- 1Department of Ophthalmology, Research Laboratory of Macular Disease, Department of Biotherapy, Cancer Center and State Key Laboratory of Biotherapy, West China Hospital, Sichuan University, Chengdu, Sichuan, China
- 2Center of Scientific Research, Chengdu Medical College, Chengdu, Sichuan, China
Age-related macular degeneration (AMD) is the predominant threat to human vision and ultimately results in blindness. With the increase in the aging population, it has become a more crucial issue to human health. AMD is a multifactorial disease with the unique feature of uncontrollable angiogenesis during initiation and progression. Although increasing evidence indicates that AMD is largely hereditary, the predominant efficient treatment is antiangiogenesis, which mainly involves VEGF and HIF-α as therapeutic targets. The repeated administration of this treatment over the long term, generally through intravitreal injection, has called for the introduction of long-term drug delivery systems, which are expected to be achieved by biomaterials. However, the clinical results of the port delivery system indicate that the optimization of medical devices toward prolonging the activities of therapeutic biologics in AMD therapy seems more promising. These results indicate that we should rethink the possibility and potential of biomaterials as drug delivery systems in achieving long-term, sustained inhibition of angiogenesis in AMD therapy. In this review, the etiology, categorization, risk factors, pathogenesis, and current clinical treatments of AMD are briefly introduced. Next, the development status of long-term drug delivery systems is discussed, and the drawbacks and shortages of these systems are emphasized. By comprehensively considering the pathological aspect and the recent application of drug delivery systems in AMD therapy, we hope to find a better solution for the further development of long-term therapeutic strategies for AMD.
1 Introduction
Age-related macular degeneration (AMD) remains the leading cause of low vision and blindness in among geriatrics over 55 years worldwide (Wong et al., 2014; Mitchell et al., 2018). In early- and intermediate-stage AMD, the pathological event is deposits of lipoprotein called drusen, or diffused form as basal linear deposits (BLinD), which commonly locates in subretinal space, along with overlying photoreceptors and retinal pigment epithelium (RPE) degeneration. AMD progresses to advanced form, designated as geographic atrophy (GA) or neovascular AMD (NVAMD) (Apte, 2021). NVAMD is the main clinical type, occupying 10%–20% of AMD and inducing severe loss of center vision while pathologic new blood vessel growth into the macula. This “leaking” vessel development is called choroidal neovascularization (CNV), which results in repeated macular bleeding, exudation, and fibrosis, potentially permanently damaging the morphology and function of the macula (Figure 1).
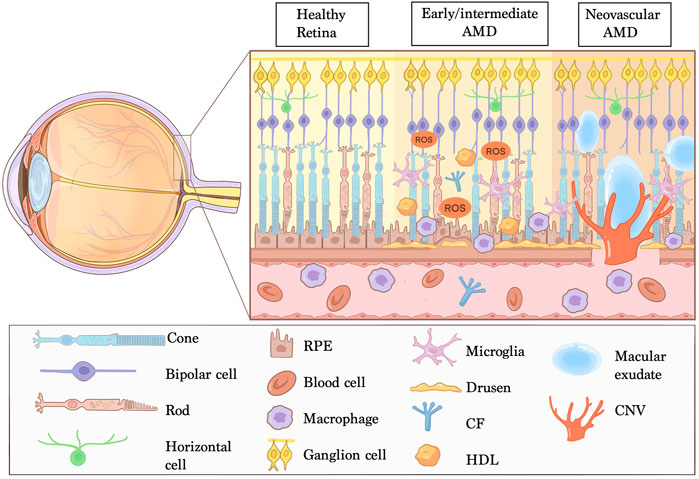
FIGURE 1. Manifestations of NVAMD. Age-related macular degeneration (AMD) is a multifactorial disease affecting the complex of photoreceptors, retinal pigment epithelium (RPE), Bruch’s membrane and choriocapillaris. The retina-RPE-Bruch’s membrane choroid complex is normal in healthy retina. The early and intermediate AMD are typical of deposition of extracellular debris named Drusen and pathological changes in the RPE. Neovascular AMD is characterized by invasion of choroidal neovascularization (CNV) into the outer retina, subretinal space or sub-RPE space and imbalanced angiogenesis factors. In addition, chronic inflammation, lipid deposition, oxidative stress and impaired extracellular matrix maintenance are strongly associated with AMD pathogenesis. Abbreviations: CF, complement factors; HDL, high density lipoproteins; ROS, reactive oxygen species.
Since 2016, anti-vascular endothelial growth factor (VEGF) therapy have remarkably ameliorate vision prognosis and life quality of NVAMD patients (Campochiaro et al., 2016). However, a significant proportion of patients with NVAMD present with persistent exudation, unresolved or new hemorrhages, and progressive fibrosis, and vision cannot be restored (Mettu et al., 2021). Additionally, the predominate phenomenon of choriocapillaris dropout found by newly developed retinal imaging modules like optical coherence tomography (OCT) and OCT-Angiography strongly sustain histopathologic discoveries in NVAMD patients. This may indicate that hypoxia and VEGF upregulation are less impossible self-eliminate (McLeod et al., 2009; Mullins et al., 2011; Biesemeier et al., 2014). Exudation, one aspect of disease activity, could be worsened while the agent is cleared. Additionally, repeated intravitreal injections in a long term can lead to complications, such as elevated intraocular pressure, uveitis, retinal detachment and endophthalmitis (Mehta et al., 2018). Thus, there is an urgent need to develop alternatives, which have low adherence, high socioeconomic costs, and long-lasting or sustained release of anti-angiogenesis drugs. In this review, we will briefly discuss the clinical aspects and treatment modalities of NVAMD and highlight the progress and future perspectives of nanotherapeutics.
2 Epidemiology, categorization, and risk factors for NVAMD
2.1 Epidemiology of NVAMD
A systematic review and meta-analysis including 12, 727 AMD cases from 39 studies based on a population of 129, 664 individuals showed a prevalence of 8.69%, 8.01% and 0.37% related to any, early and late AMD respectively, the estimated number of patients with AMD in 2020 is 196 million, increasing to 288 million in 2040 (Wong et al., 2014). Furthermore, population increase by 38% globally between 2000 and 2050, among those the proportion of individuals older than 60 will increase by 400%; the number of individuals with NVAMD is estimated to be 80.4 million by 2050, posing a major public health problem with substantial socioeconomic implications (Smith, 2010).
2.2 Categorization of NVAMD
In 2020, an international study group discussed and codified a set nomenclature framework for classifying the subtypes of neovascular AMD and associated lesion components (Spaide et al., 2020). The consensus is that neovascularization does not necessarily originate from the choroid in patients with NVAMD. Compared to CNV, macular neovascularization (MNV) is a more specific term describing the pathologic blood vessel of NVAMD. The group proposed three categories of MNV (Spaide et al., 2020): Type 1 and Type 2 MNV refer to neovascularization that originates from the choroid, the former grows under the sub-RPE space leading to different types of pigment epithelium detachment (PED), while the latter broke through RPE-Bruch’s membrane (BrM) into the subretinal space. Type 3 MNV refers to neovascularization that originates from the deep capillary plexus of retinal circulation and grows toward the outer retina. The same treatment may have different efficacies in different subtypes of NVAMD, indicating the complexity of its pathogenesis, which deserves more exploration in the future.
2.3 Risk factors for NVAMD
Many risk factors have been associated with AMD (including both non-neovascular AMD and NVAMD), with aging being the strongest, making AMD a multifactorial, spectrum of disease. In addition to age, AMD is strongly susceptible to genetic variants. Genetic factors account for 40%–60% of all AMD attribution risk, and variants in CFH and ARMS2–HTRA1 confer the highest risk of AMD (Seddon, 2017; Mitchell et al., 2018). AMD risk is also influenced by nongenetic and environmental factors, such as smoking and diet (Lambert et al., 2016; Seddon, 2017). Smoking is the most important modifiable risk factor for AMD, and the pathogenesis of smoking as a risk factor may include choroidal thinning, oxidative stress, and activation of compliment factors (Myers et al., 2014). Increased overall fat intake accelerates the development of AMD, while increasing ω-3 fatty acid intake in the diet, such as fish, reduces the risk of AMD (Tan et al., 2008; Zhu et al., 2016). Cardiovascular events like hypertension and hyperlipidemia have been inconsistently related to AMD (Cheung and Wong, 2014). Serum lipids concentration increased risk of intermediate AMD in some studies but not in others (Kabasawa et al., 2011; Pennington and DeAngelis, 2016).
3 Pathogenesis of NVAMD
In recent years, due to the in-depth study of AMD, we have a deeper understanding of its pathogenesis, and the pieces of puzzles have gradually formed a clear network of etiology. Age and lipid metabolism, genetic susceptibility, inflammation, innate immunity, and angiogenesis, these pathological events promote and interact with each other, leading to the terminal stage of AMD disease, namely, NVAMD. Moreover, there is growing evidence that inflammatory activation plays an important role in the pathogenesis of NVAMD.
3.1 Ageing related changes to RPE-BrM-choriocapillaris complex
RPE-BrM-choriocapillaris complex and photoreceptor are the key structures in pathogenesis of AMD. They are anatomic adjacent and metabolic dependent to each other. REP dysfunction is considered as a trigger for early AMD, but not a single event. In normal aged RPE cells, a series of physiological changes include: multinucleation, decreased mitochondrial numbers, accumulation of lipofuscin, and et cl (Tong et al., 2022). These changes make RPE cells more sensitive to oxidative stress and cytotoxicity such as smoking and other toxic substances. Lipid metabolism abnormality mainly refers to the accumulation of lipoprotein in the cell. N-retinylidene-N-retinylethanolamine (A2E) is a well-known fluorophore of lipofuscin contributing to RPE cells degeneration (Sparrow and Boulton, 2005). Photosensitization of A2E can increase DNA damage and cells senescence which could affect the microenvironment of the retina by expressing several factors of the secretory phenotype including IL1B, IL13RA2, and CXCR4 through the NF-κB pathway, expression of these factors also may create a pro-inflammatory environment that drives retina degeneration (Wang et al., 2018). Meanwhile, Bruch’s membrane is responsible for fluid exchange between out layer retina including RPE and choriocapillaris. With ageing, Bruch’s membrane becomes thicker and more fragile, which may weaken capacity of transportation resulting in lipid accumulation. The rupture of Bruch’s membrane is considered to a must condition during the formation of choroidal neovascularization. Age-related changes of choriocapillaris mainly manifest the decreased of vessel density and widened distance between the lumens (Ramrattan et al., 1994; Spraul and Grossniklaus, 1997). This phenomenon of choriocapillaris drop out make clearance of lipid particles and cell-debris less sufficient, further generate hypoxia in the complex of RPE-BrM-choriocapillaris, an important prerequisite for angiogenesis.
3.2 Complement activation
Variants of genes encoding complement system regulatory proteins (e.g., CFH, CFI, C3 and FB/C2) contain major risk susceptibility alleles for AMD (Seddon, 2017; Toomey et al., 2018). The complement system is part of the innate immune system whose main role is to recognize and identify foreign pathogens, apoptotic cells, and cellular debris. Activation of the complement system revolves around the cleavage of C3. C3 produces C3a and C3b under the action of invertase (Kato et al., 2020). Alternative pathways also exist with automatic activation, which is controlled by C3 invertase degradation. The alternative pathway is automatically activated when C3 invertase degradation is weakened, and one of the main cofactors for C3 invertase degradation is complement factor H (CFH). When the alternative pathway is activated, large amounts of C3b are produced, which in turn induces the formation of C5 convertase and membrane attack complex (MAC). MAC stimulates signaling pathways related to tissue remodeling, inflammation, and vascularization (Park et al., 2021).
C3 and other complement proteins can be detected in the composition of drusen, indicating that drusen itself will further stimulate inflammatory response and C-reactive protein, and local inflammation caused by abnormal RPE will aggravate drusen aggregation (Bhutto et al., 2011). There is also a lot of evidence that complement activation first occurs in the choroidal capillary layer. Complement activation also stimulates the natural inflammatory response of choroidal endothelial cell. Choroidal capillaries are the only capillaries that express the intercellular adhesion factor ICAM-1 in endothelial cells, which mainly regulates leukocyte infiltration. In vitro studies have shown that C5a can increase the expression of ICAM-1, indicating that complement activation can promote choroidal capillary leukocyte recruitment (Johnson et al., 2006; Skeie et al., 2010).
3.3 Inflammation
Low-level inflammation (parainflammation) is essential for maintaining homeostasis and monitoring dysfunction of cells and tissue. Once the optimal homeostatic balance is broke, excessive parainflammatory responses may lead to chronic inflammation and subsequent tissue dysfunction (Copland et al., 2018). AMD patients show high expression of inflammatory/immune-related factors in the eyes, retina and choroid can recruit many macrophages and microglia (Wooff et al., 2019). These are distributed along nonimmune cells (RPE and Müller cells) and participate in disease development (Nussenblatt et al., 2013). Macrophages have different effects, with macrophages in the young choroid helping Bruch’s membrane to clear precipitation and cholesterol, whereas macrophages may also have a destructive effect with age, participating in the formation of CNV by stimulating high expression of VEGF-A (Cherepanoff et al., 2010). According to the functional phenotype of polarization, macrophages can be divided into the classical activating type with anti-angiogenesis function (M1) and the surrogate-activated type with pro-angiogenesis function (M2) (Funes et al., 2018), and the M1/M2 macrophage ratio can regulate the development of CNV (Lutty and McLeod, 2018). Transition of macrophages from a protective mechanism to a destructive mechanism may be related to the disruption of the build-clear homeostasis of lipid oxidation products with age. In addition, studies in humans and rodents have shown that macrophages are the main infiltrating cells of CNV (Campochiaro, 2015). IL-6 produced by macrophages stimulates CNV formation by classical activation of IL-6 receptor (IL-6R)-positive macrophages (Droho et al., 2021). Another immune cell was found to exist each stage of AMD is mast cell, and some researchers have speculated that mast cells may be involved in regulating pro-inflammatory and anti-inflammatory responses, resulting in damage to the vascular wall and Bruch’s membrane, RPE degradation and thinning of the choriocapillaris and other pathological changes that promote CNV production (Bhutto et al., 2016).
