- Zymvol Biomodeling SL, Barcelona, Spain
In recent years, simulations have been used to great advantage to understand the structural and dynamic aspects of distinct enzyme immobilization strategies, as experimental techniques have limitations in establishing their impact at the molecular level. In this review, we discuss how molecular dynamic simulations have been employed to characterize the surface phenomenon in the enzyme immobilization procedure, in an attempt to decipher its impact on the enzyme features, such as activity and stability. In particular, computational studies on the immobilization of enzymes using i) nanoparticles, ii) self-assembled monolayers, iii) graphene and carbon nanotubes, and iv) other surfaces are covered. Importantly, this thorough literature survey reveals that, while simulations have been primarily performed to rationalize the molecular aspects of the immobilization event, their use to predict adequate protocols that can control its impact on the enzyme properties is, up to date, mostly missing.
1 Introduction
Enzymes are diverse natural catalysts able to perform a wide range of chemical reactions with high specificity and selectivity. In addition to these inherent properties, their ease of production, sustainability and often low cost compared to several metal catalysts (Sheldon, 2018; Adams et al., 2019; Heckmann and Paradisi, 2020) has been steadily increased the industrial use of enzymes (Choi et al., 2015; Singh R. et al., 2016; Chapman et al., 2018; Abdelraheem et al., 2019; Wackett, 2019; Wu et al., 2021). Due to these advantages, biocatalysis is nowadays applied in a wide variety of sectors, ranging from agrochemicals (Aleu et al., 2006) to textiles (Madhu and Chakraborty, 2017), cosmetics (Sá et al., 2017; Yarosh et al., 2019), commodity chemicals (Woodley, 2020), detergents (Al-Ghanayem and Joseph, 2020), food (Raveendran et al., 2018), leather (Khambhaty, 2020), paper and pulp (Hakala et al., 2013; Singh G. et al., 2016), biomaterials, and (bio)pharmaceutical manufacture (Meghwanshi et al., 2020). However, despite their excellent features, a full exploitation of enzymes’ industrial potential has not been yet achieved. This is due to many reasons like instability in extreme pH, high temperature, presence of surfactants, solvents, or metal ions; short shelf-life; and high substrate concentrations (Sheldon and Woodley, 2018; Silva et al., 2018; Basso and Serban, 2019; Sheldon, 2019). In the last decades, the development of enzyme engineering approaches has aided to overcome these issues while bridging the gap between biocatalysis and industry. Enzyme tuning throughout rational design, directed evolution and, more recently, by the use of in silico tools has been key to speed up the application of enzymes into industry. These efforts aimed to specialize the enzymes towards their target reactions have led to a wide range of examples in which properties like activity, substrate specificity, selectivity and stability have been successfully increased (Chen, 2001; Chowdhury and Maranas, 2020; Nirantar, 2021). Still, these strategies are in some cases not enough to meet the industrial needs, especially when the experimental conditions are too hazardous leading to enzyme inhibition or when the process is not efficient enough due to limitations in enzyme recovery and recycling. Enzyme immobilization has been used as one of the most prominent methods to overcome these flaws and further boost the enzyme’s application (Garcia-Galan et al., 2011; Tran and Balkus, 2011; Zdarta et al., 2018). The term “immobilized enzymes” have been defined as “enzymes physically confined or localized in a certain defined region of space with retention of their catalytic activities, and which can be used repeatedly and continuously” (Brena et al., 2013). This provides various practical advantages which include cost savings, easier reusability, enzyme recovery, generally improved enzyme stability under storage and operating conditions, enhanced activity, optimized selectivity or specificity, etc (Mateo et al., 2007a; 2007b; Barbosa et al., 2013; 2015; Secundo, 2013; Sheldon and van Pelt, 2013; Mohamad et al., 2015; Mehta et al., 2016; Nguyen and Kim, 2017; Bernal et al., 2018; Rajangam et al., 2018; Ramakrishna et al., 2018; Zdarta et al., 2018). The first immobilized enzyme was discovered more than a century ago while demonstrating that activity of an invertase enzyme is not hampered when it is adsorbed on a solid matrix, such as charcoal or an aluminum hydroxide (Nelson and Griffin, 1916). However, it was not until the end of the twentieth century that considerable interest in the combination of enzymes with materials was raised with the path breaking work by Klibanov, Russell, Halling, and others on the preparation and utilization of immobilized enzymes (Klibanov, 1979; 1983; Yang et al., 1995; Zacharis et al., 1997; LeJeune et al., 1998), including strategies such as covalent attachment, encapsulation, adsorption on solid supports, entrapment in polymeric gels, cross-linking and PEGylation. Nowadays, the enzyme immobilization has widely spread and has been used successfully in applications like antibacterial or/and antifouling coatings (Banerjee et al., 2011; Yu et al., 2011), industrial catalysis (Liese and Hilterhaus, 2013), drug delivery (Liu et al., 2009; Liese and Hilterhaus, 2013), biosensors (Rusmini et al., 2007; Talbert and Goddard, 2012), and biofuel cells (Minteer et al., 2007). Despite its potential, immobilizing enzymes is still a growing field and there is no universal method or carrier material used for this purpose. Instead, in accordance with different kinds of enzyme attachment, enzyme immobilization strategies can be classified into physical adsorption, encapsulation, entrapment, cross-linking, covalent attachments, and bioaffinity interactions (Figure 1).
Although immobilization is nowadays widely used and incorporated in standard experimental pipelines, the mechanism of immobilization has not been satisfactorily clarified at the microscopic level owing to the complexity of the immobilizing agent and the molecular nature of the protein-surface interactions. Furthermore, how the immobilization event can ultimately affect the enzymatic efficiency is a complex process which is poorly understood and difficult to rationalize. Molecular simulations can help in deciphering the mechanisms of chemical and biological processes as well as be used for design and development of new products. Hence, with access to highly developed fast computers in recent years, molecular simulation techniques have become a powerful tool to investigate the immobilization phenomenon at molecular level and can act as strategic complement to experiments. Among the available simulation techniques, Molecular Dynamics (MD) simulations, which can disclose the microscopic nature of intermolecular interactions, have been used successfully to investigate the immobilization of enzymes over the last two decades. Some recent reviews have discussed adsorption, the initial step of immobilization, using simulation techniques on various surfaces (Ganazzoli and Raffaini, 2019; Quan et al., 2019). Other reviews have highlighted the MD simulation studies of nanoparticle interactions with proteins and other biomolecules (Khan and Nandi, 2014; Brancolini and Tozzini, 2019; Casalini et al., 2019), or the protein corona, which forms protein layers on the nanoparticle surface (Lee, 2021). However, a detailed description on the use of in silico methods to assess the structural aspects of the enzyme immobilization is lacking.
In this review, we discuss how MD simulations -either all atoms or coarse grained- have been employed to understand the surface phenomenon in the enzyme immobilization procedure, in an attempt to decipher its impact on the enzyme activity. These are presented in this article based upon different types of surfaces on which the immobilization of the enzymes was carried out. The subsequent part of the review begins with discussion of MD simulations performed to study enzyme immobilization on different types of nanoparticles followed by similar works done on Self Assembled Monolayers (SAMs). Works involving graphene or carbon nanotubes are presented thereafter. This is followed by discussion on other surfaces used for enzyme immobilization and have been rationalized by MD simulations. We also briefly discuss surfaces used for immobilization for which simulation studies are lacking, emphasizing possible difficulties in studying such surfaces. These include polymers, metal-organic-framework (MOF) or metal based affinity tags. A thorough literature survey performed for writing this review revealed that, while the distinct studies have been focused on rationalizing the molecular aspects of the immobilization process, the use of computation to predict adequate protocols that can control the impact on the enzyme properties is very limited. The final section of this article discusses the few recent proposals for predicting immobilization protocols using MD simulations and future perspectives in this direction.
2 Immobilization on nanoparticles
The immobilization of enzymes using nanoparticles offers some advantages such as: high enzyme loading, improved enzyme stability, and ease of separation from the reaction products (Cao et al., 2003). Among these, the surface of silica nanoparticles (SNPs) is often used for enzyme immobilization due to the high adsorption capacity of the nanoporous silica particles. One example is the study of the papain enzyme and its adsorption mechanism, that was studied by classical MD simulations (He et al., 2014). The results showed that papain, following initial binding to silica, optimizes its structure which allows more of its atoms to come in contact with the surface reaching the most favored immobilization mode. Although the major secondary structures were preserved, small rearrangements of structures at the entrance of the catalytic site were observed. These led to an increase in the accessibility of the active site for the solvent as well as the substrates, which could therefore facilitate productive binding modes between the substrates and the enzyme. In the same year, Sun et al. used MD simulations to study the orientation and adsorption of three different enzymes, namely, cytochrome c, RNase A and lysozyme on SNPs (Sun et al., 2014b). These enzymes were found to be induced with greater structural stabilization by small SNPs and the results indicated selective interactions between the enzymes and SNPs, where deprotonated silanol groups were used leading to silica with a negative surface charge. Hildebrand et al. (2015) also showed, through the use of MD simulation, that α-chymotrypsin and lysozyme, both positively charged, have preferential binding modes to the amorphous silica based on their surface residues. They showed that α-chymotrypsin, with its α-helical domain turned towards the surface, has a preferred adsorption orientation while lysozyme shows not so clear preference in the orientation. This is due to the fact that α-chymotrypsin has a large dipole moment, leading to preferential adsorption through its positive surface-potential region, while its negative surface-potential region is exposed toward the solvent. However, lysozyme has more homogeneously distributed surface-potential and hence much less pronounced orientational preference. A schematic view of this is shown in Figure 2. In a later work Yu and Zhou. (2016) used coarse grain MD simulation to study the adsorption of lysozyme, which showed a narrow orientation distribution on the silica surface. Interestingly, with increasing nanoparticles size greater conformational changes were observed. This indicates that a decrease in the nanoparticle’s surface curvature may result in a higher degree of electrostatic interactions with the enzyme which can perturb the dynamics of the enzyme. These effects are not due to the area of contact between lysozyme and SNPs. Instead, they are because of the dissimilarities in the interfacial layer of hydration found over the SNPs with different sizes. This is due to the fact that strength of interfacial hydration is inversely proportional to the nanoparticle curvature. Hence, a more ordered distribution of interfacial water molecules is observed. In addition to that, negatively charged SNPs are found to have less effect on the conformation of lysozyme. This phenomenon is more pronounced for larger SNPs at greater ionic strength. In a more recent work, coarse grain MD and constant-pH Monte Carlo (MC) simulations were used to study the lysozyme adsorption on negatively charged SNPs. The results showed that the increase of pH leads to changes in orientation of the adsorbed lysozyme when the solution pH gets closer to the enzyme’s isoelectric point (Caetano et al., 2021). MD simulations were also used by Wang et al. (2020) to tune the immobilization of a lipase on SNPs. Along with structural analysis and catalytic characterizations the results confirmed that the reoriented lipase immobilized through hydrophobic adsorption in its open conformation was crucial for achieving the highest efficiency in the catalytic process.
