- New Energy Research Institute, Jining University, Qufu, Shandong, China
Digestates from different anaerobic digesters are promising substrates for microalgal culture, leading to effective wastewater treatment and the production of microalgal biomass. However, further detailed research is needed before they can be used on a large scale. The aims of this study were to investigate the culture of Chlorella sp. in DigestateM from anaerobic fermentation of brewer’s grains and brewery wastewater (BWW) and to explore the potential use of the biomass produced under different experimental conditions, including diverse cultivation modes and dilution ratios. Cultivation in DigestateM initiated from 10% (v/v) loading, with 20% BWW, obtained maximum biomass production, reaching 1.36 g L−1 that was 0.27g L−1 higher than 1.09 g L−1 of BG11. In terms of DigestateM remediation, the maximum removal of ammonia nitrogen (NH4+-N), chemical oxygen demand, total nitrogen, and total phosphorus reached 98.20%, 89.98%, 86.98%, and 71.86%, respectively. The maximum lipid, carbohydrate, and protein contents were 41.60%, 32.44%, and 27.72%, respectively. The growth of Chlorella sp. may be inhibited when the Y(II)–Fv/Fm ratio is less than 0.4.
1 Introduction
In recent years, the fast development of human society has led to the rapid consumption of various nonrenewable resources and people’s demand for sustainable new sources of food and energy. The versatility of microalgal biomass is exactly what people need now given its ability to assimilate carbon dioxide through photosynthesis and high areal productivity on nonarable land (Cheng et al., 2016). The cultivation of microalgae is often coupled with the treatment of wastewater to obtain microalgal biomass and to treat the wastewater (Zhang et al., 2016), which can solve the problem of water shortage and environmental pollution (Shahid et al., 2019). The direct discharge of anaerobic digestive effluents, owing to their high nutrient value, not only causes environmental pollution but also wastes various nutrient elements in the digestive effluents; in recent years, extensive investigations have been conducted to find the digestive effluents suitable for the growth of microalgae (Koutra et al., 2018a; Alavijeh et al., 2020). It is a good strategy to treat digested effluents with microalgae (Chuka-ogwude et al., 2020). The high pH value, high turbidity and low C/N ratio of effluents will affect the growth of microalgae, and the inhibiting factors will also vary with the anaerobic fermentation substrate, which brings challenges to the large-scale treatment digestives by microalgae (Bonetta et al., 2014; Xia and Murphy, 2016). To solve this difficulty, researchers often carry out pretreatment of digested effluents, such as physical adsorption, flocculation, biological contact oxidation, and ozone treatment (Kim et al., 2014). Although these methods can reduce inhibitors for the survival of microalgae in digested effluents, the nutrients in effluents are greatly reduced, and the cost is high (Qian et al., 2019). Dilution of digested effluents is also a pretreatment method. Different dilution ratios, effluent types, and experimental conditions will change the production and biochemical components of microalgal biomass (Mayers et al., 2017; Romero-Villegas et al., 2018; Zhao et al., 2019). Therefore, applied conditions and the biological composition of microalgae obtained from different digested effluents play critical roles in subsequent applications (Eppink et al., 2017).
Chlorella sp. comprises various nutritional modes: autotrophy, photoheterotrophy, and heterotrophy (Zhu, 2015). This property makes Chlorella have the potential to utilize organic wastewater and adapt to harsh environments (Chew et al., 2018). Moreover, Chlorella biomass is rich in polysaccharides, proteins, lipids, and other organic matter, which have broad application prospects in food, energy, materials, and other fields (Safi et al., 2014; Sakarika and Kornaros, 2019), and it is one of the few microalgae that can be cultivated on a large scale (Stiles et al., 2018). About different pretreatment methods of digestate, Franco et al. (Tfab et al., 2020) used a Chlorella–bacteria combined system to treat waste food biogas slurry. When the concentration of effluents reached 100%, the removal rates for total organic carbon, total nitrogen (TN), and total phosphorus (TP) were still as high as 85.00% ± 3%, 73.00% ± 3%, and 28.00% ± 16%, respectively. Through the pretreatment of the digestate effluent with indigenous bacteria in the anaerobic digestion liquid of pig manure, the modification of waste liquid by Chlorella vulgaris was also enhanced, and the biomass yield of C. vulgaris was increased (Gu et al., 2021). Concerning the use of digestate, Dinnebier et al. (2021) used Chlorella sorokiniana to treat swine digestate, reaching the maximum biomass productivity of 198 mg L−1 d−1 (dry weight) and removal of 90.00% and 70.00% of TP and TN, respectively. The protein content of biomass was as high as 59.50%. With a three-step process for treating anaerobically digested swine manure, the maximum biomass concentration of C. vulgaris reached 2.33 g L−1, accompanied by the removal of 97.20%, 94.00%, 99.70%, and 99.90% of chemical oxygen demand (COD), TN, TP, and ammonia nitrogen (NH4+-N) (Wang et al., 2019). Recently, an integrated effective treatment process for digestate from agro-industrial and municipal organic wastes, combining different dilution ratios, different culture models, and nutrient supplement of digestate, resulted in the maximum biomass concentration of C. vulgaris up to 1.63 g L−1 and organic carbon, TN, and TP removal rates of 92.00%, 77.00%, and 94.00%, respectively (Koutra et al., 2021). Through the addition of 2 g L−1 of a glycerol solution to digested municipal wastewater as a supplementary carbon source, the growth rate and lipid production rate of C. vulgaris under mixotrophic conditions reached 0.064 g L−1 d−1 and 20.4 mg L−1 d−1, respectively (Ge et al., 2018). About the use of wastewater, using Chlorella sorokiniana CY-1 to treat waste molasses showed a reduction in COD, total suspended solid, and nitrate content of 61.48%, 63.94%, and 63.79%, respectively and 155.0 mg L−1 of molasses concentration medium obtained the highest biomass productivity of 160.9 mg L−1 d−1 (Guo et al., 2020). Moreover, Chlorella sp. and Chlorococcum sp. were effectively cultivated in industrial wastewater with varying concentrations of coal-fired flue gas from 1% to 10% CO2, resulting in the highest biomass production of 1.52 g L−1 and COD, ammonia nitrogen (NH4+-N), TP, and nitrate maximum removal rates of 91.9%, 95.9%, 98.8%, and 100%, respectively (Yadav et al., 2019). Comparing the effects of urban wastewater (UWW) and Bold’s basal medium (BBM) on microalgal growth, the lipid productivity (LP) in UWW was 1.15 fold that in BBM, and the largest fat yield in UWW was 14.31 mg L−1 d−1, which simultaneously had removal rates of up to 87.90% and 98.40% for TN and TP by C. vulgaris, respectively (Singh et al., 2017). Microalgae can grow under different culture modes, such as photoautotrophic cultivation widely used in microalgal cultivation, heterotrophic cultivation that mainly uses organic matter to grow (Chojnacka and Marquez-Rocha, 2004), and mixotrophic cultivation using organic matter and inorganic matter at the same time (Wang et al., 2014). Cultivation modes will affect the metabolism pathway and biomass composition of C. vulgaris (Changling et al., 2014).
China is a major producer of beer, with output reaching 35.62 million tons in 2021 despite the impact of the COVID-19. Each ton of beer produced will generate 3–10 tons of brewery wastewater (BWW) (Fillaudeau et al., 2006) and 0.25 tons of brewer’s grains (BWG) (Aliyu and Bala, 2011). There are different treatment and utilization methods for BWW and BWG. Lu et al. (2019) obtained photosynthetic bacteria biomass while treating BWW, and the maximum yield of the biomass could reach 483.5 mg L−1 d−1, realizing 420.9, 177.6, and 2.53 mg g−1 of protein, polysaccharide, and carotenoid in the photosynthetic bacteria biomass, respectively. Exploring the cultivation of Chlorella sp. in BWW, Wang et al. (2022) obtained not only the maximum biomass concentration of 2.03 g L−1 but also the maximum lipid, carbohydrate, and protein contents of 36.19%, 36.51%, and 19.77%, respectively, with the maximum removal of NH4+-N, COD, TN, and TP reaching 94.38%, 88.52%, 96.71%, and 98.68%, respectively. Previous studies have reported the production of methane (Chen et al., 2016) and hydrogen (Shi et al., 2010) by anaerobic fermentation of BWW, but the production of biogas by anaerobic fermentation of BWW–BWG mixtures has not been studied. The main objective of this study was to cultivate Chlorella sp. in effluents from anaerobic digestion of BWW–BWG mixtures and under various culture conditions. The potential of microalgal treatment of digestate was investigated, and the potential uses of microalgal biomass were explored. The application treatment methods included different culture models (mixotrophic and heterotrophic conditions) and dilution ratios of digestate. In the experimental process, microalgal biomass production and BWW–BWG mixture digestate (DigestateM) remediation were evaluated, accompanied by the investigation of photosynthetic fluorescence parameters and microalgal biomass components, including pigments, proteins, carbohydrates, and lipids.
2 Materials and methods
2.1 DigestateM collection and treatment
The digested effluents were produced by the process of single-phase anaerobic fermentation, which was carried out at medium temperature (42°C) on lab-scale. Mixtures of BWW and BWG were treated at HRT of 45 days.
After the above experiment, the fermentation mixture was filtered using a 100-mesh filter bag, and the digested effluents were collected and centrifuged (XL5A, XIANG LI) for 10 min at 4,000 rpm. A sand core filter, combined with a 0.45 μm filter membrane, was also used to filter the supernatant to remove the solid components.
Subsequently, the main physicochemical characteristics of DigestateM were analyzed, as detailed in Table 1. DigestateM was kept in a refrigerator at −18°C.
