Corrigendum: The mycoremediation potential of the armillarioids: a comparative genomics analysis
- 1Functional Genomics and Bioinformatics Group, Institute of Forest and Natural Resource Management, Faculty of Forestry, University of Sopron, Sopron, Hungary
- 2Department of Microbiology, Faculty of Science and Informatics, University of Szeged, Szeged, Hungary
- 3Fibre and Nanotechnology Program, Faculty of Wood Engineering and Creative Industries, University of Sopron, Sopron, Hungary
Genes involved in mycoremediation were identified by comparative genomics analysis in 10 armillarioid species and selected groups of white-rot Basidiomycota (14) and soft-rot Ascomycota (12) species to confine the distinctive bioremediation capabilities of the armillarioids. The genomes were explored using phylogenetic principal component analysis (pPCA), searching for genes already documented in a biocatalysis/biodegradation database. The results underlined a distinct, increased potential of aromatics-degrading genes/enzymes in armillarioids, with particular emphasis on a high copy number and diverse spectrum of benzoate 4-monooxygenase [EC:1.14.14.92] homologs. In addition, other enzymes involved in the degradation of various monocyclic aromatics were more abundant in the armillarioids than in the other white-rot basidiomycetes, and enzymes involved in the degradation of polycyclic aromatic hydrocarbons (PAHs) were more prevailing in armillarioids and other white-rot species than in soft-rot Ascomycetes. Transcriptome profiling of A. ostoyae and A. borealis isolates confirmed that several genes involved in the degradation of benzoates and other monocyclic aromatics were distinctively expressed in the wood-invading fungal mycelia. Data were consistent with armillarioid species offering a more powerful potential in degrading aromatics. Our results provide a reliable, practical solution for screening the likely fungal candidates for their full biodegradation potential, applicability, and possible specialization based on their genomics data.
1 Introduction
Polycyclic aromatic hydrocarbons (PAHs), chemical dyes, plastics, heavy metals, and pharmaceutical waste, all of which are distinct chemical wastes, pose a significant threat to all life forms on Earth and therefore require the development of environmentally friendly, cost-effective, and efficient solutions to combat them (Barh et al., 2019). Even though strides have been made to combat these issues using traditional chemical remediation methods, namely, precipitation, reverse osmosis, and reduction, they have often proved inefficient and too costly (Kapahi and Sachdeva, 2017). However, various bioremediation procedures like myco- and phytoremediation, biostimulation, bioaugmentation, and composting have emerged as potential alternatives to chemical-based interventions by offering greater flexibility and cost-effectiveness (Taiwo, 2011; Tyagi et al., 2011; Rhodes, 2014; Herrero and Stuckey, 2015; Ali et al., 2017; Ansari et al., 2020; Yadav et al., 2021).
Mycoremediation, as one of these potential alternatives, represents the degradation of pollutants in the soil or water with the aid of fungi and their enzymes (Barr and Aust, 1994). The two main activities of the mycoremediation processes are biodegradation and biosorption (Kulshreshtha et al., 2014). In biodegradation, the fungus uses its enzymes to break down the pollutants into less complex and non- or less-toxic products. Biosorption is the process by which fungi become tolerant to specific toxic contaminants, e.g., heavy metals, accumulate them, and then remove such impurities from the soil by continuous sorption. Finally, bioconversion or biotransformation refers to the utilization of organic waste by fungi to generate energy and support their growth.
Lignin-degrading white-rot fungi from Basidiomycota, including Phanerochaete chrysosporium, Pleurotus ostreatus, Irpex lacteus, and Schizopora spp., and also certain Ascomycota species like Botryosphaeria spp. and Aspergillus niger, utilize enzymes, such as laccases, cytochrome p450 monooxygenases, FAD monooxygenases, cutinases, peroxidases, hydrolases, and antioxidants to cope with toxic pollutants like heavy metals, PAHs, and fuel oils (Akhtar and Mannan, 2020). Due to their lignin-degrading enzymes including lignin peroxidases (LiP), manganese peroxidases (MnP), and laccases (Lac), white-rot fungi primarily have an extracellularly exposed oxidative potential which can target both phenolic and non-phenolic substances in their environment. LiPs act on non-phenolic compounds, whereas MnPs and Lacs act on phenolic ones (McMullan et al., 2001).
