- College of Science and Technology, Hebei Agricultural University, Cangzhou, China
In recent years, the unique and diverse physicochemical properties of nanoparticles have brought about their wide use in many fields; however, it is necessary to better understand the possible human health risks caused by their release in the environment. Although the adverse health effects of nanoparticles have been proposed and are still being clarified, their effects on lung health have not been fully studied. In this review, we focus on the latest research progress on the pulmonary toxic effects of nanoparticles, and we summarized their disturbance of the pulmonary inflammatory response. First, the activation of lung inflammation by nanoparticles was reviewed. Second, we discussed how further exposure to nanoparticles aggravated the ongoing lung inflammation. Third, we summarized the inhibition of the ongoing lung inflammation by nanoparticles loaded with anti-inflammatory drugs. Forth, we introduced how the physicochemical properties of nanoparticles affect the related pulmonary inflammatory disturbance. Finally, we discussed the main gaps in current research and the challenges and countermeasures in future research.
1 Introduction
Owing to their unique physicochemical properties, nanoparticles are widely used in many fields, such as catalysis, optoelectronic devices, energy storage, coatings, environmental protection and biomedicine (Zhou et al., 2020; Ettlinger et al., 2022; Kankala et al., 2022). According to statistics, 5,036 nanoparticls-based products have been officially put on the market by 2023. With the increase in the scale of production and use, nanoparticle-based products will inevitably enter the environment in the process of production, transportation, use, and emission, and they will diffuse into the environment through air, water, food and other media (Iglesias, 2022; Wang et al., 2023). This will increase the chances of nanoparticles entering the human body through respiration, skin contact, food intake and other ways. In addition, nanoparticles used in the field of biomedicine can directly enter the blood circulation system and reach all tissues and organs (Chu et al., 2022; Mundekkad and Cho, 2022). Therefore, the health effects related to human exposure to nanoparticles need to be evaluated urgently.
Lung inflammation is the direct response of the respiratory system to external stimuli. An imbalance in the lung inflammatory response leads to the occurrence of many major lung diseases, such as pulmonary hypertension (Rong et al., 2022), acute lung injury (Zhai et al., 2022), pneumoconiosis (Li et al., 2017), chronic obstructive pulmonary disease (Kim et al., 2023), and lung cancer (Ahmad et al., 2022). 3-Bromopyruvic acid, fucoidan oligosaccharide and astragaloside IV alleviate monocrotaline-induced pulmonary hypertension in rats through an anti-inflammatory pathway (Liu et al., 2020; Jin et al., 2021); The downregulation of miR-let-7e suppresses lung inflammation by targeting the SCOS1/NFκB signaling pathway, thereby reducing acute lung injury induced by lipopolysaccharide (LPS) in mice (Li W. et al., 2021). Ghrelin protects rats from pulmonary vascular dysfunction caused by acute lung injury by inhibiting pulmonary inflammatory response (Li G. et al., 2021); LPS promotes pulmonary fibrosis in silicosis by aggravating the inflammatory response of alveolar macrophages (Tan et al., 2020). The overexpression of myotubularin-related protein 14 (MTMR14) inhibits lung inflammation induced by cigarette smoke extract and improves mitochondrial function. This may be one of the mechanisms by which MTMR14 alleviates chronic obstructive pulmonary disease (Gu et al., 2022). According to the World Health Organization, the death rate due to pulmonary inflammatory response disorders accounts for 75% of the total death rate of acute respiratory infections, which poses a huge threat to health and life of people. To study the effects of high-risk exposure factors on lung inflammation and the related molecular mechanism is of great significance for safeguarding human health and life.
Lung is one of the main target organs of nanoparticles (Montigaud et al., 2020; Scolari et al., 2021). The disturbance of the pulmonary inflammatory response is an important indicator of the pulmonary toxicity of nanoparticles (Guo et al., 2022). Prior studies have shown that exposure to nanoparticles, such as silica nanoparticles (Wang M. X. et al., 2020), titanium dioxide nanoparticles (Ma et al., 2019; Sagawa et al., 2021), and zinc oxide nanoparticles (Guo et al., 2022), interferes with pulmonary inflammatory response in mice, which affects the normal function of the lungs. In this review, we briefly summarize the impact of nanoparticles on lung inflammation from the following four aspects: how nanoparticles activate lung inflammation; how nanoparticles aggravate lung inflammation; how nanoparticles inhibit lung inflammation; and how the physicochemical properties of nanoparticles affect the related lung inflammation disturbance. The challenges and prospects of the disturbance of lung inflammation caused by nanoparticles are also discussed.
2 Induction of lung inflammation by nanoparticles
Nanoparticle-induced lung inflammation has been carried out in vivo and in vitro (Table 1). In vivo studies have shown that carbon-based nanoparticles, metal-based nanoparticles, oxide-based nanoparticles, and sulfide-based nanoparticles cause pulmonary inflammatory response in mice or rats after respiratory exposure. First, exposure of C75Bl/6 mice to multi-walled carbon nanotubes (MWCNTs) by intratracheal instillation significantly increases the number of pulmonary macrophages and induces a pulmonary influx of neutrophils and histological analysis has shown the presence of MWCNTs in alveolar macrophages (Luyts et al., 2018). Mesoporous carbon nanoparticles (MCNs) induce biophysical inhibition of the natural pulmonary surfactant, which increases the surface tension of the alveolar, thereby leading to severe alveolar collapse in mice. MCNs also activate macrophages and stimulate lung inflammation associated with lung fibrosis in mice after inhalation exposure (Chen et al., 2017). Similarly, carbon dots (CDs) induce acute lung inflammation and airway macrophages have been identified as target cells of CDs (Weiss et al., 2021). The potential of carbon black nanoparticles (CB nanoparticles) and single-walled carbon nanotubes (SWCNTs) to induce lung inflammation has also been studied in apolipoprotein E-knockout mice (ApoE −/−) and in C57BL/6 J mice. Both SWCNTs and CB nanoparticles significantly increase the expression of IL-6, MIP-2 and MCP-1 mRNA in the lung tissue. They also greatly increases the proportion of neutrophils in bronchoalveolar lavage fluid (BALF) (Jacobsen et al., 2009). Intratracheal administration of SWCNTs remarkably increases the levels of TNF-α, IL-1β, and IL-6 in BALF via the activation of the PI3K/AKT/NF-κB signaling pathway (Zhang et al., 2022). A single exposure to graphene oxide (GO) induces lung inflammation by causing DNA damage in the lung alveolar epithelium of C57Bl/6 mice (de Luna et al., 2022). Second, oropharyngeal aspiration of aggregated-MoS2 nanosheets induces the neutrophilic exudation into BALF and increases proinflammatory cytokines in C57Bl/6 mice (Wang et al., 2015). After inhalation exposure, PbS nanoparticles induce lung inflammation by causing oxidative stress, thus damaging the blood capillary endothelial cells and alveolar epithelial cells in male Sprague–Dawley rats (Li et al., 2013). Third, following intravenous injection of gold nanoparticles and silver nanoparticles in male Wistar rats, there is an accumulation of gold nanoparticles in the lungs. Histopathological results have shown that infiltrating lymphocytes appear in the lung interstitial tissues, and IL-1α immunostaining is enhanced in the lung tissue, which may be related to the downregulation of miR-327 (Ng et al., 2016). Acute exposure of C57BL/6 mice to Ni nanoparticles elevates the levels of inflammatory factors, IL-6 and CXCL1, along with an increased STAT3 phosphorylation level (You et al., 2020). Intranasal instillation of chitosan-modified Cu nanoparticles also induces lung inflammation in C57BL/6 mice (Worthington et al., 2013). Forth, the widespread use of titanium dioxide nanoparticles (TiO2 nanoparticles) as white pigment causes their unintentional release into the environment, which increases the probability of human exposure through the respiratory system. There are more and more studies about the effect of TiO2 nanoparticles on lung inflammation. Intratracheal exposure to rutile TiO2 nanoparticles resultes in leukocyte