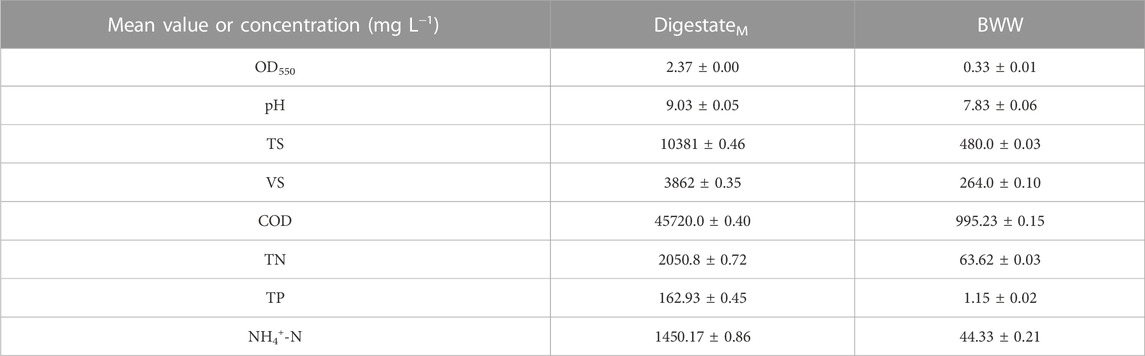
TABLE 1. Physicochemical properties of BWW–BWG mixture digestate (DigestateM) and brewery wastewater (BWW). Data are means ± SD (n = 2).
2.2 Chlorella sp. cultures and experimental conditions
Chlorella sp. was obtained from the Freshwater Algae Culture Collection at the Institute of Hydrobiology, Wuhan, China. First, the obtained Chlorella sp. was incubated in BG11 AGAR medium at 25°C for 7 days in a sterile procedure (SW-CJ-1F ISO-5, AIRTECH) to obtain single algal strains. A single algal strain was selected and placed in 200 mL triangular bottles with 50 mL of BG11 medium for static culture, maintaining the extended culture for 7 days. After the expanded culture, Chlorella sp. was cultured by passage, and the fourth generation was used as experimental inoculum for subsequent experiments. The experiment was conducted in an artificial greenhouse at 25°C, with a red-blue-yellow LED above the triangular bottle providing photosynthetic photon flux density of 225 μmol m−2 s−1 and aeration of 1.25 L min−1. Under different experimental conditions, the initial concentration of Chlorella sp. was 0.26 g L−1 (dry weight).
The absorbance of DigestateM at 550 nm was 2.37 ± 0.00 and based on previous results (Koutra et al., 2021), digestate was initially used at 10% (v/v) to research the growth of Chlorella sp. without adding nutrient source. In consideration of the large amount of fresh water used in dilution, to reduce the consumption of water resources, part of fresh water was replaced with BWW. In the meantime, the macronutrients and micronutrients in the digestive solution were supplemented in the process. Similar to the pretreatment method of DigestateM, after centrifugation, the BWW was first filtered through a 100-mesh filter bag and then filtered again through a sand core filter combined with a 0.45 µm filter membrane. Finally, the physicochemical properties (Table 1) were analyzed, and the BWW was placed at −18°C for subsequent use. The proportion of BWW added was fixed at 20% (v/v), based on Koutra et al. (2019) experimental study on the addition of nutrient elements in similar digestate. When BWW was used to replace part of the deionized water, the proportion of DigestateM still started from 10% and then increased to 30%, 50%, and 80%, during which the proportion of BWW was maintained at 20%. Experimental groups designed according to different dilution ratios of DigestateM included 10% DigestateM, 10% DigestateM + 20% BWW, 30% DigestateM + 20% BWW, 50% DigestateM + 20% BWW and 80% DigestateM + 20% BWW, which were all photoheterotrophic cultivation. As a control group, Chlorella sp. was also cultivated in 20% BWW in the absence of DigestateM. Heterotrophic cultivation in 30% DigestateM + 20% BWW was performed. Chlorella sp. cultured in BG11 medium served as the control group for other experimental conditions. In order to simplify the expression, “DigestateM” in the name of the experimental group will be omitted in subsequent articles and the expression after omission is shown in Table 2.
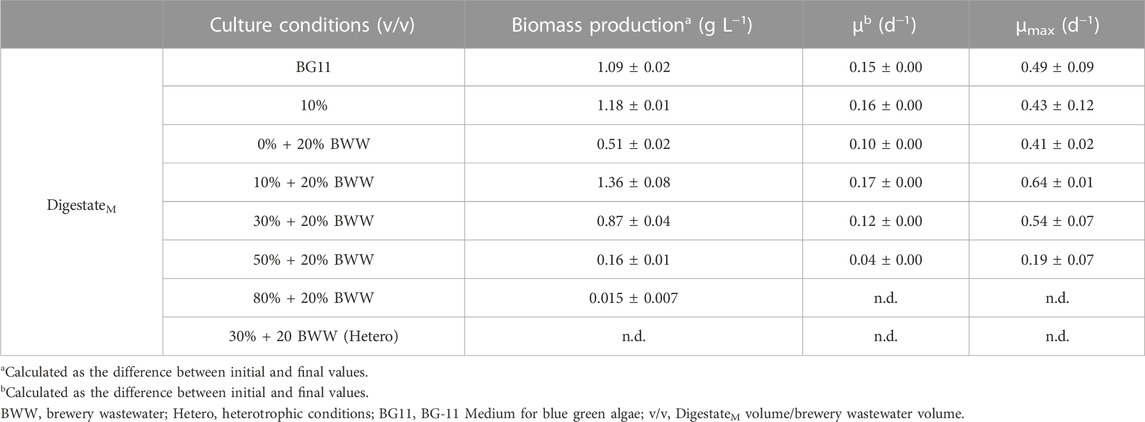
TABLE 2. Biomass production, average growth rate (µ), and maximum growth rate (µmax) of Chlorella sp. under different experimental conditions. Data are means ± SD (n = 2).
2.3 Analytical methods
2.3.1 Determination of biomass concentration and specific growth rate
First, the mass of the centrifuge tube was measured and denoted as m1. Then, 10 mL of the sample was centrifuged (D3024, DLAB) to remove the supernatant. Rinse with deionized water for three times and then add 5 mL of deionized water for resuscitation. Density gradient centrifugation was used to separate the suspensions. The gradient medium was 5 mL 50% sucrose solution and 5 mL 15% sucrose solution, centrifuged at 8,000 r min−1 for 5 min. The bacteria were located in the upper liquid and Chlorella sp. precipitates to the bottom of the centrifuge tube, which was collected separately and vacuum freeze-dried (JC-LDGZ-125, Tsingtao Juchuang) for 24 h. The mass of the centrifuge tube was measured again and denoted as m2. The dry weight (m) of Chlorella sp. was determined by
The formula for calculating the specific growth rate of microalgae was as follows:
where mt is the dry weight at time t, and m0 is the dry weight at time t0.
The maximum specific growth rate µmax (d−1) is related to the exponential growth period during the growth of microalgae. µmax was estimated by the slope of the logarithmic plot of dry weight concentration versus cultivation time.
2.3.2 Determination of pigment content of Chlorella sp.
First, 2 mL of samples were collected at the same time every day during the cultivation of microalgae and centrifuged at 10,000 r min−1 for 5 min to remove the supernatant. Then, they were washed with deionized water for three times and centrifuged again to remove the supernatant. Next, the pellet was mixed with methanol and cultured at 45°C away from light for 24 h. Lastly, the supernatant was taken after centrifugation to measure the absorbance. The contents of chlorophyll a (Ch a), chlorophyll b (Ch b), and carotenoids were calculated using the following formulas (Pancha et al., 2015):
The absorbances at 470, 652.4, and 665.2 nm were corrected by subtracting the absorbance at 750 nm.
2.3.3 DigestateM and BWW physicochemical analysis
DigestateM and BWW colors were determined as optical density measured at 550 nm (X-5 UV/VIS MWTASH). pH was determined with a pH meter (PHS–3C, INESA). The COD, TN, TP, and NH4+-N in DigestateM and BWW were measured using an automatic moisture analyzer (YKM-T4, YOKE). The kits were supplied by the same company. Total solids and volatile solids were measured in accordance with standard methods (Association and Washington, 1995).
2.3.4 Biomass composition analysis
After the experiment, the microalgal solution under various experimental conditions was centrifuged, and the supernatant was removed to keep the algal pellet. The algal pellet was washed 3 times with deionized water and then placed in a vacuum freeze-dryer (JC-LDGZ-125, Tsingtao Juchuang) for 24 h. The freeze-dried algal powder was stored in the dryer at 4°C for following use.
The algal powder was resuspended with phosphate buffer. The microalgal resuspension solution was crushed by 400 W ultrasonic wave (XH-300UA Soniwave, Beijing Xianghu) for 30 min (ice bath) and subsequently centrifuged at 10,000 r min−1 for 10 min, and the supernatant was taken. The protein content was analyzed using a BCA protein concentration assay kit (Beyotime, China). The carbohydrate content was analyzed using a carbohydrate content assay kit (Boxbio China).
The lipid of Chlorella sp. was extracted using the method of Bligh and Dyer (Bligh and Dyer, 1959). A certain amount of algal powder was weighed and fully ground in a mortar. Microalgal lipids were extracted with mixed organic solvents of chloroform–methanol (2:1; v/v). The mixture phase was oscillated at 150 r min−1 in a shaker for 30 min and then centrifuged at 4,000 r min−1 for 10 min to recover the supernatant (oil-bearing phase). The above extraction steps were repeated for the ground algal powder several times until the algal powder turned white. All lipid-containing phases were mixed in a 50 mL centrifuge tube that had been dried and weighed and denoted as W1 (g). They were then placed in a drying oven (WHL-65B, TAI-SITE) to dry to a constant weight at 37°C and denoted as W2 (g). The lipid content (LC) of Chlorella sp. was calculated in accordance with the following formula:
where W2 (g) is the total weight, W1 (g) is the tube weight, and W (g) is the sample weight.
2.3.5 Measurement of photosynthetic fluorescence parameters
Fo, Fm, Fs, Fm’, F’, Fv/Fm, and ΦPSII were directly measured with a pulse modulation fluorometer (PHYTO-PAM-II/ED, WALZ). The electron transport rate (ETR) and effective photochemical quantum yield of PSII [Y(II)] were calculated using the following formulas (Roháek and Barták, 1999):
ETR represents the effective photosynthetic ETR, and PFD represents the actual luminous flux density. ΦPSII represents the effective quantum yield of PSII photochemical energy conversion. Fv/Fm represents the maximum quantum yield of PSII photochemistry. PSII minimal fluorescence Fo and maximum fluorescence Fm were measured after dark adaptation. Fs represents the steady-state fluorescence yield, and Fm’ represents the fluorescence yield after light adaptation; all PSII reaction centers are closed, and all nonphotochemical reactions are at the optimal state. F’ represents the real-time fluorescence yield before performing the saturation pulse.