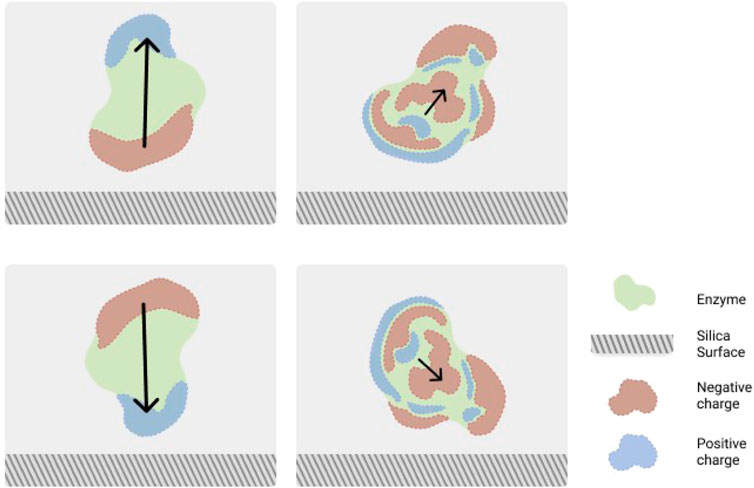
FIGURE 2. Schematic view of most (bottom) and least (top) attractive orientations of chymotrypsin (left) and lysozyme (right) placed over the SiO2 surface. The scheme is based upon Figure 4 from Hildebrand et al., 2015.
Other types of nanoparticles, such as gold-, silver- or titanium-based have been used for enzyme immobilization. All atom MD simulations in combination with absorption, fluorescence and infrared spectroscopy were used to investigate the interaction between silver nanoparticles (AgNPs) and a catechol O-methyltransferase (Usman et al., 2021). The results suggested that AgNPs influence the catalytic activity of the catechol O-methyltransferase by interacting with six amino acids from four of the enzyme’s predominant helical structures which are in close proximity to the active site, and therefore own the potential to control the enzyme features. Baig et al. (2015) studied the immobilization of a yeast alcohol dehydrogenase on polypyrrole–titanium(IV)phosphate (PPy–TiP) nanocomposite. The hydrogen bonding and van der Waals interactions were observed to play an important role in the formation of complexes which are favored energetically. The estimated activity of the enzyme was consistent with the experimental findings. More recently, Tavanti et al. (2019) studied the adsorption of trypsin along with myoglobin and hemoglobin over gold (Au) nanoparticles (AuNPs) using coarse grained MD simulations. No significant conformational changes were observed in this case and the AuNP binding site did not interfere with important functional sites of the enzyme. AuNPs were also prevalently functionalized with organic thiol molecules prior immobilization leading to a self assembled monolayer, which will be discussed in the next section. Although out of the scope of the current review, in the last years, these computational studies have not been limited only to the study of enzymes, but they also have been applied to assess the binding of antibodies, nanobodies and others with nanoparticle surfaces (Xiao et al., 2018; Simões et al., 2021; Martí et al., 2022).
3 Immobilization on self assembled monolayers (SAMs)
Self assembled monolayers (SAMs) are formed by a pool of thiol molecules located over gold surfaces in an organized manner. SAMs offer a perfect platform for studying immobilization of enzymes due to their adjustable structures and ease of functionalization. These properties allow SAMs not only to be used as immobilizing material but also as membrane mimics to better understand the nature of the protein-membrane interactions (Ulman, 1996; Smith et al., 2003; Yeung et al., 2018). One of the earliest in silico studies of a protein interacting with a SAM was performed by Tobias et al. (1996). They investigated the behavior of cytochrome (cyt) c covalently attached with hydrophilic (SH-terminated) and hydrophobic (CH3-terminated) SAMs. The results suggested that the enzyme undergoes minor structural changes when attached to these surfaces. However, the changes are still significant with the enzyme being less spherical when attached to the hydrophilic SAM with the polar surface residues reaching out for making interactions with the SAM surface. Regarding the orientation of the enzyme, it is such that the heme plane is almost parallel to the surface when attached to the hydrophobic SAM, whereas it is nearer to the perpendicular orientation when attached to the hydrophilic surface (Figure 3). Subsequent studies have further investigated the nature of the interactions between cyt c and different types of SAMs (Nordgren et al., 2002; Zhou et al., 2004; Rivas et al., 2005; Xie et al., 2015; Peng et al., 2016). Nordgren et al. (2002) further studied the effect of SAMs in the enzyme properties by introducing several modifications such as tuning the polarity of the SAM end groups, the degree of hydration around the monolayer and the coordination number of the heme iron present on the cyt c. The overall structure of the enzyme was found to be preserved, while the SAM structure was perturbed only in regions with direct contact to the enzyme. The work of Zhou et al. (2004) have shed more light on the orientation of cyt c with respect to negatively charged carboxyl-terminated SAM. They have shown that the most efficient orientation of the enzyme places the heme group perpendicular to the SAM surface. In combination with spectroscopic experiments Rivas et al. (2005) performed MD simulations of cyt c docked into negatively charged SAM via its lysine rich domain. The results from the work suggested that cyt c, upon binding to biomembranes or partner proteins, may have electric-field-induced redox potential shift and hence may affect the biological electron transfer process of cyt c. Xie et al. (2015) performed MD simulations to further probe the modulation of cyt c behaviors on the zwitterionic phosphorylcholine SAMs which are electrically responsive. The results showed that it is possible to regulate enzyme behavior through observing deformation of the enzyme and application of electric fields on responsive surfaces, The behaviors regulated were promotion or retardation of enzyme adsorption and regulation of enzyme orientation. Peng et al. (2016) studied the phosphate and chloride ions effect on the adsorption of cyt c using MD simulations. The results showed unstable adsorption of cyt c on the surface in presence of chloride ions despite relatively high ionic strength. Alternatively, the presence of phosphate ions was found to promote stable adsorption.
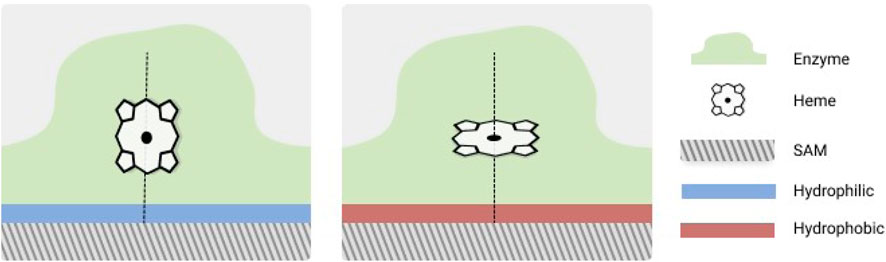
FIGURE 3. Diagrammatic representation of cytochrome c heme group orientation with respect to different SAM surfaces. The scheme is based upon Figure 2 from Zhou et al., 2004, Figure 12 from Xie et al., 2015, Figure 3 from Rivas et al., 2005 and Figure 6 from Peng et al., 2016.
In addition to cyt c, the Zhou group have studied adsorption behaviors of other enzymes upon immobilization on SAMs using a protocol which combines both MC and MD simulations (Xie et al., 2013; 2020; Liu et al., 2015a; Liu et al., 2015b; Zhao et al., 2015; Liu et al., 2017; Yang et al., 2018; Yang et al., 2020). The protocol involves first a quick sampling of the protein over the surface using MC for predicting which is the dominant orientation of the enzyme. Then, MD simulation is used to obtain atomic level adsorption details such as contacting residues, conformational changes, interaction energy, etc. Using this protocol, the adsorption of lysozyme on carboxylated SAMs under the effect of an external electric field was studied by Xie et al. (2013). It was observed that lysozyme is adsorbed with “bottom end-on” or “side-on” orientation in absence of electric fields. However, when an electric field is applied, it preferes “side-on”, “back-on” and “top end-on” orientations. This supported the possibility of tuning the immobilization process of a protein with the desired orientation by applying different electric fields. Zheng et al. (2004), Zheng et al. (2005) studied the interaction of lysozyme with alkanethiolate SAMs terminating with different chemical and observed that the flexibility and the conformation of the SAMs together with bound water near the interface are features responsible for strengthening the interaction between the enzyme and the SAM. A similar observation was made by He et al. (2008) while studying the nature of the lysozyme interactions with zwitterionic phosphorylcholine-terminated SAMs. In a more recent work, Xie et al., 2020 studied the lysozyme adsorption on electrically responsive carboxyl/hydroxyl SAM, further supporting that the behavior of the lysozyme over the SAM surface is affected by the nature of the applied electric field to the system. The combined MC and MD protocol was also used by Liu et al. (2015a), Liu et al. (2015b), Liu et al. (2017) to study the conformation and orientation of a feruloyl esterase, laccase and ribonuclease A (RNase A) adsorbed on SAMs. Two different types of SAMs-positively charged NH2−SAM and negatively charged COOH−SAM-were used for these studies. RNase A was found to adsorb on both the charged SAMs in opposite orientations, i.e., when adsorbed on COOH−SAM RNase A it was oriented towards the surface, while on NH2−SAM, the active site was oriented towards the solution (Liu et al., 2015b). Figure 4 schematically shows this active site orientation with respect to different types of SAMs. Based on these studies, they concluded that the adsorption over negatively charged surfaces could be used to remove the redundant RNase A, while the adsorption over positively charged surfaces favors the enzymatic activity of RNase A. This control of the orientation by using different charged surfaces was also observed for feruloyl esterase from Aspergillus niger (AnFaeA) (Liu et al., 2015a). In addition, ionic strength (IS) and surface charge density (SCD) effects were also considered and the results suggested that positively charged surfaces at high IS and low SCD can maximize the immobilized AnFaeA utilization. In a later work by Liu et al. (2017), it was observed that for a laccase the orientation of the electrodes is very important for achieving a fast direct electron transfer (DET)-and hence to find a small pathway-between the substrate and the T1 copper site during the immobilization of laccase immobilization. They studied the Trametes versicolor laccase (TvL) immobilized on COOH−SAM and NH2−SAM, showing that the T1 copper site of TvL is closer to the positively charged surface. This is due to the TvL orientation on a negatively charged surface being broader in comparison to its orientation on a positively charged surface, which leads to more conductivity in the latter’s case. In contrast, a similar work using bilirubin oxidase showed that negatively charged surfaces were more favorable for the direct electron transfer (Yang et al., 2018). In another separate work, it was shown that the enhanced catalytic activity of a laccase immobilized in SAM was ascribed to high hydrophobic interaction energy (Miyazawa et al., 2017). The combined MC and MD protocol was also used to study Candida antarctica lipase B (CalB) adsorption on hydrophobic graphite surface and hydrophilic TiO2 surface, as well as on both positively and negatively charged (NH2- and COOH-) SAMs (Zhao et al., 2015). The results show that CalB forms strong adsorption on both hydrophobic and hydrophilic surfaces. When adsorbed to NH2-SAM the catalytic center of CalB is oriented towards the surface and hence not preferable for binding of the substrate. While when it is adsorbed on the COOH-SAM the catalytic center is facing the solution and is preferable for substrate binding. In a more recent work, Yang et al. (2020) have performed simulations of acetylcholinesterase from Torpedo californica (TcAChE) immobilized on COOH- and NH2- SAMs. Positively charged NH2-SAM surface was able to provide a better microenvironment needed for coherent bio-catalytic reaction of the enzyme. This led to quicker DET between the enzyme and the electrode surface. A more recent work emphasized the orientation and activity of enzymes while tethered into SAMs. Li et al. (2018) studied the immobilization of two variants of β-glactocidase tethered on a SAM surface terminated with pure maleimide and mixed hydrophilic surface made of hydroxyl groups and maleimide. Using coarse-grained MD simulations together with sum frequency generation (SFG) vibrational spectroscopy, they showed that the orientation of the immobilized enzyme plays an important role in its activity: A significant increase in activity was observed upon immobilization on mixed SAM in comparison to pure SAM. In further advanced work by the same group using a nitro-reductase (NfsB) as a model enzyme it was shown that two strategically placed surface tethering points could provide a better catalytic efficiency and stability (Zou et al., 2018). These immobilization sites were designed based upon the coarse-grained MD simulation results, and variants of the enzyme with cysteinyl residues at these sites were expressed and purified. These variants were thereafter immobilized upon maleimide terminated SAM. The results showed that in comparison to the enzyme tethered at a single site, immobilizing variants of NfsB using two tethering positions display general improvement in thermal stability.
