 31 h of fermentation time. Afterwards, the production rate increased to 0.06 g·L−1 h−1 between 39 h and an estimated 58 h of fermentation time. The maximum concentration of 1.56 g·L−1 was measured after 64 h when the culture had already become stationary.
FIGURE 1. Stirred-tank batch cultivation of C. drakei with lactate as substrate. (A) Optical cell density (OD600, orange circles), lactate concentration measured in the medium (blue triangles); (B) acetate concentration (cyan squares), butyrate concentration (green triangles), caproate concentration measured in the medium (red diamonds). (T = 30°C; pH = 7.0; P V−1 = 0.04 W·L−1; FCO2 = 0.9 L·h−1; V0 = 1.4 L).
The concept of controlling the lactate production of A. woodii [PbgaL_ldhD_NFP] by dynamic on-off switching of the AiO-electrode raises the question regarding the impact of a H2 limited phase on the lactate metabolism of A. woodii [PbgaL_ldhD_NFP]. Specifically, it is unclear whether the lactate metabolism of A. woodii [PbgaL_ldhD_NFP] would be halted permanently or if A. woodii [PbgaL_ldhD_NFP] would resume lactate production when H2 is present in the medium again. To answer this question, a controlled batch fermentation with the AiO-electrode was conducted where 10 h after induction, the AiO-electrode was manually turned off for an interval of 6 h. It was then turned on again to see whether lactate production is resumed. For comparison, a reference batch fermentation with A. woodii [PbgaL_ldhD_NFP] where the AiO-electrode was left on for the whole process time was also conducted (refer to Figure 2A). Both cultivations were inoculated from a pre-culture with an OD600 of 2.5 ± 0.3 and started growing with the maximum growth rate of 0.07 h−1. The reference fermentation reached the stationary phase after 26 h and stayed at an average OD600 of 0.85 ± 0.02 for the rest of the fermentation. The manually controlled cultivation entered stationary phase already after 25 h, at an OD600 of 0.62 ± 0.01 and stayed afterwards at an average OD600 of 0.64 ± 0.02 until the end of the fermentation. The course of the lactate concentration over time is shown in Figure 2B. The lactate production of the reference fermentation did not start until 6 h after induction. Then, lactate was produced constantly at a maximum rate of 0.05 g·h−1 until 25.5 h after induction, reaching a concentration of 0.60 g·L−1. Subsequently, the lactate production rate decreased to 0.01 g·h−1. The maximum lactate concentration of 0.69 g·L−1 was reached after 66 h of fermentation time. The lactate formation of the controlled fermentation began 2 h after induction with a rate of 0.02 g·h−1 and 8 h later, the production halted at a concentration of 0.10 g·L−1 due to switching off the AiO-electrode. The concentration stayed constant at an average of 0.11 ± 0.01 g·L−1 during the 6 h when no electrolysis was taking place. Afterwards, the lactate concentration increased at a rate of 0.02 g·h−1 for 8 h, reaching a concentration of 0.24 g·L−1. For the last 26 h of the controlled fermentation, the lactate concentration stayed constant at an average of 0.25 ± 0.02 g·L−1. The measured acetate concentration is shown in Figure 2C. The reference fermentation produced acetate constantly at a rate of 0.17 g·h−1 for 46 h. Then, the production rate decreased to 0.04 g·h−1 adding up to a final concentration of 6.8 g·L−1. The acetate concentration of the controlled fermentation increased in the beginning of the fermentation with a production rate of 0.11 g·h−1 until the AiO-electrode was switched off. Afterwards, the acetate concentration stayed constant at an average of 2.26 ± 0.11 g·L−1. In the last 26 h of the process, the acetate concentration increased slowly at a rate of 0.03 g·h−1, adding up to a final value of 3.26 g·L−1. The course of the formate concentration over time is depicted in Figure 2D. In the reference fermentation, almost no formate was accumulated in the first 42 h of the process (average 0.09 ± 0.01 g·L−1). Subsequently, formate concentration rapidly increased with a constant rate of 0.10 g·h−1 adding up to a final concentration of 1.67 g·L−1. The concentration of formate in the controlled cultivation increased at the beginning to an average of 0.51 ± 0.06 g·L−1 until the AiO-electrode was switched off. Then, all formate was consumed during the first 2 h without active electrolysis. Afterwards, formate accumulated at a rate of 0.15 g·h−1 during the 8 h after the AiO-electrode was reactivated. Subsequently, formate production slowed down, adding up to a final value of 1.58 g·L−1.