Recently, the mechanisms of lignin degradation by peroxidases, laccases, and glutathione-dependent β-etherase enzymes were comprehensively studied by Cajnko et al. (2021). Peroxidases, known as monomeric heme-containing enzymes, depolymerize lignin through a three-step oxidation process, whereas Lacs–which are versatile low substrate specificity multicopper oxidases–catalyze the oxidation of various aromatic substrates using copper ions. A further emerging alternative lignin depolymerization technique is based on the β-etherase system, which selectively catalyzes the reductive cleavage of lignin’s β-O-4 aryl-ether bonds. Concerning the complexity of degrading lignin and PAHs, Cajnko et al. (2021) also disclosed the importance of controlling the simultaneous depolymerization and polymerization trends of aromatics during lignin degradation and that the competition between depolymerization and polymerization could be affected by several variables, including pH, temperature, enzyme/substrate ratio, enzyme concentration, and incubation time. Most importantly, the efficiency of the depolymerization process was also influenced by the kinetic parameters of various enzymes, and beyond that, it was also observed that the same type of enzyme from a different fungal source, or another isoform of the same enzyme from the same source, would have significantly altered affinities for the same substrates (Patel et al., 2014; Surendran et al., 2018).
When considering a fungal resource in a bioremediation intervention, multilevel tasks must be evaluated and fulfilled, starting from selecting the appropriate fungal isolates and then moving toward optimizing the most suitable technological solutions and engineering conditions.
Regarding the practical biotechnological implementations when employing white-rot fungi, Babič et al. (2012) used response surface methodology (RSM) to demonstrate the activity and productivity of the ligninolytic MnP and Lac enzymes produced by the white rot fungus Ceriporiopsis subvermispora in submerged cultures. They observed that increasing Lac activities required a two-step approach, including liquid media optimization and appropriate immobilization support, the latter benefitting from the innate ability of filamentous fungi to adhere to surfaces and boost Lac activities.
At the level of bioengeneering, Kogej et al. (2010) studied the lead (Pb) biosorption potential of Rhizopus nigricans using an experimental packed bed reactor and modeled the biosorption process mathematically. The model was numerically solved and found to be in good agreement with the experimental data. A parameter sensitivity study revealed that the model was sensitive to biomass properties and various operating conditions, including liquid flow rate, and initial Pb concentration.
Glenn and Gold (1983) first observed the involvement of white-rot species in the breakdown of aromatic dyes and have since triggered a flurry of research. Recently, Yesilada et al. (2018) and Rajhans et al. (2021) have reviewed these topics comprehensively. Fungal oxidation affects all dyes, but there are significant differences between fungal species regarding their catalytic performance and dye oxidation potential. On the other hand, the biodegradation chemical pathways fungi use are also inconsistent in treating certain dyes assuming rather structure-dependent degradation mechanisms (Alam et al., 2023; Gul et al., 2023). However, so far, no obvious link has been found between the structure of a dye and its biodegradability by fungi (Fu and Viraraghavan, 2001).
When nutrient resources (mainly C, N, and S) become scarce, fungi degrade aromatic structures as a secondary metabolic event (Rabinovich et al., 2004). Earlier, researchers used biochemical assays to study the impact of enzymes on various pollutants in the presence of fungi. Current studies focus on using an in vitro fungal culture technique to investigate the mycoremediation potential of a limited number of fungal species isolated from the contaminated sites or previously reported to be involved in specific mycoremediation activities (Lee et al., 2014; Tkavc et al., 2018). Although such an approach is scientifically sound, there is a gap as the fungal species chosen for the experiments may not be the most effective in the bioremediation of the specific pollutants (Matsubara et al., 2005).