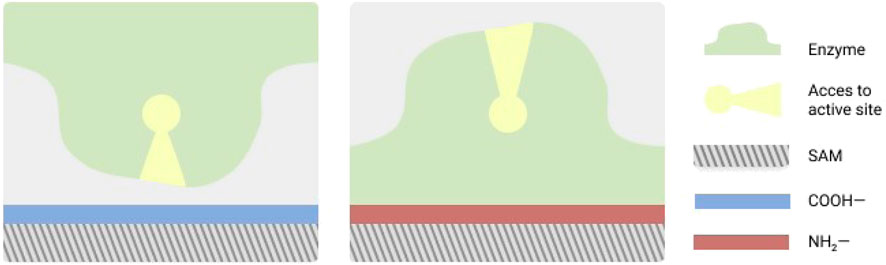
FIGURE 4. Scheme showing orientation of the RNase A active site with respect to different types of SAM surfaces. The scheme is based upon Figure 3 from Liu et al., 2015b.
Other studies have been focused on the immobilization of enzymes over SAM coated Au nanoparticles. The catalytic activity and structural integrity of an oxygen-tolerant [NiFe] hydrogenase on Au electrodes coated with SAM were studied using surface enhanced infrared adsorption (SEIRA) spectroscopy, atomic force microscopy (AFM), and protein field voltammetry (PFV) in combination with short all-atom MD simulations (Heidary et al., 2015). A more recent multiscale simulation work merging all-atom MD and coarse-grained Brownian dynamics simulation to study the adsorption of β-glucosidase A (βGA) on SAM-functionalized as well as bare gold surfaces (Bourassin et al., 2022). In this work it was observed that although there was little impact on the enzyme conformation, the adsorption process perturbed the mechanical properties and the catalytic activity of the enzyme. Although the βGA adsorption on SAM-functionalized surfaces is less stable than the bare gold, it is more specific and causes less disruption to enzymatic function.
4 Immobilization on graphene oxide and carbon nanotube
In the recent past, graphene and graphene oxide (GO), a graphene derivative which is soluble in water, have captivated immense attention due to their interesting chemical and physical properties. In particular, GO is found to be an ideal candidate for enzyme immobilization since it needs neither modification of the surface nor coupling reagents because it is enriched with oxygen-containing groups. Sun et al. (2014a) studied the interaction of α-chymotrypsin (ChT) with both graphene and GO. Although ChT was found to be adsorbed onto both surfaces, the hydrophobic and cationic residues of ChT interact stronger with GO, leading to the active site deformation and therefore its inhibition. In another work, Zhao et al. (2018) studied the orientation of cyt c on graphene and GO, supporting the hypothesis elucidated in the previous section which states that the orientation of cyt c on immobilizing surfaces is crucial for the electron transfer (ET). Using MD simulations, they investigated the conformational change, the pathways of ET, and dominant driving forces to understand the conformation, binding, and bioactivity of cyt c. It was observed that, in comparison to graphene, the cyt c heme plane was deviated moderately from the standard location and Met-80, the axial ligand, was more adjacent to the GO surface, facilitating the ET. Another enzyme family that has been immobilized using GO includes lipases. The immobilization of lipases, enzymes with several industrial applications, faces a key challenge related to opening the enzyme lid domain and maintaining this open conformation for its active site exposure. This can be achieved and tuned through chemical reduction of GO, which allows modulating the enzyme activity. MD simulations were used to study the molecular mechanisms driving the lid-opening, shedding light on the key role of the hydrophobic interactions occurring between the interface of the lipase and GO (Mathesh et al., 2016). Additionally, Zhuang et al. (2020) observed that the lipase immobilized on functionalized GO is around 20 times more active than when immobilized on GO. Regarding the effect of GO on enzyme stability, Li et al. (2021) showed that the thermal stability of D-psicose 3-epimerase (DPEase) enzyme is improved upon immobilization. Aiming to rationalize this effect all atom MD simulations of DPEase complexed with its natural substrate D-Fructose were performed with and without anchoring to GO. It was observed that the active site of DPEase is stabilized at high temperature in presence of GO. This is due to the fact that strong interaction between DPEase and GO can prevent the loops α1′-α1 and β4-α4 of the DPEase, which contains the active site residues, to drastically fluctuate. The simulation results supported previous experimental observations about the improvement in thermal stability of DPEase upon immobilization on GO. In contrast, the immobilization of the hen egg white lysozyme (HEWL) on GO led to active site blocking, affecting the flexibility of surrounding residues and hampering the activity (Bera et al., 2018). In a separate work, Ray et al. (2018) used GO to study the structure-function relationship of the nucleoside diphosphate kinase (NDPK), whose inhibition may be a potential therapy for patients with end-stage heart failure. MD simulations suggested that the interaction of GO with the NDPK His118 residue is very favorable, leading to the inhibition of adenosine triphosphate (ATP) binding to the enzyme, which can otherwise trigger mutated G protein phosphorylation. More recently, the interaction between COVID-19 protein from severe acute respiratory coronavirus (SARS-CoV-2), 3C-like (3CL) main protease (Mpro), with intact graphene (IG), defective graphene (DG) and GO, was investigated (Wang et al., 2022). It was observed that DG and GO interact with Mpro more intensely making its overall structure to become more flexible. Furthermore, it was shown that, in contrast to IG and GO, DG can inactivate Mpro and inhibit its expression effectively by hampering its active pocket.
Due to their unique thermal, mechanical and biocompatible characteristics, carbon nanotubes (CNTs), among all nanomaterials, are a very promising surface for supporting enzymes (Zaboli et al., 2019). Some of the first MD simulation studies elucidating protein-CNT interactions include proteins such as the Coxsackie-Adenovirus Receptor (CAR) (Johnson et al., 2009), human serum proteins (Ge et al., 2011), lysozyme (Vaitheeswaran and Garcia, 2011). Johnson et al. used all-atom MD simulations of the CAR and the CAR-Knob complex covalently attached to CNT to assess the degree of structural deformation upon binding. It was observed that, despite significant structural fluctuations, the overall structure of CAR underwent minor deformation from its native structure and did not affect the CAR’s ability to bind Knob. These results supported that CAR retains its biological functionality when attached to CNT. Ge et al. performed all-atom MD simulations in conjugation with experimental approaches to investigate the interactions of human serum proteins with single-wall CNTs (SWCNTs), finding a binding competitiveness of these proteins with different adsorption capacities and packing modes. They observed that π-π stacking interactions between SWCNTs and the aromatic residues of the protein are critical to the adsorption capacity. This in turn affects cellular responses resulting in different degrees of cytotoxicity. Vaitheeswaran and Garcia used coarse-grained replica exchange MD simulations to study the stability of lysozyme on CNT and found that it is dependent on the equilibrium between the unfavorable enthalpy and favorable entropy change upon adsorption. Subsequent work using all-atom MD simulations showed that the lysozyme-CNT interacting region is far away from the catalytic site, leading to intact catalytic activity. It was also observed that the Aminic and Amidic moieties of the protein behave like surfactants, blocking the access of the solvent to the CNT (Calvaresi et al., 2012). In a separate study, Zhang et al. (2015) studied the α-chymotrypsin-CNT system both in aqueous and heptane media. The results showed that, although the immobilization of the enzyme caused significant structure deviation from the native structure, insignificant changes in secondary structure were observed. Moreover, CNT was found to display a stabilization role in retaining the catalytic H-bond network, which was associated with the enhanced activity observed. Zhao and Zhou. (2017) studied the structure-function relationship of α-chymotrypsin (α-ChT) in interaction with pristine CNTs and carboxylated CNTs. It was observed that while interacting with the pristine CNTs through hydrophobic forces the active site of α-ChT is facing towards the solution and hence shows a non-competitive arrangement. However, the active pocket of the enzyme binds to carboxylated CNTs through a dominant electrostatic interaction which inhibits the enzyme in a competitive-like mode. In addition to this work, Di Giosia et al. (2020) studied the α-chymotrypsin-pristine CNT interactions using MD simulations together with spectroscopic, microscopic and kinetics experiments. They showed that CNT was occupying the α-chymotrypsin substrate binding site, reducing its available volume and therefore competing with the substrate and hampering the activity of the enzyme. The pristine and carboxyl-functionalized CNTs were further studied as immobilizing agents using the D-lactate dehydrogenase enzyme (Zaboli et al., 2019). It was observed that D-lactate dehydrogenase displayed an improved thermal stability when immobilized in comparison to the free enzyme. The simulations showed that the hydrogen bonding networks occurring between the enzyme and the functional groups of fCNT were responsible for maintaining the enzyme conformation more than was observed for pristine CNT. CNT surfaces were also used in order to understand the enzyme activation mechanism in non-aqueous media. The subtilisin carlsberg (SC) enzyme was immobilized onto CNT using water, acetonitrile and heptane as solvents (Zhang et al., 2016). It was found that the affinity of SC on CNT decreases with acetonitrile and in more degree with heptane, in contrast to water. This was explained by observing that the substrate binding pocket appeared significantly expanded by immobilization in the presence of acetonitrile and heptane. In a very recent work, Xu et al. (2022) studied the structural basis for the immobilization of a laccase enzyme on SWCNT. They used multi-scale simulations to gain insight into the direct electron transfer (DET) event of the Thermus thermophilus laccase (TtLac) adsorbed on carboxyl- and amino-functionalized CNTs (COOH-CIN and NH2-CNT respectively). It was observed that the laccase stability and its catalytic efficiency are more preserved when immobilized on NH2- than in COOH-CNT, since the enzyme undergoes less conformational perturbation. Other recent studies have focused on the interaction of CNTs with other biomolecules such as hormones and receptors (Mahmoodi et al., 2018; Zhang et al., 2018).