3 Results and discussion
3.1 Growth of Chlorella sp. under different experimental conditions
DigestateM from anaerobic fermentation of BWG and BWW was used to cultivate Chlorella sp. under different experimental conditions in this study. As shown in Table 1, the COD, pH, TN, and TP of DigestateM were relatively high, and the value of OD550 reached 2.37, which indicated a darker color and required a higher dilution ratio before use for Chlorella sp. Cultivation (Koutra et al., 2018b). Therefore, the DigestateM ratio started from 10% (v/v); the biomass production of Chlorella sp. reached 1.18 g L−1 with µ of 0.15 and µmax of 0.43, as presented in Table 2; the incubation time was 11 days under all experimental conditions. When 20% BWW was added, the biomass production and µmax were increased to 1.36 g L−1 and 0.64 d−1, respectively. As shown in Figure 1A, when only 20% BWW was added, the growth trend was similar to that with 10% + 20% BWW, and a significant reduction in biomass production, by 63.57%, occurred, which was due to the insufficient nutrient of BWW (Chiranjeevi and Mohan, 2016). The BWW addition was maintained at 20%, and the proportion of effluent was increased during the subsequent cultivation process. When the proportion of DigestateM was increased to 50%, the biomass production of Chlorella sp. was seriously reduced. When the effluent was increased to 80% loading, the growth of microalge was completely inhibited.
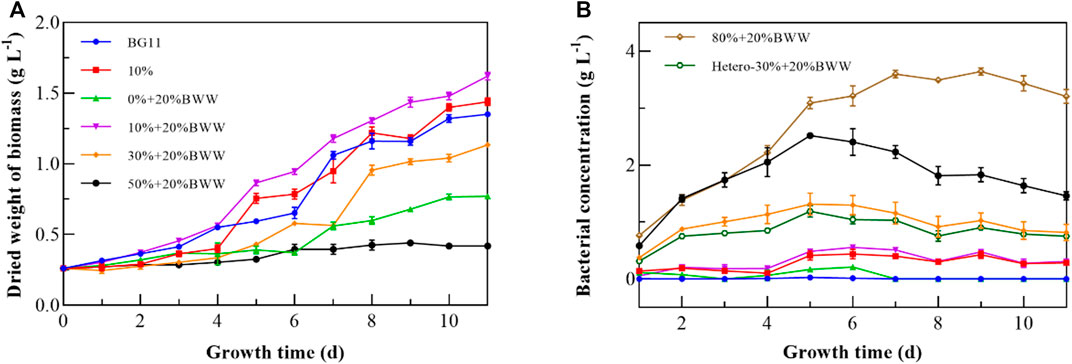
FIGURE 1. Growth curves of Chlorella sp. (A) and bacteria (B) under different experimental conditions. BWW: brewery wastewater; Hetero: heterotrophic conditions; BG11: BG-11 Medium for blue green algae; 10%: 10% DigestateM; 10% + 20% BWW: 10% DigestateM + 20% BWW.
In this study, proliferation of Chlorella sp. could not proceed under heterotrophic conditions, which indicated that the present nutrient elements in DigestateM did not allow Chlorella sp. to grow without light. Similar to Perez-Garcia et al. (2011) findings, C. vulgaris could survive only when organic carbon was added to municipal wastewater under heterotrophic conditions. The reason is that with the increase in the proportion of DigestateM, the color darkening reduced the transmittance, which in turn decreased the biomass production (Marcilhac et al., 2014). The inhibitory effect of a high concentration of digestate on microalgae was also reflected in the destructive effect of a high concentration of NH4+-N on microalgal cells (Collos and Harrison, 2014). However, previous studies have shown that Chlorella sp. that was selected in the paper is resistant to high levels of NH4+-N (Ayre et al., 2017). Bacterial proliferation in digestate, which causes survival competition, is also a factor that inhibits the growth of microalgae and is inevitable (Erkelens et al., 2014), but a certain amount of bacterial growth is acceptable and necessary for microalgae in wastewater (Peccia et al., 2013). The group of 10% + 20% BWW obtained the highest biomass production of 1.40 g L−1, which may exert a positive effect by producing symbiotic bacteria that break down large molecules into smaller ones used by microalgae directly (Monlau et al., 2015). Compared with the control group BG11, the biomass production of 10% + 20% BWW was improved by 0.31 g L−1, and µmax was increased by 0.15 d−1, which might be due to the synergistic effect of symbiotic bacteria. By comparing Figures 1A, B, we can draw the same conclusion that low concentration of symbiotic bacteria corresponds to high growth rate of Chlorella sp. From Figure 1B, the endophytic bacteria entered a stable phase from about the fifth day, and the concentration of symbiotic bacteria at this time affected the lag phase of Chlorella sp. When the proportion of DigestateM increased from 10% to 30%, the concentration of symbiotic bacteria increased by 2.71 times, which led to the extension of the lag phase. Koutra et al. (2021) found that when the loading of effluent increased to more than 30%, the lag phase increased to about 40 days, accompanied by significant bacterial growth. Accordingly, the growth of Chlorella sp. will be inhibited when the concentration of symbiotic bacteria on the fifth day, which is the common starting point of Chlorella sp.’s exponential phase and symbiotic bacteria’s stable phase, is about twice that of Chlorella sp.
3.2 Photosynthetic fluorescence parameters
Figure 2 shows the variation curves of the photosynthetic fluorescence parameters of Chlorella sp. with time under different experimental conditions. Combined with Figure 1, Figure 2 depicts that when Chlorella sp.’s growth was not inhibited, ETR (Figure 2A) showed a trend of first increasing and then stabilizing. Group 0% + 20% BWW experienced a rapid reduction in ETR caused by the pressure of nutrient consumption (Hideg et al., 1998). In the early stage of group 20% + 20% BWW, the rapid growth of symbiotic bacteria inhibited the proliferation of Chlorella sp., and the inflection point of the rapid increase in ETR was the time node when the symbiotic bacteria entered the stable phase. Subsequently, the rapid increase in ETR greatly improved the biomass yield of Chlorella sp. The ETR of 50% + 20% BWW began to increase slowly from zero at the seventh day, which verified that the massive proliferation of bacteria would inhibit the growth of Chlorella, resulting in a longer lag phase. Fv/Fm (Figure 2B) and Y (II) (Figure 2C) had similar trends to ETR, which were all positively correlated with microalgal growth curves (Cheng et al., 2019; Xu et al., 2021). Hence, the photosynthetic fluorescence parameters ETR, Fv/Fm, and Y (II) can be used to quickly evaluate the final relative value of microalgal concentration in wastewater (Wang et al., 2022). Rapid evaluation of the relative values of microalgal concentration under different experimental conditions is very important for selecting the best culture conditions and for subsequent large-scale applications (Uysal and Ekinci, 2021). Given that most wastewater contains color and particles, the relative concentration of microalgal biomass is difficult to evaluate by spectrophotometry (Cai et al., 2013).
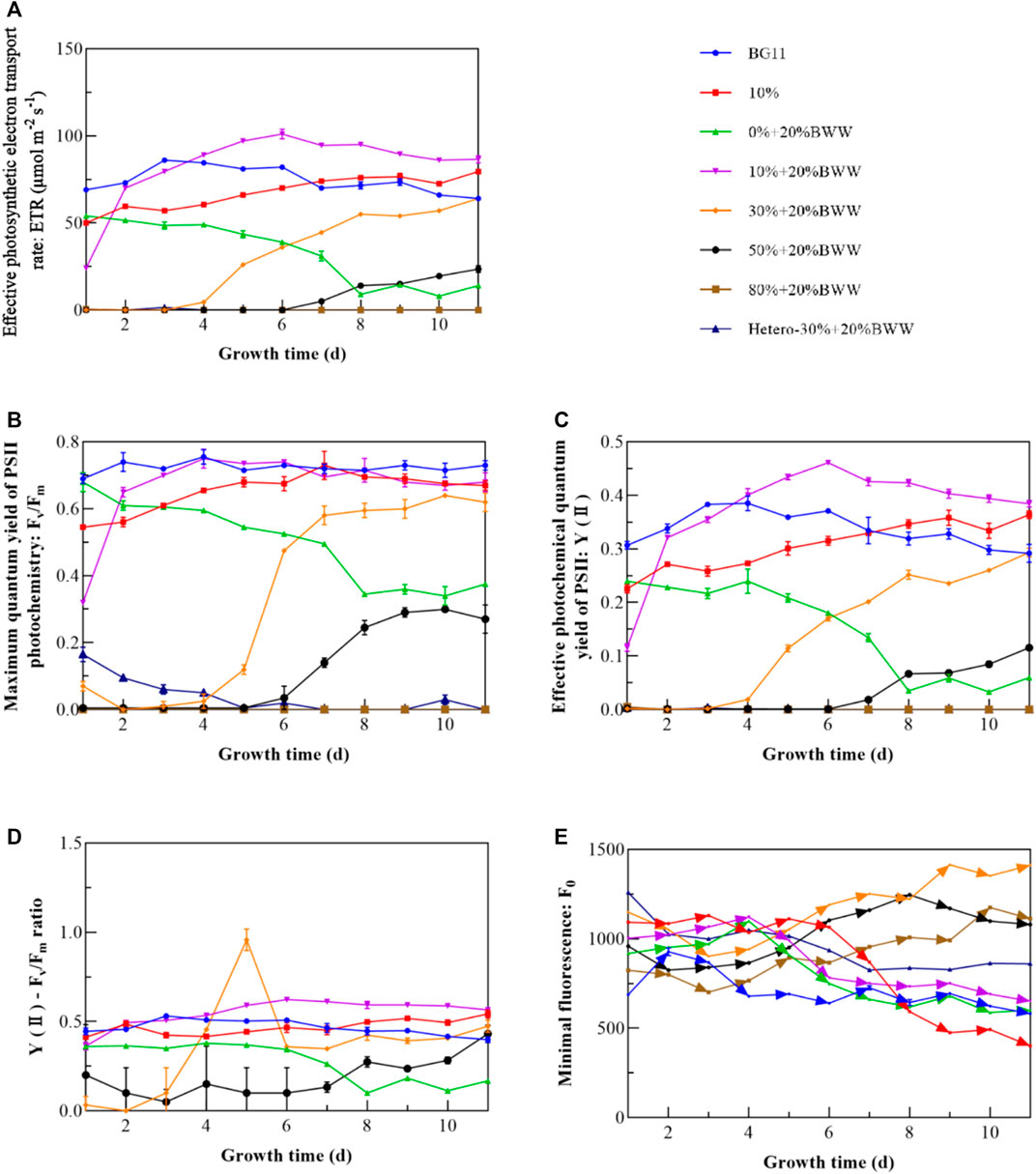
FIGURE 2. Photosynthetic fluorescence parameters of Chlorella sp. under different experimental conditions. (A) Effective photosynthetic electron transport rate (ETR); (B) maximum quantum yield of PSII photochemistry (Fv/Fm); (C) effective photochemical quantum yield of PSII [Y(II)]; (D) Y(II)–Fv/Fm ratio; (E) minimal fluorescence (Fo). BWW: brewery wastewater; Hetero: heterotrophic conditions. BG11: BG-11 Medium for blue green algae; 10%: 10% DigestateM; 10% + 20% BWW: 10% DigestateM + 20% BWW.