migration into alveolar region and significantly increases the secretion of C-C motif ligand (CCL) 3 into BALF. Necrosis inhibitors inhibite the increase of CCL3 secretion in BALF and the increase of leukocytes in BALF. Necrosis of alveolar macrophages that have phagocytosed TiO2 nanoparticles is part of the mechanism of acute lung inflammation induced by TiO2 nanoparticles (Sagawa et al., 2021). The pulmonary inflammatory response to TiO2 nanoparticles shows differences between old and young mice. Compared with old mice, nasal inhalation of TiO2 nanoparticles causes more severe lung inflammation and fibrosis in young mice. Decreased levels of global methylation and hydroxymethylation have been found in young mice, in particular, altered methylation in the promoter of TNF-α and Thy-1 have been proven to play a key role in inflammatory response and fibrosis (Ma et al., 2019). Nrf2, a positive modulator of the cytokines IFN-γ, TNF-α and TGF-β, seems to interfere with lung inflammation caused by TiO2 nanoparticles exposure (Delgado-Buenrostro et al., 2015). Similarly, Nrf2 also plays a negative regulatory role when zinc-oxide nanoparticles (ZnO nanoparticles) cause the pulmonary inflammatory response (Guo et al., 2022; Sehsah et al., 2022). In both Nrf2 −/− mice and wild-type mice, the exposure to ZnO nanoparticles increases the number of total cells, lymphocytes, macrophages, and eosinophils in BALF in a dose-dependent manner, but the magnitude of the increase is significantly higher in Nrf2 −/− mice than in wild-type mice (Sehsah et al., 2019). Silica nanoparticles (SiO2 nanoparticles) (Park et al., 2021), nickel-oxide nanoparticles (NiO nanoparticles) (Nishi et al., 2020; Jeong et al., 2022), cobalt-oxide nanoparticles (CoO nanoparticles) (Jeong et al., 2015), and cerium-dioxide nanoparticles (CeO2 nanoparticles) (Nemmar et al., 2017) also cause pulmonary inflammatory response in mice or rats. Subchronic intratracheal instillation of Fe2O3 nanoparticles causes the collagen deposition and infiltration of inflammatory cells via the activation of TLR4, TLR2 and downstream myeloid differentiation factor (MyD)88 and NFκB in the lungs of male C57BL/6 mice (Sun et al., 2023). In addition, after exposure through intratracheal instillation, MgO nanoparticles, Cr2O3 nanoparticles, Co3O4 nanoparticles, ZnFe2O4 nanoparticles, NiFe2O4 nanoparticles, and NiZnFe4O8 nanoparticles also cause inflammation in female Wistar rats or female C57BL/6 mice (Cho et al., 2012; Hadrup et al., 2020).
In vitro studies have also proven that nanoparticles induce lung inflammation by activating various cell signaling pathways. TRPM2, IL-1α, NFκB, PKC-α, and EGFR participate in the inflammatory response caused by nanoparticles in lung cells (Peuschel et al., 2012). In BEAS-2B cells, SiO2 nanoparticles cause an increase in ROS production, the activation of TRPM2 channel, and the alteration of intracellular Zn2+ and Ca2+ homeostasis mediated by TRPM2, thereby resulting in lysosome impairment and subsequent blockade of autophagy flux. The abnormal autophagy triggers the production of proinflammatory mediators, leading to lung inflammation (Wang M. X. et al., 2020). Exposure to SiO2 nanoparticles causes the rapid release of IL-1α from the preexisting reserve in alveolar macrophages and stimulates subsequent lung inflammation through the production of IL-1β. Further, the release of IL-1α can be used to predict the induction of acute lung inflammation (Rabolli et al., 2014). Amorphous negatively charged SiO2 nanoparticles induce the production of proinflammatory markers by upregulating NFκB and reducing the activity of MMP in MRC-5 lung fibroblasts (Voicu et al., 2019). A similar mechanism has been found in A549 cells exposed to NiO nanoparticles. NiO nanoparticle-induced proinflammatory cytokines are dependent on the mitogen-activated protein kinases (MAPK) cascade via the activation of the NFκB pathway (Capasso et al., 2014). In A549 cells, CB nanoparticles induce the activation of PKC-α and significantly increase the secretion of inflammatory factors, including COX-2, NO, iNOS and PGE(2). PKC-α inhibitor reduces CB nanoparticle-induced inflammation by downregulation of NO, PGE(2), and ROS, which indicates that PKC-α might participate in CB nanoparticle-induced inflammation (Hsu et al., 2018). In short, there are a large number of receptors or proteins that regulate inflammation on the cell surface or intracellularly, such as TLR4 (Cao et al., 2023), TNFR (McDaniel et al., 2022), P2X7R (Jin et al., 2017), cathepsins (de Mingo et al., 2016), and caspase-1 (Flores et al., 2022). Intracellular inflammation-related signaling pathways are very complex. Thus, the molecular mechanism of lung inflammation induced by nanoparticles is still in its infancy, and there are still numerous unknown signaling proteins to be examined in further research.
Existing studies have proven that the exposure to a variety of traditional nanoparticles causes lung inflammation in mice, rats, and other experimental animals. Due to the increasingly mature synthesis methods of nanoparticles, novel nanoparticles, such as two-dimensional transition metal dichalcogenides (Kirubasankar et al., 2022), black phosphorus nanoflakes (Wang et al., 2021), and metal-organic framework nanoparticles (Chen et al., 2022), have begun to enter the market. However, it is not yet completely clear whether the exposure to these novel nanoparticles can cause lung inflammation. To understand the lung health risks of nanoparticles, it is necessary to fully clarify the disturbance of lung inflammation by nanoparticles and the related molecular mechanisms.
3 Aggravation of lung inflammation by nanoparticles
When different types of lung inflammation occur, the exposure to nanoparticles can further aggravate the inflammatory response. First, after acute lung inflammation caused by LPS in rats or mice, nanoparticle treatment aggravates the existing inflammatory response through various pathways. The glycolipids of Gram-negative bacteria and LPS stimulate host cells through innate immunity. In animal models, intratracheal instillation of LPS can cause lung neutrophil recruitment, lung cytokine expression, and lung injury. When rats are exposed to LPS and then treated with MWCNTs intratracheally for 24 h, it is obvious that LPS alone does not cause lung fibrosis, but the co-treatment of LPS and MWCNTs enhances pulmonary fibrosis. The reason may be that MWCNTs increase the level of platelet-derived growth factor-AA (PDGF-AA), the main mediator of fibrosis. LPS cooperatively enhances the PDGF-AA generation by MWCNTs. In vitro experiments in rat lung macrophages (NR8383 cells) and rat lung fibroblasts have also verified that LPS exposure enhances the mRNA level of PDGF-AA induced by MWCNTs. That is, LPS aggravates MWCNT-induced pulmonary fibrosis by increasing the production of PDGF-AA in macrophages and epithelial cells, and by amplifying PDGF-AA on lung fibroblasts (Cesta et al., 2010). Fourteen-nanometer CB nanoparticles significantly aggravate LPS-induced lung inflammation and pulmonary edema, accompanied by the increased pulmonary expression of macrophage inflammatory protein-1α (MIP-1α), IL-1β, keratinocyte chemoattractant, macrophage chemoattractant protein-1 and MIP-2 (Inoue et al., 2006). Intratracheal instillation of TiO2 nanoparticles (Inoue et al., 2008) nanoparticles and ZnO nanoparticles (Wang P. et al., 2020) into mice further aggravates LPS-induced pulmonary inflammatory response in mice by enhancing the expression of proinflammatory cytokines and chemokines, promoting oxidative stress, and causing DNA damage and cell apoptosis. Second, nanoparticles aggravate the pulmonary inflammatory response caused by ovalbumin or dust mites. TiO2 nanoparticles treatment exacerbates ovalbumin-induced lung inflammation in mice, which may be due to the increased ROS level, enhanced expression of IL-18 and IL-1β, and activation of NLRP3 inflammasome (Kim B.-G. et al., 2017). Similarly, intranasal administration of spherical SiO2 nanoparticles aggravates ovalbumin-induced allergic airway inflammation in mice (Han et al., 2016). Inhalation exposure to MWCNTs aggravates the pulmonary inflammatory response caused by dust mites (Shipkowski et al., 2015; Ihrie et al., 2019). In short, the existing studies have confirmed that the respiratory system exposure to nanoparticles exacerbates the ongoing lung inflammatory response. There are different types of lung inflammation, including LPS- or ovalbumin-induced lung inflammation. The effect of nanoparticles on lung inflammation may be related to the specific type of lung inflammation. There is an urgent need for in-depth research to clarify this issue.