5 Immobilization on other surfaces
This section of the review covers structural studies of enzymes that have been immobilized on other surfaces such as polymers, membranes or zeolite. Petrosino et al. (2019) used a polysulfone (PSU) membrane surface to immobilize a phosphotriesterase (PTE) at a fixed pH. Hybrid quantum mechanics/molecular mechanics (QM/MM) was used to calculate the interaction energies between the enzyme and the surface, finding good agreement with the experimental adsorption free energies and supporting that PTE was effectively adsorbed on PSU. Interestingly, it was also observed that, with respect to the orientation observed onto pristine PTE, there was less accessibility for the immobilized enzyme binding site. This is because of the steric hindrance to the polymer, which may lead to a reduction in catalytic efficiency of the enzyme. In a recent work, the adsorption-desorption mechanisms of lysozyme over three antifouling polymer membranes were studied through MD simulations (Zhang et al., 2022). These are poly(3-(methacryloyloxy)propane-1-sulfonate) (T4-SP), poly(sulfobetaine methacrylate) (T4-SB), and poly(2-(dimethylamino)ethyl methacrylate) (T4-DM) which are grafted on polysiloxane membranes. The antifouling membranes lie in the core to prevent membrane fouling. The results showed that, after adsorption of lysozyme, the interaction is higher for T4-SP than T4-SB, being T4-DM showing the least interaction. The overall structure of the enzyme was not observed to change during the adsorption. However, slight fluctuation was noticed near the binding sites which was caused by structural adjustment for tighter combination. Although T4-DM has lowest interaction energy with lysozyme, the desorption of lysozyme on T4-DM is the hardest due to its larger hydrodynamic radius. The simulation results from this study are consistent with the experimental observation that T4-SB and T4-SP have good antifouling effects. Regarding other synthetic surfaces, Wei et al. (2011) studied the adsorption of lysozyme onto a polyethylene (PE) surface via MD simulations. The long axis of lysozyme, while adsorbed, was found to be parallel to the surface and displayed an anisotropic mobility over the surface. Interestingly, this observation is contrary to what was observed in the lysozyme adsorption to SNP (Hildebrand et al., 2015), but analogous to its adsorption on SAMs (Xie et al., 2013), as discussed in the previous sections. Additionally (Kubiak-Ossowska and Mulheran, 2010), also studied lysozyme adsorption on a model charged surface using MD simulations (Kubiak-Ossowska and Mulheran, 2010). The results showed that, although electrostatics steer the enzyme to a favorable binding orientation with respect to the surface, immobilization only occurs through the strong interaction of Arg128, a charged residue in a flexible location of the protein surface, with the model charge surface. More recently, the Zhou group combined MC and MD simulations to study the lysozyme adsorption on porous organic cages and MXenes (Zhao et al., 2020; 2021). For both surfaces van der Waals interactions-as well as electrostatics interactions for the later-played an important role in adsorption, while the conformation of the lysozyme remained stable suggesting a good biocompatibility. It was also observed that the interfacial water layer present over the surface plays a significant impact on adsorption. Regarding covalent attachment, an effective technique for irreversible enzyme immobilization, the cross-linking by glutaraldehyde (GA) is one of the most popular immobilization techniques. Hozhabr Araghi et al. (2021) combined MD simulations and quantum calculations to get a comprehensive molecular understanding of the interactions between the β-glucosidase (BGL) enzyme with propylamine-GA molecules. The results observed that all propylamine-GA molecules interacted with the lysine residues of BGL through their head side with Lys384, Lys376 and Lys247 being the most interactive residues. In another recent work Li et al. (2023) performed MD simulations along with surface residue microenvironment analysis to study thrombin adsorption on Ca2+ -exchanged LTA-type (CaA) zeolite. The observed thrombin deactivation on CaA zeolite seemed to be due to changes in the thrombin secondary structure upon adsorption. Additionally, some substrate binding sites of the enzyme are blocked by the zeolite surface after adsorption leading to the deactivation of thrombin. Moreover, few of the sites which bind heparin and fibrinogen are part of the positively charged area of thrombin which forms electrostatic interaction with CaA zeolite and plays an important role in thrombin adsorption. Since these sites are in vicinity to the catalytic sites of thrombin, this further affects thrombin coagulation activity on the CaA zeolite surface.
6 Immobilization on surfaces lacking molecular dynamics simulations
There are several surfaces on which immobilization of enzymes are studied experimentally but no detailed computational studies exist for them. For instance, due to several of their inherent properties, polymer brushes have received considerable attention as enzyme immobilization agents. Several studies have used brush polymers to immobilize lipase (Weltz et al., 2019; Weltz et al., 2020; Sánchez-Morán et al., 2021). A significant enhancement of the catalytic performance of Bacillus subtilis lipase A (lipA) was observed when immobilized on poly(sulfobetaine methacrylate) brushes, PSBMA. This was because of the stabilization of lipA structure through changes in its conformational dynamics, which resulted in the enhancement of its catalytic performance, which strongly depends on the chemistry of the brush. The basic mechanism for this structural stabilization by multipoint covalent immobilization to the brush polymer was also studied. The results showed that, with the increase in the number of lipA-brush attachments, the enzyme stability is increased and it is correlated directly with the enzyme rigidification. Additionally, several structurally diverse but related lipases were immobilized on random copolymer brush surfaces made up of sulfobetaine methacrylate (SBMA) and poly(ethylene glycol) methacrylate (PEGMA) aiming to shed light on the design of synthetic materials for enzyme stabilization. The results showed that the thermal stability of each lipase was strongly dependent on the fraction of PEGMA with respect to SBMA in the brush layer. However, a detailed structural understanding of this observation is lacking. The use of affinity tags or protein tags is another well established strategy used for enzyme immobilization (Ley et al., 2011). These are peptide sequences that are appended to proteins-normally at the N-terminus- so that they can be purified from their crude biological source. Polyhistidine tag, also known as His-tag, is one of the most commonly used affinity tags which is a string of usually between six to nine histidine residues. His-tag was used in recent works to immobilize β-glucosidase (Zhou et al., 2017), transketolase (Kulsharova et al., 2018), glucose dehydrogenase (Zhou et al., 2021), ketoreductase (Basso et al., 2022), and phosphomannose isomerase (Wang et al., 2023), between others. In a recent work, MD simulations were performed to study immobilization of fluorescent proteins through His-tag (Wasserberg et al., 2017). However, the application of this pipeline to predict sites for enzyme immobilization is still lacking. Metal-organic frameworks (MOFs) appeared recently as a promising immobilization material due to their attractive properties such as a high surface area, excellent stability, designable functionality, and tunable porosity (Ye et al., 2020). However, MOF-immobilization is still in its infancy and its mechanistic knowhow is still under development. Computational studies based on MD simulations may emerge as a tool to shed some light on these mechanisms and improve this arising immobilization strategy. Separately, recent studies have shown that magnetic nanoparticles (MNPs), which gained a special place as supporting matrices and versatile carriers, are considered a future trend for enzyme immobilization (Aber et al., 2016; Atacan et al., 2016; Mehrasbi et al., 2017; Zhou et al., 2017; Darwesh et al., 2019; Muley et al., 2020; Zanker et al., 2021; Zou et al., 2021). This is due to the easy recovery and reuse of MNPs by applying an external magnetic field in addition to their exceptional properties as nanoparticles like large surface-to-volume ratio, large surface area, high mass transfer and mobility (Bilal et al., 2018; Darwesh et al., 2020). However, molecular modeling studies in this field are still lacking and could shed light on the usage of MNPs, which paves the way towards an efficient green chemistry approach.
7 Computational protocols for predicting immobilization sites
The literature survey shown in above sections conveys that the computational studies were done primarily to rationalize the molecular aspects of the immobilization experiments. However, the use of state-of-the-art protocols that allow controlling the impact of immobilization on the enzyme properties is, up to date, very limited. In a recent work in this regard, the Knotts group tried to devise a reliable heuristics to identify optimal attachment locations in typical proteins (Smith et al., 2021), a protocol which may be promising when applied to enzyme immobilization. Using coarse-grained MD simulations they initially predicted how external factors-like confinement, tethering configuration, surface hydrophobicity and binding site valency-may affect protein stability and folding pathways (Rathore et al., 2006; Bush et al., 2015; Bush et al., 2017; Bush et al., 2018). Additionally, they performed tethering and PEGylation at a limited number of sites in the loop regions of lysozyme to prove the capability of predicting well-performing functionalization sites (Wei et al., 2010; Wei et al., 2011; Wei et al., 2013). Although no rigorous testing was performed for these heuristics, later they suggested regions involving secondary structures can be optimal functionalization sites (Wilding et al., 2018; Wilkerson et al., 2020). Very recently, they provided a screening protocol to search for accessible sites for functionalization on β-lactamase (TEM-1), either for tethering onto a surface or for PEGylation (Smith et al., 2021). The proposed heuristics of finding the accessible sites was also later validated through experiments (Soltani et al., 2022; Zhao et al., 2022). Overall, these studies may be a stepping stone to further design an adequate prediction protocol that helps to control and understand the structural and functional implications of the enzyme immobilization process, which will be key for its rapid implementation.
8 Conclusion
Immobilization of enzymes refers to physically confining or localizing enzymes in a defined region of space while preserving their catalytic performance. This phenomenon can significantly improve the stability, reusability, and enzyme lifetime. In addition to that, immobilization can also widen the enzyme application by further improving their stability under extreme conditions. Enzyme immobilization is often performed due to their use in applications like antimicrobial or/and antifouling coatings, drug delivery, industrial catalysis, biosensors or biofuel cells. Based on the different materials employed, various enzyme immobilization methods such as covalent bonding, adsorption, entrapment, and cross-linking were developed over the years. Although immobilization of enzymes has been a successful strategy to improve the catalytic properties of enzymes, in detailed understanding towards the correlation between the enzyme attachment site, the chemical properties of the attached surface, and activity of the enzyme remains elusive.
Over the past two decades, in silico investigations-in particular MD simulations-have emerged as an important tool to study the immobilization of enzymes that can disclose the microscopic nature of intermolecular interactions. Both all-atoms and coarse-grained MD simulations have been extensively used to study mechanistic details of enzyme immobilization on different surfaces like nanoparticles, SAM, graphene, CNT and others (summary presented in Table 1), often in combination with MC based approaches to study adsorption, the initial step of immobilization. These studies have revealed that enzyme performance can be tuned by controlling their orientation on a charged surface by the electric dipole. In the same manner, an hydrophobic dipole of the enzyme could be used as a criterion to predict its orientation on hydrophobic surfaces. Additionally, apart from the nature of the surface, the electrostatic potential distribution on the enzyme surface is also responsible for its orientation on the immobilizing material. Simulation studies of tethered enzymes show that tethering to multiple sites increases thermal stability of the enzyme. In addition, as speculated, they also reveal that immobilizing enzymes through positions that are far away from the active site leads to less disturbances in the catalytic regions, as well as that the choice of the solvent can also affect the enzyme immobilization, facts which are in accordance with the experimental observations.
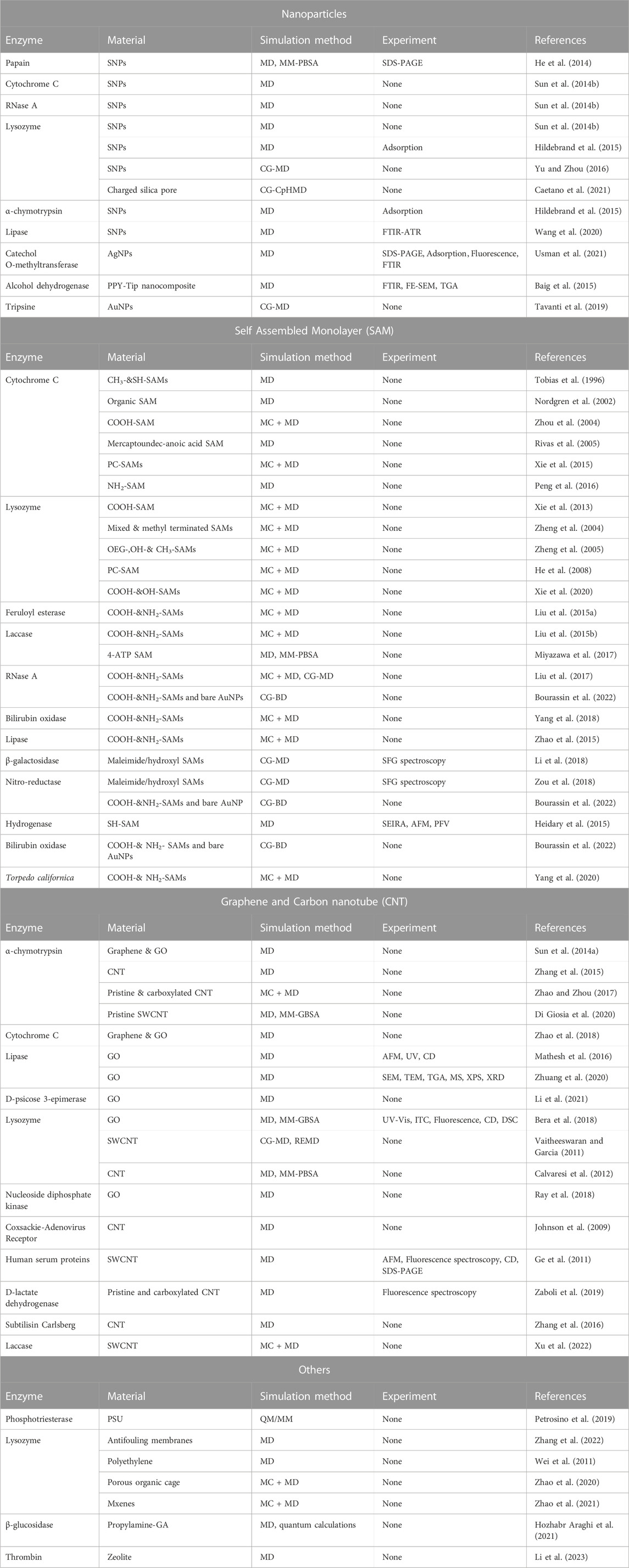
TABLE 1. Summary of Molecular Dynamics simulation studies of immobilization of different enzymes on different surfaces.