3.4 Angiogenesis
Recent clinical and histologic features studies suggests that non-exudative Type 1 CNV acts as a compensation mechanism, providing nutritional support and functional maintenance for outer retinal photoreceptors. This mechanism mainly causes by aggravating hypoxia in the complex of RPE-BrM-choriocapillaris, induced by the drop out of choroidal capillaries, and accumulation of local lipid components and cellular fragments which hinder exchange of oxygen and nutrients. When the compensatory equilibrium is broken, neovascularization occurs, breaking through BrM to form type 2 CNV (Chen et al., 2020) (Figure 2).
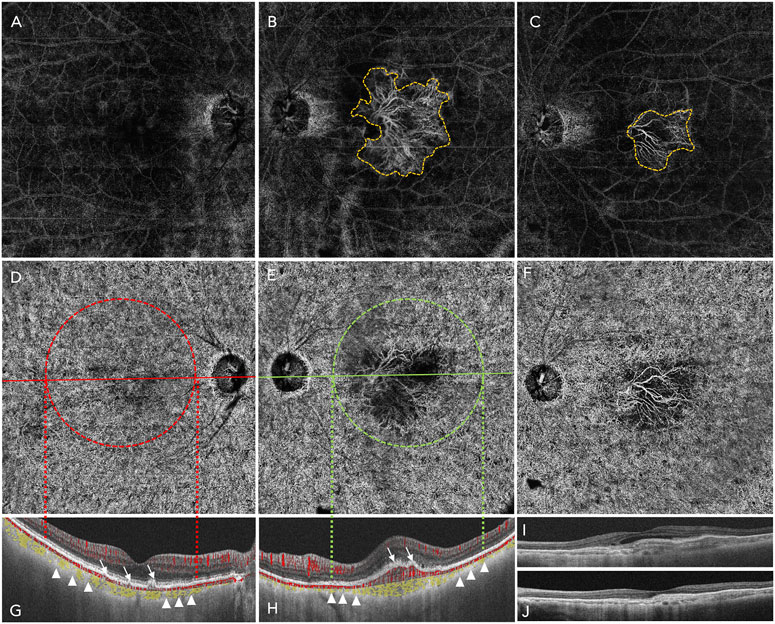
FIGURE 2. A 12 mm × 12 mm wide-field swept-source optic coherence tomography angiography (SS-OCTA) image of an 80-year-old woman who complained of vision loss of her left eye for 2 weeks. She was diagnosed of early AMD of right eye and NVAMD of left eye. (A–C) shows the avascular lay (defined by the software from the out plexiform layer to Bruch’s membrane of the retina): no abnormal blood vessel grows in the right eye (A); the yellow dashed line outlines a fan-like treat-naïve type 2 choroidal neovascularization (CNV) of the left eye (B); 1 month after the patient received an intravitreal anti-VEGF therapy of Aflibercept 2.0 mg, the area of type 2 CNV reduced markedly compared to the baseline (C). (D,E) shows the choriocapillaris layer. (G,H) shows the B-scan of the right eye (G) and left eye (H) accordingly: the white arrows of the right eye indicates the deposition of extracellular debris (drusen within which there is no blood signals) and the white arrows of the left eye indicates the CNV invasion into the subretinal space with blood signals (red), and the yellow area lining with the choroid shows that the NVAMD eye is thinner than the early stage AMD eye (small white triangles), this is the phenomenon of the drop out of choriocapillaris of NVAMD. (I,J) showed the B -scan of the left eye: after treatment, the intraretinal edema was absorbed, and the central retina thickness reduced.
The pathogenesis of CNV is complex, and vascular endothelial growth factor (VEGF) is one of the main regulators of angiogenesis in NVAMD, specifically acting on vascular endothelial cells to promote proliferation, induce the generation of new blood vessels, and increase vascular leakage (Campochiaro, 2015). VEGF is mainly bound to vascular endothelial growth factor receptor 1 and 2 (VEGFR-1 and VEGFR-2), which is widely distributed in chorioretinal endothelial cells (Simons et al., 2016). During the cascade of angiogenesis, binding to VEGFR-2 is the most important process (Ferrara et al., 2003). VEGFR2 in endothelial cells is transported to the cell surface, and its tyrosine kinase can be activated by VEGF-A to induce angiogenesis and increase vascular permeability (Ferrara et al., 2003; Waters et al., 2021). VEGFR1 can be used as bait to weaken VEGF-A-mediated angiogenesis and leakage. In addition, VEGFR1 activation leads to neovascularization, increases vascular permeability, and induces macrophages and microglia to produce proinflammatory and angiogenic mediators (Uemura et al., 2021).
4 Clinical trials of therapies for NVAMD
The first anti-VEGF therapy approved by the FDA in 2004 for the treatment of NVAMD was pegaptanib (Macugen®), a synthesized 28-base oligonucleotide (aptamer) that selectively neutralizes the VEGF165 isoform, being modified with two polyethylene glycol (PEG) to increase its intraocular half-life (Gao and Talalay, 2004; Gragoudas et al., 2004). Bevacizumab (Avastin®) is a first-generation humanized monoclonal antibody to VEGF-A that is effective against angiogenesis via systemic and intravitreal injection (Michels et al., 2005). Subsequently, the second-generation monoclonal antibody ranibizumab (Lucentis®) was approved by the FDA for NVAMD by intravitreal administration in 2006 (Rosenfeld et al., 2006). Ranibizumab contains an antigen-binding fragment (Fab) that binds and inhibits all identified human VEGF isoforms (Ferrara et al., 2006). The third FDA-approved drug targeting ocular VEGF is the cytokine trap aflibercept. Aflibercept is a fusion protein composed of the extracellular domain of VEGF1 and VEGF2 receptors bound to the Fc portion of human IgG1 and acts as a decoy receptor, blocking the binding of VEGF to its receptors and inhibiting downstream signaling and angiogenesis (Holash et al., 2002).
The MARINA trial (NCT00056836) enrolled 716 patients. At 12 months, 94.5% of the patients given 0.3 mg of ranibizumab and 94.6% of those given 0.5 mg lost fewer than 15 letters compared with 62.2% of patients receiving sham injections, and the benefit in visual acuity was maintained at 24 months (Rosenfeld et al., 2006; Mitchell et al., 2010). The ANCHOR trial (NCT00061594) reported the inspiring result that ranibizumab was superior to verteporfin as an intravitreal treatment of predominantly classic NVAMD, with low rates of serious ocular adverse events. Treatment improved visual acuity on average at 1 year (Brown et al., 2006).
Although the remarkably good results from all prior trials are exciting, anti-VEGF therapy still faces challenges and limitations. In real-world studies, many patients with NVAMD are not treated at optimal frequency, which is not the same as that observed in precisely designed and conducted clinical trials (Mehta et al., 2018; Rao et al., 2018). In clinical practice, poor compliance also reflects the burden that frequent treatment places on patients and healthcare systems (Cohen et al., 2013; Finger et al., 2013).
To reduce the frequency of IVT or extend the gap of IVT, other strategies are developing. In the VIEW 1 and VIEW 2 trials, intravitreal injection (IVT) of 2 mg aflibercept every 2 months were noninferior to monthly injections of 0.5 mg ranibizumab (Heier et al., 2012). A port delivery system (PDS), a fillable, continuously administered intraocular implant filled with 100 mg/mL ranibizumab for sustained drug release, was developed. However, this PDS device must be surgically sewn into the scleral wall, and the overall price is not advantageous compared to current intraocular injections (Campochiaro et al., 2019). Brolucizumab is a 26 kDa humanized antibody fragment targeting VEGF-A. It has a smaller molecular weight than ranibizumab (48 kDa) and aflibercept (115 kDa), but the molar concentration is 13 times that of aflibercept (Holz et al., 2016). In the HAWK and HARRIER clinical trials, brolucizumab achieved the primary efficacy endpoint of nondisadvantage compared with aflibercept; the central retinal thickness (CRT) was more reduced, the anatomy was better visualized, and 5%–7% of HAWK patients and 5% to 2% of HARRIER patients could maintain a 12-week administration interval up to 48 weeks (Dugel et al., 2020). Among the treated patients who completed 12-week dosing intervals, 82% of patients in the HAWK trial and 75% of patients in the HARRIER trial were in the first 2A stage and still maintained the 12-week dosing interval (Dugel et al., 2020). Brolucizumab has been shown to have a longer persistence in vision and greater anatomical improvement, which is expected to reduce the number of injections in patients and reduce the burden of treatment (Nguyen et al., 2020; Dugel et al., 2021).
5 Clinical trials of therapies for NVAMD
5.1 Novel anti-VEGF drugs/analogs
Abicipar-pegol (Allergan) is a DARPin molecule that contains engineered ankyrin repeat domain(s) targeting VEGF-A with high specificity and affinity and a longer ocular half-life (Rodrigues et al., 2018). The Phase III SEQUOIA and CEDAR trials (NCT02462928, NCT02462928) recruited patients with treatment naïve NVAMD, and abicipar showed comparable best corrected visual acuity (BCVA) and CRT up to 52 weeks with fewer injections than ranibizumab (Ferro Desideri et al., 2020; Kunimoto et al., 2020). However, because of unfavorable inflammation occurrence and risk-benefit ratio, it did not gain approval from FDA in 2020 (Kunimoto et al., 2020).
OPT-032 is a fusion protein of Fc fragments of VEGFR-3 and human IgG1 for binding VEGF-C and VEGF-D (Joukov et al., 1996). The results of the Phase IIb clinical trial (NCT003345082) presented at EURETINA 2019, and recent public results showed that treatment with 2.0 mg OPT-032 in combination with ranibizumab in patients with NVAMD achieved a statistically significant average visual acuity compared with ranibizumab alone, with a good safety profile indicating that the combination of VEGF-A, VEGF-C and VEGF-D to inhibit CNV as a treatment for NVAMD may have great potential (Jackson et al., 2023). Two Phase III clinical trials (ShORe trial NCT04757610; COAST trial NCT 04757636) are ongoing.
BAT5905 (Bio-Thera) is a recombinant humanized full monoclonal antibody targeting VEGF. A Phase I clinical trial (NCT04151212) to evaluate the safety and pharmacokinetics of BAT5905 (single dose starting from 0.3 mg, intravitreal injection (IVT) in patients with NVAMD when the injection dosage escalates is underway. The secondary objective is to evaluate the immunogenicity profile. To date, no results have been reported.
5.2 Novel targets
5.2.1 Platelet-derived growth factor
Platelet-derived growth factor (PDGF) mediates the recruitment and maturation of pericytes, providing VEGF-A and other growth factors via paracrine signaling (Caporarello et al., 2019). Studies have suggested that limitations of anti-VEGF-A therapy may be related to pericytes (Jo et al., 2006) Pegpleranib (Fovista®, Ophthotec) is aPEGylated DNA aptamer that binds to PDGF-BB and blocks PDGF binding to PDGF receptor-β (PDGFRβ) on pericytes, resulting in the death of cells (Jaffe et al., 2016; Jaffe et al., 2017). A Phase IIb clinical trial (NCT01089517) included 499 patients with NVAMD, and the results showed that visual acuity improved by 10.6 letters in the treatment group of pegpleranib 1.5 mg plus ranibizumab 0.5 mg, while an improvement of only 6.5 letters was observed in the ranibizumab monotherapy group (Jaffe et al., 2017). However, the Phase III (NCT01940900) trial failed to replicate the success of the Phase II trial (Rosenfeld and Feuer, 2018). HL-217 is a synthetic inhibitor targeting PDGF-BB: PDGFRβ signaling pathway, which effectively inhibits subretinal neovascularization when applied topically in rodent studies (Kim et al., 2015). A Phase I clinical trial (NCT03648346) has already finished, and HL-217 is now entering Phase II trials (EudraCT number: 2019–000642-35). Rinucumab (an anti-PDGFRβ antibody) coformulated with aflibercept stopped its Phase II clinical trial (NCT02418754) because no visual or anatomical structure improvements were observed compared with aflibercept monotherapy at 3 months (Heier et al., 2020). To date, no strong evidence has shown that anti-PDGF drugs have reinforced benefits in NVAMD treatment.
5.2.2 CCR3
The eosinophil/mast cell chemokine receptor CCR3 is specifically expressed in choroidal neovascular endothelial cells in humans with AMD, and genetic or pharmacological targeting of CCR3 or eotaxin inhibited injury induced CNV in mice (Takeda et al., 2009). A 6-week Phase IIa trial of AKST4290 (Alkahest, Inc.), an orally administered small-molecule CCR3 inhibitor (400 mg twice per day), met the primary endpoint of achieving an increase in BCVA (mean BCVA improved by +7.0 letters; In 24 of the patients, the BCVA was stable or improved, with six (20/7%) gaining 15 letters or more) in patients with NVAMD. AKST4290 was also found to be safe and well tolerated, meeting the secondary endpoint of the trial (Stewart et al., 2022).
5.2.3 Tissue factor
Tissue factor (TF), known as coagulation factor III, is an integral membrane protein that triggers the blood coagulation cascade and is involved in many pathological processes (Harlos et al., 1994); it is also an angiogenic-specific receptor and the target molecule for fVII-targeted therapeutics (Hu et al., 2017). The expression of TF increases in the retinal pigment epithelium in NVAMD patients (Grossniklaus et al., 2002) but not in normal blood vessels (Belting et al., 2004). Anti-TF monoclonal antibodies can predominantly decrease the expression of TF, VEGF, and F4/80 and reduce the area of experimental CNV induced by laser photocoagulation of the retina in mice (Wang et al., 2014). ICON-1 (hl-con1) (Ionic Therapeutics) is an immunoconjugate protein against TF. Basically, it consists of two identical chains of proteins: human Factor VII (fVII) as the targeting domain and human immunoglobulin G1 Fc as the effector domain, functioning as binding to pathological vessels overexpressing tissue factor (Bora et al., 2003; Tezel et al., 2007). Although intravitreous administration of a single 0.3 mg dose of ICON-1 was safe and well tolerated, its biological activity was evident in a Phase I clinical trial (Wells et al., 2018), and the results reported from a Phase II clinical trial (NCT02358889) with improved BCVA as the primary outcome showed that hl-con1 0.3 mg in combination with ranibizumab 0.5 mg did not surpass ranibizumab 0.5 mg monotherapy. Another phase II trial (NCT03452527) showed that the CNV size assessed by OCT angiography in the ICON-1 0.6 mg maintenance therapy group did not exceed that in the ICON-1 0.6 mg combination therapy group (with aflibercept 2 mg). A new tissue factor monoclonal antibody, ICON-4, a potential therapeutic for NVAMD, is now beginning to enter clinical trials.