4 Inhibition of lung inflammation by nanoparticles
Recent studies have verified that nanoparticles loaded with special drugs inhibit lung inflammation. Lipid nanoparticles loaded with cepharanthine and coated with macrophage membrane (Lu et al., 2021), dexamethasone-loaded ROS-responsive poly (thioketal) nanoparticles (Zhai et al., 2022), nanoparticles containing dexamethasone modified with hyaluronic acid (Camara et al., 2021), neutrophil membrane-coated, antibiotic agent-loaded nanoparticles (Wang K. Y. et al., 2020), platelet vesicle-decoyed poly (lactic-co-glycolic acid) nanoparticles (Jin et al., 2022), silymarin/curcumin-loaded albumin nanoparticles coated with chitosan (Hanafy and El-Kemary, 2022), shell-crosslinked-knedel-like nanoparticles (Ibricevic et al., 2013), and bilirubin nanoparticles (Kim D. E. et al., 2017) inhibit lung inflammation in mice. Nanoparticles loaded with multiple drugs inhibited lung inflammation via different molecular mechanisms. For example, bixin-loaded polymeric nanoparticle treatment significantly reduces the number of leukocytes and TNF-α level, and it strongly inhibits the increase of MDA and PNK in lung homogenates in BALF of mice exposed to cigarette smoke. The beneficial effect may be attributed to the ability of bixin to clear and neutralize oxidative substances and block the harmful continuous events caused by cigarette smoke (Figueiredo-Junior et al., 2022). Fluorous-tagged peptide nanoparticles significantly ameliorate LPS-induced acute lung inflammation by maintaining the stability of lysosomal membrane and increasing the expression levels of Nrf2, NQO1, and HO-1 (Wang et al., 2022). The pulmonary deposition of CeO nanoparticles alleviates the lung inflammation induced by hypobaric hypoxia by inhibiting the formation of ROS, lipid peroxidation, and glutathione oxidation, and preventing the oxidative modification of proteins (Arya et al., 2013). A new pH-responsive drug-delivery system, TPCA-1-loaded nanoparticles coated with anti-ICAM-1, selectively targets inflammatory endothelium and mouse lungs after intravenous injection, and then the acid environment triggers drug release, thereby reducing lung inflammation and injury (Figure 1) (Zhang et al., 2019). In short, due to the great specific surface area and surface modifiability, the surface of nanoparticles can be modified with targeting molecules and anti-inflammatory drugs, so as to achieve effective inflammatory treatment by targeting specific inflammatory sites, which is a very promising idea for the treatment inflammatory diseases.
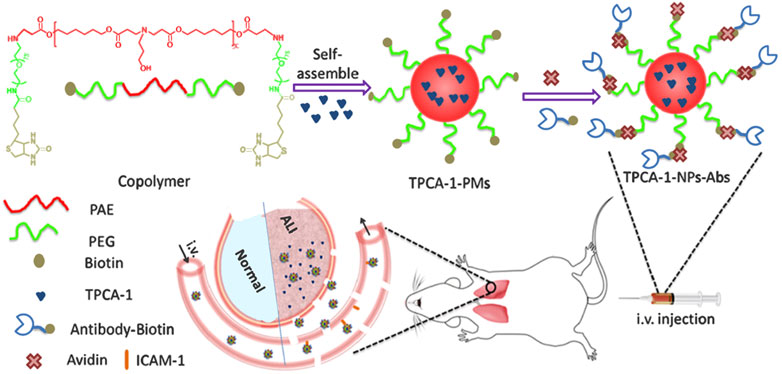
FIGURE 1. Illustration of the development of Ab-decorated nanoparticles targeted to mouse lungs for the treatment of acute lung inflammation (Zhang et al., 2019). Copyright © 2019, American Chemical Society.
Persistent inflammatory conditions can induce DNA damage and mutations, thereby increasing cell division rate damage in lung tissue. Generally, lung cancer usually occurs in the inflammatory tumor microenvironment (Rajasegaran et al., 2023). The occurrence of lung cancer is related to various factors that cause lung inflammation, such as IL-1 β. In vitro and in vivo data have indicated that IL-1β in particular promotes the migration and invasion of lung cancer cells, triggering more aggressive cancer phenotypes (Gelfo et al., 2020; Zhang et al., 2020) (Voronov et al., 2003; Das et al., 2020). Inhibiting the expression of IL-1β has been shown to reduce tumor growth and prevent the shedding of tumor cells from the primary site into circulation (Kaplanov et al., 2019; Tulotta et al., 2019; Zhang and Veeramachaneni, 2022). In a study of 28 advanced non-small-cell lung cancer patients, plasma IL-1β level was increased (McLoed et al., 2016). Another study demonstrated that the inhibition of IL-1β in combination with 5-fluorouracil overcame the resistance mechanisms and enhanced the antitumor function (Bruchard et al., 2013). Some nanoparticles loaded with drugs can effectively inhibit lung inflammation caused by LPS and allergens, thereby reducing the level of inflammatory factor IL-1β. Considering the important role of inflammatory conditions and IL-1β in the occurrence and development of lung cancer, we hope that the inhibition of IL-1β induced by these nanoparticles will open new avenues for cancer treatment by targeting lung tumor inflammation.
According to their composition, nanoparticles can be divided into hard nanoparticles and soft nanoparticles. Metal-based nanoparticles, metal-oxide nanoparticles, and carbon-based nanoparticles belong to hard nanoparticles, while liposome nanoparticles and polymer nanoparticles belong to soft nanoparticles. It is generally believed that soft nanoparticles are less toxic than hard nanoparticles due to their biodegradability. When focusing on the toxic effects of disturbing the pulmonary inflammatory response, hard nanoparticles are more likely to cause and exacerbate lung inflammation. For example, CNTs, MCNs, CDs, CB nanoparticles (Inoue et al., 2006; Jacobsen et al., 2009), TiO2 nanoparticles, ZnO nanoparticles, CoO nanoparticles (Huang et al., 2015; Jeong et al., 2015; Ma et al., 2019), NiO nanoparticles, SiO2 nanoparticles, CeO2 nanoparticles, and gold nanoparticles (Bachand et al., 2012; Han et al., 2016; Nemmar et al., 2017; Nishi et al., 2020) induce or exacerbate lung inflammation in mice, rats, or lung cells. In contrast, soft nanoparticles loaded with drugs, such as lipid nanoparticles (Lu et al., 2021), polymeric nanoparticles (Ibricevic et al., 2013; Zhang et al., 2019; Wang K. Y. et al., 2020; Figueiredo-Junior et al., 2022; Jin et al., 2022; Zhai et al., 2022), protein nanoparticles (Hanafy and El-Kemary, 2022), bilirubin-based nanoparticles (Kim D. E. et al., 2017), and nanoparticles with a shell of hyaluronic acid and a core of dexamethasone (Camara et al., 2021) are more likely to inhibit lung inflammation.
The conclusion that nanoparticles cause or aggravate lung inflammation is mostly drawn from the research of nanoparticles without drug loading, and the conclusion that nanoparticles inhibit lung inflammation is mainly drawn from the research of nanoparticles loaded with special drugs. There are relatively few studies on the inhibition of lung inflammation after exposure to nanoparticles alone, and the specific molecular mechanism remains to be further studied. In order to fully understand the interference of nanoparticles with lung inflammation, it is necessary to clarify the effects and related molecular mechanisms of nanoparticle exposure when lung inflammation has already occurred.