In spite of tremendous efforts made on investigating the enzyme immobilization phenomenon through in silico simulations, several key issues still require further exploration. The fact that a large variety of immobilizing agents exists, makes it difficult to decide which is the most adequate for a particular enzyme, and in silico strategies helping in this direction could speed up this selection process. Moreover, the simulations performed till date in this regard were primarily to rationalize the molecular aspects of the immobilization experiments. Only a very limited effort has been made in the use of computation to predict adequate protocols that can control the impact on the enzyme properties and the research field is still in its infancy. Overall, state-of-the-art force-field based computational methods like MD simulations are tedious and might not be the best approach to meet the industrial needs for predicting immobilization sites. In contrast, data driven methods like Machine Learning (ML)-based approaches might be key in the near future to design better pipelines that can aid in the growing immobilization field. For the time being, developing pipelines based on distinct levels of accuracy allowing a proper sampling and determination of the enzymatic performance might profoundly minimize the time consumption, costly trials, and investigation errors when developing highly efficient immobilized enzymes.
Author contributions
NB contributed to the research and review of the articles in literature and wrote the manuscript. LA-C and ML revised the manuscript. All authors contributed to the article and approved the submitted version.
Funding
NB is employed by Zymvol Biomodeling SL on a project (Software Development for Biocatalytic Process Optimization, ENZIMMO-P) which received funding from the European Union’s Horizon 2020 research and innovation programme under Marie Skłodowska-Curie grant agreement No. 801342 (Tecniospring INDUSTRY) and the Government of Catalonia’s Agency for Business Competitiveness (ACCIÓ).
Acknowledgments
The authors gratefully acknowledge Valeria González, Brand & Communications Specialist, Zymvol Biomodeling SL for helping in preparing the Figures. The authors would also like to thank the reviewers for their thoughtful comments and efforts towards improving the manuscript.
Conflict of interest
NB, LA-C, and ML were employed by the Zymvol Biomodeling SL.
Publisher’s note
All claims expressed in this article are solely those of the authors and do not necessarily represent those of their affiliated organizations, or those of the publisher, the editors and the reviewers. Any product that may be evaluated in this article, or claim that may be made by its manufacturer, is not guaranteed or endorsed by the publisher.
References
Abdelraheem, E. M. M., Busch, H., Hanefeld, U., and Tonin, F. (2019). Biocatalysis explained: From pharmaceutical to bulk chemical production. React. Chem. Eng. 4, 1878–1894. doi:10.1039/c9re00301k
Aber, S., Mahmoudikia, E., Karimi, A., and Mahdizadeh, F. (2016). Immobilization of glucose oxidase on Fe3O4 magnetic nanoparticles and its application in the removal of acid yellow 12. Water Air Soil Pollut. 227, 93. doi:10.1007/s11270-016-2754-x
Adams, J. P., Brown, M. J. B., Diaz-Rodriguez, A., Lloyd, R. C., and Roiban, G.-D. (2019). Biocatalysis: A pharma perspective. Adv. Synth. Catal. 2019, 201900424. doi:10.1002/adsc.201900424
Al-Ghanayem, A. A., and Joseph, B. (2020). Current prospective in using cold-active enzymes as eco-friendly detergent additive. Appl. Microbiol. Biotechnol. 104, 2871–2882. doi:10.1007/s00253-020-10429-x
Aleu, J., Bustillo, A., Hernandez-Galan, R., and Collado, I. (2006). Biocatalysis applied to the synthesis of agrochemicals. Curr. Org. Chem. 10, 2037–2054. doi:10.2174/138527206778742687
Atacan, K., Çakıroğlu, B., and Özacar, M. (2016). Improvement of the stability and activity of immobilized trypsin on modified Fe3O4 magnetic nanoparticles for hydrolysis of bovine serum albumin and its application in the bovine milk. Food Chem. 212, 460–468. doi:10.1016/j.foodchem.2016.06.011
Baig, U., Gondal, M. A., Alam, M. F., Laskar, A. A., Alam, M., and Younus, H. (2015). Enzyme immobilization and molecular modeling studies on an organic–inorganic polypyrrole–titanium(iv)phosphate nanocomposite. New J. Chem. 39, 6976–6986. doi:10.1039/c5nj01463h
Banerjee, I., Pangule, R. C., and Kane, R. S. (2011). Antifouling coatings: Recent developments in the design of surfaces that prevent fouling by proteins, bacteria, and marine organisms. Adv. Mat. 23, 690–718. doi:10.1002/adma.201001215
Barbosa, O., Ortiz, C., Berenguer-Murcia, Á., Torres, R., Rodrigues, R. C., and Fernandez-Lafuente, R. (2015). Strategies for the one-step immobilization-purification of enzymes as industrial biocatalysts. Biotechnol. Adv. 33, 435–456. doi:10.1016/j.biotechadv.2015.03.006
Barbosa, O., Torres, R., Ortiz, C., Berenguer-Murcia, A., Rodrigues, R. C., and Fernandez-Lafuente, R. (2013). Heterofunctional supports in enzyme immobilization: From traditional immobilization protocols to opportunities in tuning enzyme properties. Biomacromolecules 14, 2433–2462. doi:10.1021/bm400762h
Basso, A., Brown, M. S., Cruz-Izquierdo, A., Martinez, C. A., and Serban, S. (2022). Optimization of metal affinity ketoreductase immobilization for application in batch and flow processes. Org. Process Res. Dev. 26, 2075–2084. doi:10.1021/acs.oprd.1c00483
Basso, A., and Serban, S. (2019). Industrial applications of immobilized enzymes—a review. Mol. Catal. 479, 110607. doi:10.1016/j.mcat.2019.110607
Bera, S., Dhar, J., Dasgupta, R., Basu, G., Chakraborti, S., and Chakrabarti, P. (2018). Molecular features of interaction involving hen egg white lysozyme immobilized on graphene oxide and the effect on activity. Int. J. Biol. Macromol. 120, 2390–2398. doi:10.1016/j.ijbiomac.2018.09.007
Bernal, C., Rodríguez, K., and Martínez, R. (2018). Integrating enzyme immobilization and protein engineering: An alternative path for the development of novel and improved industrial biocatalysts. Biotechnol. Adv. 36, 1470–1480. doi:10.1016/j.biotechadv.2018.06.002
Bilal, M., Zhao, Y., Rasheed, T., and Iqbal, H. M. N. (2018). Magnetic nanoparticles as versatile carriers for enzymes immobilization: A review. Int. J. Biol. Macromol. 120, 2530–2544. doi:10.1016/j.ijbiomac.2018.09.025
Bourassin, N., Barbault, F., Baaden, M., and Sacquin-Mora, S. (2022). Between two walls: Modeling the adsorption behavior of β-glucosidase A on bare and SAM-functionalized gold surfaces. Langmuir 38, 1313–1323. doi:10.1021/acs.langmuir.1c01774
Brancolini, G., and Tozzini, V. (2019). Multiscale modeling of proteins interaction with functionalized nanoparticles. Curr. Opin. Colloid Interface Sci. 41, 66–73. doi:10.1016/j.cocis.2018.12.001
Brena, B., González-Pombo, P., and Batista-Viera, F. (2013). Immobilization of enzymes: A literature survey. Methods Mol. Biol. 1051, 15–31. doi:10.1007/978-1-62703-550-7_2
Bush, D. B., Knotts, T. A., and 4th, (2015). Communication: Antibody stability and behavior on surfaces. J. Chem. Phys. 143, 061101. doi:10.1063/1.4928455
Bush, D. B., Knotts, T. A., and 4th, (2017). Probing the effects of surface hydrophobicity and tether orientation on antibody-antigen binding. J. Chem. Phys. 146, 155103. doi:10.1063/1.4980083
Bush, D. B., Knotts, T. A., and 4th, (2018). The effects of antigen size, binding site valency, and flexibility on fab-antigen binding near solid surfaces. J. Chem. Phys. 149, 165102. doi:10.1063/1.5045356
Caetano, D. L. Z., Metzler, R., Cherstvy, A. G., and de Carvalho, S. J. (2021). Adsorption of lysozyme into a charged confining pore. Phys. Chem. Chem. Phys. 23, 27195–27206. doi:10.1039/d1cp03185f
Calvaresi, M., Hoefinger, S., and Zerbetto, F. (2012). Probing the structure of lysozyme-carbon-nanotube hybrids with molecular dynamics. Chemistry 18, 4308–4313. doi:10.1002/chem.201102703
Cao, L., van Langen, L., and Sheldon, R. A. (2003). Immobilised enzymes: Carrier-bound or carrier-free? Curr. Opin. Biotechnol. 14, 387–394. doi:10.1016/s0958-1669(03)00096-x
Casalini, T., Limongelli, V., Schmutz, M., Som, C., Jordan, O., Wick, P., et al. (2019). Molecular modeling for nanomaterial-biology interactions: Opportunities, challenges, and perspectives. Front. Bioeng. Biotechnol. 7, 268. doi:10.3389/fbioe.2019.00268
Chapman, J., Ismail, A., and Dinu, C. (2018). Industrial applications of enzymes: Recent advances, techniques, and outlooks. Catalysts 8, 238. doi:10.3390/catal8060238
Chen, R. (2001). Enzyme engineering: Rational redesign versus directed evolution. Trends Biotechnol. 19, 13–14. doi:10.1016/s0167-7799(00)01522-5
Choi, J.-M., Han, S.-S., and Kim, H.-S. (2015). Industrial applications of enzyme biocatalysis: Current status and future aspects. Biotechnol. Adv. 33, 1443–1454. doi:10.1016/j.biotechadv.2015.02.014
Chowdhury, R., and Maranas, C. D. (2020). From directed evolution to computational enzyme engineering—a review. AIChE J. 66. doi:10.1002/aic.16847
Darwesh, O. M., Ali, S. S., Matter, I. A., Elsamahy, T., and Mahmoud, Y. A. (2020). Enzymes immobilization onto magnetic nanoparticles to improve industrial and environmental applications. Methods Enzymol. 630, 481–502. doi:10.1016/bs.mie.2019.11.006
Darwesh, O. M., Matter, I. A., and Eida, M. F. (2019). Development of peroxidase enzyme immobilized magnetic nanoparticles for bioremediation of textile wastewater dye. J. Environ. Chem. Eng. 7, 102805. doi:10.1016/j.jece.2018.11.049