5.2.4 Fibroblast growth factor 2
Fibroblast growth factor 2 (FGF-2) modulates the function of many cell types by binding heparin and heparan sulfate, stimulating angiogenesis, normal wound healing, and tissue growth (Nugent and Iozzo, 2000). RBM-007 (Ribomic, Inc.) is an anti-FGF-2 aptamer that inhibits angiogenesis and scar formation (Matsuda et al., 2019; Nakamura, 2021). A Phase II trial (TOFU trial, NCT04200248) compared monthly IVT of RBM-007 alone and RBM-007 combined with aflibercept dosed every other month for 16 weeks. However, no results have been reported yet.
5.2.5 Angiopoietin-2
The angiopoietin/tyrosine kinase with immunoglobulin and epidermal growth factor homology domains (Ang/Tie) pathway plays an important role in regulating vascular development and maintenance (Maisonpierre et al., 1997; Saharinen et al., 2008; Thomas and Augustin, 2009). Angiopoietin-1 (Ang-1) binds and phosphorylates the Tie2 receptor to maintain stable vasculature and homeostasis, and angiopoietin-2 (Ang-2) inversely inhibits the Ang-1/Tie2 axis, inducing inflammation and pathologic new vessels (Hackett et al., 2000; Biel and Siemann, 2016). Nesvacumab (REGN910/SAR307746) is a fully human immunoglobulin G1 (IgG1) monoclonal antibody that specifically binds and inactivates Ang-2 with high affinity. A Phase I clinical trial (NCT01688960) of nesvacumab combined with aflibercept showed positive results in vision and anatomical structure improvement. However, in a Phase II clinical trial (the ONYX study, NCT02713204) comparing the efficacy of nesvacumab in combination with aflibercept and aflibercept monotherapy, the results did not suggest a significant difference (Brown et al., 2022). A Phase III clinical trial will not be conducted. However, clinical evidence still suggests a potential benefit of targeting the angiopoietin/Tie pathway and VEGF-A over anti-VEGF-A monotherapy alone (Sharma et al., 2020; Heier et al., 2021), as we will describe below.
5.3 Multitargets
Ang-2 and VEGF-A are simultaneously blocked with Faricimab (Hoffmann-LaRoche), a bispecific antibody that binds to both simultaneously and independently (Regula et al., 2019). A Phase II clinical trial (STAIRWAY NCT0303888) administered extended dosing regimens in seventy-six treatment-naïve participants with NVAMD. After the initial dose of faricimab 6 mg was administered by intravitreal injection (IVT) once every 4 weeks (Q4W), two different extended treatment plans of Q16W and Q12W were performed and compared with ranibizumab 0.5 mg IVT Q4W. The results showed that the visual improvement in extended treatment with faricimab was comparable to the effect of monthly ranibizumab use, and the mean total numbers of injections through Week 52 were 6.7, 6.2, and 12.9, respectively, which supports its potential to provide sustained efficacy (Khanani et al., 2020). In Phase III clinical trials TENAYA (NCT03823287) and LUCERNE (NCT03823300), patients were randomly assigned (1:1) to two groups:IVT of faricimab 6.0 mg up to every 16 weeks and aflibercept 2.0 mg every 8 weeks. In 1,329 patients with NVAMD, the improvement of BCVA with group of faricimab was noninferior to group of aflibercept. The mean change of letters in TENAYA was 5.8 letters and 5.1 letters in two group respectively, the treatment difference was 0.7 letters; In LUCERNE, the mean change of letters was 6.6 letters and 6.6 letters respectively, the treatment difference 0.0 letters; rates of ocular adverse events were comparable between groups (Heier et al., 2022). The results were promising to show that faricimab administered at interval up to 16-week has the potential to meaningfully extend the time between injections, thereby reducing the treatment burdens in patients (Chia and Keane, 2022; Heier et al., 2022). Vabysmo ® (faricimab, Genentech) was approved by the FDA in January 2023.
IBI302 (efdamrofusp alfa, Innovent Biologics, Suzou Co. Ltd.), an innovative bispecific decoy receptor fusion protein, inhibits both VEGF and the complement cascade connected by the fc region of human immunoglobulin (Ren et al., 2016; Wang et al., 2018). The Phase I clinical trial (NCT03814291) showed a mean improvement in BCVA of +6.0 letters by Day 29 and 6.1 letters by Day 43 after a single injection of IBI302 (Yang S. et al., 2022). Another Phase I clinical trial (NCT04370379) conducted the safety and tolerability evaluation of a multidose IVT of IBI302 every 4 weeks with a 3+ PRN regimen (initial 3 injections loading doses followed by IVT pro re nata). The results were first announced at the Annual Meeting of the American Academy of Ophthalmology (AAO) in January 2020 that IBI302 improved BCVA in patients with NVAMD, decreased central retinal thickness (CRT), and reduced CNV leakage and area, indicating that the overall safety and efficacy characteristics of IBI302 were the same as those of currently available clinical anti-VEGF drugs. A dose escalation Phase II trial (NCT04820452) is ongoing.
RC28-E (RemeGen) is a decoy receptor trap that blocks both VEGF and FGF-2. In preclinical studies, RC28-E was suggested to be more effective in inhibiting pathological angiogenesis than other VEGF antagonists in CNV (Jiang et al., 2018; Jiang et al., 2020). The Phase I and phase II clinical trials (NCT03777254, NCT04270669) were dose escalation studies of RC28-E (0.5–2 mg IVT loading dose plus PRN treatment) for up to 48 weeks. To date, no public results have been reported.
5.4 Tyrosine kinase receptor inhibitors (TKIs)
To date, most of the emerging therapies entering clinical trials discussed in this section are administered via intravitreal injection and have been applied as regular, ongoing treatments in monthly “treat-and-extend” (T&E) or pro re nata (PRN, or “as needed”) regimens (Mettu et al., 2021). Hence, replacement of IVT injections by noninvasive drug delivery, such as topical or oral agents, is under development (Zeitz and Joussen, 2017). Large peptides less able to penetrate ocular tissue membranes make topical administration challenging. Tyrosine kinase receptor inhibitors are cell-penetrating peptides that enhance cellular and tissue penetration, showing potential as topical anti-VEGF agents (Pescina et al., 2018). Pazopanib is a multitargeted TKI that inhibited VEGFR 1, 2, and 3 and PDGF signaling and reduced CNV size in a preclinical animal model (Takahashi et al., 2009). In a Phase II trial (NCT00612456), BCVA in patients with NVAMD was improved by topical administration of 5 mg mL-1 and 3 times daily of pazopanib; nevertheless, pazopanib was not able to achieve the primary endpoint in a Phase IIb trial (NCT01134055) at Week 52, neither did it reduce the number of ranibizumab injections by 50% nor was it inferior to ranibizumab (Danis et al., 2014; Csaky et al., 2015). Acrizanib (LHA510, Alcon) is a tyrosine kinase inhibitor administered via suspension eye drops (Adams et al., 2018). A Phase II clinical trial (NCT02355028) evaluated the efficacy of 84 successive days of topically administered LHA510 compared to vehicle in reducing the number of patients requiring ranibizumab IVT therapy for the recurrence of CNV. Initial results showed that the LHA510 group failed to gain superiority over the vehicle group at the endpoint (Poor et al., 2022). A Phase IIa trial (NCT02222207) of another therapeutic agent regorafenib which inhibits VEGF-R 2/3 and PDGFR, as a result of losing 2.4 EDTRS letters at 12 weeks, was discontinued (Joussen et al., 2019). Although the results for these drugs have not been favorable, the VEGFR2 tyrosine kinase inhibitor PAN-90806 (NCT0347937) with an improved formulation showed safety and biological response in NVAMD as monotherapy, accomplishing the goal of 51% of participants not receiving rescue anti-VEGF injections. These results were presented at the American Academy of Ophthalmology annual meeting.
Vorolanib (X-82, CM082) is an oral multikinase inhibitor that targets VEGFR, PDGFR and CSF1R. A Phase I clinical trial (NCT03038880) of dose escalation showed improvement in BCVA and CRT in recruited subjects (Jackson et al., 2017)). The results from a Phase II clinical trial (NCT02348359) showed that patients treated with X-82 gained noninferiority efficacy in improving visual acuity compared to those who received placebo and had a lower IVT burden. Some patients could even completely stop receiving IVT of anti-VEGF therapy. However, X-82 did not achieve sufficient risk-benefit, and its systemic toxicity limits its clinical application (Cohen et al., 2021).
GB-102 is a novel depot formulation of sunitinib malate, which is a bispecific tyrosine inhibitor of VEGFR-2 and PDGFRβ. The drug is encapsulated in a biodissolving polymer nanoparticle capsule to achieve a sustained release effect at 2 doses per year for the treatment of NVAMD. A Phase I clinical trial (NCT03249740) showed favorable tolerability and safety, with some patients maintaining stable vision up to 8 months. However, vision reduction occurred in the 2 mg therapeutic dose group due to the dispersion of particles into the anterior chamber. A new manufacturing process to eliminate particle dispersion and incomplete aggregation is under development. The purpose of the Phase IIb trial (NCT03953079) was to evaluate the safety and duration of the effect of GB-102 by measuring the time to first rescue treatment with IVT of GB-102 1 mg or 2 mg at Baseline and then 1 mg at month 6, compared to aflibercept administered every 2 months, and the patients recruited was received prior anti-VEGF therapy. The results showed that both doses of GB-102 elevated the visual acuity; interestingly, the primary outcome was 5 months (3–8 months) and 4 months (3–4 months) in group of GB-102 1mg and 2 mg administrated at Baseline respectively.
5.5 Gene therapy
AAV2-sFLT01 (Genzyme, Sanofi Company) is an AAV2 vector expresses SFLT01, a VEGF-neutralizing protein containing the VEGF/PlGF binding domain of human VEGFR1/Flt-1 (hVEGFR1) coupled to a Fc portion, and domains chained by polyplycine linker (Bagley et al., 2011). A Phase I clinical trial (NCT01024998) showed that intravitreous injection of AAV2-sFLT01 seemed to be safe and well tolerated at all doses (Heier et al., 2017), and NVAMD patients maintained stable visual improvement. In 19 patients, among 11 patients with reversible intraretinal or subretinal fluid at baseline, six showed a significant reduction of fluid and improvement in vision, whereas five did not. However, although the AAV2-sFLT01 group received a lower retreatment rate of ranibizumab compared with the control group, anti-VEGF drugs were still used as a supplement. Therefore, the significant advantages of a single injection of gene therapy have not been realized.
RGX-314 (Regenxbio Inc.) RGX-314 is being developed as a potential novel single gene therapy treatment for NVAMD via applanation of a novel NAV-AAV8 vector containing a gene for a monoclonal antibody fragment to neutralize VEGF (Khanani et al., 2022). Two phase II Randomized, dose-escalation trials (NCT04514653, NCT04832724) have been designed to evaluate whether RGX-314 gene therapy is effective, safe, and tolerable in subjects with NVAMD, no result has been public reported yet.
We selectively summarized and categorized the clinical trials of novel therapies for NVAMD (Table 1). Most of the clinical trials described above were stopped before Phase II/III; some succeeded but only showed noninferiority of efficacy compared with the currently available anti-VEGF monotherapy, which means it is not able to replace its counterpart. To maintain efficacy, patients must receive intraocular injections for life, usually every four to 12 weeks (Table 2 and Figure 3). Due to the treatment burden, patients often experience a decline in vision with reduced frequency of treatment over time. Thus, there are substantial unmet needs in the treatment of NVAMD.
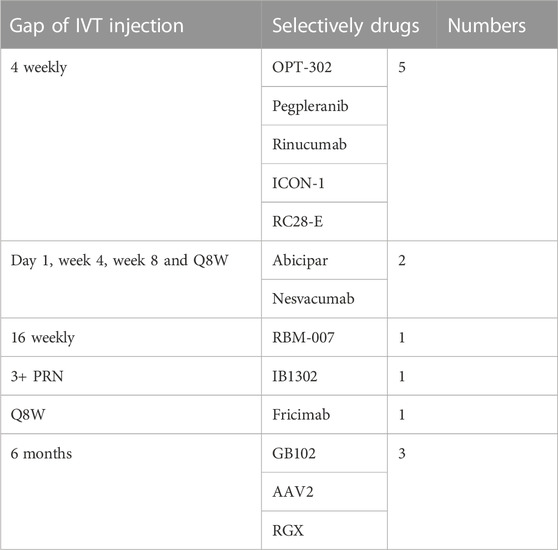
TABLE 2. IVT injection gap of part of clinical trials for NVAMD (A deuterogenic paradigm of column Dose regimen of Table 1).