FIGURE 2. Stirred-tank batch cultivations of A. woodii [PbgaL_ldhD_NFP] with manually controlled AiO-electrode as well as reference cultivation. (A) Optical cell density (OD600), reference cultivation in light orange circles and manual AiO-electrode control fermentation in darker orange circles. (B) lactate concentration measured in the medium, reference fermentation in light blue triangles, controlled fermentation in dark blue triangles; (C) acetate concentration; reference fermentation in light cyan squares and controlled fermentation in dark cyan squares; (D) formate concentration measured in the medium, reference fermentation in light purple triangles and controlled fermentation in dark purple triangles manual. The dotted line indicates the time point of induction of the manually controlled fermentation while the dashed line indicates the time point of induction of the reference fermentation. Both cultivations were induced with the addition of 0.3 g·L−1 lactose. The grey bar represents the time interval when the AiO-electrode was turned off and then on again. (T = 30°C; pH = 7.0; P V−1 = 2.4 W·L−1; FCO2 = 0.9 L·h−1; IAiO = 600 mA; V0 = 1.4 L).
A co-cultivation of A. woodii [PbgaL_ldhD_NFP] and C. drakei was conducted to test the automated process control for feasibility. For this, the lactate threshold of the automation was set to 0.35 g·L−1 and C. drakei addition was timed 1.5 h after the process control had turned off the AiO-electrode. The threshold value was chosen to be lower than the average lactate concentration of 0.47 ± 0.11 g·L−1 based on previous data of fermentations with A. woodii [PbgaL_ldhD_NFP] and the AiO-electrode. The results of the co-cultivation are shown in Figure 3. Cell growth of the A. woodii [PbgaL_ldhD_NFP] strain increased after about 7 h with a maximum growth rate of 0.1 h−1. At an OD600 of 0.5 the cells were induced with 0.3 g·L−1 of lactose for lactate production and afterwards, the cell growth declined to 0.02 h−1. C. drakei was added to the bioreactor at an OD600 of 0.65, and afterwards, the combined OD600 of both strains kept increasing until reaching a final value of 1.0 after 88 h. After induction the A. woodii [PbgaL_ldhD_NFP] strain started producing lactate with a maximum formation rate of 0.03 g·h−1. As seen in Figure 3B, the lactate measurement was recalibrated after 38 h due to deviation to a control measurement performed with HPLC. After 42.5 h, the measurement system detected lactate concentrations higher than the threshold and therefore, the AiO-electrode was automatically turned off. Due to residues of lactate in the pre-culture of C. drakei, the concentration increased slightly at the beginning of the co-cultivation phase. Furthermore, the lactate measurement system showed strong fluctuations subsequently which triggered the process control to turn on the AiO-electrode again, after 45.5 h and made a recalibration necessary. Subsequently, lactate concentrations decreased due to its consumption by C. drakei with a rate of 0.02 g·h−1. Caproate concentrations were first detected after 63 h and C. drakei kept producing caproate at a rate of 0.01 g·h−1. Final caproate concentrations added up to 0.08 g·L−1. Acetate was produced by the A. woodii [PbgaL_ldhD_NFP] strain once it had entered the exponential growth phase reaching 3.8 g·L−-1 when C. drakei was added. Afterwards, both strains contributed to the increase of acetate throughout the subsequent fermentation. Final acetate concentrations added up to of 7.3 g·L−1. Butyrate was produced only during the co-cultivation phase by C. drakei, reaching 0.6 g·L−1 at the end of the fermentation. As shown in Figure 3D, formate was produced by the A. woodii [PbgaL_ldhD_NFP] strain and concentrations reached a first peak after 16 h at 0.4 g·L−1. Subsequently, the A. woodii [PbgaL_ldhD_NFP] strain consumed almost all of the formate before starting to produce again, reaching a second concentration peak at the moment the AiO-electrode was turned off at 0.5 g·L−1. During the period where no H2 was produced, the A. woodii [PbgaL_ldhD_NFP] strain consumed parts of the formate and afterwards started producing it a third time, reaching a maximum concentration of 0.7 g·L−1. During the last 20 h of the process, formate was consumed again. The electrical current of the AiO-electrode was maintained constant at 600 mA until the electrode was turned off by the automation. During the co-cultivation of both strains, O2 started accumulating inside the counter electrode channel, causing an increase in electrical resistance and thus also in electrical voltage. Therefore, the electrical current decreased twice for short intervals, but refilling the counter electrode channel with culture medium resolved the issue. Relevant process performance parameters are listed in Table 2.