Lately, with advances in next-generation sequencing, we can better predict and understand the bioremediation potential of a fungus by using a comparative genomics approach. Genome-level comparisons can reveal significant changes in metabolic pathways and project the physiological capacity of fungi for mycoremediation (Dao et al., 2019; Park and Choi, 2020). This approach has been substantiated by Park et al. (2019), who used comparative genomics and transcriptomics analysis to study the PAH degradation potential of the white rot fungus Dentipellis sp. KUC8613, which was previously reported by Lee et al. (2014). In their study, they identified the enzymes that were used for degrading PAHs. The genes upregulated during the stage of PAH degradation were P450s, epoxide hydrolases, alcohol/aldehyde dehydrogenases, monooxygenases, and dioxygenases. Also, while studying the DDT-resistant Trichoderma hamatum FBL 587, Davolos et al. (2021) observed that the fungi had increased repertoires of xenobiotic-degrading enzymes and specialized DNA-repairing mechanisms. Additionally, Krijger et al. (2014) observed that the ability of a fungus to grow and survive in a complex, challenging environment is directly correlated with the expansion of certain genes/protein families. Similarly, Muszewska et al. (2017) identified that specific families of serine peptidases like S1, S8, S41, S54, S64, and S66 correlated with the fungal lifestyle. These studies indicate that screening for the xenobiotic-degrading enzyme repertoires in different fungal species can help us identify the best fungal candidates and make informed decisions when choosing a specific fungal species for particular pollutants.
Taking all of the above into consideration, we utilized a recently established database of biodegradative enzymes to compare the putative bioremediation-related gene sets of the armillarioid species (Kedves et al., 2021) with those of 14 white-rot Basidiomycota and 12 Ascomycota fungi (Supplementary File 1; Supplementary Figure 1) and by doing so, based on their gene/enzyme profiles, to identify which armillarioid species would have the highest potential for use in the mycoremediation process. Our comparative analysis included Ascomycota species due to recent findings that, besides lignin-degrading activities, an ancestral soft-rot machinery was also shared across Asco- and Basidiomycota (Sahu et al., 2021).
2 Materials and methods
2.1 Genomic datasets
Amino acid sequences of 7 Armillaria species (A. borealis (Armbor1), A. cepistipes B5 (Armcep1), A. gallica 21-2 v1.0 (Armga1), A. luteobubalina HWK02 v1.0 (Armlut1), A. mellea (Armmel1), A. ostoyae C18/9 (Armosto1), A. solidipes 28-4 v1.0 (Armost1)), 2 Desarmillaria species (D. tabescens CCBAS 213 v1.0 (Armtab1), D. ectypa FPL83.16 v1.0 (Armect1)), Guyanagaster necrorhizus MCA 3950 v1.0 (Guyne1), 14 white-rot basidiomycetous fungi (Heterobasidion annosum v2.0 (Hetan2), Polyporus squamosus CCBS 676 v1.0 (Polsqu1), Schizophyllum commune H4-8 v3.0 (Schco3), Bjerkandera adusta v1.0 (Bjead1_1), I. lacteus CCBAS Fr. 238 617/93 v1.0 (Irplac1), Lentinula edodes Le (Bin) 0899 ss11 v1.0 (Lenedo1), Marasmius fiardii PR-910 v1.0 (Marfi1), P. chrysosporium RP-78 v2.2 (Phchr2), Phlebia radiata Fr. (isolate 79, FBCC0043) (Phlrad1), Pleurotus eryngii ATCC 90797 v1.0 (Pleery1), P. ostreatus PC9 v1.0 (PleosPC9_1), Trametes betulina CIRM-BRFM 1801 v1.0 (Trabet1), Trametes versicolor v1.0 (Trave1), Dentipellis sp. KUC8613 (Densp1), and 12 ascomycetes (Trichoderma citrinoviride TUCIM 6016 v4.0 (Trici4), Trichoderma harzianum TR274 v1.0 (Trihar1), Aspergillus flavus NRRL3357 (Aspfl2_3), Aspergillus glaucus v1.0 (Aspgl1), Botryosphaeria dothidea (Botdo1_1), Cladosporium sphaerospermum UM 843 (Clasph1), Cochliobolus lunatus m118 v2.0 (Coclu2), Fusarium oxysporum Fo5176 (FoxFo5176), Galactomyces geotrichum Phaff 72-186 (Galgeo1), Penicillium arizonense CBS 141311 (Penar1), Penicillium chrysogenum Wisconsin 54-1255 (PenchWisc1_1), Purpureocillium lilacinum PLFJ-1 (Purli1), and 1 mucormycete (Mucor circinelloides f. lusitanicus MU402 v1.0 (Muccir1_3)) as an outlier species were downloaded from JGI (https://mycocosm.jgi.doe.gov/mycocosm/home) (Supplementary File 1).