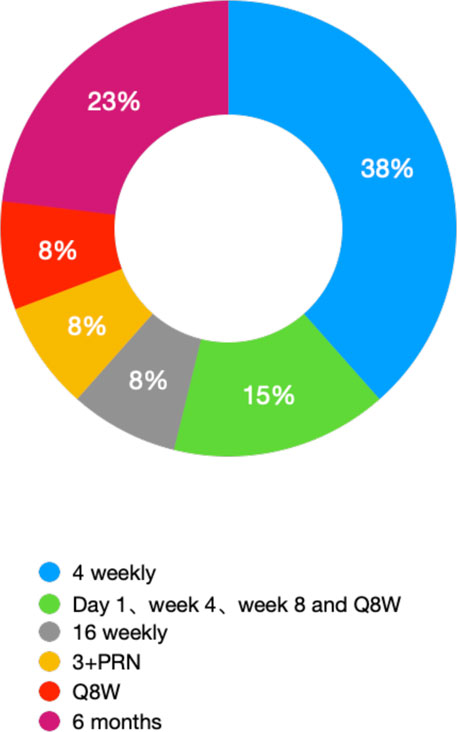
FIGURE 3. Ratio of different IVT gaps of treatment for NVAMD (statistic result form Table 2).
6 NVAMD drug delivery systems
To realize or enhance the activities of the therapeutics listed above, suitable delivery systems, especially long-term delivery systems for small molecular drugs or antibodies, are required (Campochiaro and Akhlaq, 2021). Various types of biomaterials, including hydrogels and microparticles, have been designed and fabricated as delivery systems for the long-term delivery of neovascularization inhibitors, e.g., anti-VEGF-A and recombinant fusion proteins, as replacements for intravitreal administration (Iyer et al., 2019; Jiang et al., 2020). However, due to the unique microenvironment of NVAMD and the strict requirements of the formulations for intravitreal injection, many of these systems are still being evaluated in preclinical assays, and there is a wide gap to be surmounted; they need to preserve the bioactivities of therapeutics; have a large payload (capacity), targeted delivery, sustained release (long period for drug delivery), and biocompatibility; and remain optically clear across the entire implanted or injected period (Kulkarni and Kuppermann, 2005; Fleckenstein et al., 2021).
The development and performance of port delivery systems (PDSs) in intravitreal delivery of anti-VEGF for long-term control of the progression of NVAMD must be mentioned. The release of the Phase I and Phase II clinical trial results of PDSs has demonstrated that PDSs not only realize long-term delivery of anti-VEGF with a significant reduction in administration time but also spur the further development of long-term delivery systems for intravitreal administration. Although it has been reported and highlighted by some other reviews, the importance and significance of its clinical trials are worthy of brief mention. PDSs are fabricated from nondegradable materials, mainly silicon-based walls and metal-based outlets, to form a hollow sealed tube with 20 µL space for cargo loading. A PDS can be implanted and fixed in the sclera, and the tube is inserted into the vitreous body at a depth of 4 mm; the outside end is an extrascleral flange with a self-sealing septum in the center for further refilling (Campochiaro et al., 2019), and the end inside the vitreous body is plugged with a metal element for controlled release of the cargo, which is typically anti-VEGF-A and ranibizumab. The most predominant advantage of a PDS is its performance in prolonging the interval between intravitreal injections. The median time to first refill is approximately 15.0 months when the PDS is initiated with a 100 mg/mL dosage (20 μL, 2 mg in total), and in general, the standard injection frequency is monthly injections of 0.5 mg ranibizumab. The visual and anatomic outcomes of the PDS 100 mg/mL arm were comparable to those of the monthly injection arm. From the aspect of pharmacokinetics, ranibizumab can still be detected in the serum, and the mean concentration is approximately 50.8 pg/mL 16 months after the implantation of the PDS (100 mg/mL) (Khanani et al., 2021). This further demonstrates the capacity of PDS in the long-term release of antibodies. The results of Phase I and Phase II clinical trials of PDSs further established the criteria for long-term intravitreal delivery of biomacromolecules (including anti-VEGF and recombinant fusion proteins, although the recombinant fusion proteins have not been loaded into PDSs and evaluated for their potential in prolonging alleviation of NVAMD): refillability; median time to first refill, ∼15.0 months; comparable NVAMD control with monthly injection of 0.5 mg ranibizumab; and low probability of adverse events. Under these criteria, some reported systems for biologics (biomacromolecules) delivery still need to be further improved (Figure 4).
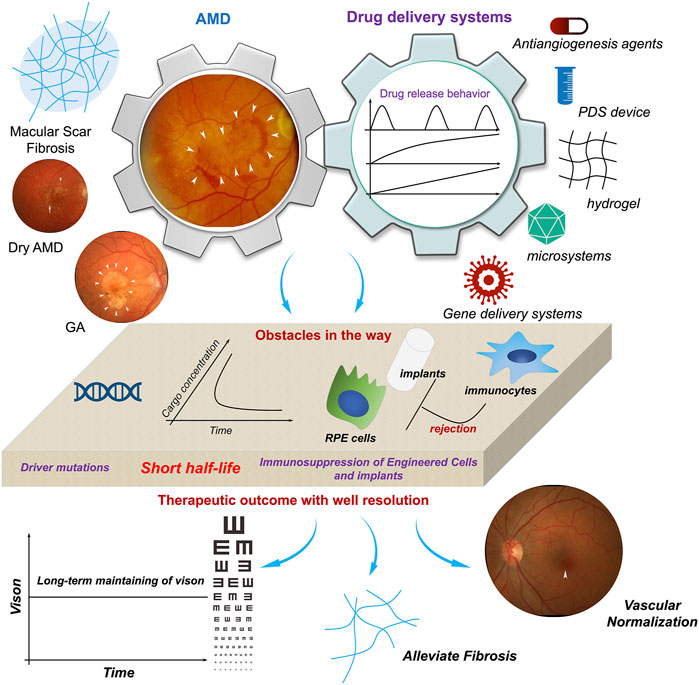
FIGURE 4. The potentials and obstacles of drug delivery systems utilized in AMD therapy. With finish machining, prolonged activities of therapeutics in AMD therapy as well as gene intervention can be achieved. However, the uncertainty of gene mutation controlling the initiation and progression of AMD, the short half-life of anti-VEGF-based therapeutics, and immunosuppression of host to engineered cells or biomaterials-based implants, etc. are impeding the realization of well management of AMD. It spurs the development of more ideal delivery system for AMD therapy. Adapted with permission from (Nat. Commun. 2019, 10, 3347) (Handa et al., 2019).
6.1 Hydrogel
The vitreous body contains more than 95% water and approximately 1% collagen and matrix, which can be considered a hydrogel system. Therefore, the utilization of hydrogels for biologic delivery is considered a suitable choice. Some promising results have been obtained in preclinical assays (Wang and Han, 2017; Ilochonwu et al., 2020). In theory, biologics can be loaded by hydrogels according to the solubility of the biologics. Furthermore, the in situ gelation properties impart the hydrogels with injectability, which not only favors intravitreal injection but also favors the loading of biologics with hydrogels by simple mixing. Among these injectable hydrogel systems, thermoresponsive hydrogels are promising. Hydrogels formed by block copolymers, e.g., PLGA-PEG-PLGA, PCL-PEG-PCL, PDLLA-PEG-PDLLA, have been reported as thermoresponsive hydrogels, and the sol-gel transition temperature can be fine-tuned at 37°C, the body temperature (Ballios et al., 2010). In an ex vivo environment (room temperature, 25°C), the hydrogels exhibit a solution form, and the biologics can be well dissolved and mixed with the hydrogel solution. After intravitreal injection, under the circumstances of body temperature, the hydrogel undergoes a sol-gel transition to form a hydrogel in situ, which encapsulates the biologics inside the network structure of the hydrogel, thus realizing controlled release of biologics (Gao et al., 2023). In ideal cases, these types of hydrogels are the most promising delivery systems for long-term delivery intravitreal injections. However, the drawbacks of the injectable hydrogels are also obvious. One concern is achieving slow release of the cargos; the release rate and duration time are controlled by the degradation of the hydrogels. Most hydrogel systems formed by block polyesters degrade less than 1 month after in vivo injection (Lin et al., 2021). Another issue that needs to be considered is whether the molecules of the degraded hydrogels aggravate the inflammation of the macular microenvironment. Both the PLGA- and PDLLA-based block copolymers degrade into lactic acid, which can exacerbate the inflamed microenvironment during the progression of NVAMD. Although an aflibercept-loaded thermoresponsive hydrogel has demonstrated a 6-month persistent release of aflibercept, from the perspective of long-term delivery of biologics, hydrogel systems still have to be further optimized.
6.2 Microparticles
Beyond macrobiologics, small molecular inhibitors are also promising candidates for inhibiting the progression of neovasculature, e.g., tyrosine kinase inhibitors (TKIs) (Tsujinaka et al., 2020). Many types of TKIs have been developed, including single-target and multitarget. Although most TKIs are fabricated for oral administration, the blood-eye barrier or blood‒retinal barrier and the inherent hydrophobic characteristics of TKI molecules dramatically reduce the bioavailability of TKIs in the area of focus of NVAMD. Meanwhile, it has been found that the degradation of some biomaterials approved by the FDA for implantation can be sustained for more than 2 years (PCL and PDLLA). The hydrophobic nature of these biomaterials favors the encapsulation of TKIs for long-term delivery. Sunitinib-loaded poly (ethylene glycol) methyl ether-b-poly (lactide-co-glycolide) (mPEG-PLGA) microparticles have achieved 6 months of sustained release of sunitinib with strong inhibition of type II CNV as well as alleviation of inflammation (Tsujinaka et al., 2020). In addition, fabricating small molecular drugs into microcrystals and combining them with hydrogels also achieved sustained release of axitinib (ClinicalTrial.gov, ID: NCT03630315), which has also been evaluated in patients with NVAMD. Therefore, microsystems (including microparticles and hydrogels) are more suitable for the long-term delivery of TKIs.
6.3 Fillable microparticles for pulsatile drug delivery
To achieve desirable drug delivery, the interactions between cargos and carriers need to be considered (Nazila et al., 2016; Mitchell et al., 2021), particularly the microstructures of the carriers, material compatibility, drug distribution inside the microstructures, and the effect of the technique used to fabricate microsystems on the activities of cargos, especially biologics. If the cargos can be encapsulated into a fully enclosed microbox, not only can the bioactivities of cargos be maintained at their initiating station, but the release behavior of the microbox can also be controlled by the properties of the microbox wall. Recently, a microfabrication method termed SEAL (stamped assembly of polymer layers) has been developed to fabricate this type of microbox (sealed particles) with dimensions of 400 × 400 × 300 μm and a cavity dimension of 200 × 200 × 100 μm (McHugh et al., 2017). The most interesting feature of this microbox system is the realization of pulsatile drug delivery after mixing microboxes with different degradation behaviors. The pulsatile drug delivery performance was verified by encapsulating OVA and STING agonists to inhibit tumor growth in vivo. By fine-tuning the molecular weight of the polymer used to construct the wall of the microboxes, a release as long as 100 days can be triggered (Lu et al., 2020). Pulsatile drug delivery behavior is the ideal method for long-term delivery, which can avoid burst release. However, for intravitreal long-term delivery (16 months), the time still needs to be further prolonged.
6.4 Matrix scaffold simulation for cell delivery
As AMD develops, it progresses from neovascular AMD to exudative or “wet” AMD and then to atrophic “dry” AMD. During AMD progression, impairment and irreversible cell loss are more or less constant. The primary cell type is retinal pigment epithelium (RPE) cells. RPE cells play a critical role in maintaining vison. In the case of NVAMD, RPE cells, which form a tight, smooth cell layer in normal eyes (Bruch’s membrane), may be ruptured due to expansion of macular edema but are not yet lost. In the late stage of AMD, severe amounts of RPE cells are lost. Thus, cell transplantation or cell therapy has become the only efficient way to rescue vision from blindness (Schnichels et al., 2021; Sugita et al., 2021). In 2015, in the first clinical trial related to use autologous induced-pluripotent derived stem cell (iPSC) transplants as treating NVAMD, there was only one subject (Garber, 2015). Even with favorable preclinical experiments and an intact transplanted iPSC derived RPE cell sheet under the retina 1 year after surgery, no alterations of BCVA were observed (Kamao et al., 2014; Mandai et al., 2017a; Mandai et al., 2017b). To realize cell transplantation, a scaffold for cell delivery is required to avoid draining the implanted cells. Human-vitronectin-coated polyester membranes have been evaluated for their performance as RPE cell scaffolds. RPE cells can be derived from human embryonic stem cells (hESCs). The cell density of the implant patch was ∼6,000 cells per mm2. The cells cultured in the patch can be induced to differentiate and form a barrier that is as tight as the native configuration. The polyester used in this patch is 10-μm thick polyethylene terephthalate (PET), with a 0.4-μm pore size at a density of 1 × 108 pores/cm2 (Sterlitech, Kent, Washington, USA) (da Cruz et al., 2018). Vitronectin is a multifunctional glycoprotein that can connect glycosaminoglycans, collagens, and profibrinolysins in the blood and the extracellular matrix. Thus, the combination of polyester and vitronectin forms a matrix to support the in vivo in situ transplantation of RPE cells and their survival for 12 months with only local immunosuppression (Sharma et al., 2019). This strategy can achieve long-term resolution for the control of AMD progression; however, its potential has only been verified in late AMD, in the form of “dry” AMD or geographic atrophy, but not in wet AMD or neovascular AMD. In addition, the immunosuppression possibility of hESC-derived RPE cells, which may result in ocular morbidity, and the effect of the foreign body reaction (FBR) of the scaffold on long-term transplantation also need to be further investigated (Yang Q. et al., 2022).