According to F0 (Figure 2E), the Fv/Fm values of groups BG11, 10%, and 10% + 20% BWW remained high in the late growth period, mainly due to the rapid reduction in F0 and its maintenance at a small value. This result indicated that microalgae can synthesize enough reaction center and maintain a high photochemical quenching potential of PSII under the condition of adequate nutrition and no exogenous bacterial stress. As the F0 of 30% + 20% BWW increased, Fv/Fm also increased, probably because Fm increased more than F0. The analysis of the ratio of Y(Ⅱ) to Fv/Fm (Figure 2D) demonstrated that the average ratio of the experimental groups 10% and 10% + 20% BWW was larger than that of the control group BG11. Thus, when Chlorella sp.’s growth was not inhibited in DigestateM, mixotrophic cultivation might improve the effective photochemical quantum yield of PSII. Compared with the control group BG11, the Y(II)–Fv/Fm ratio of 30% + 20% BWW and 50% + 20% BWW exceeded that of the control group after the growth of Chlorella sp. recovered, indicating that the utilization rate of visible light by Chlorella sp. would be significantly improved in an environment with low light transmission rate. From Figures 1A, 2D, the growth of Chlorella sp. may be inhibited when the Y(II)–Fv/Fm ratio is less than 0.4.
3.3 Pigment characterization
To study the influence of different culture conditions on the photosynthetic process of Chlorella sp., the pigment composition of Chlorella sp. was measured throughout the experiments. The change in photosynthetic pigment components is a common stress response of microalgae to environmental change (Park et al., 2015). From Figures 3A, B, excluding heterotrophic cultivation, the chlorophyll content in the experimental group 10% + 20% BWW was the highest (18.49 mg L−1), and the lowest was observed for 50% + 20% BWW (0.20 mg L−1). When BWW was added to the medium (10% + 20% BWW), the chlorophyll content was increased by 2.75 mg L−1 compared with that in the presence of 10% DigestateM alone (10%), which was similar to the previous research result of Yu et al. (2017); that is, the increase in NH4+-N concentration in the medium was conducive to the accumulation of chlorophyll. The chlorophyll content in BG11 was 27.73 mg L−1, the highest among all experimental conditions. Compared with BG11, the experimental group 10% + 20% BWW did not show photoacclimation (Deng et al., 2017), which might be due to the higher ETR and effective photochemical quantum yield of PSII in this experimental group, thus reducing the influence of low irradiance stress. Further analysis showed that when 10% DigestateM was added to the medium (10% + 20% BWW), the chlorophyll content was significantly increased by 16.37 mg L−1 compared with that when 20% BWW was added alone, which was similar to the results of Benavente-Valdés et al. (2017). Nevertheless, the results of this paper were contrary to the previous fidings of chlorophyll concentration decrease when organic carbon was added to stimulate heterotrophic metabolism (Ge et al., 2018). The Ch a–carotenoid ratio (Figure 3D) is an important indicator to determine whether microalgae are stressed, and the composition of medium (N stress) has an important relationship with the ratio (Scarponi et al., 2021). In 1988, Hooks et al. (1988) found that the Ch a–carotenoid ratio of microalgae under normal physiological conditions ranged from 2 to 7. In 1994, Becker (1994) reported that nitrogen starvation during the cultivation process would lead to a decrease in chlorophyll and an increase in carotenoids, which provided a theoretical basis for Hooks’s experimental data. Except for group 30% + 20% BWW, the Ch a–carotenoid ratio decreased gradually with the extension of culture time in both autotrophic and mixotrophic cultivation. As shown in Figures 1, 3, the reason why the Ch a–carotenoid ratio of 30% + 20% BWW decreased first and then increased rapidly might be the rapid growth of symbiotic bacteria in the early stage, which then lysed to release nitrogen sources, inducing the endophytic bacteria’s decline period and making Chlorella sp. became the dominant group. The Ch a–carotenoid ratio of 50% + 20% BWW and 80% + 20% BWW on the fifth day were 1.78 and 2.83, respectively, indicating severe nitrogen starvation at 50% and 80% effluent ratios, which may provide a way to improve the LC of microalgae (Safi et al., 2014). When the proportion of digestive fluid was 10%, the growth of Chlorella sp. was not only normal, but the ratio of Ch a–carotenoid was also higher than that in BG11 (2.60), indicating that the nitrogen source in biogas slurry was relatively sufficient. Photosynthetic pigment was almost not synthesized in heterotrophic culture (Mohammad Mirzaie et al., 2016), which was also the reason why the Ch a–carotenoid ratio of Hetero-30% + 20% BWW was always high.
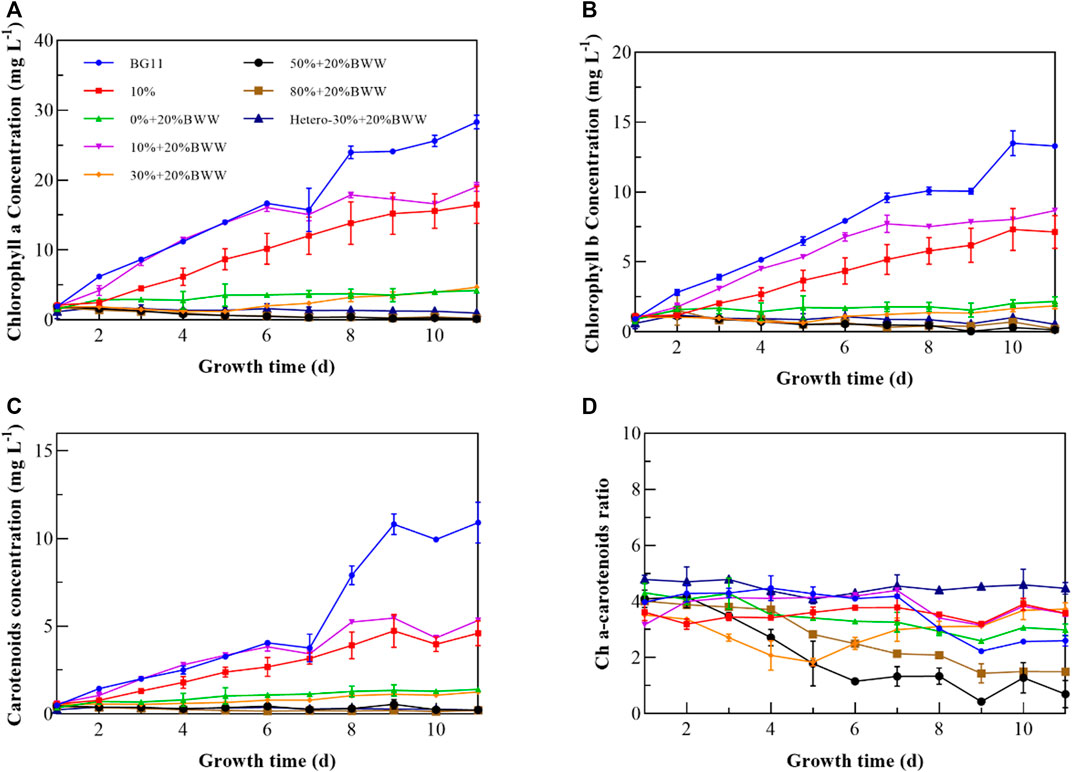
FIGURE 3. Pigment characterization of Chlorella sp. cells under different experimental conditions. (A) Chlorophyll a content; (B) chlorophyll b content; (C) carotenoid content; (D) chlorophyll a–carotenoid ratio. BWW, brewery wastewater; Hetero, heterotrophic conditions. BG11, BG-11 Medium for blue green algae; 10%: 10% DigestateM; 10% + 20% BWW: 10% DigestateM + 20% BWW.