2.2 In vitro stem invasion assays
Gene expression data from recently published in vitro stem invasion assays (Sahu et al., 2023) were further analysed to investigate the expression of mycoremediation-related genes in Armillaria isolates growing on RSTO media (Sipos et al., 2017), which provides an artificially rich nutrient source, and in mycelia invading and growing in plant tissues. In the stem invasion assays, fresh spruce stem segments were placed on the mycelial lawn grown on RSTO media. Gene expression profiles of highly virulent and less virulent isolates of two conifer-specific Armillaria species (A. borealis and A. ostoyae) were examined for the expression of mycoremediation genes. All further experimental details, analysis of the RNA-Seq data, and identification of the gene expression levels of the genes of interest are described in Sahu et al. (2023).
2.3 Data analysis
KofamScan (Aramaki et al., 2020), which employs a hidden Markov model for identifying the KEGG (Kyoto Encyclopedia of Genes and Genomes) orthology of the sequences, was then used for functional annotation of amino acid sequences. KofamScan was run by applying the default parameters, and the significant annotations were selected based on the KofamScan’s adaptive score thresholds and marked with “*.” KEGG enzyme IDs were then used to curate the significant hits using the Biocatalysis/Biodegradation Database (http://eawag-bbd.ethz.ch/) (Gao et al., 2010). The remaining data which could not be curated using the Biocatalysis/Biodegradation Database were manually curated using the KEGG database (Kanehisa et al., 2016). Finally, the enzymes involved in xenobiotic degradation from the KEGG database were considered for comparison using R v4.2 for computation and calculations.
Phylogenetic principal component analysis (pPCA) was performed using the R packages adenophylo v1.1-11 (Jombart et al., 2010). The enzyme copy numbers were counted for each fungus and converted into the n x m matrix to create the pPCA. The phylogenetic tree (Supplementary Figure 1) used in the pPCA analysis was constructed using single copy orthologs. MAFFT v7 aligner (Katoh and Standley, 2013) was applied for sequence alignment, and FastTree v2.1 (Price et al., 2010) was operated to generate the maximum-likelihood phylogenetic trees using the aligned sequences. All images were plotted applying the R package ggplot2 (Wickham, 2016). Multiple sequence alignment of amino acid sequences was performed using MEGAx (Stecher et al., 2020), and motif analysis was completed using the MEME Suite (Bailey et al., 2015). Heme and substrate binding motifs were predicted using the NCBI Conserved Domain Database (Marchler-Bauer et al., 2015). The phylogenetic trees, incorporating structural motifs and/or expression data, were visualized using the Interactive Tree of Life (iTol) tool (Letunic and Bork, 2021).