FIGURE 3. Stirred-tank batch co-cultivation of A. woodii [PbgaL_ldhD_NFP] and C. drakei with automatically controlled AiO-electrode based on lactate concentration. (A) Optical cell density (OD600, orange circles); (B) lactate concentration measured in the medium with the TRACE C2 control (sample frequency 1 h, blue triangles), caproate concentrations measured in the medium (red diamonds); (C) acetate concentration measured in the medium (cyan squares), butyrate concentration measured in the medium (green triangles); (D) formate concentration measured in the medium (purple triangles), electrical current of the AiO-electrode (magenta line). The dashed line indicates the time point of induction of the culture with 0.3 g·L−1 lactose. The dotted line indicates the time point when C. drakei was added to the culture. The grey bar represents the time interval when the AiO-electrode was turned off automatically. (T = 30°C; pH = 7.0; P V−1 = 2.4 W·L−1; FCO2 = 0.9 L·h−1; IAiO = 600 mA; V0 = 1.4 L; cLac, limit = 0.3 g·L−1).
TABLE 2. Maximum cell density and growth rate, maximum lactate and caproate concentration as well as lactate and caproate formation rates of all fermentations described in this study.
C. drakei has been known to grow on lactate for some time (Küsel et al., 2000; Liou et al., 2005), yet to the authors best knowledge, this is the first study to show data of a stirred tank batch fermentation with C. drakei monoculture on lactate as carbon and energy source. Cell growth showed two growth phases (see Figure 1A), which could suggest the usage of a second substrate by C. drakei during the first growth phase, i.e., components of the yeast extract, given that during this phase only 7% (g g−1) of the lactate was consumed while cell density had already increased 1.8-fold. The maximum lactate consumption rate added up to 7.3 ± 1.7 g d−1 which is 20-fold higher than reported for previous co-cultivation (Herzog et al., 2022). The data also shows that the main caproate production takes place in the second growth phase, with only 17% of the total caproate being produced in the first 31 h of the fermentation and the remaining 83% in the following 35 h. It is assumed that the reduction of butyrate after 21 h of fermentation time is due to the reassimilation of butyrate to butyryl-CoA, possibly using ethanol as electron donor. Butyryl-CoA could have then be used in the chain elongation towards additional caproate. The caproate yield from lactate added up to 13% (g g−1) which is 2.3-fold lower than reported by the CPB6 strain of the Oscillospiraceae family in a similar process (Zhu et al., 2017), however these data were obtained with different bacteria and in a fed-batch fermentation. Data from other caproate producing novel clostridia species closely related to Clostridium jeddahense JCD in single cultures show comparable 18% (g g−1) yield from lactate (Liu et al., 2020). The process was conducted as batch fermentation, though as bottle fermentation and not as fermentation in a bioreactor as the presented results in this study. The volumetric productivity of 0.6 g·L−1 d−1 for caproate is 10-fold higher than reported recently by us in a co-cultivation (Herzog et al., 2022), suggesting that the potential for caproate production in the co-cultivation set up is not yet exhausted. Changing the fermentation mode from batch to fed-batch or continuous mode would help increase the volumetric productivity (Kucek et al., 2016a). Furthermore, the possibility to increase the chain-elongation rate of acetate to butyrate and then to caproate should be investigated, given that caproate adds up to only 23% of the three main products, while acetate accounts for almost double (43%). Testing feeding strategies and operating the process with an in situ product recovery technique might improve overall caproate yield (Kucek et al., 2016a; Kucek et al., 2016b).