3.4 Nutrient removal from DigestateM
In recent years, the effectiveness of microalgal biological process in removing organic matter and nutrients from wastewater is the main target of related research. Ammonia concentration was measured to observe the trend under those test conditions during the microalgal cultivation (Figure 4). As shown in Table 3, except for 0% + 20% BWW, the removal rate of ammonia was higher than 97.00% after 11 days. The highest removal rate of NH4+-N was 98.20% (30% + 20% BWW), and the maximum removal amount was 1138.8 mg L−1 (80% + 20% BWW), which was similar to the previous research results almost achieving complete removal of NH4+-N. Ledda et al. (2015) treated swine manure obtained by ultrafiltration with wild Chlorella sp., and the maximum removal rate for NH4+-N was 98.00% after 14 days. Franchino et al. (2013) used C. vulgaris for nutrition correction of agro-zootechnical digestate, and the NH4+-N removal rate reached 99.90% after 20 days. Although the removal rate for NH4+-N in group 0% + 20% BWW was only 68.70%, the remaining amount of NH4+-N in the medium was only 2.78 mg L−1, which was caused by the low initial concentration of ammonia nitrogen. When C. vulgaris was used to treat digestate from piggery effluent with an initial concentration of ammonia nitrogen of 20.60 mg L−1, the removal rate for NH4+-N was 54% after 10 days (Jeevan Kumar et al., 2017). Cicci and Bravi (2014) diluted cattle digestate with an initial concentration of ammonia nitrogen of 82 mg L−1 by 10 times and cultured Scenedesmus dimorphus, but the removal rate for NH4+-N was only 30%. According to previous experimental results, when the ammonia nitrogen content in wastewater is less than 3–5 mg L−1, its concentration is difficult to reduce even if the removal rate is above 99.00% (Massa et al., 2017). To sum up, when the concentration of ammonia nitrogen in wastewater is lower than 5 mg L−1, complete removal of ammonia nitrogen can be assumed even if the removal rate is very low. From Figure 4, the ammonia concentration under all experimental conditions dropped rapidly to about 50.00% on the fourth day, which was similar to the experimental result of Scarponi et al. (2021), who found that the ammonia concentration dropped by 50% on the first day because of the air stripping effect. The air stripping effect plays a dominant role in the removal of NH4+-N under heterotrophic conditions (Ruiz-Martinez et al., 2012; Kim et al., 2016). In this study, the removal rate for ammonia nitrogen under heterotrophic conditions was 97.69%. In the presence of DigestateM, the minimum COD removal rate was 87.33% (10% + 20% BWW). With the increase in DigestateM proportion, the COD removal rate gradually reached the maximum, which was 89.89% (80% + 20% BWW). Furthermore, during cultivation in 20% BWW, 121.85 mg L−1 of COD was removed (61.22% removal rate), while 10% DigestateM addition resulted in COD consumption of 4.2 g L−1, in contrast to 4.0 g L−1 in 10% DigestateM alone. When the proportion of effluent was 30%, the COD removal rate of mixotrophic cultivation was only increased by 1.30% compared with that in heterotrophic cultivation, which might be caused by the proliferation of symbiotic bacteria (Praveen et al., 2018) that consumed COD in digestate in the absence of Chlorella sp. growth.
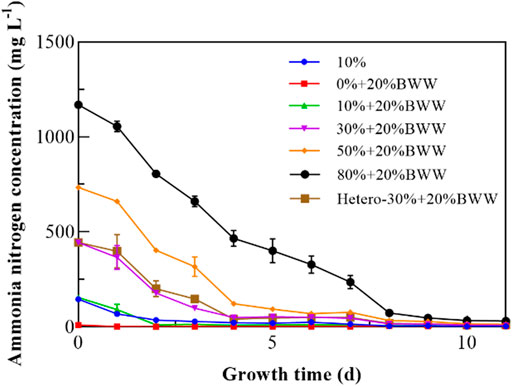
FIGURE 4. Variation in the concentration of ammonia nitrogen with time. BWW: brewery wastewater; Hetero: heterotrophic conditions. BG11: BG-11 Medium for blue green algae; 10%: 10% DigestateM; 10% + 20% BWW: 10% DigestateM + 20% BWW.
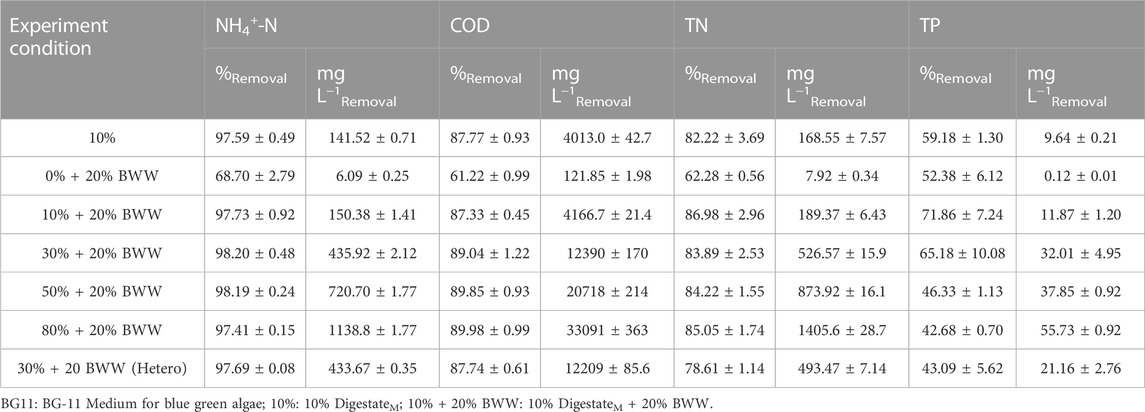
TABLE 3. Remediation of DigestateM during cultivation of Chlorella sp., expressed as NH4+-N, COD, TN, and TP removal. BWW: brewery wastewater; Hetero: heterotrophic conditions.
Nitrogen is an essential element in microalgal growth environment. In this paper, the removal rate for TN during cultivation of Chlorella sp. varied between 62.28% and 86.98%, observed in 0% + 20% BWW and in 10% + 20% BWW, respectively, and the maximum TN removal concentration was 1.41 g L−1 (80% + 20% BWW). However, when the proportion of DigestateM increased to more than 30% and the growth of Chlorella sp. was severely inhibited, the TN removal rate could still reach more than 84.00%, which might be caused by the removal of NH4+-N through the air stripping effect and the growth of heterotrophic microorganisms consuming nitrogen sources (Ledda et al., 2015). Bacteria can remove nitrogen source from digestates and is the main force in removing nitrogen source. By comparing 30% + 20 BWW (Hetero) and 30% + 20% BWW, it can be found that the removal rate of nitrogen source by microalgae is only 5.28%, while the removal rate of endophytic bacteria is 78.61%. Comparison of Tables 2, 3 showed that the removal rate for TP under photoheterotrophic condition was positively correlated with the concentration of microalgal biomass. The TP removal rate of group 10% + 20% BWW with the highest concentration of microalgal biomass was 71.86%; meanwhile, that of group 80% + 20% BWW with severely inhibited growth of microalgae was only 42.68%, which was similar to the result of a previous experiment (Liang et al., 2010). Under the heterotrophic condition, the TP removal rate was 43.09, which was 22.09% lower than that of the control group (30% + 20% BWW), indicating that symbiotic heterotrophic bacteria played an auxiliary role in the absorption of TP (De-Bashan et al., 2002). In conclusion, in the symbiotic system of algae–bacteria, the absorption of TP by microalgae is dominant (de-Bashan et al., 2004; Cruz et al., 2013).
3.5 Biochemical composition of Chlorella sp. under different regimes
Chlorella sp. was collected after culture, and its biomass chemical composition was tested to determine its subsequent applications, such as production of biofuels, feed additives, and antioxidant agents. From Figure 5, the main components of Chlorella sp. biomass under all photoheterotrophic experimental conditions were carbohydrates and lipids. LC ranged from 19.90% (Herero - 30% + 20% BWW) to 41.60% (0% + 20% BWW) under all experimental conditions, and the LC of the experimental groups 0% + 20% BWW, 10% + 20% BWW (27.80%), and 50% + 20% BWW (36.80%) was higher than that of control group BG11 (27.10%). The highest LC in this experiment was 41.60%, which was 1.60% higher than the 40.00% LC obtained by Yang et al. using domesticated C. ellipsoideaYJ1 cultivated in domestic secondary effluent (Jia et al., 2011). This phenomenon might be caused by the absence of nitrogen in the medium (Zhu et al., 2015), and LC increased by 9.00% when the nitrate concentration was reduced from 1.50 g L−1 to 0.38 g L−1 (Converti et al., 2009). Mujtaba et al. (2012) found that the LC of Chlorella sp. was as high as 53% when the concentration of nitrogen source was only 5 mg L−1. For further comparison, Li et al. (2011) cultured Chlorella sp. in municipal wastewater and obtained 11.00% LC, Qin et al. (2014) cultured C. vulgaris in dairy wastewater and obtained 10.30% LC, and Cho et al. (2013) cultured Chlorella sp. 227 in wastewater from an anaerobic digestion tank and obtained 10.80% LC, which was about 50% of the lowest LC in photoheterotrophic experimental conditions. Under heterotrophic conditions, the LC was 8.82%, which was slightly higher than Ge et al.'s 5.60% (Ge et al., 2018).
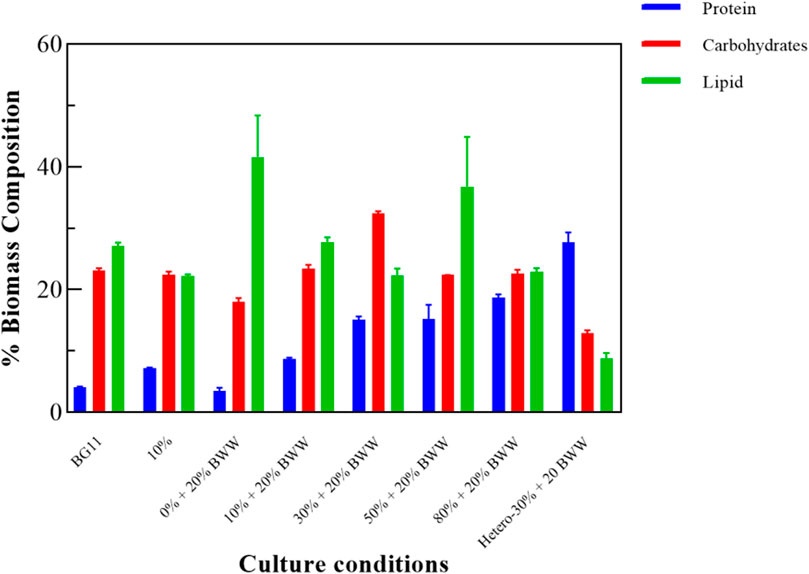
FIGURE 5. Biochemical composition of Chlorella sp. biomass under different experimental conditions. BWW: brewery wastewater; Hetero: heterotrophic conditions. BG11: BG-11 Medium for blue green algae; 10%: 10% DigestateM; 10% + 20% BWW: 10% DigestateM + 20% BWW.