5 Roles of nanoparticles characteristics on the regulation of lung inflammation
The disturbance of lung inflammation caused by nanoparticles is closely related to their physicochemical properties. First, research based on CB nanoparticles, TiO2 nanoparticles, and silica-dioxide nanoparticles has confirmed that the smaller the particle size of nanoparticles, the easier it is to cause or aggravate lung inflammation. When the weight of nanoparticles is equal, the airway exposure to 14-nm CB nanoparticles strongly aggravates LPS-induced pulmonary edema and lung inflammation, while 56-nm nanoparticles do not show obvious effects (Inoue et al., 2006). Next, 20-nm silica nanoparticles, but not 50-nm silica nanoparticles, induce lung inflammation in rats after repeated exposure for 14 days. Compared with the cells treated with 50-nm silica nanoparticles, the structural damage of organelles in the cells treated with 20-nm silica nanoparticles is more obvious, and the increase of mitochondrial membrane potential and mitochondrial calcium accumulation is only observed in 20-nm silica nanoparticle-treated cells. The lung inflammation induced by 20-nm silica nanoparticles may be related to the paraptosis of alveolar macrophages (Park et al., 2021). Three sizes (15, 50, and 100 nm) of TiO2 nanoparticles aggravate LPS-induced lung inflammation and vascular permeability in a size-dependent manner after 24 h of intratracheal instillation in mice. Compared with LPS alone, LPS plus silica nanoparticles, especially those smaller than 50 nm in size, improve the circulatory level of MCP-l, fibrinogen, KC, IL-l p and von Willebrand factor (Inoue et al., 2008). This may be because the smaller size of nanoparticles make it easier for them to enter the lungs. Second, the surface modification of nanoparticles influences many biological effects, such as autophagy, apoptosis, and oxidative stress (Zhou et al., 2022). As an immune response to exogenous substances, lung inflammation is also interfered by the surface modification of nanoparticles. Coating copper-oxide nanoparticles with chitosan reduces their ability to be removed from the lungs, prolongs the exposure time of lung cells and tissues to metal oxides, and produces significant acute lung inflammation (Worthington et al., 2013). This may be attributed to the fact that the surface modification may change the charge, hydrophobicity and steric hindrance of nanoparticles, thereby affecting the cellular uptake, subcellular localization of nanoparticles, and interaction between nanoparticles and cell surface proteins (Sun et al., 2018; Bai et al., 2020). Solubility and thickness also affect the disturbance of lung inflammation caused by nanoparticles (Cho et al., 2012; Wang et al., 2015). Forty hours after oropharyngeal aspiration in C57Bl/6 mice, thick aggregated-MoS2 nanosheets induce robust production of IL-6, MCP-1, and LIX along with the neutrophilic exudation into BALF, whereas thin MoS2 nanosheets do not trigger chemokine or cytokine induction in the lungs. Histopathological changes confirme the formation of focal areas of inflammation around small airways induced by thick aggregated-MoS2 nanosheets, while thin MoS2 nanosheets have little or no effect (Wang et al., 2015). After 24 h of intratracheal instillation, high-solubility CoO nanoparticles produce a dose-dependent eosinophilic influx into the lungs. The inflammatory potential of CoO nanoparticles is comparable to that evaluated after treatment with an identical Co. ion mass of CoCl2, while the medium-solubility Co3O4 nanoparticles do not induce the eosinophilic inflammation. Eosinophilic inflammation produced by CoO nanoparticles might originate from the dissolution of Co. ions inside the cells (Jeong et al., 2015). The physicochemical properties of nanoparticles affect their biological effects. We speculate that the shape, composition and surface protein corona of nanoparticles also influence their disturbance of lung inflammation. Further research is urgently needed to clarify the specific link between these physicochemical properties and lung inflammation.
6 Discussion
This review summarized the activation of lung inflammation caused by nanoparticles, the aggravation of lung inflammation caused by nanoparticles, the inhibition of lung inflammation caused by nanoparticles, and the influence of physicochemical properties of nanoparticles on the disturbance of lung inflammation. Due to unique physicochemical properties and increasingly mature synthesis methods, nanoparticles have widely been used in many fields, thereby increasing the opportunities for human exposure. Once nanoparticles enter the human body, they may interact with the biological system, disturb the steady state of the physiological system, and pose a threat to human health. Therefore, it is necessary to evaluate the biological safety of nanoparticles. As the lungs are an important target organ of nanoparticles, it is significant to evaluate the disturbance of lung inflammation by nanoparticles. The relevant fields are still in the initial stage, and there are many key issues that still need to be studied in depth.
From the perspective of lung inflammation, a large number of studies have focused on how nanoparticles trigger or aggravate lung inflammation. The conclusions are mainly drawn from studies of nanoparticles without drug loading. In contrast, limited studies have found that nanoparticles loaded with anti-inflammatory drugs inhibit the ongoing lung inflammation. Research about the inhibition of lung inflammation due to an individual’s exposure to drug-free nanoparticles is still in its infancy.
Existing studies have shown that the physicochemical properties (size, shape, composition, and surface chemistry) of nanoparticles affect their interaction with biological systems. However, research on the influence of the physicochemical properties of nanoparticles on the disturbance of lung inflammation is still in its infancy. Limited studies have preliminarily found that size, shape, and surface charge may affect the disturbance of lung inflammation caused by nanoparticles. Therefore, it is necessary to systematically study how various physicochemical properties affect the disturbance of lung inflammation caused by nanoparticles and the specific molecular mechanism. The ultimate goal of the lung safety assessment of nanoparticles is to reveal the potential risks of nanoparticles to human lung health. The conclusions obtained in vitro need to be further verified by in vivo experiments. The specific relationship between the physicochemical properties and the disturbance of lung inflammation caused by nanoparticles should be clarified through the systematic study at the body level.
Author contributions
JM designed this review, WJ performed the literature search of the databases, XZ wrote the manuscript, and JM revised the manuscript. All authors contributed to the article and approved the submitted version.
Funding
This work was supported by the Special Scientific Research Fund for Talents Introduced of Hebei Agricultural University (YJ2019030).
Conflict of interest
The authors declare that the research was conducted in the absence of any commercial or financial relationships that could be construed as a potential conflict of interest.
Publisher’s note
All claims expressed in this article are solely those of the authors and do not necessarily represent those of their affiliated organizations, or those of the publisher, the editors and the reviewers. Any product that may be evaluated in this article, or claim that may be made by its manufacturer, is not guaranteed or endorsed by the publisher.