6.5 Gene mutation during AMD
The formation and progression of AMD is considered to be regulated by the combinational interactions of multiple factors: aging is the predominant factor, and it is also the main driver that stimulates the overexpression of VEGF-A in the macular microenvironment in elderly patients (aging induces choriocapillaris depletion and results in the hypoxia of RPE cells, thus promoting the overexpression of HIF-α and VEGF-A by RPE cells and the degeneration of RPE cells and the Bruch’s membrane) (Botto et al., 2021; de Guimaraes et al., 2021), which makes VEGF-A/HIF-α -related genes (vegf, vegfr, pdgf, pegf) primary targets for gene therapy (de Guimaraes et al., 2021). AAVs and nonviral delivery systems have been used for the delivery of RNAs or DNAs targeting VEGF-A or hypoxia-inducing factor 1α (HIF-α, a gene controlling the metabolism of cells in the macular microenvironment and promoting the formation of inflammation after transcription), such as lentiviral codelivery of Streptococcus pyogenes Cas9 mRNA and RNA targeting VEGF-A or intravitreal delivery of LbCpf1 by AAVs cotargeting VEGF-A and HIF-1α (Koo et al., 2018; Shen et al., 2020; Ling et al., 2021). With the introduction of CRISPR, the therapeutic activities of gene therapy have been further improved. However, beyond VEGF-A, whether the formation and progression of AMD are genetically controlled and which genes are involved, e.g., the K-RAS gene, in promoting the formation of tumors remain to be further clarified. It is speculated that the probability of late AMD containing an inherited feature is ∼70%, while the other ∼30% of uncertainty is ascribed to other factors, including smoking and environmental risk factors (Seddon et al., 2005; Fritsche et al., 2016). With the development of single-cell sequencing and genome-wide association studies (GWASs), the mutations and factors promoting the progression of AMD have been gradually uncovered. Through GWASs, CFH (rs1061170, p.Y402H) 44–47 on chromosome 1 and two neighboring genes, ARMS2 and HTRA1, on chromosome 10, have been identified as two major loci that are closely associated with late AMD (Edwards et al., 2005; Hageman et al., 2005). The CFH variant is mainly associated with drusen area, while the ARMS2-HTRA1 variant is associated with subretinal or sub-RPE hemorrhages (van Asten et al., 2018). Other genes, such as MMP9, CETP and TIMP3, are mainly linked to NVAMD due to their regulatory capacities in ECM remodeling (Fritsche et al., 2016). The FGD6, HTRA1 and CFH genes play critical roles in regulating oxidative stress and inflammation, which control the progression of angiogenesis, thus promoting the aggravation of NVAMD (Huang et al., 2016). However, to our knowledge, no study has reported the development of therapeutics targeting these uncovered genes. The main targets for gene therapy in AMD are still VEGF-related loci, including VEGFR, PDGF and PEGF, as well as HIF-α. The genetic drivers controlling the initiation and progression of AMD still need to be further investigated. Before the discovery of mature targets for gene intervention in AMD, efforts should focus on the development and optimization of gene delivery systems, e.g., optimization of ionizable lipid nanoparticles (Han et al., 2021) and the development of zwitterionic networks (Blackman et al., 2019; Li et al., 2020). This further promotes the invention of gene therapy, which is also required for suitable gene delivery systems.
7 Perspectives
Age-related macular degeneration is a lethal threat to the vision of older people. The progression of AMD has become a major cause of blindness. Although in-depth study of the driver mutations that initiate the formation and promote the progression of AMD is ongoing, the predominant strategies for NVAMD management are VEGF-A-based antiangiogenics. The developed therapeutics are VEGF antibodies or recombinant fusion proteins (aflibercept and conbercept). While TKIs have proven their potential in inhibiting angiogenesis, their useability in intravitreal administration still needs further thorough investigation. Antiangiogenesis via anti-VEGF-A has shown promising clinical outcomes; however, monthly repetitive intravitreal injection of antibodies only achieves limited alleviation of NVAMD, and it is far from achieving essential control of NVAMD progression. Drug delivery systems have been introduced in NVAMD therapy to impart biological therapeutics with long-term delivery capacity. Due to their reported potential in redistribution, drug release control, and off-target release reduction of cargos, biomaterials are expected to be ideal carriers for long-term delivery. However, in NVAMD therapy, only PDSs have achieved long-term performance in delivering VEGF-A antibodies in Phase II trials, while a Phase III clinical trial has been initiated. A PDS is formed by totally undegradable materials (silicon- and metal-based materials) with refillable properties and provides a median time to first refill of ∼15 months. In the case of biomaterial-based delivery systems, injectable hydrogels can only be maintained for one or 2 months. Even hydrophobic polyester (PCL or PLA) can maintain the bulk structure for even 2 years, its inherent hydrophobicity restricts its application to the long-term delivery of hydrophobic small molecular inhibitors, e.g., TKIs. Recently, microboxes fabricated by fine-tuning techniques have been shown to achieve long-term delivery of versatile types of therapeutics, and their potential in intravitreal administration needs further evaluation. Under such circumstances, gene therapy may provide a way to reduce repetitive administration in NVAMD, but the multifactorial character of NVAMD means that gene therapy will require considerable effort to realize desirable therapeutic outcomes.
Besides, numerous studies have shown that both inflammation and oxidative stress-induced retinal pigment epithelial cell damage are involved in the pathogenesis of abnormal vascular development in AMD (AMD). Conventional anti-VEGF therapy (ranibizumab) inhibits the growth of neovascular; however, the adverse effects would occur during the repeated monthly intravitreal injections. Some nano-biomaterials with satisfied biocompatibility and biostability are designed to control the intracellular inflammatory signals by scavenging the reactive oxygen species (ROS), prevent excessive inflammatory activation of innate and adaptive immune cells, and maintain redox balance in biological systems (Yang et al., 2023). In addition, these nanoplatforms can deliver anti-inflammatory agents to the disease region. The anti-VEGF-A antibody-loaded betasone phosphate-based hydrogel (BetP-Gel) not only provides long-term sustained release of anti-VEGF to inhibit retinal vascular proliferation and attenuate choroidal neovascularization, but also scavenge ROS to reduce local inflammation. This BetP-Gel can significantly prolong the effective time of conventional anti-VEGF therapy with a potential clinical application (Gao et al., 2023).
In summary, all the existing challenges inspire us to rethink the innovative development of NVAMD therapeutic strategies, not only to further identify and classify the risk factors that control the initiation and progression of NVAMD (including genetic risk loci and environmental risk factors) but also to design and construct delivery systems with a brand-new perspective from the combination of pathology and materials as well as engineering.
Author contributions
XH wrote the draft; XH, QY and JP conceived and structured this review; LZ, YF and MZ did literature search for this work; QY and JP revised the draft. The manuscript has been read and approved by all named authors. All authors listed have made a substantial, direct, and intellectual contribution to the work and approved it for publication.
Funding
National Natural Science Foundation of China (NSFC32171376, 31871008); the Key Research and Development Program of Sichuan Province (2022YFS0192); the Science and Technology Program for Distinguished Young Scholar of Sichuan Province (grant number: 2020JDJQ0048), Sichuan Provincial Science and Technology Support Project(2021ZYD0110).
Conflict of interest
The authors declare that the research was conducted in the absence of any commercial or financial relationships that could be construed as a potential conflict of interest.
Publisher’s note
All claims expressed in this article are solely those of the authors and do not necessarily represent those of their affiliated organizations, or those of the publisher, the editors and the reviewers. Any product that may be evaluated in this article, or claim that may be made by its manufacturer, is not guaranteed or endorsed by the publisher.
Abbreviations
A2E, N-retinylidene-N-retinylethanolamine; AMD, age-related macular degeneration; Ang/Tie, angiopoietin/tyrosine kinase; BCVA, best corrected visual acuity; BlinD, basal linear deposits; BrM, Bruch’s membrane; CNV, choroidal neovascularization; CRT, central retinal thickness; Fab, antigen-binding fragment; FGF-2, fibroblast growth factor 2; GA, geographic atrophy; hESCs, human embryonic stem cells; ICAM, intercellular adhesion factor; MAC, membrane attack complex; MNV, macular neovascularization; NVAMD, neovascular AMD; OCT, optical coherence tomography; PDS, port delivery system; PDGF, platelet-derived growth factor; PDGFRβ, PDGF receptor-β; PED, pigment epithelium detachment; PRN, pro re nata; RPE, retinal pigment epithelium; SEAL, stamped assembly of polymer layers; T&E, treat-and-extend; TKI, tyrosine kinase inhibitor; VEGF, vascular endothelial growth factor.
References
Adams, C. M., Anderson, K., Artman, G., Bizec, J. C., Cepeda, R., Elliott, J., et al. (2018). The discovery of N-(1-Methyl-5-(trifluoromethyl)-1H-pyrazol-3-yl)-5-((6- ((methylamino)methyl)pyrimidin-4-yl)oxy)-1H-indole-1-carboxamide (acrizanib), a VEGFR-2 inhibitor specifically designed for topical ocular delivery, as a therapy for neovascular age-related macular degeneration. J. Med. Chem. 61, 1622–1635. doi:10.1021/acs.jmedchem.7b01731
Apte, R. S. (2021). Age-related macular degeneration. New Engl. J. Med. 385, 539–547. doi:10.1056/nejmcp2102061
Bagley, R. G., Kurtzberg, L., Weber, W., Nguyen, T. H., Roth, S., Krumbholz, R., et al. (2011). sFLT01: a novel fusion protein with antiangiogenic activity. Mol. Cancer Ther. 10, 404–415. doi:10.1158/1535-7163.mct-10-0813
Ballios, B. G., Cooke, M. J., Van Der Kooy, D., and Shoichet, M. S. (2010). A hydrogel-based stem cell delivery system to treat retinal degenerative diseases. Biomaterials 31, 2555–2564. doi:10.1016/j.biomaterials.2009.12.004
Belting, M., Dorrell, M. I., Sandgren, S., Aguilar, E., Ahamed, J., Dorfleutner, A., et al. (2004). Regulation of angiogenesis by tissue factor cytoplasmic domain signaling. Nat. Med. 10, 502–509. doi:10.1038/nm1037
Bhutto, I. A., Baba, T., Merges, C., Juriasinghani, V., Mcleod, D. S., and Lutty, G. A. (2011). C-reactive protein and complement factor H in aged human eyes and eyes with age-related macular degeneration. Br. J. Ophthalmol. 95, 1323–1330. doi:10.1136/bjo.2010.199216
Bhutto, I. A., Mcleod, D. S., Jing, T., Sunness, J. S., Seddon, J. M., and Lutty, G. A. (2016). Increased choroidal mast cells and their degranulation in age-related macular degeneration. Br. J. Ophthalmol. 100, 720–726. doi:10.1136/bjophthalmol-2015-308290
Biel, N. M., and Siemann, D. W. (2016). Targeting the Angiopoietin-2/Tie-2 axis in conjunction with VEGF signal interference. Cancer Lett. 380, 525–533. doi:10.1016/j.canlet.2014.09.035
Biesemeier, A., Taubitz, T., Julien, S., Yoeruek, E., and Schraermeyer, U. (2014). Choriocapillaris breakdown precedes retinal degeneration in age-related macular degeneration. Neurobiol. Aging 35, 2562–2573. doi:10.1016/j.neurobiolaging.2014.05.003
Blackman, L. D., Gunatillake, P. A., Cass, P., and Locock, K. E. (2019). An introduction to zwitterionic polymer behavior and applications in solution and at surfaces. Chem. Soc. Rev. 48, 757–770. doi:10.1039/c8cs00508g
Bora, P. S., Hu, Z., Tezel, T. H., Sohn, J. H., Kang, S. G., Cruz, J. M., et al. (2003). Immunotherapy for choroidal neovascularization in a laser-induced mouse model simulating exudative (wet) macular degeneration. Proc. Natl. Acad. Sci. U. S. A. 100, 2679–2684. doi:10.1073/pnas.0438014100
Botto, C., Rucli, M., Tekinsoy, M. D., Pulman, J., Sahel, J.-A., and Dalkara, D. (2021). Early and late stage gene therapy interventions for inherited retinal degenerations. Prog. Retin Eye Res. 86, 100975. doi:10.1016/j.preteyeres.2021.100975
Brown, D. M., Boyer, D. S., Csaky, K., Vitti, R., Perlee, L., Chu, K. W., et al. (2022). Intravitreal nesvacumab (antiangiopoietin 2) plus aflibercept in diabetic macular edema: Phase 2 RUBY randomized trial. Retina 42, 1111–1120. doi:10.1097/iae.0000000000003441
Brown, D. M., Kaiser, P. K., Michels, M., Soubrane, G., Heier, J. S., Kim, R. Y., et al. (2006). Ranibizumab versus verteporfin for neovascular age-related macular degeneration. N. Engl. J. Med. 355, 1432–1444. doi:10.1056/nejmoa062655
Campochiaro, P. A., Aiello, L. P., and Rosenfeld, P. J. (2016). Anti-vascular endothelial growth factor agents in the treatment of retinal disease: From bench to bedside. Ophthalmology 123, S78–S88. doi:10.1016/j.ophtha.2016.04.056
Campochiaro, P. A., and Akhlaq, A. (2021). Sustained suppression of VEGF for treatment of retinal/choroidal vascular diseases. Prog. Retin Eye Res. 83, 100921. doi:10.1016/j.preteyeres.2020.100921
Campochiaro, P. A., Marcus, D. M., Awh, C. C., Regillo, C., Adamis, A. P., Bantseev, V., et al. (2019). The port delivery system with ranibizumab for neovascular age-related macular degeneration: Results from the randomized phase 2 ladder clinical trial. Ophthalmology 126, 1141–1154. doi:10.1016/j.ophtha.2019.03.036
Campochiaro, P. A. (2015). Molecular pathogenesis of retinal and choroidal vascular diseases. Prog. Retin Eye Res. 49, 67–81. doi:10.1016/j.preteyeres.2015.06.002
Caporarello, N., D'angeli, F., Cambria, M. T., Candido, S., Giallongo, C., Salmeri, M., et al. (2019). Pericytes in microvessels: From "mural" function to brain and retina regeneration. Int. J. Mol. Sci. 20, 6351. doi:10.3390/ijms20246351
Chen, L., Messinger, J. D., Sloan, K. R., Swain, T. A., Sugiura, Y., Yannuzzi, L. A., et al. (2020). Nonexudative macular neovascularization supporting outer retina in age-related macular degeneration: A clinicopathologic correlation. Ophthalmology 127, 931–947. doi:10.1016/j.ophtha.2020.01.040
Cherepanoff, S., Mcmenamin, P., Gillies, M. C., Kettle, E., and Sarks, S. H. (2010). Bruch's membrane and choroidal macrophages in early and advanced age-related macular degeneration. Br. J. Ophthalmol. 94, 918–925. doi:10.1136/bjo.2009.165563