To determine if the lactate production of A. woodii [PbgaL_ldhD_NFP] is controllable, the AiO-electrode was switched off for an interval of 6 h during the controlled batch fermentation with A. woodii [PbgaL_ldhD_NFP] (refer to Chapter 3.2). As Figure 2B shows, lactate production was successfully halted for 6 h and resumed after the AiO-electrode was switched on again. Lactate concentration increased 2.1-fold after the halting period at the same formation rate of 0.02 g·h−1 as before, which indicates that a lactate control by adjusting H2 production as proposed is possible. The lactate production afterwards only lasted for 8 h before entering a stationary phase, however a similar trend can be seen in the reference fermentation, where the lactate formation rate was reduced 5-fold at about the same time, 30 h after induction. A comparable effect was also observed in the recently published A. woodii [PbgaL_ldhD_NFP] fermentation with the AiO-electrode, where lactate production stops 26 h after induction (Herzog et al., 2022). The 6 h of H2 limitation influenced cell growth and acetate production as well. Cell density differed on average 23% ± 4% from the reference fermentation while acetate added up to a 52% ± 2% lower concentration in comparison to the reference after the H2 limitation phase. However, the fermentation where the AiO-electrode was temporarily switched off showed lower product rates, as well as an earlier stationary growth phase already before the switching off of the electrode. Acetate formation rate was 1.3-fold and lactate formation rate 2.6-fold lower than compared to the reference fermentation. A possible explanation, next to slight differences in induction, could be, the inherent energy-limited regime for this autotrophic fermentation process. One indicator for energy-limitation in acetogens is the formation of formate, as the formyl-THF synthetase, catalyzing the conversion of formate to formyl-THF is ATP dependent (Moon et al., 2021). Disruption of Na+ homeostasis, and thereby ATP-synthase activity, has led to increased formate production in Thermoanaerobacter kivui (Yang and Drake, 1989) and A. woodii (Schwarz et al., 2022). The A. woodii [PbgaL_ldhD_NFP] culture where the AiO-electrode was regulated accumulated 19 times more formate before the H2 limitation phase than the reference fermentation (see Figure 2D), hinting at a bottleneck in C1 and energy metabolism, even before the electrode was turned off. Interestingly, in the AiO-electrode off phase with no available H2, the accumulated formate was quickly consumed. Oxidation of formate yields CO2 and H2 which probably allow for basic cell maintenance and even some product formation (Moon et al., 2021) as seen with the slightly increased acetate concentration. As H2-supply is reestablished, growth and production of acetate, lactate and formate is immediately resumed with formate production rates of up to 0.15 g·h−1. The reference fermentation neared a comparable formation rate of 0.10 g·h−1 11 h later than the process with manual AiO-electrode control. As the reference fermentation constantly produced lactate, it stands to reason that the ATP-negative production of lactate (Bertsch and Müller, 2015; Mook et al., 2022) in the low H2 process with the AiO-electrode (Herzog et al., 2022) leads to energetic bottlenecks over the course of the fermentation. This in turn results in formate accumulation, which is even more severe and starts earlier when the electrode is turned off mid-fermentation.