Di Giosia, M., Marforio, T. D., Cantelli, A., Valle, F., Zerbetto, F., Su, Q., et al. (2020). Inhibition of α-chymotrypsin by pristine single-wall carbon nanotubes: Clogging up the active site. J. Colloid Interface Sci. 571, 174–184. doi:10.1016/j.jcis.2020.03.034
Ganazzoli, F., and Raffaini, G. (2019). Classical atomistic simulations of protein adsorption on carbon nanomaterials. Curr. Opin. Colloid Interface Sci. 41, 11–26. doi:10.1016/j.cocis.2018.11.008
Garcia-Galan, C., Berenguer-Murcia, Á., Fernandez-Lafuente, R., and Rodrigues, R. C. (2011). Potential of different enzyme immobilization strategies to improve enzyme performance. Adv. Synth. Catal. 353, 2885–2904. doi:10.1002/adsc.201100534
Ge, C., Du, J., Zhao, L., Wang, L., Liu, Y., Li, D., et al. (2011). Binding of blood proteins to carbon nanotubes reduces cytotoxicity. Proc. Natl. Acad. Sci. U. S. A. 108, 16968–16973. doi:10.1073/pnas.1105270108
Hakala, T. K., Liitiä, T., and Suurnäkki, A. (2013). Enzyme-aided alkaline extraction of oligosaccharides and polymeric xylan from hardwood kraft pulp. Carbohydr. Polym. 93, 102–108. doi:10.1016/j.carbpol.2012.05.013
He, J., Wu, M., Feng, X., Shao, X., and Cai, W. (2014). Immobilization of papain on nanoporous silica. RSC Adv. 4, 13304–13312. doi:10.1039/c3ra47346e
He, Y., Hower, J., Chen, S., Bernards, M. T., Chang, Y., and Jiang, S. (2008). Molecular simulation studies of protein interactions with zwitterionic phosphorylcholine self-assembled monolayers in the presence of water. Langmuir 24, 10358–10364. doi:10.1021/la8013046
Heckmann, C. M., and Paradisi, F. (2020). Looking back: A short history of the discovery of enzymes and how they became powerful chemical tools. ChemCatChem 12, 6082–6102. doi:10.1002/cctc.202001107
Heidary, N., Utesch, T., Zerball, M., Horch, M., Millo, D., Fritsch, J., et al. (2015). Orientation-controlled electrocatalytic efficiency of an adsorbed oxygen-tolerant hydrogenase. PLoS One 10, e0143101. doi:10.1371/journal.pone.0143101
Hildebrand, N., Köppen, S., Derr, L., Li, K., Koleini, M., Rezwan, K., et al. (2015). Adsorption orientation and binding motifs of lysozyme and chymotrypsin on amorphous silica. J. Phys. Chem. C Nanomater. Interfaces 119, 7295–7307. doi:10.1021/acs.jpcc.5b00560
Hozhabr Araghi, S., John, A., and Sadeghi Googheri, M. S. (2021). How a crosslinker agent interacts with the β-glucosidase enzyme surface in an aqueous solution: Insight from quantum mechanics calculations and molecular dynamics simulations. Colloids Surf. B Biointerfaces 203, 111761. doi:10.1016/j.colsurfb.2021.111761
Johnson, R. R., Rego, B. J., Johnson, A. T. C., and Klein, M. L. (2009). Computational study of a nanobiosensor: A single-walled carbon nanotube functionalized with the coxsackie-adenovirus receptor. J. Phys. Chem. B 113, 11589–11593. doi:10.1021/jp901999a
Khambhaty, Y. (2020). Applications of enzymes in leather processing. Environ. Chem. Lett. 18, 747–769. doi:10.1007/s10311-020-00971-5
Khan, S., and Nandi, C. K. (2014). Optimizing the underlying parameters for protein-nanoparticle interaction: Advancement in theoretical simulation. Nanotechnol. Rev. 3. doi:10.1515/ntrev-2014-0002
Klibanov, A. M. (1979). Enzyme stabilization by immobilization. Anal. Biochem. 93, 1–25. doi:10.1016/s0003-2697(79)80110-4
Klibanov, A. M. (1983). Immobilized enzymes and cells as practical catalysts. Science 219, 722–727. doi:10.1126/science.219.4585.722
Kubiak-Ossowska, K., and Mulheran, P. A. (2010). What governs protein adsorption and immobilization at a charged solid surface? Langmuir 26, 7690–7694. doi:10.1021/la101276v
Kulsharova, G., Dimov, N., Marques, M. P. C., Szita, N., and Baganz, F. (2018). Simplified immobilisation method for histidine-tagged enzymes in poly(methyl methacrylate) microfluidic devices. N. Biotechnol. 47, 31–38. doi:10.1016/j.nbt.2017.12.004
Lee, H. (2021). Molecular modeling of protein corona formation and its interactions with nanoparticles and cell membranes for nanomedicine applications. Pharmaceutics 13, 637. doi:10.3390/pharmaceutics13050637
LeJeune, K. E., Wild, J. R., and Russell, A. J. (1998). Nerve agents degraded by enzymatic foams. Nature 395, 27–28. doi:10.1038/25634
Ley, C., Holtmann, D., Mangold, K.-M., and Schrader, J. (2011). Immobilization of histidine-tagged proteins on electrodes. Colloids Surf. B Biointerfaces 88, 539–551. doi:10.1016/j.colsurfb.2011.07.044
Li, C., Lu, Z., Wang, M., Chen, S., Han, L., and Han, W. (2021). A possible mechanism of graphene oxide to enhance thermostability of D-psicose 3-epimerase revealed by molecular dynamics simulations. Int. J. Mol. Sci. 22, 10813. doi:10.3390/ijms221910813
Li, J., Chen, H., Kang, Z., Liu, Y., Tu, Y., Wang, Q., et al. (2023). A combined computational and experimental approach predicts thrombin adsorption to zeolites. Colloids Surf. B Biointerfaces 221, 113007. doi:10.1016/j.colsurfb.2022.113007
Li, Y., Ogorzalek, T. L., Wei, S., Zhang, X., Yang, P., Jasensky, J., et al. (2018). Effect of immobilization site on the orientation and activity of surface-tethered enzymes. Phys. Chem. Chem. Phys. 20, 1021–1029. doi:10.1039/c7cp06063g
Liese, A., and Hilterhaus, L. (2013). Evaluation of immobilized enzymes for industrial applications. Chem. Soc. Rev. 42, 6236–6249. doi:10.1039/c3cs35511j
Liu, J., Peng, C., Yu, G., and Zhou, J. (2015a). Molecular simulation study of feruloyl esterase adsorption on charged surfaces: Effects of surface charge density and ionic strength. Langmuir 31, 10751–10763. doi:10.1021/acs.langmuir.5b01491
Liu, J., Xie, Y., Peng, C., Yu, G., and Zhou, J. (2017). Molecular understanding of laccase adsorption on charged self-assembled monolayers. J. Phys. Chem. B 121, 10610–10617. doi:10.1021/acs.jpcb.7b08738
Liu, J., Yu, G., and Zhou, J. (2015b). Ribonuclease A adsorption onto charged self-assembled monolayers: A multiscale simulation study. Chem. Eng. Sci. 121, 331–339. doi:10.1016/j.ces.2014.07.021
Liu, Z., Tabakman, S., Welsher, K., and Dai, H. (2009). Carbon nanotubes in biology and medicine: In vitro and in vivo detection, imaging and drug delivery. Nano Res. 2, 85–120. doi:10.1007/s12274-009-9009-8
Madhu, A., and Chakraborty, J. N. (2017). Developments in application of enzymes for textile processing. J. Clean. Prod. 145, 114–133. doi:10.1016/j.jclepro.2017.01.013
Mahmoodi, Y., Mehrnejad, F., and Khalifeh, K. (2018). Understanding the interactions of human follicle stimulating hormone with single-walled carbon nanotubes by molecular dynamics simulation and free energy analysis. Eur. Biophys. J. 47, 49–57. doi:10.1007/s00249-017-1228-4
Martí, D., Martín-Martínez, E., Torras, J., Betran, O., Turon, P., and Alemán, C. (2022). In silico study of substrate chemistry effect on the tethering of engineered antibodies for SARS-CoV-2 detection: Amorphous silica vs gold. Colloids Surf. B Biointerfaces 213, 112400. doi:10.1016/j.colsurfb.2022.112400
Mateo, C., Grazúj, V., Pessela, B. C. C., Montes, T., Palomo, J. M., Torres, R., et al. (2007a). Advances in the design of new epoxy supports for enzyme immobilization-stabilization. Biochem. Soc. Trans. 35, 1593–1601. doi:10.1042/bst0351593
Mateo, C., Palomo, J. M., Fernandez-Lorente, G., Guisan, J. M., and Fernandez-Lafuente, R. (2007b). Improvement of enzyme activity, stability and selectivity via immobilization techniques. Enzyme Microb. Technol. 40, 1451–1463. doi:10.1016/j.enzmictec.2007.01.018
Mathesh, M., Luan, B., Akanbi, T. O., Weber, J. K., Liu, J., Barrow, C. J., et al. (2016). Opening lids: Modulation of lipase immobilization by graphene oxides. ACS Catal. 6, 4760–4768. doi:10.1021/acscatal.6b00942
Meghwanshi, G. K., Kaur, N., Verma, S., Dabi, N. K., Vashishtha, A., Charan, P. D., et al. (2020). Enzymes for pharmaceutical and therapeutic applications. Biotechnol. Appl. Biochem. 67, 586–601. doi:10.1002/bab.1919
Mehrasbi, M. R., Mohammadi, J., Peyda, M., and Mohammadi, M. (2017). Covalent immobilization of Candida Antarctica lipase on core-shell magnetic nanoparticles for production of biodiesel from waste cooking oil. Renew. Energy 101, 593–602. doi:10.1016/j.renene.2016.09.022
Mehta, J., Bhardwaj, N., Bhardwaj, S. K., Kim, K.-H., and Deep, A. (2016). Recent advances in enzyme immobilization techniques: Metal-organic frameworks as novel substrates. Coord. Chem. Rev. 322, 30–40. doi:10.1016/j.ccr.2016.05.007
Minteer, S. D., Liaw, B. Y., and Cooney, M. J. (2007). Enzyme-based biofuel cells. Curr. Opin. Biotechnol. 18, 228–234. doi:10.1016/j.copbio.2007.03.007
Miyazawa, N., Tanaka, M., Hakamada, M., and Mabuchi, M. (2017). Molecular dynamics study of laccase immobilized on self-assembled monolayer-modified Au. J. Mat. Sci. 52, 12848–12853. doi:10.1007/s10853-017-1392-z
Mohamad, N. R., Marzuki, N. H. C., Buang, N. A., Huyop, F., and Wahab, R. A. (2015). An overview of technologies for immobilization of enzymes and surface analysis techniques for immobilized enzymes. Biotechnol. Biotechnol. Equip. 29, 205–220. doi:10.1080/13102818.2015.1008192
Muley, A. B., Mulchandani, K. H., and Singhal, R. S. (2020). Immobilization of enzymes on iron oxide magnetic nanoparticles: Synthesis, characterization, kinetics and thermodynamics. Methods Enzymol. 630, 39–79. doi:10.1016/bs.mie.2019.10.016
Nelson, J. M., and Griffin, E. G. (1916). Adsorption of invertase. J. Am. Chem. Soc. 38, 1109–1115. doi:10.1021/ja02262a018
Nguyen, H. H., and Kim, M. (2017). An overview of techniques in enzyme immobilization. Appl. Sci. Convergence Technol. 26, 157–163. doi:10.5757/asct.2017.26.6.157
Nirantar, S. R. (2021). Directed evolution methods for enzyme engineering. Molecules 26, 5599. doi:10.3390/molecules26185599