Cheung, C. M., and Wong, T. Y. (2014). Is age-related macular degeneration a manifestation of systemic disease? New prospects for early intervention and treatment. J. Intern Med. 276, 140–153. doi:10.1111/joim.12227
Chia, M. A., and Keane, P. A. (2022). Beyond anti-VEGF: Can faricimab reduce treatment burden for retinal disease? Lancet 399, 697–699. doi:10.1016/s0140-6736(22)00105-2
Cohen, M. N., O'shaughnessy, D., Fisher, K., Cerami, J., Awh, C. C., Salazar, D. E., et al. (2021). APEX: A phase II randomised clinical trial evaluating the safety and preliminary efficacy of oral X-82 to treat exudative age-related macular degeneration. Br. J. Ophthalmol. 105, 716–722. doi:10.1136/bjophthalmol-2020-316511
Cohen, S. Y., Mimoun, G., Oubraham, H., Zourdani, A., Malbrel, C., Quere, S., et al. (2013). Changes in visual acuity in patients with wet age-related macular degeneration treated with intravitreal ranibizumab in daily clinical practice: The LUMIERE study. Retina 33, 474–481. doi:10.1097/iae.0b013e31827b6324
Copland, D. A., Theodoropoulou, S., Liu, J., and Dick, A. D. (2018). A perspective of AMD through the eyes of immunology. Invest. Ophthalmol. Vis. Sci. 59, AMD83–AMD92. doi:10.1167/iovs.18-23893
Csaky, K. G., Dugel, P. U., Pierce, A. J., Fries, M. A., Kelly, D. S., Danis, R. P., et al. (2015). Clinical evaluation of pazopanib eye drops versus ranibizumab intravitreal injections in subjects with neovascular age-related macular degeneration. Ophthalmology 122, 579–588. doi:10.1016/j.ophtha.2014.09.036
Da Cruz, L., Fynes, K., Georgiadis, O., Kerby, J., Luo, Y. H., Ahmado, A., et al. (2018). Phase 1 clinical study of an embryonic stem cell–derived retinal pigment epithelium patch in age-related macular degeneration. Nat. Biotechnol. 36, 328–337. doi:10.1038/nbt.4114
Danis, R., Mclaughlin, M. M., Tolentino, M., Staurenghi, G., Ye, L., Xu, C. F., et al. (2014). Pazopanib eye drops: A randomised trial in neovascular age-related macular degeneration. Br. J. Ophthalmol. 98, 172–178. doi:10.1136/bjophthalmol-2013-303117
De Guimaraes, T. . a. C., Georgiou, M., Bainbridge, J. W., and Michaelides, M. (2021). Gene therapy for neovascular age-related macular degeneration: Rationale, clinical trials and future directions. Br. J. Ophthalmol. 105, 151–157. doi:10.1136/bjophthalmol-2020-316195
Droho, S., Cuda, C. M., Perlman, H., and Lavine, J. A. (2021). Macrophage-derived interleukin-6 is necessary and sufficient for choroidal angiogenesis. Sci. Rep. 11, 18084. doi:10.1038/s41598-021-97522-x
Dugel, P. U., Koh, A., Ogura, Y., Jaffe, G. J., Schmidt-Erfurth, U., Brown, D. M., et al. (2020). HAWK and HARRIER: Phase 3, multicenter, randomized, double-masked trials of brolucizumab for neovascular age-related macular degeneration. Ophthalmology 127, 72–84. doi:10.1016/j.ophtha.2019.04.017
Dugel, P. U., Singh, R. P., Koh, A., Ogura, Y., Weissgerber, G., Gedif, K., et al. (2021). HAWK and HARRIER: Ninety-Six-Week outcomes from the phase 3 trials of brolucizumab for neovascular age-related macular degeneration. Ophthalmology 128, 89–99. doi:10.1016/j.ophtha.2020.06.028
Edwards, A. O., Ritter, R., Abel, K. J., Manning, A., Panhuysen, C., and Farrer, L. A. (2005). Complement factor H polymorphism and age-related macular degeneration. Science 308, 421–424. doi:10.1126/science.1110189
Ferrara, N., Damico, L., Shams, N., Lowman, H., and Kim, R. (2006). Development of ranibizumab, an anti-vascular endothelial growth factor antigen binding fragment, as therapy for neovascular age-related macular degeneration. Retina 26, 859–870. doi:10.1097/01.iae.0000242842.14624.e7
Ferrara, N., Gerber, H. P., and Lecouter, J. (2003). The biology of VEGF and its receptors. Nat. Med. 9, 669–676. doi:10.1038/nm0603-669
Ferro Desideri, L., Traverso, C. E., and Nicolo, M. (2020). Abicipar pegol: An investigational anti-VEGF agent for the treatment of wet age-related macular degeneration. Expert Opin. Investig. Drugs 29, 651–658. doi:10.1080/13543784.2020.1772754
Finger, R. P., Wiedemann, P., Blumhagen, F., Pohl, K., and Holz, F. G. (2013). Treatment patterns, visual acuity and quality-of-life outcomes of the WAVE study - a noninterventional study of ranibizumab treatment for neovascular age-related macular degeneration in Germany. Acta Ophthalmol. 91, 540–546. doi:10.1111/j.1755-3768.2012.02493.x
Fleckenstein, M., Keenan, T. D., Guymer, R. H., Chakravarthy, U., Schmitz-Valckenberg, S., Klaver, C. C., et al. (2021). Age-related macular degeneration. Nat. Rev. Dis. Prim. 7, 31. doi:10.1038/s41572-021-00265-2
Fritsche, L. G., Igl, W., Bailey, J. N., Grassmann, F., Sengupta, S., Bragg-Gresham, J. L., et al. (2016). A large genome-wide association study of age-related macular degeneration highlights contributions of rare and common variants. Nat. Genet. 48, 134–143. doi:10.1038/ng.3448
Funes, S. C., Rios, M., Escobar-Vera, J., and Kalergis, A. M. (2018). Implications of macrophage polarization in autoimmunity. Immunology 154, 186–195. doi:10.1111/imm.12910
Gao, H., Chen, M., Liu, Y., Zhang, D., Shen, J., Ni, N., et al. (2023). Injectable anti-inflammatory supramolecular nanofiber hydrogel to promote anti-VEGF therapy in age-related macular degeneration treatment. Adv. Mater 35, 2204994. doi:10.1002/adma.202204994
Gao, X., and Talalay, P. (2004). Induction of phase 2 genes by sulforaphane protects retinal pigment epithelial cells against photooxidative damage. Proc. Natl. Acad. Sci. U. S. A. 101, 10446–10451. doi:10.1073/pnas.0403886101
Garber, K. (2015). RIKEN suspends first clinical trial involving induced pluripotent stem cells. Nat Biotechnol 33, 890–891.
Gragoudas, E. S., Adamis, A. P., Cunningham, E. T., Feinsod, M., Guyer, D. R., and Group, V. I. S. I. O. N. C. T. (2004). Pegaptanib for neovascular age-related macular degeneration. N. Engl. J. Med. 351, 2805–2816. doi:10.1056/nejmoa042760
Grossniklaus, H. E., Ling, J. X., Wallace, T. M., Dithmar, S., Lawson, D. H., Cohen, C., et al. (2002). Macrophage and retinal pigment epithelium expression of angiogenic cytokines in choroidal neovascularization. Mol. Vis. 8, 119–126.
Hackett, S. F., Ozaki, H., Strauss, R. W., Wahlin, K., Suri, C., Maisonpierre, P., et al. (2000). Angiopoietin 2 expression in the retina: Upregulation during physiologic and pathologic neovascularization. J. Cell Physiol. 184, 275–284. doi:10.1002/1097-4652(200009)184:3<275::aid-jcp1>3.0.co;2-7
Hageman, G. S., Anderson, D. H., Johnson, L. V., Hancox, L. S., Taiber, A. J., Hardisty, L. I., et al. (2005). A common haplotype in the complement regulatory gene factor H (HF1/CFH) predisposes individuals to age-related macular degeneration. Proc. Natl. Acad. Sci. U. S. A. 102, 7227–7232. doi:10.1073/pnas.0501536102
Han, X., Zhang, H., Butowska, K., Swingle, K. L., Alameh, M.-G., Weissman, D., et al. (2021). An ionizable lipid toolbox for RNA delivery. Nat. Commun. 12, 7233. doi:10.1038/s41467-021-27493-0
Handa, J. T., Bowes Rickman, C., Dick, A. D., Gorin, M. B., Miller, J. W., Toth, C. A., et al. (2019). A systems biology approach towards understanding and treating non-neovascular age-related macular degeneration. Nat. Commun. 10, 3347. doi:10.1038/s41467-019-11262-1
Harlos, K., Martin, D. M., O'brien, D. P., Jones, E. Y., Stuart, D. I., Polikarpov, I., et al. (1994). Crystal structure of the extracellular region of human tissue factor. Nature 370, 662–666. doi:10.1038/370662a0
Heier, J. S., Brown, D. M., Chong, V., Korobelnik, J. F., Kaiser, P. K., Nguyen, Q. D., et al. (2012). Intravitreal aflibercept (VEGF trap-eye) in wet age-related macular degeneration. Ophthalmology 119, 2537–2548. doi:10.1016/j.ophtha.2012.09.006
Heier, J. S., Khanani, A. M., Ruiz, C. Q., Basu, K., Ferrone, P. J., Brittain, C., et al. (2022). Efficacy, durability, and safety of intravitreal faricimab up to every 16 weeks for neovascular age-related macular degeneration (TENAYA and LUCERNE): Two randomised, double-masked, phase 3, non-inferiority trials. Lancet 399, 729–740. doi:10.1016/s0140-6736(22)00010-1
Heier, J. S., Kherani, S., Desai, S., Dugel, P., Kaushal, S., Cheng, S. H., et al. (2017). Intravitreous injection of AAV2-sFLT01 in patients with advanced neovascular age-related macular degeneration: A phase 1, open-label trial. Lancet 390, 50–61. doi:10.1016/s0140-6736(17)30979-0
Heier, J. S., Singh, R. P., Wykoff, C. C., Csaky, K. G., Lai, T. Y. Y., Loewenstein, A., et al. (2021). The angiopoietin/tie pathway in retinal vascular diseases: A review. Retina 41, 1–19. doi:10.1097/iae.0000000000003003
Heier, J. S., Wykoff, C. C., Waheed, N. K., Kitchens, J. W., Patel, S. S., Vitti, R., et al. (2020). Intravitreal combined aflibercept + anti-platelet-derived growth factor receptor beta for neovascular age-related macular degeneration: Results of the phase 2 CAPELLA trial. Ophthalmology 127, 211–220. doi:10.1016/j.ophtha.2019.09.021
Holash, J., Davis, S., Papadopoulos, N., Croll, S. D., Ho, L., Russell, M., et al. (2002). VEGF-trap: A VEGF blocker with potent antitumor effects. Proc. Natl. Acad. Sci. U. S. A. 99, 11393–11398. doi:10.1073/pnas.172398299
Holz, F. G., Dugel, P. U., Weissgerber, G., Hamilton, R., Silva, R., Bandello, F., et al. (2016). Single-chain antibody fragment vegf inhibitor RTH258 for neovascular age-related macular degeneration: A randomized controlled study. Ophthalmology 123, 1080–1089. doi:10.1016/j.ophtha.2015.12.030
Hu, Z., Cheng, J., Xu, J., Ruf, W., and Lockwood, C. J. (2017). Tissue factor is an angiogenic-specific receptor for factor VII-targeted immunotherapy and photodynamic therapy. Angiogenesis 20, 85–96. doi:10.1007/s10456-016-9530-9
Huang, L., Zhang, H., Cheng, C.-Y., Wen, F., Tam, P. O., Zhao, P., et al. (2016). A missense variant in FGD6 confers increased risk of polypoidal choroidal vasculopathy. Nat. Genet. 48, 640–647. doi:10.1038/ng.3546
Ilochonwu, B. C., Urtti, A., Hennink, W. E., and Vermonden, T. (2020). Intravitreal hydrogels for sustained release of therapeutic proteins. J. Control Release 326, 419–441. doi:10.1016/j.jconrel.2020.07.031
Iyer, S., Radwan, A. E., Hafezi-Moghadam, A., Malyala, P., and Amiji, M. (2019). Long-acting intraocular Delivery strategies for biological therapy of age-related macular degeneration. J. Control Release 296, 140–149. doi:10.1016/j.jconrel.2019.01.007
Jackson, T. L., Boyer, D., Brown, D. M., Chaudhry, N., Elman, M., Liang, C., et al. (2017). Oral tyrosine kinase inhibitor for neovascular age-related macular degeneration: A phase 1 dose-escalation study. Jama Ophthalmol. 135, 761–767. doi:10.1001/jamaophthalmol.2017.1571
Jackson, T. L., Slakter, J., Buyse, M., Wang, K., Dugel, P. U., Wykoff, C. C., et al. (2023). A randomized controlled trial of OPT-302, a VEGF-C/D inhibitor for neovascular age-related macular degeneration. Ophthalmology S0161-6420, 00066. doi:10.1016/j.ophtha.2023.02.001
Jaffe, G. J., Ciulla, T. A., Ciardella, A. P., Devin, F., Dugel, P. U., Eandi, C. M., et al. (2017). Dual antagonism of pdgf and vegf in neovascular age-related macular degeneration: A phase IIb, multicenter, randomized controlled trial. Ophthalmology 124, 224–234. doi:10.1016/j.ophtha.2016.10.010
Jaffe, G. J., Eliott, D., Wells, J. A., Prenner, J. L., Papp, A., and Patel, S. (2016). A phase 1 study of intravitreous E10030 in combination with ranibizumab in neovascular age-related macular degeneration. Ophthalmology 123, 78–85. doi:10.1016/j.ophtha.2015.09.004
Jiang, J., Wang, L., Kou, X. Y., Liu, Z. H., Huang, M., Li, H. Z., et al. (2020). In vivo characterization of RC28-E, a fusion protein targeting VEGF and bFGF: Pharmacokinetics and ocular distribution in primates. Exp. Eye Res. 190, 107823. doi:10.1016/j.exer.2019.107823
Jiang, J., Xu, K., Wang, L., Xin, W., Zhao, G., Huang, M., et al. (2018). Pharmacology study of a chimeric decoy receptor trap fusion protein on retina neovascularization by dual blockage of VEGF and FGF-2. Eur. J. Pharm. Sci. 121, 251–259. doi:10.1016/j.ejps.2018.04.043
Jo, N., Mailhos, C., Ju, M., Cheung, E., Bradley, J., Nishijima, K., et al. (2006). Inhibition of platelet-derived growth factor B signaling enhances the efficacy of anti-vascular endothelial growth factor therapy in multiple models of ocular neovascularization. Am. J. Pathol. 168, 2036–2053. doi:10.2353/ajpath.2006.050588
Johnson, P. T., Betts, K. E., Radeke, M. J., Hageman, G. S., Anderson, D. H., and Johnson, L. V. (2006). Individuals homozygous for the age-related macular degeneration risk-conferring variant of complement factor H have elevated levels of CRP in the choroid. Proc. Natl. Acad. Sci. U. S. A. 103, 17456–17461. doi:10.1073/pnas.0606234103
Joukov, V., Pajusola, K., Kaipainen, A., Chilov, D., Lahtinen, I., Kukk, E., et al. (1996). A novel vascular endothelial growth factor, VEGF-C, is a ligand for the Flt4 (VEGFR-3) and KDR (VEGFR-2) receptor tyrosine kinases. EMBO J. 15, 1751–2298. doi:10.1002/j.1460-2075.1996.tb00521.x
Joussen, A. M., Wolf, S., Kaiser, P. K., Boyer, D., Schmelter, T., Sandbrink, R., et al. (2019). The developing regorafenib eye drops for neovascular age-related macular degeneration (DREAM) study: An open-label phase II trial. Br. J. Clin. Pharmacol. 85, 347–355. doi:10.1111/bcp.13794
Kabasawa, S., Mori, K., Horie-Inoue, K., Gehlbach, P. L., Inoue, S., Awata, T., et al. (2011). Associations of cigarette smoking but not serum fatty acids with age-related macular degeneration in a Japanese population. Ophthalmology 118, 1082–1088. doi:10.1016/j.ophtha.2010.10.012
Kamao, H., Mandai, M., Okamoto, S., Sakai, N., Suga, A., Sugita, S., et al. (2014). Characterization of human induced pluripotent stem cell-derived retinal pigment epithelium cell sheets aiming for clinical application. Stem Cell Rep. 2, 205–218. doi:10.1016/j.stemcr.2013.12.007
Kato, Y., Oguchi, Y., Omori, T., Shintake, H., Tomita, R., Kasai, A., et al. (2020). Complement activation products and cytokines in pachychoroid neovasculopathy and neovascular age-related macular degeneration. Invest. Ophthalmol. Vis. Sci. 61, 39. doi:10.1167/iovs.61.13.39
Khanani, A. M., Callanan, D., Dreyer, R., Chen, S., Howard, J. G., Hopkins, J. J., et al. (2021). End-of-study results for the ladder phase 2 trial of the port delivery system with ranibizumab for neovascular age-related macular degeneration. Ophthalmol. Retina 5, 775–787. doi:10.1016/j.oret.2020.11.004
Khanani, A. M., Patel, S. S., and Ferrone, P. J. (2020). Efficacy of every four monthly and quarterly dosing of faricimab vs ranibizumab in neovascular age-related macular degeneration: The STAIRWAY phase 2 randomized clinical trial (jul, 10.1001/jamaophthalmol.2020.2699, 2020). Jama Ophthalmol. 138, 1006.