In this study we proposed a controlled lactate production in A. woodii [PbgaL_ldhD_NFP] to ideally match the lactate production rate to the maximum lactate consumption rate of C. drakei in a co-culture process. This would decrease H2 production to the necessary minimum and would reduce energy costs greatly. As the data in Figure 3 show, the controlled lactate production was successfully implemented. Once the lactate online measurement detected the defined threshold, the process control turned the AiO-electrode automatically off. As the lactate concentration decreased, the AiO-electrode was turned on again, showing that the process control can react dynamically to changing lactate levels. However, a reliable working online measurement system is necessary for exact automation. Frequent reference measurements and automated calibration cycles should provide more steady data stream. The comparison of the lactate production and consumption rates of A. woodii [PbgaL_ldhD_NFP] (0.03 g·h−1) and C. drakei (0.24 g·h−1) determined in this study indicates that C. drakei has a higher rate of lactate consumption than A. woodii [PbgaL_ldhD_NFP] has in production. It therefore seems that controlling lactate production is not necessary given that C. drakei could consume lactate faster than A. woodii [PbgaL_ldhD_NFP] produces. However, the process with AiO-electrode as described here does not allow H2 production rates higher than 10 mmol·L−1·h−1 at the moment, which results in a H2 limitation of A. woodii [PbgaL_ldhD_NFP] and thus lower lactate production rates. The H2 limitation and the resulting low lactate concentration also caused substrate limitation for C. drakei, resulting in lower caproate concentrations. As reported recently, cultivating A. woodii [PbgaL_ldhD_NFP] with a H2 surplus of 971 mmol·L−1·h−1, the maximum lactate production rate can reach up to 0.21 g·h−1 (Herzog et al., 2022), which demonstrates that more efficient A. woodii [PbgaL_ldhD_NFP] processes would benefit from the lactate process control presented in this study. Furthermore, this maximum lactate uptake rate of C. drakei was only reached in the last third of the fermentation (Figure 1A). In the first 15 h of the process, the lactate uptake rate was 4.9-fold lower. More importantly, in the co-cultivation where the lactate control is supposed to be implemented, lactate uptake rates of C. drakei reached only 0.01 g·h−1 and did not increase although the co-cultivation phase lasted 45 h. This indicates, that during a co-cultivation of C. drakei and A. woodii [PbgaL_ldhD_NFP] with these low lactate concentrations, the lactate process control could reduce the amount of H2 being produced via electrolysis without limiting the availability of lactate for C. drakei, if lactate is already present in the medium.
The datasets presented in this study can be found in online repositories. The names of the repository/repositories and accession number(s) can be found below: Mendeley data, doi: 10.17632/gmjvy88nd5.1 https://data.mendeley.com/datasets/gmjvy88nd5.
JH designed, performed, and analyzed all experiments, drafted, and wrote the manuscript. TU designed and programmed the lactate control in LabVIEW. AM and FB drafted the manuscript and designed experiments. AM, FB, TU, and A-PZ revised the manuscript and made substantial comments. FB and A-PZ proposed and supervised the project. All authors contributed to the article and approved the submitted version.
This research was funded by the Deutsche Forschungsgemeinschaft (DFG, German Research Foundation) as part of the priority program InterZell (SPP 2170). DFG requests the following statement: “gefördert durch die Deutsche Forschungsgemeinschaft. (DFG—427864786)”. Publishing fees supported by Funding Programme Open Access Publishing of Hamburg University of Technology (TUHH).
The authors gratefully thank the Deutsche Forschungsgemeinschaft (DFG, German Research Foundation) for funding the joint research project CaproMix as part of the priority program InterZell (SPP 2170).
The authors declare that the research was conducted in the absence of any commercial or financial relationships that could be construed as a potential conflict of interest.
All claims expressed in this article are solely those of the authors and do not necessarily represent those of their affiliated organizations, or those of the publisher, the editors and the reviewers. Any product that may be evaluated in this article, or claim that may be made by its manufacturer, is not guaranteed or endorsed by the publisher.