Nordgren, C. E., Tobias, D. J., Klein, M. L., and Blasie, J. K. (2002). Molecular dynamics simulations of a hydrated protein vectorially oriented on polar and nonpolar soft surfaces. Biophys. J. 83, 2906–2917. doi:10.1016/s0006-3495(02)75300-9
Peng, C., Liu, J., Xie, Y., and Zhou, J. (2016). Molecular simulations of cytochrome c adsorption on positively charged surfaces: The influence of anion type and concentration. Phys. Chem. Chem. Phys. 18, 9979–9989. doi:10.1039/c6cp00170j
Petrosino, F., Curcio, S., Chakraborty, S., and De Luca, G. (2019). Enzyme immobilization on polymer membranes: A quantum and molecular mechanics study. Comput. (Basel) 7, 56. doi:10.3390/computation7040056
Quan, X., Liu, J., and Zhou, J. (2019). Multiscale modeling and simulations of protein adsorption: Progresses and perspectives. Curr. Opin. Colloid Interface Sci. 41, 74–85. doi:10.1016/j.cocis.2018.12.004
Rajangam, B., Daniel, D. K., and Krastanov, A. I. (2018). Progress in enzyme inhibition based detection of pesticides. Eng. Life Sci. 18, 4–19. doi:10.1002/elsc.201700028
Ramakrishna, T. R. B., Nalder, T. D., Yang, W., Marshall, S. N., and Barrow, C. J. (2018). Controlling enzyme function through immobilisation on graphene, graphene derivatives and other two dimensional nanomaterials. J. Mat. Chem. B Mat. Biol. Med. 6, 3200–3218. doi:10.1039/c8tb00313k
Rathore, N., Knotts, T. A., and de Pablo, J. J. (2006). Confinement effects on the thermodynamics of protein folding: Monte Carlo simulations. Biophys. J. 90, 1767–1773. doi:10.1529/biophysj.105.071076
Raveendran, S., Parameswaran, B., Ummalyma, S. B., Abraham, A., Mathew, A. K., Madhavan, A., et al. (2018). Applications of microbial enzymes in food industry. Food Technol. Biotechnol. 56, 16–30. doi:10.17113/ftb.56.01.18.5491
Ray, A., Macwan, I., Singh, S., Silwal, S., and Patra, P. (2018). A computational approach for understanding the interactions between graphene oxide and nucleoside diphosphate kinase with implications for heart failure. Nanomater. (Basel) 8, 57. doi:10.3390/nano8020057
Rivas, L., Soares, C. M., Baptista, A. M., Simaan, J., Di Paolo, R. E., Murgida, D. H., et al. (2005). Electric-field-induced redox potential shifts of tetraheme cytochromes c3 immobilized on self-assembled monolayers: Surface-enhanced resonance Raman spectroscopy and simulation studies. Biophys. J. 88, 4188–4199. doi:10.1529/biophysj.104.057232
Rusmini, F., Zhong, Z., and Feijen, J. (2007). Protein immobilization strategies for protein biochips. Biomacromolecules 8, 1775–1789. doi:10.1021/bm061197b
Sá, A. G. A., Meneses, A. C., de Araújo, P. H. H., and Oliveira, D. (2017). A review on enzymatic synthesis of aromatic esters used as flavor ingredients for food, cosmetics and pharmaceuticals industries. Trends Food Sci. Technol. 69, 95–105. doi:10.1016/j.tifs.2017.09.004
Sánchez-Morán, H., Weltz, J. S., Schwartz, D. K., and Kaar, J. L. (2021). Understanding design rules for optimizing the interface between immobilized enzymes and random copolymer brushes. ACS Appl. Mat. Interfaces 13, 26694–26703. doi:10.1021/acsami.1c02443
Secundo, F. (2013). Conformational changes of enzymes upon immobilisation. Chem. Soc. Rev. 42, 6250–6261. doi:10.1039/c3cs35495d
Sheldon, R. A. (2018). Metrics of green chemistry and sustainability: Past, present, and future. ACS Sustain. Chem. Eng. 6, 32–48. doi:10.1021/acssuschemeng.7b03505
Sheldon, R. A., and van Pelt, S. (2013). Enzyme immobilisation in biocatalysis: Why, what and how. Chem. Soc. Rev. 42, 6223–6235. doi:10.1039/c3cs60075k
Sheldon, R. A., and Woodley, J. M. (2018). Role of biocatalysis in sustainable chemistry. Chem. Rev. 118, 801–838. doi:10.1021/acs.chemrev.7b00203
Sheldon, R. (2019). CLEAs, combi-CLEAs and “smart” magnetic CLEAs: Biocatalysis in a bio-based economy. Catalysts 9, 261. doi:10.3390/catal9030261
Silva, C., Martins, M., Jing, S., Fu, J., and Cavaco-Paulo, A. (2018). Practical insights on enzyme stabilization. Crit. Rev. Biotechnol. 38, 335–350. doi:10.1080/07388551.2017.1355294
Simões, B., Guedens, W. J., Keene, C., Kubiak-Ossowska, K., Mulheran, P., Kotowska, A. M., et al. (2021). Direct immobilization of engineered nanobodies on gold sensors. ACS Appl. Mat. Interfaces 13, 17353–17360. doi:10.1021/acsami.1c02280
Singh, G., Capalash, N., Kaur, K., Puri, S., and Sharma, P. (2016a). “Enzymes,” in Agro-industrial wastes as feedstock for enzyme production (Amsterdam, Netherlands: Elsevier), 157–172.
Singh, R., Kumar, M., Mittal, A., and Mehta, P. K. (2016b). Microbial enzymes: Industrial progress in 21st century. 3 Biotech. 6, 174. doi:10.1007/s13205-016-0485-8
Smith, A. K., Soltani, M., Wilkerson, J. W., Timmerman, B. D., Zhao, E. L., Bundy, B. C., et al. (2021). Coarse-grained simulation of PEGylated and tethered protein devices at all experimentally accessible surface residues on β-lactamase for stability analysis and comparison. J. Chem. Phys. 154, 075102. doi:10.1063/5.0032019
Smith, E. A., Thomas, W. D., Kiessling, L. L., and Corn, R. M. (2003). Surface plasmon resonance imaging studies of protein-carbohydrate interactions. J. Am. Chem. Soc. 125, 6140–6148. doi:10.1021/ja034165u
Soltani, M., Hunt, J. P., Smith, A. K., Zhao, E. L., Knotts, T. A., 4th, , et al. (2022). Assessing the predictive capabilities of design heuristics and coarse-grain simulation toward understanding and optimizing site-specific covalent immobilization of β-lactamase. Biotechnol. J. 17, e2100535. doi:10.1002/biot.202100535
Sun, X., Feng, Z., Hou, T., and Li, Y. (2014a). Mechanism of graphene oxide as an enzyme inhibitor from molecular dynamics simulations. ACS Appl. Mat. Interfaces 6, 7153–7163. doi:10.1021/am500167c
Sun, X., Feng, Z., Zhang, L., Hou, T., and Li, Y. (2014b). The selective interaction between silica nanoparticles and enzymes from molecular dynamics simulations. PLoS One 9, e107696. doi:10.1371/journal.pone.0107696
Talbert, J. N., and Goddard, J. M. (2012). Enzymes on material surfaces. Colloids Surf. B Biointerfaces 93, 8–19. doi:10.1016/j.colsurfb.2012.01.003
Tavanti, F., Pedone, A., and Menziani, M. C. (2019). Multiscale molecular dynamics simulation of multiple protein adsorption on gold nanoparticles. Int. J. Mol. Sci. 20, 3539. doi:10.3390/ijms20143539
Tobias, D. J., Mar, W., Blasie, J. K., and Klein, M. L. (1996). Molecular dynamics simulations of a protein on hydrophobic and hydrophilic surfaces. Biophys. J. 71, 2933–2941. doi:10.1016/s0006-3495(96)79497-3
Tran, D. N., and Balkus, K. J. (2011). Perspective of recent progress in immobilization of enzymes. ACS Catal. 1, 956–968. doi:10.1021/cs200124a
Ulman, A. (1996). Formation and structure of self-assembled monolayers. Chem. Rev. 96, 1533–1554. doi:10.1021/cr9502357
Usman, A., Lobb, K., Pletschke, B. I., Whiteley, C. G., and Wilhelmi, B. S. (2021). Interaction of silver nanoparticles with catechol O-methyltransferase: Spectroscopic and simulation analyses. Biochem. Biophys. Rep. 26, 101013. doi:10.1016/j.bbrep.2021.101013
Vaitheeswaran, S., and Garcia, A. E. (2011). Protein stability at a carbon nanotube interface. J. Chem. Phys. 134, 125101. doi:10.1063/1.3558776
Wackett, L. P. (2019). Microbial industrial enzymes: An annotated selection of World Wide Web sites relevant to the topics in microbial biotechnology. Microb. Biotechnol. 12, 405–406. doi:10.1111/1751-7915.13389
Wang, J., Yu, Y., Leng, T., Li, Y., and Lee, S.-T. (2022). The inhibition of SARS-CoV-2 3CL Mpro by graphene and its derivatives from molecular dynamics simulations. ACS Appl. Mat. Interfaces 14, 191–200. doi:10.1021/acsami.1c18104
Wang, K., Zhao, L., Li, T., Wang, Q., Ding, Z., and Dong, W. (2023). Selective immobilization of His-tagged enzyme on Ni-chelated ion exchange resin and its application in protein purification. Int. J. Mol. Sci. 24, 3864. doi:10.3390/ijms24043864
Wang, L., Marciello, M., Estévez-Gay, M., Soto Rodriguez, P. E. D., Luengo Morato, Y., Iglesias-Fernández, J., et al. (2020). Enzyme conformation influences the performance of lipase-powered nanomotors. Angew. Chem. Int. Ed. Engl. 59, 21266–21273. doi:10.1002/ange.202008339
Wasserberg, D., Cabanas-Danés, J., Prangsma, J., O’Mahony, S., Cazade, P.-A., Tromp, E., et al. (2017). Controlling protein surface orientation by strategic placement of oligo-histidine tags. ACS Nano 11, 9068–9083. doi:10.1021/acsnano.7b03717
Wei, S., Knotts, , and Thomas, A. (2013). A coarse grain model for protein-surface interactions. J. Chem. Phys. 139, 095102. doi:10.1063/1.4819131
Wei, S., Knotts, , and Thomas, A. (2011). Effects of tethering a multistate folding protein to a surface. J. Chem. Phys. 134, 185101. doi:10.1063/1.3589863
Wei, S., Knotts, , and Thomas, A. (2010). Predicting stability of alpha-helical, orthogonal-bundle proteins on surfaces. J. Chem. Phys. 133, 115102. doi:10.1063/1.3479039
Wei, T., Carignano, M. A., and Szleifer, I. (2011). Lysozyme adsorption on polyethylene surfaces: Why are long simulations needed? Langmuir 27, 12074–12081. doi:10.1021/la202622s
Weltz, J. S., Kienle, D. F., Schwartz, D. K., and Kaar, J. L. (2019). Dramatic increase in catalytic performance of immobilized lipases by their stabilization on polymer brush supports. ACS Catal. 9, 4992–5001. doi:10.1021/acscatal.9b01176
Weltz, J. S., Kienle, D. F., Schwartz, D. K., and Kaar, J. L. (2020). Reduced enzyme dynamics upon multipoint covalent immobilization leads to stability-activity trade-off. J. Am. Chem. Soc. 142, 3463–3471. doi:10.1021/jacs.9b11707
Wilding, K. M., Smith, A. K., Wilkerson, J. W., Bush, D. B., Knotts, T. A., et al. (2018). The locational impact of site-specific PEGylation: Streamlined screening with cell-free protein expression and coarse-grain simulation. ACS Synth. Biol. 7, 510–521. doi:10.1021/acssynbio.7b00316