References
Ahmad, S., Manzoor, S., Siddiqui, S., Mariappan, N., Zafar, I., Ahmad, A., et al. (2022). Epigenetic underpinnings of inflammation: Connecting the dots between pulmonary diseases, lung cancer and COVID-19. Semin. Cancer Biol. 83, 384–398. doi:10.1016/j.semcancer.2021.01.003
Almutairi, B., Albahser, G., Almeer, R., Alyami, N. M., Almukhlafi, H., Yaseen, K. N., et al. (2020). Investigation of cytotoxicity apoptotic and inflammatory responses of biosynthesized zinc oxide nanoparticles from ocimum sanctum linn in human skin keratinocyte (hacat) and human lung epithelial (A549) cells. Oxid. Med. Cell. Longev. 2020, 1. doi:10.1155/2020/1835475
Arya, A., Sethy, N. K., Singh, S. K., Das, M., and Bhargava, K. (2013). Cerium oxide nanoparticles protect rodent lungs from hypobaric hypoxia-induced oxidative stress and inflammation. Int. J. Nanomed. 8, 4507–4520. doi:10.2147/ijn.s53032
Bachand, G. D., Allen, A., Bachand, M., Achyuthan, K. E., Seagrave, J. C., and Brozik, S. M. (2012). Cytotoxicity and inflammation in human alveolar epithelial cells following exposure to occupational levels of gold and silver nanoparticles. J. Nanopart. Res. 14, 1212. doi:10.1007/s11051-012-1212-y
Bai, X., Wang, S., Yan, X., Zhou, H., Zhan, J., Liu, S., et al. (2020). Regulation of cell uptake and cytotoxicity by nanoparticle core under the controlled shape, size, and surface chemistries. ACS Nano 14, 289–302. doi:10.1021/acsnano.9b04407
Blum, J. L., Rosenblum, L. K., Grunig, G., Beasley, M. B., Xiong, J. Q., and Zelikoff, J. T. (2014). Short-term inhalation of cadmium oxide nanoparticles alters pulmonary dynamics associated with lung injury, inflammation, and repair in a mouse model. Inhal. Toxicol. 26, 48–58. doi:10.3109/08958378.2013.851746
Bourgois, A., Saurat, D., De Araujo, S., Boyard, A., Guitard, N., Renault, S., et al. (2021). Nose-only inhalations of high-dose alumina nanoparticles/hydrogen chloride gas mixtures induce strong pulmonary pro-inflammatory response: A pilot study. Inhal. Toxicol. 33, 308–324. doi:10.1080/08958378.2021.1996492
Braakhuis, H. M., Gosens, I., Krystek, P., Boere, J. a. F., Cassee, F. R., Fokkens, P. H. B., et al. (2014). Particle size dependent deposition and pulmonary inflammation after short-term inhalation of silver nanoparticles. Part. Fibre Toxicol. 11, 49. doi:10.1186/s12989-014-0049-1
Bruchard, M., Mignot, G., Derangere, V., Chalmin, F., Chevriaux, A., Vegran, F., et al. (2013). Chemotherapy-triggered cathepsin B release in myeloid-derived suppressor cells activates the Nlrp3 inflammasome and promotes tumor growth. Nat. Med. 19, 57–64. doi:10.1038/nm.2999
Camara, C. I., Bertocchi, L., Ricci, C., Bassi, R., Bianchera, A., Cantu, L., et al. (2021). Hyaluronic acid-dexamethasone nanoparticles for local adjunct therapy of lung inflammation. Int. J. Mol. Sci. 22, 10480. doi:10.3390/ijms221910480
Cao, Z. Y., Xing, C. H., Cheng, X. Y., Luo, J. R., Hu, R. M., Cao, H. B., et al. (2023). Luteolin attenuates APEC-induced oxidative stress and inflammation via inhibiting the HMGB1/TLR4/NF-kappa B signal Axis in the ileum of chicks. Animals 13, 83. doi:10.3390/ani13010083
Capasso, L., Camatini, M., and Gualtieri, M. (2014). Nickel oxide nanoparticles induce inflammation and genotoxic effect in lung epithelial cells. Toxicol. Lett. 226, 28–34. doi:10.1016/j.toxlet.2014.01.040
Cesta, M. F., Ryman-Rasmussen, J. P., Wallace, D. G., Masinde, T., Hurlburt, G., Taylor, A. J., et al. (2010). Bacterial lipopolysaccharide enhances PDGF signaling and pulmonary fibrosis in rats exposed to carbon nanotubes. Am. J. Respir. Cell Mol. Biol. 43, 142–151. doi:10.1165/rcmb.2009-0113OC
Chen, Y. L., Su, J. Q., Dong, W. X., Xu, D. X., Cheng, L., Mao, L. K., et al. (2022). Cyclodextrin-based metal-organic framework nanoparticles as superior carriers for curcumin: Study of encapsulation mechanism, solubility, release kinetics, and antioxidative stability. Food Chem. 383, 132605. doi:10.1016/j.foodchem.2022.132605
Chen, Y. N., Yang, Y., Xu, B. L., Wang, S. H., Li, B., Ma, J., et al. (2017). Mesoporous carbon nanomaterials induced pulmonary surfactant inhibition, cytotoxicity, inflammation and lung fibrosis. J. Environ. Sci. 62, 100–114. doi:10.1016/j.jes.2017.08.018
Cho, W. S., Duffin, R., Thielbeer, F., Bradley, M., Megson, I. L., Macnee, W., et al. (2012). Zeta potential and solubility to toxic ions as mechanisms of lung inflammation caused by metal/metal oxide nanoparticles. Toxicol. Sci. 126, 469–477. doi:10.1093/toxsci/kfs006
Chu, Z. Y., Tian, T., Tao, Z. C., Yang, J., Chen, B. J., Chen, H., et al. (2022). Upconversion nanoparticles@AgBiS2 core-shell nanoparticles with cancer-cell-specific cytotoxicity for combined photothermal and photodynamic therapy of cancers. Bioact. Mat. 17, 71–80. doi:10.1016/j.bioactmat.2022.01.010
Das, S., Shapiro, B., Vucic, E. A., Vogt, S., and Bar-Sagi, D. (2020). Tumor cell-derived IL1 beta promotes desmoplasia and immune suppression in pancreatic cancer. Cancer Res. 80, 1088–1101. doi:10.1158/0008-5472.can-19-2080
De Luna, L. V., Loret, T., Fordham, A., Arshad, A., Drummond, M., Dodd, A., et al. (2022). Lung recovery from DNA damage induced by graphene oxide is dependent on size, dose and inflammation profile. Part. Fibre Toxicol. 19, 62. doi:10.1186/s12989-022-00502-w
De Mingo, A., De Gregorio, E., Moles, A., Tarrats, N., Tutusaus, A., Colell, A., et al. (2016). Cysteine cathepsins control hepatic NF-kappa B-dependent inflammation via sirtuin-1 regulation. Cell Death Dis. 7, e2464. doi:10.1038/cddis.2016.368
Delgado-Buenrostro, N. L., Medina-Reyes, E. I., Lastres-Becker, I., Freyre-Fonseca, V., Ji, Z. X., Hernandez-Pando, R., et al. (2015). Nrf2 protects the lung against inflammation induced by titanium dioxide nanoparticles: A positive regulator role of Nrf2 on cytokine release. Environ. Toxicol. 30, 782–792. doi:10.1002/tox.21957
Ettlinger, R., Lachelt, U., Gref, R., Horcajada, P., Lammers, T., Serre, C., et al. (2022). Toxicity of metal-organic framework nanoparticles: From essential analyses to potential applications. Chem. Soc. Rev. 51, 464–484. doi:10.1039/d1cs00918d
Figueiredo-Junior, A. T., Valenca, S. S., Finotelli, P. V., Dos Anjos, F. D. F., De Brito-Gitirana, L., Takiya, C. M., et al. (2022). Treatment with bixin-loaded polymeric nanoparticles prevents cigarette smoke-induced acute lung inflammation and oxidative stress in mice. Antioxidants 11, 1293. doi:10.3390/antiox11071293
Flores, J., Fillion, M. L., and Leblanc, A. C. (2022). Caspase-1 inhibition improves cognition without significantly altering amyloid and inflammation in aged Alzheimer disease mice. Cell Death Dis. 13, 864. doi:10.1038/s41419-022-05290-x