The Supplementary Material for this article can be found online at: https://www.frontiersin.org/articles/10.3389/fbioe.2023.1212044/full#supplementary-material
Abubackar, H. N., Veiga, M. C., and Kennes, C. (2011). Biological conversion of carbon monoxide: Rich syngas or waste gases to bioethanol. Biofuels, Bioprod. Bioref. 5, 93–114. doi:10.1002/bbb.256
Arbter, P., Widderich, N., Utesch, T., Hong, Y., and Zeng, A.-P. (2022). Control of redox potential in a novel continuous bioelectrochemical system led to remarkable metabolic and energetic responses of Clostridium pasteurianum grown on glycerol. Microb. Cell Fact. 21, 178. doi:10.1186/s12934-022-01902-5
Balch, W. E., Schoberth, S., Tanner, R. S., and Wolfe, R. S. (1977). Acetobacterium, a new genus of hydrogen-oxidizing, carbon dioxide-reducing, anaerobic bacteria. Int. J. Syst. Bacteriol. 27, 355–361. doi:10.1099/00207713-27-4-355
Beck, M. H., Flaiz, M., Bengelsdorf, F. R., and Dürre, P. (2020). Induced heterologous expression of the arginine deiminase pathway promotes growth advantages in the strict anaerobe Acetobacterium woodii. Appl. Microbiol. Biotechnol. 104, 687–699. doi:10.1007/s00253-019-10248-9
Bengelsdorf, F. R., and Dürre, P. (2017). Gas fermentation for commodity chemicals and fuels. Microb. Biotechnol. 10, 1167–1170. doi:10.1111/1751-7915.12763
Bertsch, J., and Müller, V. (2015). Bioenergetic constraints for conversion of syngas to biofuels in acetogenic bacteria. Biotechnol. Biofuels 8, 210. doi:10.1186/s13068-015-0393-x
Biegel, E., and Müller, V. (2010). Bacterial Na+-translocating ferredoxin:NAD+ oxidoreductase. Proc. Natl. Acad. Sci. U. S. A. 107, 18138–18142. doi:10.1073/pnas.1010318107
Cavalcante, W. d. A., Leitão, R. C., Gehring, T. A., Angenent, L. T., and Santaella, S. T. (2017). Anaerobic fermentation for n-caproic acid production: A review. Process Biochem. 54, 106–119. doi:10.1016/j.procbio.2016.12.024
Desbois, A. P. (2012). Potential applications of antimicrobial fatty acids in medicine, agriculture and other industries. Recent Pat. antiinfect. Drug Discov. 7, 111–122. doi:10.2174/157489112801619728
Detman, A., Mielecki, D., Chojnacka, A., Salamon, A., Błaszczyk, M. K., and Sikora, A. (2019). Cell factories converting lactate and acetate to butyrate: Clostridium butyricum and microbial communities from dark fermentation bioreactors. Microb. Cell Fact. 18, 36. doi:10.1186/s12934-019-1085-1
Drake, H. L., Gössner, A. S., and Daniel, S. L. (2008). Old acetogens, new light. Ann. N. Y. Acad. Sci. 1125, 100–128. doi:10.1196/annals.1419.016
Dürre, P., and Eikmanns, B. J. (2015). C1-carbon sources for chemical and fuel production by microbial gas fermentation. Curr. Opin. Biotechnol. 35, 63–72. doi:10.1016/j.copbio.2015.03.008
Herzog, J., Mook, A., Guhl, L., Bäumler, M., Beck, M. H., Weuster-Botz, D., et al. (2022). Novel synthetic co-culture of Acetobacterium woodii and Clostridium drakei using CO2 and in situ generated H2 for the production of caproic acid via lactic acid. Eng. Life Sci. 23, e2100169. doi:10.1002/elsc.202100169
Hoffmeister, S., Gerdom, M., Bengelsdorf, F. R., Linder, S., Flüchter, S., Öztürk, H., et al. (2016). Acetone production with metabolically engineered strains of Acetobacterium woodii. Metab. Eng. 36, 37–47. doi:10.1016/j.ymben.2016.03.001