3 Results
3.1 Comparative analysis of genes potentially involved in biodegradation
To identify genes involved in mycoremediation in various white-rot specific fungi, 10 armillarioids (seven Armillaria, two Desarmillaria, and one Guyanagaster), 14 white-rot Basidiomycota combined with 12 Ascomycota and one Mucoromycota as outlier species were considered for the genome-level comparative analysis (Supplementary File 1). We first performed KofamScan on 536,770 amino acid sequences from the 37 selected fungal genomes and found 159,088 unique fungal proteins that could be significantly associated with KEGG functions. Then, following manual curation, 5,526 protein sequences related to biodegradation could be identified. The gene repertoire patterns of the key enzyme classes were consistent in all fungal genomes except for five basidiomycetous (B. adusta, T. betulina, T. versicolor, P. squamosus, I. lacteus) and two ascomycetous (T. citrinoviride, G. candidum) species, and in M. circinelloides f. lusitanicus, where the total number of transferase enzymes was higher than the total number of hydrolases (Figure 1; Supplementary File 1).
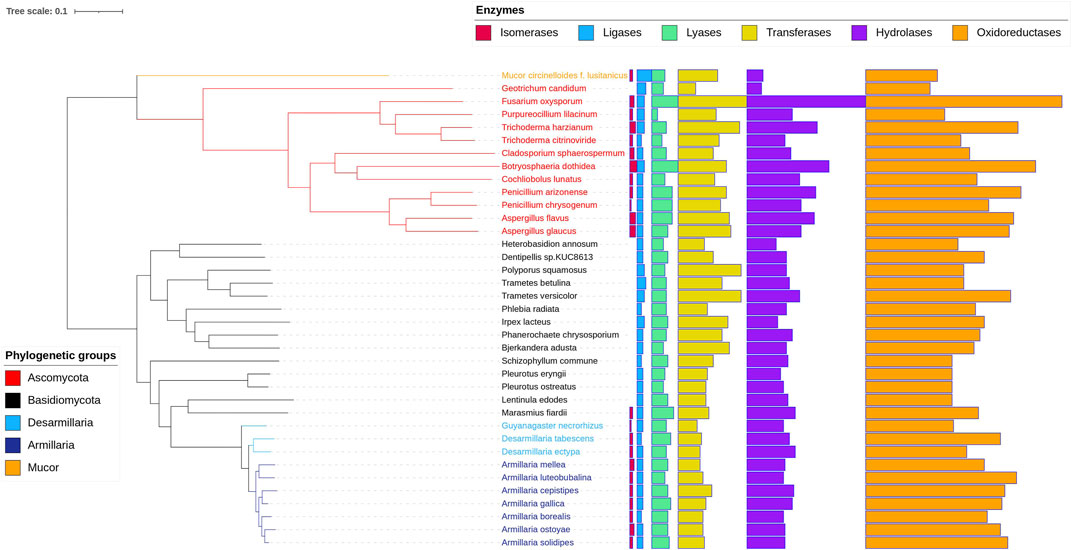
FIGURE 1. Diagrams of the KEGG enzyme classes of the fungi used in the study. The top X-axis lists the enzyme classes, while the Y-axis lists the names of the fungi. The maximum likelihood phylogenetic tree of fungal species was constracted using orthologous proteins.
When assessing the possible specialization within white-rot species, the copy numbers of 92 unique enzymes representing a broad spectrum of biodegradative activities (Figures 2, 3; Supplementary File 1) were analyzed and compared in all selected genomes. pPCA of the prospective biodegradation genes demonstrated that armillarioid species clustered together and separated well from the other white-rot species (Supplementary Figures 2, 3). Notably, based on the individual copy number profiles, the genes coding for benzoate-4-monooxygenase [EC:1.14.14.92] and NADPH2 dehydrogenase [EC:1.6.99.1] (or xenobiotic reductase, "EAWAG-BBD enzyme, enzymeID# e0038″) homologs, both cases with exclusively high copy numbers in the armillarioid genomes appeared to have significantly contributed to their separate clustering (Figure 2).