Gelfo, V., Romaniello, D., Mazzeschi, M., Sgarzi, M., Grilli, G., Morselli, A., et al. (2020). Roles of IL-1 in cancer: From tumor progression to resistance to targeted therapies. Int. J. Mol. Sci. 21, 6009. doi:10.3390/ijms21176009
Gu, Y. Y., Chen, J. K., Huang, Q., Zhan, Y., Wang, T., Wu, J. X., et al. (2022). MTMR14 alleviates chronic obstructive pulmonary disease as a regulator in inflammation and emphysema. Oxid. Med. Cell. Longev. 2022, 1–21. doi:10.1155/2022/9300269
Guo, T. Y., Fang, X., Liu, Y. T., Ruan, Y. H., Hu, Y., Wang, X. N., et al. (2022). Acute lung inflammation induced by zinc oxide nanoparticles: Evolution and intervention via NRF2 activator. Food Chem. Toxicol. 162, 112898. doi:10.1016/j.fct.2022.112898
Hadrup, N., Saber, A. T., Kyjovska, Z. O., Jacobsen, N. R., Vippola, M., Sarlin, E., et al. (2020). Pulmonary toxicity of Fe2O3, ZnFe2O4, NiFe2O4 and NiZnFe4O8 nanomaterials: Inflammation and DNA strand breaks. Environ. Toxicol. Pharmacol. 74, 103303. doi:10.1016/j.etap.2019.103303
Han, H., Park, Y. H., Park, H. J., Lee, K., Um, K., Park, J.-W., et al. (2016). Toxic and adjuvant effects of silica nanoparticles on ovalbumin-induced allergic airway inflammation in mice. Respir. Res. 17, 60. doi:10.1186/s12931-016-0376-x
Hanafy, N., and El-Kemary, M. A. (2022). Silymarin/curcumin loaded albumin nanoparticles coated by chitosan as muco-inhalable delivery system observing anti-inflammatory and anti COVID-19 characterizations in oleic acid triggered lung injury and in vitro COVID-19 experiment. Int. J. Biol. Macromol. 198, 101–110. doi:10.1016/j.ijbiomac.2021.12.073
Hsu, H.-T., Tseng, Y.-T., Wong, W.-J., Liu, C.-M., and Lo, Y.-C. (2018). Resveratrol prevents nanoparticles-induced inflammation and oxidative stress via downregulation of PKC-alpha and NADPH oxidase in lung epithelial A549 cells. BMC Complement. Altern. Med. 18, 211. doi:10.1186/s12906-018-2278-6
Huang, K. L., Lee, Y. H., Chen, H. I., Liao, H. S., Chiang, B. L., and Cheng, T. J. (2015). Zinc oxide nanoparticles induce eosinophilic airway inflammation in mice. J. Hazard. Mat. 297, 304–312. doi:10.1016/j.jhazmat.2015.05.023
Ibricevic, A., Guntsen, S. P., Zhang, K., Shrestha, R., Liu, Y. J., Sun, J. Y., et al. (2013). PEGylation of cationic, shell-crosslinked-knedel-like nanoparticles modulates inflammation and enhances cellular uptake in the lung. Nanomed.-Nanotechnol. 9, 912–922. doi:10.1016/j.nano.2013.02.006
Iglesias, M. (2022). Silver nanoparticles: Synthesis, detection, characterization and assessment in environment. Nanomaterials 12, 167. doi:10.3390/nano12010167
Ihrie, M. D., Taylor-Just, A. J., Walker, N. J., Stout, M. D., Gupta, A., Richey, J. S., et al. (2019). Inhalation exposure to multi-walled carbon nanotubes alters the pulmonary allergic response of mice to house dust mite allergen. Inhal. Toxicol. 31, 192–202. doi:10.1080/08958378.2019.1643955
Inoue, K. I., Takano, H., Yanagisawa, R., Hirano, S., Sakurai, M., Shimada, A., et al. (2006). Effects of airway exposure to nanoparticles on lung inflammation induced by bacterial endotoxin in mice. Environ. Health Perspect. 114, 1325–1330. doi:10.1289/ehp.8903
Inoue, K., Takano, H., Ohnuki, M., Yanagisawa, R., Sakurai, M., Shimada, A., et al. (2008). Size effects of nanomaterials on lung inflammation and coagulatory disturbance. Int. J. Immunopathol. Pharmacol. 21, 197–206. doi:10.1177/039463200802100122
Jacobsen, N. R., Moller, P., Jensen, K. A., Vogel, U., Ladefoged, O., Loft, S., et al. (2009). Lung inflammation and genotoxicity following pulmonary exposure to nanoparticles in ApoE(-/-) mice. Part. Fibre Toxicol. 6, 2. doi:10.1186/1743-8977-6-2
Jeong, J., Han, Y., Poland, C. A., and Cho, W.-S. (2015). Response-metrics for acute lung inflammation pattern by cobalt-based nanoparticles. Part. Fibre Toxicol. 12, 13. doi:10.1186/s12989-015-0089-1
Jeong, M.-J., Jeon, S., Yu, H.-S., Cho, W.-S., Lee, S., Kang, D., et al. (2022). Exposure to nickel oxide nanoparticles induces acute and chronic inflammatory responses in rat lungs and perturbs the lung microbiome. Int. J. Env. Res. Public Health 19, 522. doi:10.3390/ijerph19010522
Jin, H. F., Jiao, Y., Guo, L. N., Ma, Y., Zhao, R. J., Li, X. M., et al. (2021). Astragaloside IV blocks monocrotaline-induced pulmonary arterial hypertension by improving inflammation and pulmonary artery remodeling. Int. J. Mol. Med. 47, 595–606. doi:10.3892/ijmm.2020.4813
Jin, H., Luo, R. X., Li, J. N., Zhao, H. X., Ouyang, S. D., Yao, Y. L., et al. (2022). Inhaled platelet vesicle-decoyed biomimetic nanoparticles attenuate inflammatory lung injury. Front. Pharmacol. 13, 1050224. doi:10.3389/fphar.2022.1050224
Jin, R. L., Shen, M. D., Yu, L. D., Wang, X. W., and Lin, X. J. (2017). Adipose-derived stem cells suppress inflammation induced by IL-1 beta through down-regulation of P2X7R mediated by miR-373 in chondrocytes of osteoarthritis. Mol. Cells 40, 222–229. doi:10.14348/molcells.2017.2314
Kankala, R. K., Han, Y. H., Xia, H. Y., Wang, S. B., and Chen, A. Z. (2022). Nanoarchitectured prototypes of mesoporous silica nanoparticles for innovative biomedical applications. J. Nanobiotechnol. 20, 126. doi:10.1186/s12951-022-01315-x
Kaplanov, I., Carmi, Y., Kornetsky, R., Shemesh, A., Shurin, G. V., Shurin, M. R., et al. (2019). Blocking IL-1 beta reverses the immunosuppression in mouse breast cancer and synergizes with anti-PD-1 for tumor abrogation. Proc. Natl. Acad. Sci. U. S. A. 116, 1361–1369. doi:10.1073/pnas.1812266115
Kim, B.-G., Lee, P.-H., Lee, S.-H., Park, M.-K., and Jang, A.-S. (2017a). Effect of TiO2 nanoparticles on inflammasome-mediated airway inflammation and responsiveness. Allergy. Asthma. Immun. 9, 257–264. doi:10.4168/aair.2017.9.3.257
Kim, D. E., Lee, Y., Kim, M., Lee, S., Jon, S., and Lee, S. H. (2017b). Bilirubin nanoparticles ameliorate allergic lung inflammation in a mouse model of asthma. Biomaterials 140, 37–44. doi:10.1016/j.biomaterials.2017.06.014
Kim, M. S., Kim, D. S., Yuk, H. J., Kim, S. H., Yang, W. K., Park, G. D., et al. (2023). Siraitia grosvenorii extract attenuates airway inflammation in a murine model of chronic obstructive pulmonary disease induced by cigarette smoke and lipopolysaccharide. Nutrients 15, 468. doi:10.3390/nu15020468
Kirubasankar, B., Won, Y. S., Adofo, L. A., Choi, S. H., Kim, S. M., and Kim, K. K. (2022). Atomic and structural modifications of two-dimensional transition metal dichalcogenides for various advanced applications. Chem. Sci. 13, 7707–7738. doi:10.1039/d2sc01398c
Li, G., Zhou, C.-L., Xia, W.-F., Zhang, D., and Lin, H.-Q. (2021a). Ghrelin protects lipopolysaccharide-induced acute lung injury rats against pulmonary vascular dysfunction by inhibiting inflammation. Can. Respir. J. 2021, 1–6. doi:10.1155/2021/6643398