Khanani, A. M., Thomas, M. J., Aziz, A. A., Weng, C. Y., Danzig, C. J., Yiu, G., et al. (2022). Review of gene therapies for age-related macular degeneration. Eye (Lond) 36, 303–311. doi:10.1038/s41433-021-01842-1
Kim, J., Kim, C. S., Jo, K., Cho, Y. S., Kim, H. G., Lee, G. H., et al. (2015). HL-217, a new topical anti-angiogenic agent, inhibits retinal vascular leakage and pathogenic subretinal neovascularization in Vldlr(-)/(-) mice. Biochem. Biophys. Res. Commun. 456, 53–58. doi:10.1016/j.bbrc.2014.11.033
Koo, T., Park, S. W., Jo, D. H., Kim, D., Kim, J. H., Cho, H.-Y., et al. (2018). CRISPR-LbCpf1 prevents choroidal neovascularization in a mouse model of age-related macular degeneration. Nat. Commun. 9, 1855. doi:10.1038/s41467-018-04175-y
Kulkarni, A. D., and Kuppermann, B. D. (2005). Wet age-related macular degeneration. Adv. Drug Deliv. Rev. 57, 1994–2009. doi:10.1016/j.addr.2005.09.003
Kunimoto, D., Yoon, Y. H., Wykoff, C. C., Chang, A., Khurana, R. N., Maturi, R. K., et al. (2020). Efficacy and safety of abicipar in neovascular age-related macular degeneration: 52-Week results of phase 3 randomized controlled study. Ophthalmology 127, 1331–1344. doi:10.1016/j.ophtha.2020.03.035
Lambert, N. G., Elshelmani, H., Singh, M. K., Mansergh, F. C., Wride, M. A., Padilla, M., et al. (2016). Risk factors and biomarkers of age-related macular degeneration. Prog. Retin Eye Res. 54, 64–102. doi:10.1016/j.preteyeres.2016.04.003
Li, B., Yuan, Z., Jain, P., Hung, H.-C., He, Y., Lin, X., et al. (2020). De novo design of functional zwitterionic biomimetic material for immunomodulation. Sci. Adv. 6, eaba0754. doi:10.1126/sciadv.aba0754
Lin, Q., Lim, J. Y., Xue, K., Su, X., and Loh, X. J. (2021). Polymeric hydrogels as a vitreous replacement strategy in the eye. Biomaterials 268, 120547. doi:10.1016/j.biomaterials.2020.120547
Ling, S., Yang, S., Hu, X., Yin, D., Dai, Y., Qian, X., et al. (2021). Lentiviral delivery of co-packaged Cas9 mRNA and a Vegfa-targeting guide RNA prevents wet age-related macular degeneration in mice. Nat. Biomed. Eng. 5, 144–156. doi:10.1038/s41551-020-00656-y
Lu, X., Miao, L., Gao, W., Chen, Z., Mchugh, K. J., Sun, Y., et al. (2020). Engineered PLGA microparticles for long-term, pulsatile release of STING agonist for cancer immunotherapy. Sci. Transl. Med. 12, eaaz6606. doi:10.1126/scitranslmed.aaz6606
Lutty, G. A., and Mcleod, D. S. (2018). Development of the hyaloid, choroidal and retinal vasculatures in the fetal human eye. Prog. Retin Eye Res. 62, 58–76. doi:10.1016/j.preteyeres.2017.10.001
Maisonpierre, P. C., Suri, C., Jones, P. F., Bartunkova, S., Wiegand, S., Radziejewski, C., et al. (1997). Angiopoietin-2, a natural antagonist for Tie2 that disrupts in vivo angiogenesis. Science 277, 55–60. doi:10.1126/science.277.5322.55
Mandai, M., Fujii, M., Hashiguchi, T., Sunagawa, G. A., Ito, S. I., Sun, J., et al. (2017a). iPSC-derived retina transplants improve vision in rd1 end-stage retinal-degeneration mice. Stem Cell Rep. 8, 489–583. doi:10.1016/j.stemcr.2017.01.018
Mandai, M., Watanabe, A., Kurimoto, Y., Hirami, Y., Morinaga, C., Daimon, T., et al. (2017b). Autologous induced stem-cell-derived retinal cells for macular degeneration. N. Engl. J. Med. 376, 1038–1046. doi:10.1056/nejmoa1608368
Matsuda, Y., Nonaka, Y., Futakawa, S., Imai, H., Akita, K., Nishihata, T., et al. (2019). Anti-angiogenic and anti-scarring dual action of an anti-fibroblast growth factor 2 aptamer in animal models of retinal disease. Mol. Ther. Nucleic Acids 17, 819–828. doi:10.1016/j.omtn.2019.07.018
Mchugh, K. J., Nguyen, T. D., Linehan, A. R., Yang, D., Behrens, A. M., Rose, S., et al. (2017). Fabrication of fillable microparticles and other complex 3D microstructures. Science 357, 1138–1142. doi:10.1126/science.aaf7447
Mcleod, D. S., Grebe, R., Bhutto, I., Merges, C., Baba, T., and Lutty, G. A. (2009). Relationship between RPE and choriocapillaris in age-related macular degeneration. Investig. Ophthalmol. Vis. Sci. 50, 4982–4991. doi:10.1167/iovs.09-3639
Mehta, H., Tufail, A., Daien, V., Lee, A. Y., Nguyen, V., Ozturk, M., et al. (2018). Real-world outcomes in patients with neovascular age-related macular degeneration treated with intravitreal vascular endothelial growth factor inhibitors. Prog. Retin Eye Res. 65, 127–146. doi:10.1016/j.preteyeres.2017.12.002
Mettu, P. S., Allingham, M. J., and Cousins, S. W. (2021). Incomplete response to Anti-VEGF therapy in neovascular AMD: Exploring disease mechanisms and therapeutic opportunities. Prog. Retin Eye Res. 82, 100906. doi:10.1016/j.preteyeres.2020.100906
Michels, S., Rosenfeld, P. J., Puliafito, C. A., Marcus, E. N., and Venkatraman, A. S. (2005). Systemic bevacizumab (Avastin) therapy for neovascular age-related macular degeneration twelve-week results of an uncontrolled open-label clinical study. Ophthalmology 112, 1035–1047.e9. doi:10.1016/j.ophtha.2005.02.007
Mitchell, M. J., Billingsley, M. M., Haley, R. M., Wechsler, M. E., Peppas, N. A., and Langer, R. (2021). Engineering precision nanoparticles for drug delivery. Nat. Rev. Drug Discov. 20, 101–124. doi:10.1038/s41573-020-0090-8
Mitchell, P., Korobelnik, J. F., Lanzetta, P., Holz, F. G., Prunte, C., Schmidt-Erfurth, U., et al. (2010). Ranibizumab (lucentis) in neovascular age-related macular degeneration: Evidence from clinical trials. Br. J. Ophthalmol. 94, 2–13. doi:10.1136/bjo.2009.159160
Mitchell, P., Liew, G., Gopinath, B., and Wong, T. Y. (2018). Age-related macular degeneration. Lancet 392, 1147–1159. doi:10.1016/s0140-6736(18)31550-2
Mullins, R. E., Johnson, M. N., Faidley, E. A., Skeie, J. M., and Huang, J. A. (2011). Choriocapillaris vascular dropout related to density of drusen in human eyes with early age-related macular degeneration. Investig. Ophthalmol. Vis. Sci. 52, 1606–1612. doi:10.1167/iovs.10-6476
Myers, C. E., Klein, B. E., Gangnon, R., Sivakumaran, T. A., Iyengar, S. K., and Klein, R. (2014). Cigarette smoking and the natural history of age-related macular degeneration: The beaver dam eye study. Ophthalmology 121, 1949–1955. doi:10.1016/j.ophtha.2014.04.040
Nakamura, Y. (2021). Multiple therapeutic applications of RBM-007, an anti-FGF2 aptamer. Cells 10, 1671. doi:10.3390/cells10071617
Nazila, K., Basit, Y., Jun, W., and Omid, C. F. (2016). Degradable controlled-release polymers and polymeric nanoparticles: Mechanisms of controlling drug release. Chem. Rev. 116, 2602–2663. doi:10.1021/acs.chemrev.5b00346
Nguyen, Q. D., Das, A., Do, D. V., Dugel, P. U., Gomes, A., Holz, F. G., et al. (2020). Brolucizumab: Evolution through preclinical and clinical studies and the implications for the management of neovascular age-related macular degeneration. Ophthalmology 127, 963–976. doi:10.1016/j.ophtha.2019.12.031
Nugent, M. A., and Iozzo, R. V. (2000). Fibroblast growth factor-2. Int. J. Biochem. Cell B 32, 115–120. doi:10.1016/s1357-2725(99)00123-5
Nussenblatt, R. B., Liu, B., Wei, L., and Sen, H. N. (2013). The immunological basis of degenerative diseases of the eye. Int. Rev. Immunol. 32, 97–112. doi:10.3109/08830185.2012.740536
Park, Y. G., Park, Y. S., and Kim, I. B. (2021). Complement system and potential therapeutics in age-related macular degeneration. Int. J. Mol. Sci. 22, 6851. doi:10.3390/ijms22136851
Pennington, K. L., and Deangelis, M. M. (2016). Epidemiology of age-related macular degeneration (AMD): Associations with cardiovascular disease phenotypes and lipid factors. Eye Vis. (Lond) 3, 34. doi:10.1186/s40662-016-0063-5
Pescina, S., Ostacolo, C., Gomez-Monterrey, I. M., Sala, M., Bertamino, A., Sonvico, F., et al. (2018). Cell penetrating peptides in ocular drug delivery: State of the art. J. Control Release 284, 84–102. doi:10.1016/j.jconrel.2018.06.023
Poor, S. H., Weissgerber, G., Adams, C. M., Bhatt, H., Browning, D. J., Chastain, J., et al. (2022). A randomized, double-masked, multicenter trial of topical acrizanib (LHA510), a tyrosine kinase VEGF-receptor inhibitor, in treatment-experienced subjects with neovascular age-related macular degeneration. Am. J. Ophthalmol. 239, 180–189. doi:10.1016/j.ajo.2022.02.019
Ramrattan, R. S., Van Der Schaft, T. L., Mooy, C. M., De Bruijn, W. C., Mulder, P. G., and De Jong, P. T. (1994). Morphometric analysis of Bruch's membrane, the choriocapillaris, and the choroid in aging. Invest. Ophthalmol. Vis. Sci. 35, 2857–2864.