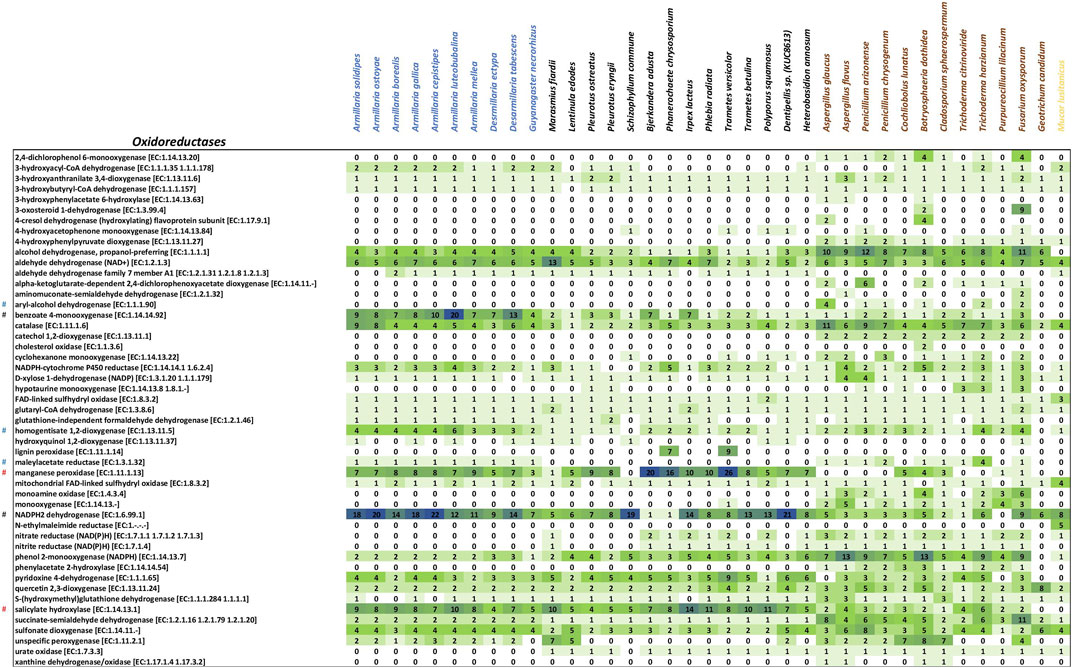
FIGURE 2. Heatmap of mycoremediation-related oxidoreductase counts. Fungal species are on the top X-axis, and the enzyme names are listed along the Y-axis. The intensity of the cell color correlates with the number of counts of a particular enzyme. # Enzyme clusters enriched in Armillarioids.
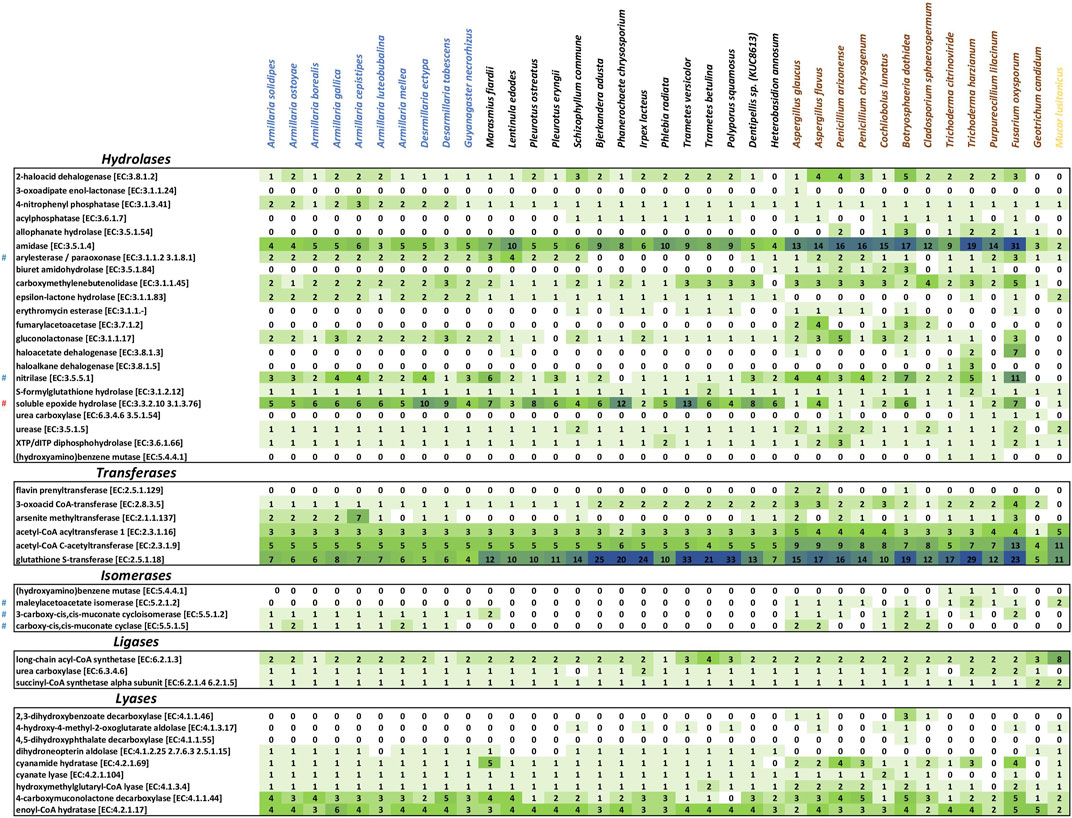
FIGURE 3. Heat maps of hydrolase, transferase, isomerase, ligase and lyase counts associated with mycoremediation. Fungal species are at the top of the X-axis, and the enzyme names are along the Y-axis. The intensity of the cell color correlates with the number of counts of a particular enzyme.
Phylogenetic analysis of 143 benzoate-4-monooxygenase and 331 NADPH2 dehydrogenase proteins from all fungi uncovered a diverse family of both proteins (Supplementary Figures 4, 5). Despite the apparent structural diversity, no significant substitutions were observed in the armillarioid NADPH2 dehydrogenase proteins compared to Ascomycota and Basidiomycota based on their MEME motif analysis of the active site, substrate-binding, and cofactor-binding sites (data not shown). However, multiple sequence alignment analysis revealed slight but significant differences in the substrate- and heme-binding sites of the benzoate-4-monooxygenases (Supplementary Figure 6). The variations in hydrophobicity