From the analysis of carbohydrates, the carbohydrate content of group 30% + 20% BWW was the highest, which was 9.32% higher than that of the control group BG11 (23.12%). Figure 3D illustrates that the nutrient change curve (the Ch a–carotenoid ratio curve) of group 30% + 20% BWW was similar to that of the fed-batch fermentation method, first decreasing and then increasing, which was conducive to carbohydrate accumulation under the mixotrophic condition (Sivakaminathan, 2012). Meanwhile, the carbohydrate content of group 10% + 20% BWW was slightly higher than that of the control group, and the rest were lower than that, which might be due to the absence of a nitrogen-deficient environment or inhibited growth (Abreu et al., 2012; Goncalves et al., 2018). Nonetheless, all the experimental groups, except group 0% + 20% BWW (3.46%), had a higher protein content than the control group (4.14%), and the highest protein content was 27.72% in group Herero—30% + 20% BWW. Previous experimental results were similar to those in this experiment: The protein content of microalgae under heterotrophic condition was as high as 63.00%, which was 13.20% higher than that in photoheterotrophic condition (Ge et al., 2018). The protein content increased with the increase in digested effluent concentration because a high-nitrogen medium was beneficial to the protein synthesis of microalgae (Zhang et al., 2018).
4 Conclusion
DigestateM from anaerobic fermentation of BWG and BWW was effectively used for cultivation of Chlorella sp. The concentration of endophytic bacteria was more than twice that of Chlorella sp. when they entered the stable stage, which would prolong the lag period of Chlorella sp. Further analysis of photosynthetic fluorescence parameters and pigment content showed that photoacclimation did not form with the increase in DigestateM proportion, which might be due to the higher ETR and effective photochemical quantum yield of PSII, thus reducing the influence of low irradiance stress. The growth of Chlorella sp. may be inhibited when the Y(II)–Fv/Fm ratio is less than 0.4. The photosynthetic fluorescence parameters ETR, Fv/Fm, and Y (II) can be used to quickly evaluate the final relative value of microalgal concentration in wastewater. Based on the results of this study and previous research, when the concentration of NH4+-N in wastewater is lower than 5 mg L−1, complete removal of NH4+-N can be assumed even if the removal rate is very low. In the symbiotic system of algae–bacteria, the absorption of TP by microalgae is dominant. The protein content increased with the increase in DigestateM concentration.
Data availability statement
The original contributions presented in the study are included in the article/Supplementary Material, further inquiries can be directed to the corresponding author.
Author contributions
SW: ideas; formulation or evolution of overarching research goals and aims. Preparation, creation and/or presentation of the published work, specifically writing the initial draft. QZ: preparation, creation and/or presentation of the published work by those from the original research group, specifically critical review, commentary or revision–including pre-or postpublication stages. The author has equal contribution with the first author. HY: application of statistical, mathematical, computational, or other formal techniques to analyze or synthesize study data. XD: application of statistical, mathematical, computational, or other formal techniques to analyze or synthesize study data. TZ: application of statistical, mathematical, computational, or other formal techniques to analyze or synthesize study data. TS: application of statistical, mathematical, computational, or other formal techniques to analyze or synthesize study data. WS: acquisition of the financial support for the project leading to this publication. Preparation, creation and/or presentation of the published work by those from the original research group, specifically critical review, commentary or revision including pre-or postpublication stages. All authors contributed to the article and approved the submitted version.
Funding
This work was supported by the Shandong Provincial Natural Science Foundation (Grant Number ZR2019MC060), Science and Technology Support Plan for Youth Innovation of Colleges and Universities of Shandong Province (Grant Number 2021KJ109), Key Project Servers the Yellow River National strategy of Jining University (Grant Number 2022HHKJ09).
Conflict of interest
The authors declare that the research was conducted in the absence of any commercial or financial relationships that could be construed as a potential conflict of interest.
Publisher’s note
All claims expressed in this article are solely those of the authors and do not necessarily represent those of their affiliated organizations, or those of the publisher, the editors and the reviewers. Any product that may be evaluated in this article, or claim that may be made by its manufacturer, is not guaranteed or endorsed by the publisher.
References
Abreu, A. P., Fernandes, B., Vicente, A. A., Teixeira, J., and Dragone, G. (2012). Mixotrophic cultivation of Chlorella vulgaris using industrial dairy waste as organic carbon source. Bioresour. Technol. 118, 61–66. doi:10.1016/j.biortech.2012.05.055
Alavijeh, R. S., Karimi, K., Wijffels, R. H., Berg, C., and Eppink, M. (2020). Combined bead milling and enzymatic hydrolysis for efficient fractionation of lipids, proteins, and carbohydrates of Chlorella vulgaris microalgae. Bioresour. Technol. 309, 123321. doi:10.1016/j.biortech.2020.123321
Aliyu, S., and Bala, M. (2011). Brewer’s spent grain: A review of its potentials and applications. Afr. J. Biotechnol. 10, 324–331.
Association, C., and Washington, D. (1995). Apha: Standard methods for the examination of water and wastewater. Am. Phys. Educ. Rev. 24, 481–486. doi:10.2105/ajph.56.3.387
Ayre, J. M., Moheimani, N. R., and Borowitzka, M. A. (2017). Growth of microalgae on undiluted anaerobic digestate of piggery effluent with high ammonium concentrations. Algal Res. 24, 218–226. doi:10.1016/j.algal.2017.03.023
Becker, E. W. (1994). Microalgae: Biotechnology and microbiology. United States: Cambridge University Press. doi:10.1017/S0014479700025126
Benavente-Valdés, J. R., Méndez-Zavala, A., Morales-Oyervides, L., Chisti, Y., and Monta?Ez, J. (2017). Effects of shear rate, photoautotrophy and photoheterotrophy on production of biomass and pigments by Chlorella vulgaris. J. Chem. Technol. Biotechnol. 92, 2453–2459. doi:10.1002/jctb.5256
Bligh, E., and Dyer, W. (1959). A rapid method of total lipid extraction and purification. Can. J. Biochem. Physiol. 37, 911–917. doi:10.1139/y59-099
Bonetta, S., Bonetta, S., Ferretti, E., Fezia, G., Gilli, G., and Carraro, E. (2014). Agricultural reuse of the digestate from anaerobic Co-digestion of organic waste: Microbiological contamination, metal hazards and fertilizing performance. Water, Air, Soil Pollut. 225, 2041–2046. doi:10.1007/s11270-014-2046-2
Cai, T., Park, S. Y., Racharaks, R., and Li, Y. (2013). Cultivation of Nannochloropsis salina using anaerobic digestion effluent as a nutrient source for biofuel production. Appl. Energy 108, 486–492. doi:10.1016/j.apenergy.2013.03.056
Changling, L., Yang, H., Yuji, L., and Wang, W. (2014). Effect of culture models on metabolism and protein components of microalgae Chlorella vulgaris. J. Food Sci. Biotechnol. 15, 16–24.
Chen, H., Chang, S., Guo, Q., Hong, Y., and Wu, P. (2016). Brewery wastewater treatment using an anaerobic membrane bioreactor. Biochem. Eng. J. 105, 321–331. doi:10.1016/j.bej.2015.10.006
Cheng, J., Qiu, Y., Huang, R., Yang, W., Zhou, J., and Cen, K. (2016). Biodiesel production from wet microalgae by using graphene oxide as solid acid catalyst. Bioresour. Technol. 221, 344–349. doi:10.1016/j.biortech.2016.09.064
Cheng, J., Wang, Z., Lu, H., Xu, J., and Cen, K. (2019). Hydrogen sulfide promotes cell division and photosynthesis of nannochloropsis oceanica with 15% carbon dioxide. ACS Sustain. Chem. Eng. 7, 16344–16354. doi:10.1021/acssuschemeng.9b03398
Chew, K. W., Chia, S. R., Show, P. L., Yap, Y. J., Ling, T. C., and Chang, J-S. (2018). Effects of water culture medium, cultivation systems and growth modes for microalgae cultivation: A review. J. Taiwan Inst. Chem. Eng. 91, 332–344. doi:10.1016/j.jtice.2018.05.039
Chiranjeevi, P., and Mohan, S. V. (2016). Critical parametric influence on microalgae cultivation towards maximizing biomass growth with simultaneous lipid productivity. Renew. Energy 98, 64–71. doi:10.1016/j.renene.2016.03.063
Cho, S., Lee, N., Park, S., Yu, J., Luong, T. T., Oh, Y-K., et al. (2013). Microalgae cultivation for bioenergy production using wastewaters from a municipal WWTP as nutritional sources. Bioresour. Technol. 131, 515–520. doi:10.1016/j.biortech.2012.12.176
Chojnacka, K., and Marquez-Rocha, F-J. (2004). Kinetic and stoichiometric relationships of the energy and carbon metabolism in the culture of microalgae. Biotechnology 3, 21–34. doi:10.3923/biotech.2004.21.34
Chuka-ogwude, D., James, O., and NavidMoheimani, R. (2020). A review on microalgal culture to treat anaerobic digestate food waste effluent. Algal Res. 47, 101841. doi:10.1016/j.algal.2020.101841
Cicci, A., and Bravi, M. (2014). Production of the freshwater microalgae scenedesmus dimorphus and arthrospira platensis by using cattle digestate. Chem. Eng. 38, 85–90. doi:10.3303/CET1438015
Collos, Y., and Harrison, P. J. (2014). Acclimation and toxicity of high ammonium concentrations to unicellular algae. Mar. Pollut. Bull. 80, 8–23. doi:10.1016/j.marpolbul.2014.01.006
Converti, A., Casazza, A., Ortiz, E. Y., Perego, P., and Borghi, M. (2009). Effect of temperature and nitrogen concentration on the growth and lipid content of Nannochloropsis oculata and Chlorella vulgaris for biodiesel production. Chem. Eng. Process. Process Intensif. 48, 1146–1151. doi:10.1016/j.cep.2009.03.006
Cruz, I., Bashan, Y., Hernàndez-Carmona, G., and de-Bashan, L. E. (2013). Biological deterioration of alginate beads containing immobilized microalgae and bacteria during tertiary wastewater treatment. Appl. Microbiol. Biotechnol. 97, 9847–9858. doi:10.1007/s00253-013-4703-6
de-Bashan, E. L., Hernandez, J-P., Morey, T., and Bashan, Y. (2004). Microalgae growth-promoting bacteria as "helpers" for microalgae: A novel approach for removing ammonium and phosphorus from municipal wastewater. Water Res. 38, 466–474. doi:10.1016/j.watres.2003.09.022
De-Bashan, L. E., Moreno, M., Hernandez, J-P., and Bashan, Y. (2002). Removal of ammonium and phosphorus ions from synthetic wastewater by the microalgae Chlorella vulgaris coimmobilized in alginate beads with the microalgae growth-promoting bacterium Azospirillum brasilense. Water Res. 36, 2941–2948. doi:10.1016/s0043-1354(01)00522-x
Deng, X. Y., Gao, K., Zhang, R. C., Addy, M., Lu, Q., Ren, H. Y., et al. (2017). Growing Chlorella vulgaris on thermophilic anaerobic digestion swine manure for nutrient removal and biomass production. Bioresour. Technol. 243, 417–425. doi:10.1016/j.biortech.2017.06.141
Dinnebier, H. C. F., Matthiensen, A., Michelon, W., Tápparo, D. C., Fonseca, T. G., Favretto, R., et al. (2021). Phycoremediation and biomass production from high strong swine wastewater for biogas generation improvement: An integrated bioprocess. Bioresour. Technol. 332, 125111. doi:10.1016/j.biortech.2021.125111
Eppink, M. H., Olivieri, G., Reith, H., Berg, C., Barbosa, M. J., and Wijffels, R. H. (2017). From current algae products to future biorefinery practices: A review. Biorefineries 2017, 99–123.