Kucek, L. A., Nguyen, M., and Angenent, L. T. (2016a). Conversion of L-lactate into n-caproate by a continuously fed reactor microbiome. Water Res. 93, 163–171. doi:10.1016/j.watres.2016.02.018
Kucek, L. A., Spirito, C. M., and Angenent, L. T. (2016b). High n-caprylate productivities and specificities from dilute ethanol and acetate: Chain elongation with microbiomes to upgrade products from syngas fermentation. Energy Environ. Sci. 9, 3482–3494. doi:10.1039/C6EE01487A
Küsel, K., Dorsch, T., Acker, G., Stackebrandt, E., and Drake, H. L. (2000). Clostridium scatologenes strain SL1 isolated as an acetogenic bacterium from acidic sediments. Int. J. Syst. Evol. Microbiol. 50, 537–546. doi:10.1099/00207713-50-2-537
Latif, H., Zeidan, A. A., Nielsen, A. T., and Zengler, K. (2014). Trash to treasure: Production of biofuels and commodity chemicals via syngas fermenting microorganisms. Curr. Opin. Biotechnol. 27, 79–87. doi:10.1016/j.copbio.2013.12.001
Liew, F. E., Nogle, R., Abdalla, T., Rasor, B. J., Canter, C., Jensen, R. O., et al. (2022). Carbon-negative production of acetone and isopropanol by gas fermentation at industrial pilot scale. Nat. Biotechnol. 40, 335–344. doi:10.1038/s41587-021-01195-w
Liew, F., Martin, M. E., Tappel, R. C., Heijstra, B. D., Mihalcea, C., and Köpke, M. (2016). Gas fermentation - a flexible platform for commercial scale production of low-carbon-fuels and chemicals from waste and renewable feedstocks. Front. Microbiol. 7, 694. doi:10.3389/fmicb.2016.00694
Liou, J. S.-C., Balkwill, D. L., Drake, G. R., and Tanner, R. S. (2005). Clostridium carboxidivorans sp. nov., a solvent-producing clostridium isolated from an agricultural settling lagoon, and reclassification of the acetogen Clostridium scatologenes strain SL1 as Clostridium drakei sp. nov. Int. J. Syst. Evol. Microbiol. 55, 2085–2091. doi:10.1099/ijs.0.63482-0
Liu, B., Popp, D., Müller, N., Sträuber, H., Harms, H., and Kleinsteuber, S. (2020). Three novel clostridia isolates produce n-caproate and iso-butyrate from lactate: Comparative genomics of chain-elongating bacteria. Microorganisms 8, 1970. doi:10.3390/microorganisms8121970
Mook, A., Beck, M. H., Baker, J. P., Minton, N. P., Dürre, P., and Bengelsdorf, F. R. (2022). Autotrophic lactate production from H2 + CO2 using recombinant and fluorescent FAST-tagged Acetobacterium woodii. Appl. Microbiol. Biotechnol. 106, 1447–1458. doi:10.1007/s00253-022-11770-z
Moon, J., Dönig, J., Kramer, S., Poehlein, A., Daniel, R., and Müller, V. (2021). Formate metabolism in the acetogenic bacterium Acetobacterium woodii. Environ. Microbiol. 23, 4214–4227. doi:10.1111/1462-2920.15598
Poehlein, A., Schmidt, S., Kaster, A.-K., Goenrich, M., Vollmers, J., Thürmer, A., et al. (2012). An ancient pathway combining carbon dioxide fixation with the generation and utilization of a sodium ion gradient for ATP synthesis. PLoS ONE 7, e33439. doi:10.1371/journal.pone.0033439
Schwarz, F. M., Moon, J., Oswald, F., and Müller, V. (2022). Biological hydrogen storage and release through multiple cycles of bi-directional hydrogenation of CO2 to formic acid in a single process unit. Joule 6, 1304–1319. doi:10.1016/j.joule.2022.04.020