Rao, P., Lum, F., Wood, K., Salman, C., Burugapalli, B., Hall, R., et al. (2018). Real-world vision in age-related macular degeneration patients treated with single anti-VEGF drug type for 1 Year in the IRIS registry. Ophthalmology 125, 522–528. doi:10.1016/j.ophtha.2017.10.010
Regula, J. T., Von Leithner, P. L., Foxton, R., Barathi, V. A., Ming, G. C. C., Tun, S. B. B., et al. (2019). Targeting key angiogenic pathways with a bispecific Cross MA b optimized for neovascular eye diseases. Embo Mol. Med. 11, e10666. doi:10.15252/emmm.201910666
Ren, X. Y., Li, J., Xu, X. X., Wang, C. M., and Cheng, Y. G. (2016). IBI302, a promising candidate for AMD treatment, targeting both the VEGF and complement system with high binding affinity in vitro and effective targeting of the ocular tissue in healthy rhesus monkeys. Exp. Eye Res. 145, 352–358. doi:10.1016/j.exer.2016.02.004
Rodrigues, G. A., Mason, M., Christie, L. A., Hansen, C., Hernandez, L. M., Burke, J., et al. (2018). Functional characterization of abicipar-pegol, an anti-VEGF DARPin therapeutic that potently inhibits angiogenesis and vascular permeability. Invest. Ophthalmol. Vis. Sci. 59, 5836–5846. doi:10.1167/iovs.18-25307
Rosenfeld, P. J., Brown, D. M., Heier, J. S., Boyer, D. S., Kaiser, P. K., Chung, C. Y., et al. (2006). Ranibizumab for neovascular age-related macular degeneration. N. Engl. J. Med. 355, 1419–1431. doi:10.1056/nejmoa054481
Rosenfeld, P. J., and Feuer, W. J. (2018). Lessons from recent phase III trial failures: Don't design phase III trials based on retrospective subgroup analyses from phase II trials. Ophthalmology 125, 1488–1491. doi:10.1016/j.ophtha.2018.06.002
Saharinen, P., Eklund, L., Miettinen, J., Wirkkala, R., Anisimov, A., Winderlich, M., et al. (2008). Angiopoietins assemble distinct Tie2 signalling complexes in endothelial cell-cell and cell-matrix contacts. Nat. Cell Biol. 10, 527–537. doi:10.1038/ncb1715
Schnichels, S., Paquet-Durand, F., Löscher, M., Tsai, T., Hurst, J., Joachim, S. C., et al. (2021). Retina in a dish: Cell cultures, retinal explants and animal models for common diseases of the retina. Prog. Retin Eye Res. 81, 100880. doi:10.1016/j.preteyeres.2020.100880
Seddon, J. M., Cote, J., Page, W. F., Aggen, S. H., and Neale, M. C. (2005). The US twin study of age-related macular degeneration: Relative roles of genetic and environmental influences. Arch. Ophthalmol. 123, 321–327. doi:10.1001/archopht.123.3.321
Seddon, J. M. (2017). Macular degeneration epidemiology: Nature-nurture, lifestyle factors, genetic risk, and gene-environment interactions - the weisenfeld award lecture. Investig. Ophthalmol. Vis. Sci. 58, 6513–6528. doi:10.1167/iovs.17-23544
Sharma, A., Kumar, N., Kuppermann, B. D., Bandello, F., and Loewenstein, A. (2020). Faricimab: Expanding horizon beyond VEGF. Eye (Lond) 34, 802–804. doi:10.1038/s41433-019-0670-1
Sharma, R., Khristov, V., Rising, A., Jha, B. S., Dejene, R., Hotaling, N., et al. (2019). Clinical-grade stem cell–derived retinal pigment epithelium patch rescues retinal degeneration in rodents and pigs. Sci. Transl. Med. 11, eaat5580. eaat5580. doi:10.1126/scitranslmed.aat5580
Shen, J., Kim, J., Tzeng, S. Y., Ding, K., Hafiz, Z., Long, D., et al. (2020). Suprachoroidal gene transfer with nonviral nanoparticles. Sci. Adv. 6, eaba1606. doi:10.1126/sciadv.aba1606
Simons, M., Gordon, E., and Claesson-Welsh, L. (2016). Mechanisms and regulation of endothelial VEGF receptor signalling. Nat. Rev. Mol. Cell Biol. 17, 611–625. doi:10.1038/nrm.2016.87
Skeie, J. M., Fingert, J. H., Russell, S. R., Stone, E. M., and Mullins, R. F. (2010). Complement component C5a activates ICAM-1 expression on human choroidal endothelial cells. Invest. Ophthalmol. Vis. Sci. 51, 5336–5342. doi:10.1167/iovs.10-5322
Smith, A. F. (2010). The growing importance of pharmacoeconomics: The case of age-related macular degeneration. Br. J. Ophthalmol. 94, 1116–1117. doi:10.1136/bjo.2010.179945
Spaide, R. F., Jaffe, G. J., Sarraf, D., Freund, K. B., Sadda, S. R., Staurenghi, G., et al. (2020). Consensus nomenclature for reporting neovascular age-related macular degeneration data: Consensus on neovascular age-related macular degeneration nomenclature study group. Ophthalmology 127, 616–636. doi:10.1016/j.ophtha.2019.11.004
Sparrow, J. R., and Boulton, M. (2005). RPE lipofuscin and its role in retinal pathobiology. Exp. Eye Res. 80, 595–606. doi:10.1016/j.exer.2005.01.007
Spraul, C. W., and Grossniklaus, H. E. (1997). Characteristics of Drusen and Bruch's membrane in postmortem eyes with age-related macular degeneration. Arch. Ophthalmol. 115, 267–273. doi:10.1001/archopht.1997.01100150269022
Stewart, M. W., Garg, S., Newman, E. M., Jeffords, E., Konopinska, J., Jackson, S., et al. (2022). Safety and therapeutic effects of orally administered Akst4290 in newly diagnosed neovascular age-related macular degeneration. Retina 42, 1038–1046. doi:10.1097/iae.0000000000003446
Sugita, S., Mandai, M., Kamao, H., and Takahashi, M. (2021). Immunological aspects of RPE cell transplantation. Prog. Retin Eye Res. 84, 100950. doi:10.1016/j.preteyeres.2021.100950
Takahashi, K., Saishin, Y., Saishin, Y., King, A. G., Levin, R., and Campochiaro, P. A. (2009). Suppression and regression of choroidal neovascularization by the multitargeted kinase inhibitor pazopanib. Arch. Ophthalmol. 127, 494–499. doi:10.1001/archophthalmol.2009.27
Takeda, A., Baffi, J. Z., Kleinman, M. E., Cho, W. G., Nozaki, M., Yamada, K., et al. (2009). CCR3 is a target for age-related macular degeneration diagnosis and therapy. Nature. 460, 225–230.
Tan, J. S. L., Wang, J. J., Flood, V., Rochtchina, E., Smith, W., and Mitchell, P. (2008). Dietary antioxidants and the long-term incidence of age-related macular degeneration. Ophthalmology 115, 334–341. doi:10.1016/j.ophtha.2007.03.083
Tezel, T. H., Bodek, E., Sonmez, K., Kaliappan, S., Kaplan, H. J., Hu, Z., et al. (2007). Targeting tissue factor for immunotherapy of choroidal neovascularization by intravitreal delivery of factor VII-Fc chimeric antibody. Ocul. Immunol. Inflamm. 15, 3–10. doi:10.1080/09273940601147760
Thomas, M., and Augustin, H. G. (2009). The role of the Angiopoietins in vascular morphogenesis. Angiogenesis 12, 125–137. doi:10.1007/s10456-009-9147-3
Tong, Y., Zhang, Z., and Wang, S. (2022). Role of mitochondria in retinal pigment epithelial aging and degeneration. Front. Aging 3, 926627. doi:10.3389/fragi.2022.926627
Toomey, C. B., Johnson, L. V., and Bowes Rickman, C. (2018). Complement factor H in AMD: Bridging genetic associations and pathobiology. Prog. Retin Eye Res. 62, 38–57. doi:10.1016/j.preteyeres.2017.09.001
Tsujinaka, H., Fu, J., Shen, J., Yu, Y., Hafiz, Z., Kays, J., et al. (2020). Sustained treatment of retinal vascular diseases with self-aggregating sunitinib microparticles. Nat. Commun. 11, 694. doi:10.1038/s41467-020-14340-x
Uemura, A., Fruttiger, M., D'amore, P. A., De Falco, S., Joussen, A. M., Sennlaub, F., et al. (2021). VEGFR1 signaling in retinal angiogenesis and microinflammation. Prog. Retin Eye Res. 84, 100954. doi:10.1016/j.preteyeres.2021.100954
Van Asten, F., Simmons, M., Singhal, A., Keenan, T. D., Ratnapriya, R., Agrón, E., et al. (2018). A deep phenotype association study reveals specific phenotype associations with genetic variants in age-related macular degeneration: Age-Related Eye Disease Study 2 (AREDS2) report no. 14. Ophthalmology 125, 559–568. doi:10.1016/j.ophtha.2017.09.023
Wang, J., Feng, Y., Han, P., Wang, F., Luo, X., Liang, J., et al. (2018). Photosensitization of A2E triggers telomere dysfunction and accelerates retinal pigment epithelium senescence. Cell Death Dis. 9, 178. doi:10.1038/s41419-017-0200-7
Wang, K., and Han, Z. (2017). Injectable hydrogels for ophthalmic applications. J. Control Release 268, 212–224. doi:10.1016/j.jconrel.2017.10.031
Wang, L., Yang, Z., Yu, Y., Cui, C., Guan, H., and Chen, H. (2014). Blockage of tissue factor ameliorates the lesion of laser-induced choroidal neovascularization in mice. Exp. Eye Res. 127, 117–123. doi:10.1016/j.exer.2014.07.006
Waters, S. B., Zhou, C., Nguyen, T., Zelkha, R., Lee, H., Kazlauskas, A., et al. (2021). VEGFR2 trafficking by KIF13B is a novel therapeutic target for wet age-related macular degeneration. Invest. Ophthalmol. Vis. Sci. 62, 5. doi:10.1167/iovs.62.2.5
Wells, J. A., Gonzales, C. R., Berger, B. B., Gonzalez, V. H., Sippy, B. D., and Burian, G. (2018). A phase 1, open-label, dose-escalation trial to investigate safety and tolerability of single intravitreous injections of ICON-1 targeting tissue factor in wet AMD. Ophthalmic Surg. Lasers Imaging Retina 49, 336–345. doi:10.3928/23258160-20180501-07
Wong, W. L., Su, X., Li, X., Cheung, C. M. G., Klein, R., Cheng, C.-Y., et al. (2014). Global prevalence of age-related macular degeneration and disease burden projection for 2020 and 2040: A systematic review and meta-analysis. Lancet Glob. Health 2, e106–e116. doi:10.1016/s2214-109x(13)70145-1
Wooff, Y., Man, S. M., Aggio-Bruce, R., Natoli, R., and Fernando, N. (2019). IL-1 family members mediate cell death, inflammation and angiogenesis in retinal degenerative diseases. Front. Immunol. 10, 1618. doi:10.3389/fimmu.2019.01618
Yang, Q., Jiang, H., Wang, Y., Leng, X., Wang, Y., Tong, J., et al. (2023). Plaque macrophage-targeting nanosystems with cooperative Co-regulation of ROS and TRAF6 for stabilization of atherosclerotic plaques. Adv. Funct. Mater. 2023, 2301053. doi:10.1002/adfm.202301053
Yang, Q., Peng, J., Xiao, H., Xu, X., and Qian, Z. (2022a). Polysaccharide hydrogels: Functionalization, construction and served as scaffold for tissue engineering. Carbohydr. Polym. 278, 118952. doi:10.1016/j.carbpol.2021.118952
Yang, S., Li, T., Jia, H., Gao, M., Li, Y., Wan, X., et al. (2022b). Targeting C3b/C4b and VEGF with a bispecific fusion protein optimized for neovascular age-related macular degeneration therapy. Sci. Transl. Med. 14, eabj2177. doi:10.1126/scitranslmed.abj2177
Zeitz, O., and Joussen, A. M. (2017). Eye drops instead of intravitreal injections? The dream of treating macular diseases by topically administered drugs. Klin. Monbl Augenheilkd 234, 1088–1093. doi:10.1055/s-0043-113253
Keywords: age-related macular degeneration (AMD), drug delivery system, long-term delivery, pathogenesis, intravitreal delivery
Citation: Huang X, Zhang L, Fu Y, Zhang M, Yang Q and Peng J (2023) Rethinking the potential and necessity of drug delivery systems in neovascular age-related macular degeneration therapy. Front. Bioeng. Biotechnol. 11:1199922. doi: 10.3389/fbioe.2023.1199922
Received: 04 April 2023; Accepted: 09 May 2023;
Published: 23 May 2023.
Edited by:
Jianxun Ding, Chinese Academy of Sciences (CAS), ChinaReviewed by:
Michael B. Powner, City University of London, United KingdomShuai Shi, Wenzhou Medical University, China
Copyright © 2023 Huang, Zhang, Fu, Zhang, Yang and Peng. This is an open-access article distributed under the terms of the Creative Commons Attribution License (CC BY). The use, distribution or reproduction in other forums is permitted, provided the original author(s) and the copyright owner(s) are credited and that the original publication in this journal is cited, in accordance with accepted academic practice. No use, distribution or reproduction is permitted which does not comply with these terms.
*Correspondence: Qian Yang, eW95b3lvdW5nQGNtYy5lZHUuY24=; Jinrong Peng, amlucm9uZ3BlbmdAc2N1LmVkdS5jbg==