Erkelens, M., Ward, A. J., Ball, A. S., and Lewis, D. M. (2014). Microalgae digestate effluent as a growth medium for Tetraselmis sp. in the production of biofuels. Bioresour. Technol. 167, 81–86. doi:10.1016/j.biortech.2014.05.126
Fillaudeau, L., Blanpain-Avet, P., and Daufin, G. (2006). Water, wastewater and waste management in brewing industries. J. Clean. Prod. 14, 463–471. doi:10.1016/j.jclepro.2005.01.002
Franchino, M., Comino, E., Bona, F., and Riggio, V. A. (2013). Growth of three microalgae strains and nutrient removal from an agro-zootechnical digestate. Chemosphere 92, 738–744. doi:10.1016/j.chemosphere.2013.04.023
Ge, S., Qiu, S., Tremblay, D., Viner, K., Champagne, P., and Jessop, P. G. (2018). Centrate wastewater treatment with Chlorella vulgaris: Simultaneous enhancement of nutrient removal, biomass and lipid production. Chem. Eng. J. 342, 310–320. doi:10.1016/j.cej.2018.02.058
Goncalves, D. M. R., Frazao, D. A., Bezerra, R. P., Correia, D. S., Cristina, D. S. V., Araujo, V. M. D. D., et al. (2018). Chlorella vulgaris mixotrophic growth enhanced biomass productivity and reduced toxicity from agro-industrial by-products. Chemosphere 204, 344–350. doi:10.1016/j.chemosphere.2018.04.039
Gu, Z., Liu, Y., Zou, G., Zhang, Q., Lu, R., Yan, H., et al. (2021). Enhancement of nutrients removal and biomass accumulation of Chlorella vulgaris in pig manure anaerobic digestate effluent by the pretreatment of indigenous bacteria. Bioresour. Technol. 328, 124846. doi:10.1016/j.biortech.2021.124846
Guo, Y. Y., Khoo, K. S., Wen, Y. C., Ho, Y. C., Show, P. L., Leong, H. Y., et al. (2020). A novel lipids recovery strategy for biofuels generation on microalgae Chlorella cultivation with waste molasses. J. Water Process Eng. 38, 101665. doi:10.1016/j.jwpe.2020.101665
Hideg, É., Kálai, T., Hideg, K., and Vass, I. (1998). Photoinhibition of photosynthesis in vivo results in singlet oxygen production detection via nitroxide-induced fluorescence quenching in broad bean leaves. Biochemistry 37, 11405–11411. doi:10.1021/bi972890+
Hooks, C. E., Bidigare, R. R., Keller, M. D., and Guillard, R. R. L. (1988). Coccoid eukaryotic marine ultraplankters with four different hplc pigment signatures. J. Phycol. 24, 571–580. doi:10.1111/j.1529-8817.1988.tb04264.x
Jeevan Kumar, S. P., Vijay Kumar, G., Dash, A., Scholz, P., and Banerjee, R. (2017). Sustainable green solvents and techniques for lipid extraction from microalgae: A review. Algal Res. 21, 138–147. doi:10.1016/j.algal.2016.11.014
Jia, Y., Xin, L., Hu, H., Xue, Z., Yin, Y., and Chen, Y. (2011). Growth and lipid accumulation properties of a freshwater microalga, Chlorella ellipsoidea YJ1, in domestic secondary effluents. Appl. Energy 88, 3295–3299. doi:10.1016/j.apenergy.2010.11.029
Kim, G., Mujtaba, G., and Lee, K. (2016). Effects of nitrogen sources on cell growth and biochemical composition of marine chlorophyte Tetraselmis sp. for lipid production. Algae 31, 257–266. doi:10.4490/algae.2016.31.8.18
Kim, H. C., Choi, W. J., Maeng, S. K., Kim, H. J., Han, S. K., and Song, K. G. (2014). Ozonation of piggery wastewater for enhanced removal of contaminants by S. quadricauda and the impact on organic characteristics. Bioresour. Technol. 159, 128–135. doi:10.1016/j.biortech.2014.02.061
Koutra, E., Economou, C. N., Tsafrakidou, P., and Kornaros, M. (2018a). Bio-based products from microalgae cultivated in digestates. Trends Biotechnol. 36, 819–833. doi:10.1016/j.tibtech.2018.02.015
Koutra, E., Grammatikopoulos, G., and Kornaros, M. (2018b). Selection of microalgae intended for valorization of digestate from agro-waste mixtures. Waste Manag. 73, 123–129. doi:10.1016/j.wasman.2017.12.030
Koutra, E., Kopsahelis, A., Maltezou, M., Grammatikopoulos, G., and Kornaros, M. (2019). Effect of organic carbon and nutrient supplementation on the digestate-grown microalga, Parachlorella kessleri. Bioresour. Technol. 294, 122232. doi:10.1016/j.biortech.2019.122232
Koutra, E., Mastropetros, G., Ali, S. S., Tsigkou, K., and Kornaros, M. (2021). Assessing the potential of Chlorella vulgaris for valorization of liquid digestates from agro-industrial and municipal organic wastes in a biorefinery approach. J. Clean. Prod. 280, 124352. doi:10.1016/j.jclepro.2020.124352
Ledda, C., Idà, A., Allemand, D., Mariani, P., and Adani, F. (2015). Production of wild Chlorella sp. cultivated in digested and membrane-pretreated swine manure derived from a full-scale operation plant. Algal Res. 12, 68–73. doi:10.1016/j.algal.2015.08.010
Li, Y., Chen, Y-F., Chen, P., Min, M., Zhou, W., Martinez, B., et al. (2011). Characterization of a microalga Chlorella sp. well adapted to highly concentrated municipal wastewater for nutrient removal and biodiesel production. Bioresour. Technol. 102, 5138–5144. doi:10.1016/j.biortech.2011.01.091
Liang, W., Li, Y., Chen, P., Min, M., Chen, Y., Zhu, J., et al. (2010). Anaerobic digested dairy manure as a nutrient supplement for cultivation of oil-rich green microalgae Chlorella sp. Bioresour. Technol. 101, 2623–2628. doi:10.1016/j.biortech.2009.10.062
Lu, H., Peng, M., Zhang, G., Li, B., and Li, Y. (2019). Brewery wastewater treatment and resource recovery through long term continuous-mode operation in pilot photosynthetic bacteria-membrane bioreactor. Sci. Total Environ. 646, 196–205. doi:10.1016/j.scitotenv.2018.07.268
Marcilhac, C., Sialve, B., Pourcher, A-M., Ziebal, C., Bernet, N., and Béline, F. (2014). Digestate color and light intensity affect nutrient removal and competition phenomena in a microalgal-bacterial ecosystem. Water Res. 64, 278–287. doi:10.1016/j.watres.2014.07.012
Massa, M., Buono, S., Langellotti, A. L., Castaldo, L., Martello, A., Paduano, A., et al. (2017). Evaluation of anaerobic digestates from different feedstocks as growth media for Tetradesmus obliquus, Botryococcus braunii, Phaeodactylum tricornutum and Arthrospira maxima. New Biotechnol. 36, 8–16. doi:10.1016/j.nbt.2016.12.007
Mayers, J. J., Nilsson, A. E., Albers, E., and Flynn, K. J. (2017). Nutrients from anaerobic digestion effluents for cultivation of the microalga Nannochloropsis sp. — impact on growth, biochemical composition and the potential for cost and environmental impact savings. Algal Res. 26, 275–286. doi:10.1016/j.algal.2017.08.007
Mohammad Mirzaie, M. A., Kalbasi, M., Mousavi, S. M., and Ghobadian, B. (2016). Investigation of mixotrophic, heterotrophic, and autotrophic growth of Chlorella vulgaris under agricultural waste medium. Prep. Biochem. Biotechnol. 46, 150–156. doi:10.1080/10826068.2014.995812
Monlau, F., Sambusiti, C., Ficara, E., Aboulkas, A., Barakat, A., and Carrere, H. (2015). New opportunities for agricultural digestate valorization: Current situation and perspectives. Energy & Environ. Sci. 8, 2600–2621. doi:10.1039/c5ee01633a
Mujtaba, G., Choi, W., Lee, C. G., and Lee, K. (2012). Lipid production by Chlorella vulgaris after a shift from nutrient-rich to nitrogen starvation conditions. Bioresour. Technol. 123, 279–283. doi:10.1016/j.biortech.2012.07.057
Pancha, I., Chokshi, K., Maurya, R., Trivedi, K., Patidar, S. K., Ghosh, A., et al. (2015). Salinity induced oxidative stress enhanced biofuel production potential of microalgae Scenedesmus sp. CCNM 1077. Bioresour. Technol. 189, 341–348. doi:10.1016/j.biortech.2015.04.017
Park, S., Kim, M., Lee, S-G., Lee, Y., Choi, H-K., and Jin, E. (2015). Contrasting photoadaptive strategies of two morphologically distinct Dunaliella species under various salinities. J. Appl. Phycol. 27, 1053–1062. doi:10.1007/s10811-014-0394-3
Peccia, J., Haznedaroglu, B., Gutierrez, J., and Zimmerman, J. B. (2013). Nitrogen supply is an important driver of sustainable microalgae biofuel production. Trends Biotechnol. 31, 134–138. doi:10.1016/j.tibtech.2013.01.010