Strätz, M., Sauer, U., Kuhn, A., and Dürre, P. (1994). Plasmid transfer into the homoacetogen Acetobacterium woodii by electroporation and conjugation. Appl. Environ. Microbiol. 60, 1033–1037. doi:10.1128/aem.60.3.1033-1037.1994
Utesch, T., Sabra, W., Prescher, C., Baur, J., Arbter, P., and Zeng, A.-P. (2019). Enhanced electron transfer of different mediators for strictly opposite shifting of metabolism in Clostridium pasteurianum grown on glycerol in a new electrochemical bioreactor. Biotechnol. Bioeng. 116, 1627–1643. doi:10.1002/bit.26963
Utesch, T., and Zeng, A.-P. (2018). A novel All-in-One electrolysis electrode and bioreactor enable better study of electrochemical effects and electricity-aided bioprocesses. Eng. Life Sci. 18, 600–610. doi:10.1002/elsc.201700198
Wasewar, K. L., and Shende, D. Z. (2011). Reactive extraction of caproic acid using tri-n-butyl phosphate in hexanol, octanol, and decanol. J. Chem. Eng. Data 56, 288–297. doi:10.1021/je100974f
Wirth, S., and Dürre, P. (2021). Investigation of putative genes for the production of medium-chained acids and alcohols in autotrophic acetogenic bacteria. Metab. Eng. 66, 296–307. doi:10.1016/j.ymben.2021.04.010
Wood, H. G. (1991). Life with CO or CO2 and H2 as a source of carbon and energy. FASEB J. 5, 156–163. doi:10.1096/fasebj.5.2.1900793
Yang, H. C., and Drake, H. L. (1989). Differential effects of sodium on hydrogen- and glucose-dependent growth of acetogenic bacterium Acetogenium kivui. Appl. Env. Microbiol. 56, 81–86. doi:10.1128/aem.56.1.81-86.1990
Keywords: Acetobacterium woodii, Clostridium drakei, caproate, lactate, bioelectrochemical system, in situ electrolysis, carbon fixation, process control
Citation: Herzog J, Mook A, Utesch T, Bengelsdorf FR and Zeng A-P (2023) Lactate based caproate production with Clostridium drakei and process control of Acetobacterium woodii via lactate dependent in situ electrolysis. Front. Bioeng. Biotechnol. 11:1212044. doi: 10.3389/fbioe.2023.1212044
Received: 25 April 2023; Accepted: 15 June 2023;
Published: 23 June 2023.
Edited by:
Antonio Marzocchella, University of Naples Federico II, ItalyReviewed by:
Helene De Wever, Flemish Institute for Technological Research (VITO), BelgiumCopyright © 2023 Herzog, Mook, Utesch, Bengelsdorf and Zeng. This is an open-access article distributed under the terms of the Creative Commons Attribution License (CC BY). The use, distribution or reproduction in other forums is permitted, provided the original author(s) and the copyright owner(s) are credited and that the original publication in this journal is cited, in accordance with accepted academic practice. No use, distribution or reproduction is permitted which does not comply with these terms.
*Correspondence: Jan Herzog, amFuLmhlcnpvZ0B0dWhoLmRl; Frank R. Bengelsdorf, ZnJhbmsuYmVuZ2Vsc2RvcmZAdW5pLXVsbS5kZQ==; An-Ping Zeng, YXplQHR1aGguZGU=
Disclaimer: All claims expressed in this article are solely those of the authors and do not necessarily represent those of their affiliated organizations, or those of the publisher, the editors and the reviewers. Any product that may be evaluated in this article or claim that may be made by its manufacturer is not guaranteed or endorsed by the publisher.
Research integrity at Frontiers
Learn more about the work of our research integrity team to safeguard the quality of each article we publish.