Wilkerson, J. W., Smith, A. K., Wilding, K. M., Bundy, B. C., and Knotts, T. A. (2020). The effects of p-azidophenylalanine incorporation on protein structure and stability. J. Chem. Inf. Model. 60, 5117–5125. doi:10.1021/acs.jcim.0c00725
Woodley, J. M. (2020). Towards the sustainable production of bulk-chemicals using biotechnology. N. Biotechnol. 59, 59–64. doi:10.1016/j.nbt.2020.07.002
Wu, S., Snajdrova, R., Moore, J. C., Baldenius, K., and Bornscheuer, U. T. (2021). Biocatalysis: Enzymatic synthesis for industrial applications. Angew. Chem. Int. Ed. Engl. 60, 88–119. doi:10.1002/anie.202006648
Xiao, H., Huang, B., Yao, G., Kang, W., Gong, S., Pan, H., et al. (2018). Atomistic simulation of the coupled adsorption and unfolding of protein GB1 on the polystyrenes nanoparticle surface. Sci. China. Ser. G. Phys. Mech. Astron. 61, 038711. doi:10.1007/s11433-017-9124-3
Xie, Y., Gong, W., Jin, J., Zhao, Z., Li, Z., and Zhou, J. (2020). Molecular simulations of lysozyme adsorption on an electrically responsive mixed self-assembled monolayer. Appl. Surf. Sci. 506, 144962. doi:10.1016/j.apsusc.2019.144962
Xie, Y., Liao, C., and Zhou, J. (2013). Effects of external electric fields on lysozyme adsorption by molecular dynamics simulations. Biophys. Chem. 179, 26–34. doi:10.1016/j.bpc.2013.05.002
Xie, Y., Pan, Y., Zhang, R., Liang, Y., and Li, Z. (2015). Modulating protein behaviors on responsive surface by external electric fields: A molecular dynamics study. Appl. Surf. Sci. 326, 55–65. doi:10.1016/j.apsusc.2014.11.078
Xu, Z., Yang, S., Xie, Y., Yu, H., and Zhou, J. (2022). Modulating the adsorption orientation of methionine-rich laccase by tailoring the surface chemistry of single-walled carbon nanotubes. Colloids Surf. B Biointerfaces 217, 112660. doi:10.1016/j.colsurfb.2022.112660
Yang, S., Liu, J., Quan, X., and Zhou, J. (2018). Bilirubin oxidase adsorption onto charged self-assembled monolayers: Insights from multiscale simulations. Langmuir 34, 9818–9828. doi:10.1021/acs.langmuir.8b01974
Yang, S., Liu, J., Zheng, H., Zhong, J., and Zhou, J. (2020). Simulated revelation of the adsorption behaviours of acetylcholinesterase on charged self-assembled monolayers. Nanoscale 12, 3701–3714. doi:10.1039/c9nr10123c
Yang, Z., Mesiano, A. J., Venkatasubramanian, S., Gross, S. H., Harris, J. M., and Russell, A. J. (1995). Activity and stability of enzymes incorporated into acrylic polymers. J. Am. Chem. Soc. 117, 4843–4850. doi:10.1021/ja00122a014
Yarosh, D. B., Rosenthal, A., and Moy, R. (2019). <p>Six critical questions for DNA repair enzymes in skincare products: A review in dialog</p>. Clin. Cosmet. Investig. Dermatol. 12, 617–624. doi:10.2147/ccid.s220741
Ye, N., Kou, X., Shen, J., Huang, S., Chen, G., and Ouyang, G. (2020). Metal-organic frameworks: A new platform for enzyme immobilization. Chembiochem 21, 2585–2590. doi:10.1002/cbic.202000095
Yeung, S. Y., Ederth, T., Pan, G., Cicėnaitė, J., Cárdenas, M., Arnebrant, T., et al. (2018). Reversible self-assembled monolayers (rSAMs) as robust and fluidic lipid bilayer mimics. Langmuir 34, 4107–4115. doi:10.1021/acs.langmuir.8b00226
Yu, G., and Zhou, J. (2016). Understanding the curvature effect of silica nanoparticles on lysozyme adsorption orientation and conformation: A mesoscopic coarse-grained simulation study. Phys. Chem. Chem. Phys. 18, 23500–23507. doi:10.1039/c6cp01478j
Yu, Q., Zhang, Y., Wang, H., Brash, J., and Chen, H. (2011). Anti-fouling bioactive surfaces. Acta Biomater. 7, 1550–1557. doi:10.1016/j.actbio.2010.12.021
Zaboli, M., Raissi, H., Zaboli, M., Farzad, F., and Torkzadeh-Mahani, M. (2019). Stabilization of d-lactate dehydrogenase diagnostic enzyme via immobilization on pristine and carboxyl-functionalized carbon nanotubes, a combined experimental and molecular dynamics simulation study. Arch. Biochem. Biophys. 661, 178–186. doi:10.1016/j.abb.2018.11.019
Zacharis, E., Moore, B. D., and Halling, P. J. (1997). Control of enzyme activity in organic media by solid-state Acid−Base buffers. J. Am. Chem. Soc. 119, 12396–12397. doi:10.1021/ja972635c
Zanker, A. A., Ahmad, N., Son, T. H., Schwaminger, S. P., and Berensmeier, S. (2021). Selective ene-reductase immobilization to magnetic nanoparticles through a novel affinity tag. Biotechnol. J. 16, e2000366. doi:10.1002/biot.202000366
Zdarta, J., Meyer, A., Jesionowski, T., and Pinelo, M. (2018). A general overview of support materials for enzyme immobilization: Characteristics, properties, practical utility. Catalysts 8, 92. doi:10.3390/catal8020092
Zhang, H., Zheng, J., Lin, C., and Yuan, S. (2022). Molecular dynamics study on adsorption and desorption of lysozyme above polymer antifouling membranes. Colloids Surf. A Physicochem. Eng. Asp. 649, 129466. doi:10.1016/j.colsurfa.2022.129466
Zhang, L., Li, Y., Yuan, Y., Jiang, Y., Guo, Y., Li, M., et al. (2016). Molecular mechanism of carbon nanotube to activate Subtilisin Carlsberg in polar and non-polar organic media. Sci. Rep. 6, 36838. doi:10.1038/srep36838
Zhang, L., Xiao, X., Yuan, Y., Guo, Y., Li, M., and Pu, X. (2015). Probing immobilization mechanism of alpha-chymotrypsin onto carbon nanotube in organic media by molecular dynamics simulation. Sci. Rep. 5, 9297. doi:10.1038/srep09297
Zhang, L., Yuan, Y., Ren, T., Guo, Y., Li, C., and Pu, X. (2018). Shining light on molecular mechanism for odor-selectivity of CNT-immobilized olfactory receptor. Sci. Rep. 8, 7824. doi:10.1038/s41598-018-26105-0
Zhao, D., Huang, C., Quan, X., Li, L., Wang, Y., and Zhou, J. (2021). Lysozyme adsorption on different functionalized MXenes: A multiscale simulation study. Langmuir 37, 5932–5942. doi:10.1021/acs.langmuir.1c00480
Zhao, D., Li, L., and Zhou, J. (2018). Simulation insight into the cytochrome c adsorption on graphene and graphene oxide surfaces. Appl. Surf. Sci. 428, 825–834. doi:10.1016/j.apsusc.2017.09.190
Zhao, D., Peng, C., and Zhou, J. (2015). Lipase adsorption on different nanomaterials: A multi-scale simulation study. Phys. Chem. Chem. Phys. 17, 840–850. doi:10.1039/c4cp04696j
Zhao, D., Wang, Y., Su, Q., Li, L., and Zhou, J. (2020). Lysozyme adsorption on porous organic cages: A molecular simulation study. Langmuir 36, 12299–12308. doi:10.1021/acs.langmuir.0c02233
Zhao, D., and Zhou, J. (2017). Electrostatics-mediated α-chymotrypsin inhibition by functionalized single-walled carbon nanotubes. Phys. Chem. Chem. Phys. 19, 986–995. doi:10.1039/c6cp04962a
Zhao, E. L., Soltani, M., Smith, A. K., Hunt, J. P., Knotts, T. A., 4th, , et al. (2022). Assessing site-specific PEGylation of TEM-1 β-lactamase with cell-free protein synthesis and coarse-grained simulation. J. Biotechnol. 345, 55–63. doi:10.1016/j.jbiotec.2021.12.016
Zheng, J., Li, L., Chen, S., and Jiang, S. (2004). Molecular simulation study of water interactions with oligo (ethylene glycol)-terminated alkanethiol self-assembled monolayers. Langmuir 20, 8931–8938. doi:10.1021/la036345n
Zheng, J., Li, L., Tsao, H.-K., Sheng, Y.-J., Chen, S., and Jiang, S. (2005). Strong repulsive forces between protein and oligo (ethylene glycol) self-assembled monolayers: A molecular simulation study. Biophys. J. 89, 158–166. doi:10.1529/biophysj.105.059428
Zhou, J., Zheng, J., and Jiang, S. (2004). Molecular simulation studies of the orientation and conformation of cytochrome c adsorbed on self-assembled monolayers. J. Phys. Chem. B 108, 17418–17424. doi:10.1021/jp038048x
Zhou, L.-J., Li, R.-F., Li, X.-Y., and Zhang, Y.-W. (2021). One-step selective affinity purification and immobilization of His-tagged enzyme by recyclable magnetic nanoparticles. Eng. Life Sci. 21, 364–373. doi:10.1002/elsc.202000093
Zhou, Y., Yuan, S., Liu, Q., Yan, D., Wang, Y., Gao, L., et al. (2017). Synchronized purification and immobilization of his-tagged β-glucosidase via Fe3O4/PMG core/shell magnetic nanoparticles. Sci. Rep. 7, 41741. doi:10.1038/srep41741
Zhuang, W., Quan, X., Wang, Z., Zhou, W., Yang, P., Ge, L., et al. (2020). Interfacial microenvironment for lipase immobilization: Regulating the heterogeneity of graphene oxide. Chem. Eng. J. 394, 125038. doi:10.1016/j.cej.2020.125038
Zou, S., Chen, T.-J., Li, D.-Y., Fan, S., Yang, Z.-Y., and Zhu, P. (2021). LXYL-P1-2 immobilized on magnetic nanoparticles and its potential application in paclitaxel production. J. Biotechnol. 50, 10–15. doi:10.1016/j.ejbt.2020.12.005
Keywords: enzyme immobilization, molecular dynamics simulations, nanoparticles, self assembled monolayers, graphene, carbon nanotube
Citation: Bhattacharjee N, Alonso-Cotchico L and Lucas MF (2023) Enzyme immobilization studied through molecular dynamic simulations. Front. Bioeng. Biotechnol. 11:1200293. doi: 10.3389/fbioe.2023.1200293
Received: 04 April 2023; Accepted: 30 May 2023;
Published: 08 June 2023.
Edited by:
Ismail Ocsoy, Erciyes University, TürkiyeReviewed by:
Seyma Dadi, Abdullah Gül University, TürkiyeZülfikar Temoçin, Kırıkkale University, Türkiye
Copyright © 2023 Bhattacharjee, Alonso-Cotchico and Lucas. This is an open-access article distributed under the terms of the Creative Commons Attribution License (CC BY). The use, distribution or reproduction in other forums is permitted, provided the original author(s) and the copyright owner(s) are credited and that the original publication in this journal is cited, in accordance with accepted academic practice. No use, distribution or reproduction is permitted which does not comply with these terms.
*Correspondence: Nicholus Bhattacharjee, bmJoYXR0YWNoYXJqZWVAenltdm9sLmNvbQ==