Li, J., Liang, C., Zhang, Z. K., Pan, X. H., Peng, S. L., Lee, W. S., et al. (2017). TAK1 inhibition attenuates both inflammation and fibrosis in experimental pneumoconiosis. Cell Discov. 3, 17023. doi:10.1038/celldisc.2017.23
Li, Q. Z., Hu, X. L., Bai, Y. P., Alattar, M., Ma, D., Cao, Y. H., et al. (2013). The oxidative damage and inflammatory response induced by lead sulfide nanoparticles in rat lung. Food Chem. Toxicol. 60, 213–217. doi:10.1016/j.fct.2013.07.046
Li, W., Zhang, W., Liu, J., Han, Y., Jiang, H., Ji, G., et al. (2021b). Down-regulation of miR-let-7e attenuates LPS-induced acute lung injury in mice via inhibiting pulmonary inflammation by targeting SCOS1/NF-kappa B pathway. Biosci. Rep. 41, BSR20201089. doi:10.1042/bsr20201089
Liu, J., Wang, W., Wang, L., Qi, X.-M., Sha, Y.-H., and Yang, T. (2020). 3-Bromopyruvate alleviates the development of monocrotaline-induced rat pulmonary arterial hypertension by decreasing aerobic glycolysis, inducing apoptosis, and suppressing inflammation. Chin. Med. J. 133, 49–60. doi:10.1097/CM9.0000000000000577
Lu, C., Zheng, J., Ding, Y., Meng, Y., Tan, F., Gong, W., et al. (2021). Cepharanthine loaded nanoparticles coated with macrophage membranes for lung inflammation therapy. Drug Deliv. 28, 2582–2593. doi:10.1080/10717544.2021.2009936
Luyts, K., Van Den Broucke, S., Hemmeryckx, B., Poels, K., Scheers, H., Casas, L., et al. (2018). Nanoparticles in the lungs of old mice: Pulmonary inflammation and oxidative stress without procoagulant effects. Sci. Total Environ. 644, 907–915. doi:10.1016/j.scitotenv.2018.06.301
Ma, Y., Guo, Y. S., Ye, H. L., Huang, K. Q., Lv, Z. Q., and Ke, Y. B. (2019). Different effects of titanium dioxide nanoparticles instillation in young and adult mice on DNA methylation related with lung inflammation and fibrosis. Ecotoxicol. Environ. Saf. 176, 1–10. doi:10.1016/j.ecoenv.2019.03.055
Mcdaniel, M. M., Chawla, A. S., Jain, A., Meibers, H. E., Saha, I., Gao, Y. J., et al. (2022). Effector memory CD4(+) T cells induce damaging innate inflammation and autoimmune pathology by engaging CD40 and TNFR on myeloid cells. Sci. Immunol. 7, eabk0182. doi:10.1126/sciimmunol.abk0182
Mcloed, A. G., Sherrill, T. P., Cheng, D. S., Han, W., Saxon, J. A., Gleaves, L. A., et al. (2016). Neutrophil-derived IL-1 beta impairs the efficacy of NF-kappa B inhibitors against lung cancer. Cell Rep. 16, 120–132. doi:10.1016/j.celrep.2016.05.085
Montigaud, Y., Pourchez, J., Leclerc, L., Tillement, O., Clotagatide, A., Bal, C., et al. (2020). <p>Nebulised gadolinium-based nanoparticles for a multimodal approach: Quantitative and qualitative lung distribution using magnetic resonance and scintigraphy imaging in isolated ventilated porcine lungs</p>. Int. J. Nanomed. 15, 7251–7262. doi:10.2147/ijn.s260640
Mundekkad, D., and Cho, W. L. C. (2022). Nanoparticles in clinical translation for cancer therapy. Int. J. Mol. Sci. 23, 1685. doi:10.3390/ijms23031685
Nemmar, A., Yuvaraju, P., Beegam, S., Fahim, M. A., and Ali, B. H. (2017). Cerium oxide nanoparticles in lung acutely induce oxidative stress, inflammation, and DNA damage in various organs of mice. Oxid. Med. Cell. Longev. 2017, 1–12. doi:10.1155/2017/9639035
Ng, C. T., Li, J. J., Balasubramanian, S. K., You, F., Yung, L. Y. L., and Bay, B. H. (2016). Inflammatory changes in lung tissues associated with altered inflammation-related MicroRNA expression after intravenous administration of gold nanoparticles in vivo. ACS Biomater. Sci. Eng. 2, 1959–1967. doi:10.1021/acsbiomaterials.6b00358
Nishi, K., Kadoya, C., Ogami, A., Oyabu, T., Morimoto, Y., Ueno, S., et al. (2020). Changes over time in pulmonary inflammatory response in rat lungs after intratracheal instillation of nickel oxide nanoparticles. J. Occup. Health 62, e12162. doi:10.1002/1348-9585.12162
Park, E. J., Kang, M. S., Jin, S. W., Lee, T. G., Lee, G. H., Kim, D. W., et al. (2021). Multiple pathways of alveolar macrophage death contribute to pulmonary inflammation induced by silica nanoparticles. Nanotoxicology 15, 1087–1101. doi:10.1080/17435390.2021.1969461
Peuschel, H., Sydlik, U., Grether-Beck, S., Felsner, I., Stockmann, D., Jakob, S., et al. (2012). Carbon nanoparticles induce ceramide- and lipid raft-dependent signalling in lung epithelial cells: A target for a preventive strategy against environmentally-induced lung inflammation. Part. Fibre Toxicol. 9, 48. doi:10.1186/1743-8977-9-48
Rabolli, V., Badissi, A. A., Devosse, R., Uwambayinema, F., Yakoub, Y., Palmai-Pallag, M., et al. (2014). The alarmin IL-1 alpha is a master cytokine in acute lung inflammation induced by silica micro- and nanoparticles. Part. Fibre Toxicol. 11, 69. doi:10.1186/s12989-014-0069-x
Rajasegaran, T., How, C. W., Saud, A., Ali, A., and Lim, J. C. W. (2023). Targeting inflammation in non-small cell lung cancer through drug repurposing. Pharmaceuticals 16, 451. doi:10.3390/ph16030451
Rong, W. W., Liu, C. C., Li, X. M., Wan, N. F., Wei, L. J., Zhu, W. T., et al. (2022). Caspase-8 promotes pulmonary hypertension by activating macrophage-associated inflammation and IL-1 beta (interleukin 1 beta) production. Arterioscler. Thromb. Vasc. Biol. 42, 613–631. doi:10.1161/atvbaha.121.317168
Sagawa, T., Honda, A., Ishikawa, R., Miyasaka, N., Nagao, M., Akaji, S., et al. (2021). Role of necroptosis of alveolar macrophages in acute lung inflammation of mice exposed to titanium dioxide nanoparticles. Nanotoxicology 15, 1312–1330. doi:10.1080/17435390.2021.2022231
Scolari, I. R., Volpini, X., Fanani, M. L., De La Cruz-Thea, B., Natali, L., Musri, M. M., et al. (2021). Exploring the toxicity, lung distribution, and cellular uptake of rifampicin and ascorbic acid-loaded alginate nanoparticles as therapeutic treatment of lung intracellular infections. Mol. Pharm. 18, 807–821. doi:10.1021/acs.molpharmaceut.0c00692
Sehsah, R., Wu, W. T., Ichihara, S., Hashimoto, N., Hasegawa, Y., Zong, C., et al. (2019). Role of Nrf2 in inflammatory response in lung of mice exposed to zinc oxide nanoparticles. Part. Fibre Toxicol. 16, 47. doi:10.1186/s12989-019-0328-y
Sehsah, R., Wu, W. T., Ichihara, S., Hashimoto, N., Zong, C., Yamazaki, K., et al. (2022). Protective role of Nrf2 in zinc oxide nanoparticles-induced lung inflammation in female mice and sexual dimorphism in susceptibility. Toxicol. Lett. 370, 24–34. doi:10.1016/j.toxlet.2022.09.004
Shipkowski, K. A., Taylor, A. J., Thompson, E. A., Glista-Baker, E. E., Sayers, B. C., Messenger, Z. J., et al. (2015). An allergic lung microenvironment suppresses carbon nanotube-induced inflammasome activation via STAT6-dependent inhibition of caspase-1. PLoS One 10, e0128888. doi:10.1371/journal.pone.0128888