and the size of substitutions at the 7th and 2nd positions of the substrate-binding site may affect the overall shape of the substrate-binding surface, thereby increasing or descreasing specificity. Furthermore, at the 6th position of the heme-binding site, a hydrophobic leucine in armillarioids is replaced with a polar, hydrophilic glutamin in Basidiomycota and Ascomycota. It is known that a more hydrophobic environment is preferred in the interaction of heme with the binding site (Liou et al., 2014). These possibilities require further extensive experimentation to validate.
3.2 Genes involved in degrading monocyclic aromatics
In general, genes other than benzoate 4-monooxygenase involved in modifying and degrading various monocyclic aromatic compounds, namely, homogentisate 1,2-dioxygenase (HGD) [EC:1.13.11.5] (Figure 2), aryl-alcohol dehydrogenase [EC:1.1.1.90] (Figure 2), maleylacetate reductase [EC:1.3.1.32] (Figure 2), arylesterase/paraoxonase [EC:3.1.1.2/3.1.8.1] (Figure 3), nitrilase [EC:3.5.5.1] (Figure 3), maleylacetoacetate isomerase [EC:5.2.1.2] (Figure 3), and 3-carboxy-cis,cis-muconate cycloisomerase [EC:5.5.1.2] (Figure 3) were randomly distributed in the genomes analyzed. HGD, maleylacetate reductase, and nitrilase genes were relatively more prevalent in armillarioids and ascomycetes (Figures 2, 3; Supplementary File 1).