Perez-Garcia, O., Bashan, Y., and Esther Puente, M. (2011). Organic carbon supplementation of sterilized municipal wastewater is essential for heterotrophic growth and removing ammonium by the microalga Chlorella Vulgaris 1. J. Phycol. 47, 190–199. doi:10.1111/j.1529-8817.2010.00934.x
Praveen, P., Guo, Y., Kang, H., Lefebvre, C., and Loh, K-C. (2018). Enhancing microalgae cultivation in anaerobic digestate through nitrification. Chem. Eng. J. 354, 905–912. doi:10.1016/j.cej.2018.08.099
Qian, L., Pei, H., Fufeng, C., Tonggui, L., Jun, L., Leng, L., et al. (2019). A novel approach of using zeolite for ammonium toxicity mitigation and value-added Spirulina cultivation in wastewater. Bioresour. Technol. 280, 127–135. doi:10.1016/j.biortech.2019.02.042
Qin, L., Shu, Q., Wang, Z., Shang, C., Zhu, S., Xu, J., et al. (2014). Cultivation of Chlorella vulgaris in dairy wastewater pretreated by UV irradiation and sodium hypochlorite. Appl. Biochem. Biotechnol. 172, 1121–1130. doi:10.1007/s12010-013-0576-5
Roháek, K., and Barták, M. (1999). Technique of the modulated chlorophyll fluorescence: Basic concepts, useful parameters, and some applications. Photosynthetica 37, 339–363. doi:10.1023/A:1007172424619
Romero-Villegas, G. I., Fiamengo, M., Acién-Fernández, F., and Molina-Grima, E. (2018). Utilization of centrate for the outdoor production of marine microalgae atthe pilot-scale in raceway photobioreactors. J. Environ. Manag. 228, 506–516. doi:10.1016/j.jenvman.2018.08.020
Ruiz-Martinez, A., Garcia, N. M., Romero, I., Seco, A., and Ferrer, J. (2012). Microalgae cultivation in wastewater: Nutrient removal from anaerobic membrane bioreactor effluent. Bioresour. Technol. 126, 247–253. doi:10.1016/j.biortech.2012.09.022
Safi, C., Zebib, B., Merah, O., Pontalier, P-Y., and Vaca-Garcia, C. (2014). Morphology, composition, production, processing and applications of Chlorella vulgaris: A review. Renew. Sustain. Energy Rev. 35, 265–278. doi:10.1016/j.rser.2014.04.007
Sakarika, M., and Kornaros, M. (2019). Chlorella vulgaris as a green biofuel factory: Comparison between biodiesel, biogas and combustible biomass production. Bioresour. Technol. 273, 237–243. doi:10.1016/j.biortech.2018.11.017
Scarponi, P., Ghirardini, A., Bravi, M., and Cavinato, C. (2021). Evaluation of Chlorella vulgaris and Scenedesmus obliquus growth on pretreated organic solid waste digestate. Waste Manag. 119, 235–241. doi:10.1016/j.wasman.2020.09.047
Shahid, A., Malik, S., Zhu, H., Xu, J., Mehmood, M. A., Nawaz, S., et al. (2019). Cultivating microalgae in wastewater for biomass production, pollutant removal, and atmospheric carbon mitigation; a review. Sci. Total Environ. 704, 135303. doi:10.1016/j.scitotenv.2019.135303
Shi, X-Y., Jin, D-W., Sun, Q-Y., and Li, W-W. (2010). Optimization of conditions for hydrogen production from brewery wastewater by anaerobic sludge using desirability function approach. Renew. Energy 35, 1493–1498. doi:10.1016/j.renene.2010.01.003
Singh, R., Birru, R., and Sibi, G. (2017). Nutrient Removal Efficiencies of <i&gt;Chlorella vulgaris&lt;/i&gt; from Urban Wastewater for Reduced Eutrophication. J. Environ. Prot. 8 (1), 1–11. doi:10.4236/jep.2017.81001
Sivakaminathan, S. (2012). Biomass and lipid production from heterotrophic and mixotrophic fed-batch cultivations of microalgae Chlorella protothecoides using glycerol. United States: Clemson University.
Stiles, W., David, S., Chapman, S. P., Sandra, E., Angela, B., Lynsey, M., et al. (2018). Using microalgae in the circular economy to valorise anaerobic digestate: Challenges and opportunities. Bioresour. Technol. 267, 732–742. doi:10.1016/j.biortech.2018.07.100
Tfab, C., Mzab, D., Hrab, D., Lfab, C., Sma, B., Sbe, F., et al. (2020). Assessment of the performance of an anoxic-aerobic microalgal-bacterial system treating digestate. Chemosphere 2020, 129437. doi:10.1016/j.chemosphere.2020.129437
Uysal, N., and Ekinci, K. (2021). Treatment of rose oil processing effluent with Chlorella sp. using photobioreactor and raceway. J. Environ. Manag. 295, 113089. doi:10.1016/j.jenvman.2021.113089
Wang, J., Yang, H., and Wang, F. (2014). Mixotrophic cultivation of microalgae for biodiesel production: Status and prospects. Appl. Biochem. Biotechnol. 172, 3307–3329. doi:10.1007/s12010-014-0729-1
Wang, L., Addy, M., Liu, J., Nekich, C., Zhang, R., Peng, P., et al. (2019). Integrated process for anaerobically digested swine manure treatment. Bioresour. Technol. Feb 273, 506–514. doi:10.1016/j.biortech.2018.11.050
Wang, S., Yin, C., Yang, Z., Hu, X., Liu, Z., and Song, W. (2022). Assessing the potential of Chlorella sp. for treatment and resource utilization of brewery wastewater coupled with bioproduct production. J. Clean. Prod. 367, 132939. doi:10.1016/j.jclepro.2022.132939
Xia, A., and Murphy, J. D. (2016). Microalgal cultivation in treating liquid digestate from biogas systems. Trends Biotechnol. 34, 264–275. doi:10.1016/j.tibtech.2015.12.010
Xu, R., Zhang, L., and Liu, J. (2021). Rotifers release a lipid-soluble agent that inhibits photosynthetic electron transport in Chlorella sp. J. Appl. Phycol. 33, 57–65. doi:10.1007/s10811-020-02065-9
Yadav, G., Dash, S. K., and Sen, R. (2019). A biorefinery for valorization of industrial waste-water and flue gas by microalgae for waste mitigation, carbon-dioxide sequestration and algal biomass production. Sci. Total Environ. 688, 129–135. doi:10.1016/j.scitotenv.2019.06.024
Yu, Z., Song, M., Pei, H., Han, F., Jiang, L., and Hou, Q. (2017). The growth characteristics and biodiesel production of ten algae strains cultivated in anaerobically digested effluent from kitchen waste. Algal Res. 24, 265–275. doi:10.1016/j.algal.2017.04.010
Zhang, L., Cheng, J., Pei, H., Pan, J., Jiang, L., Hou, Q., et al. (2018). Cultivation of microalgae using anaerobically digested effluent from kitchen waste as a nutrient source for biodiesel production. Renew. Energy 115, 276–287. doi:10.1016/j.renene.2017.08.034
Zhang, T. Y., Hu, H. Y., Wu, Y. H., Zhuang, L. L., Xu, X. Q., Wang, X. X., et al. (2016). Promising solutions to solve the bottlenecks in the large-scale cultivation of microalgae for biomass/bioenergy production. Renew. Sustain. Energy Rev. 60, 1602–1614. doi:10.1016/j.rser.2016.02.008
Zhao, X. C., Tan, X. B., Yang, L. B., Liao, J. Y., and Li, X. Y. (2019). Cultivation of Chlorella pyrenoidosa in anaerobic wastewater: The coupled effects of ammonium, temperature and pH conditions on lipids compositions. Bioresour. Technol. 284, 90–97. doi:10.1016/j.biortech.2019.03.117
Zhu, L. (2015). Microalgal culture strategies for biofuel production: A review. Biofuels, Bioprod. Biorefining. 9, 801–814. doi:10.1002/bbb.1576
Keywords: digestate, Chlorella sp., brewer’s grains, biomass composition, photosynthetic fluorescence parameters, brewery wastewater
Citation: Wang S, Zhao Q, Yu H, Du X, Zhang T, Sun T and Song W (2023) Assessing the potential of Chlorella sp. phycoremediation liquid digestates from brewery wastes mixture integrated with bioproduct production. Front. Bioeng. Biotechnol. 11:1199472. doi: 10.3389/fbioe.2023.1199472
Received: 03 April 2023; Accepted: 05 June 2023;
Published: 14 June 2023.
Edited by:
Junjun Wu, Chongqing University, ChinaReviewed by:
Tonmoy Ghosh, Indian Institute of Technology Indore, IndiaKai Zhang, Nanjing Normal University, China
Xiaojun Shen, Beijing Forestry University, China
Copyright © 2023 Wang, Zhao, Yu, Du, Zhang, Sun and Song. This is an open-access article distributed under the terms of the Creative Commons Attribution License (CC BY). The use, distribution or reproduction in other forums is permitted, provided the original author(s) and the copyright owner(s) are credited and that the original publication in this journal is cited, in accordance with accepted academic practice. No use, distribution or reproduction is permitted which does not comply with these terms.
*Correspondence: Wenlu Song, c29uZ3dlbmx1QGpueHkuZWR1LmNu
†These authors have contributed equally to this work