Sun, H. N., Liu, Y., Bai, X., Zhou, X. F., Zhou, H. Y., Liu, S. J., et al. (2018). Induction of oxidative stress and sensitization of cancer cells to paclitaxel by gold nanoparticles with different charge densities and hydrophobicities. J. Mat. Chem. B 6, 1633–1639. doi:10.1039/c7tb03153j
Sun, Y., Chen, Y. W., Wang, J. W., Yuan, W. K., Xue, R., Li, C., et al. (2023). Intratracheally administered iron oxide nanoparticles induced murine lung inflammation depending on T cells and B cells. Food Chem. Toxicol. 175, 113735. doi:10.1016/j.fct.2023.113735
Tan, S., Yang, S., Chen, M., Wang, Y., Zhu, L., Sun, Z., et al. (2020). Lipopolysaccharides promote pulmonary fibrosis in silicosis through the aggravation of apoptosis and inflammation in alveolar macrophages. Open Life Sci. 15, 598–605. doi:10.1515/biol-2020-0061
Tulotta, C., Lefley, D. V., Freeman, K., Gregory, W. M., Hanby, A. M., Heath, P. R., et al. (2019). Endogenous production of IL1B by breast cancer cells drives metastasis and colonization of the bone microenvironment. Clin. Cancer Res. 25, 2769–2782. doi:10.1158/1078-0432.ccr-18-2202
Voicu, S. N., Balas, M., Stan, M. S., Trica, B., Serban, A. I., Stanca, L., et al. (2019). Amorphous silica nanoparticles obtained by laser ablation induce inflammatory response in human lung fibroblasts. Materials 12, 1026. doi:10.3390/ma12071026
Voronov, E., Shouval, D. S., Krelin, Y., Cagnano, E., Benharroch, D., Iwakura, Y., et al. (2003). IL-1 is required for tumor invasiveness and angiogenesis. Proc. Natl. Acad. Sci. U. S. A. 100, 2645–2650. doi:10.1073/pnas.0437939100
Wang, K., Rong, G., Gao, Y., Wang, M., Sun, J., Sun, H., et al. (2022). Fluorous-tagged peptide nanoparticles ameliorate acute lung injury via lysosomal stabilization and inflammation inhibition in pulmonary macrophages. Small 18, 2203432. doi:10.1002/smll.202203432
Wang, K. Y., Lei, Y. T., Xia, D. L., Xu, P. P., Zhu, T., Jiang, Z. Y., et al. (2020a). Neutrophil membranes coated, antibiotic agent loaded nanoparticles targeting to the lung inflammation. Colloid Surf. B-Biointerfaces 188, 110755. doi:10.1016/j.colsurfb.2019.110755
Wang, M. X., Li, J., Dong, S. N., Cai, X. B., Simaiti, A., Yang, X., et al. (2020b). Silica nanoparticles induce lung inflammation in mice via ROS/PARP/TRPM2 signaling-mediated lysosome impairment and autophagy dysfunction. Part. Fibre Toxicol. 17, 23. doi:10.1186/s12989-020-00353-3
Wang, P., Zhang, L., Liao, Y., Du, J., Xu, M., Zhao, W., et al. (2020c). Effect of intratracheal instillation of ZnO nanoparticles on acute lung inflammation induced by lipopolysaccharides in mice. Toxicol. Sci. 173, 373–386. doi:10.1093/toxsci/kfz234
Wang, X., Mansukhani, N. D., Guiney, L. M., Ji, Z., Chang, C. H., Wang, M., et al. (2015). Differences in the toxicological potential of 2D versus aggregated molybdenum disulfide in the lung. Small 11, 5079–5087. doi:10.1002/smll.201500906
Wang, Y. H., Shi, H. H., Zhang, L. N., Ge, S. G., Xu, M. L., Wang, X., et al. (2021). Two-dimensional black phosphorus nanoflakes: A coreactant-free electrochemiluminescence luminophors for selective Pb2+ detection based on resonance energy transfer. J. Hazard. Mat. 403, 123601. doi:10.1016/j.jhazmat.2020.123601
Wang, Y., Wusigale, , and Luo, Y. C. (2023). Colloidal nanoparticles prepared from zein and casein: Interactions, characterizations and emerging food applications. Food Sci. Hum. Wellness 12, 337–350. doi:10.1016/j.fshw.2022.07.036
Weiss, M., Fan, J. H., Claudel, M., Lebeau, L., Pons, F., and Ronzani, C. (2021). Combined in vitro and in vivo approaches to propose a putative adverse outcome pathway for acute lung inflammation induced by nanoparticles: A study on carbon dots. Nanomaterials 11, 180. doi:10.3390/nano11010180
Worthington, K. L. S., Adamcakova-Dodd, A., Wongrakpanich, A., Mudunkotuwa, I. A., Mapuskar, K. A., Joshi, V. B., et al. (2013). Chitosan coating of copper nanoparticles reduces in vitro toxicity and increases inflammation in the lung. Nanotechnology 24, 395101. doi:10.1088/0957-4484/24/39/395101
You, D. J., Lee, H. Y., Taylor-Just, A. J., Linder, K. E., and Bonner, J. C. (2020). Sex differences in the acute and subchronic lung inflammatory responses of mice to nickel nanoparticles. Nanotoxicology 14, 1058–1081. doi:10.1080/17435390.2020.1808105
Zhai, Z., Ouyang, W., Yao, Y., Zhang, Y., Zhang, H., Xu, F., et al. (2022). Dexamethasone-loaded ROS-responsive poly(thioketal) nanoparticles suppress inflammation and oxidative stress of acute lung injury. Bioact. Mat. 14, 430–442. doi:10.1016/j.bioactmat.2022.01.047
Zhang, C. Y., Lin, W., Gao, J., Shi, X., Davaritouchaee, M., Nielsen, A. E., et al. (2019). pH-responsive nanoparticles targeted to lungs for improved therapy of acute lung inflammation/injury. ACS Appl. Mat. Inter. 11, 16380–16390. doi:10.1021/acsami.9b04051
Zhang, J., and Veeramachaneni, N. (2022). Targeting interleukin-1 beta and inflammation in lung cancer. Biomark. Res. 10, 5. doi:10.1186/s40364-021-00341-5
Zhang, W. Z., Borcherding, N., and Kolb, R. (2020). “IL-1 signaling in tumor microenvironment,” in Tumor microenvironment: The role of interleukins, Pt A. Editor A. Birbrair (Cham: Springer International Publishing Ag), 1–23.
Zhang, X. L., Li, B., Zhang, X., Zhu, J. J., Xie, Y. F., Shen, T., et al. (2022). 18β-Glycyrrhetinic acid monoglucuronide (GAMG) alleviates single-walled carbon nanotubes (SWCNT)-induced lung inflammation and fibrosis in mice through PI3K/AKT/NFκB signaling pathway. Ecotoxicol. Environ. Saf. 242, 113858. doi:10.1016/j.ecoenv.2022.113858
Zhou, X. F., Jin, W. T., Sun, H. N., Li, C. J., and Jia, J. B. (2022). Perturbation of autophagy: An intrinsic toxicity mechanism of nanoparticles. Sci. Total Environ. 823, 153629. doi:10.1016/j.scitotenv.2022.153629
Keywords: nanoparticles, lung inflammation, mechanisms, physicochemical properties, biosafety evaluation
Citation: Zhou X, Jin W and Ma J (2023) Lung inflammation perturbation by engineered nanoparticles. Front. Bioeng. Biotechnol. 11:1199230. doi: 10.3389/fbioe.2023.1199230
Received: 03 April 2023; Accepted: 09 May 2023;
Published: 25 May 2023.
Edited by:
Hongyu Zhou, Guangzhou University, ChinaCopyright © 2023 Zhou, Jin and Ma. This is an open-access article distributed under the terms of the Creative Commons Attribution License (CC BY). The use, distribution or reproduction in other forums is permitted, provided the original author(s) and the copyright owner(s) are credited and that the original publication in this journal is cited, in accordance with accepted academic practice. No use, distribution or reproduction is permitted which does not comply with these terms.
*Correspondence: Jingjun Ma, aGViYXVsZ0AxNjMuY29t