Notably, the gene copy numbers of HGDs were predominantly higher in all the armillarioid species and certain ascomycetous white-rot species than in other basidiomycetes. A. luteobubalina had 6 copies of HGD genes, and other Armillaria species had at least 3 copies. From the ascomycetous genomes, T. harzianum and F. oxysporum contained the highest copy number of HGDs (4 copies each).
Maleylacetate reductase [EC:1.3.1.32]—converting various aromatic compounds (resorcinol, fluorobenzoate, chlorocyclohexane, chlorobenzene, toluene, benzoate) to 3-oxoadipate–was present as a single copy in all armillarioid species but was missing from the genomes of all other basidiomycetes. The copy number distribution of maleylacetate reductases was not homogenous in ascomycetes as some had higher copies, and some did not show a single copy of the gene. For example, T. harzianum and P. chrysogenum manifested 4 and 2 copies of maleylacetate reductases, respectively.
Similarly to maleylacetate reductase genes, all Armillaria spp., Desarmillaria spp., and some ascomycetous fungi shared comparable copy numbers of 3-carboxy-cis,cis-muconate cyclase [EC:5.5.1.5] (Figure 3) genes. Regarding nitrilases acting potentially also on certain aromatic compounds, armillarioids and ascomycetous fungi had average copy numbers of 2 and 3 nitrilases [EC:3.5.5.1], respectively, while other basidiomycetous fungi had a mean copy of only one. The copy number of arylesterase/paraoxonase (Figure 3), an aminobenzoate-degrading enzyme, was comparatively higher in armillarioids than in other basidiomycetes and ascomycetes.
Other genes encoding aromatic-degrading enzymes, aryl-alcohol dehydrogenases, and maleylacetoacetate isomerases (Figures 2, 3) occur only in ascomycetous species.
3.3 Genes responsible for degrading PAHs
Gene copy numbers of salicylate hydroxylases [EC:1.14.13.1] (Figure 2), soluble epoxide hydrolases [EC:3.3.2.10] (Figure 3) and MnPs [EC:1.11.1.13] (Figure 2), which partake in the breakdown of PAHs were much higher in basidiomycetous fungi. More copies (≥4) of soluble epoxide hydrolases [EC:3.3.2.10] (EAWAG-BBD reaction, reacID# r1120), which are benzo [a]pyrene (PAH) -degrading enzymes (Keck et al., 2006), have their genes in armillarioids and the other white-rot basidiomycetes. One or two copies of the gene were present in the ascomycetous fungi except for F. oxysporum and B. dothidea, which possessed 7 and 6 copies, respectively.
The mean gene copy number of another PAH-degrading enzyme, salicylate hydroxylase [EC:1.14.13.1] (EAWAG-BBD enzyme, enzyme ID: e0149) (Figure 2) was also considerably much higher in basidiomycetes compared to ascomycetes. The basidiomycetous species A. luteobubalina, A. mellea, A. ostoyae, A. solidipes, I. lacteus, M. fiardii, P. chrysosporium, P. radiata, P. squamosus, T. betulina, and T. versicolor had ≥8 copies of salicylate hydroxylase genes, whereas in ascomycetes the average copy number was just 2.
The distribution of the MnP genes [EC:1.11.1.13] (Figure 2) was homogenous (8 copies on average) in all basidiomycetes except in B. adusta (20 copies), T. versicolor (26 copies) and P. chrysosporium (16 copies), the ones which showed an extremely high number of anthracene-degrading MnPs. C. lunatus, an ascomycetous fungus, had 5 copies of MnPs, which was the highest among other ascomycetes comprising only 1 or 2 copies.
3.4 Arsenite methyltransferase, epsilon-lacton hydrolase, and sulfonate dioxygenase enzymes for the bioremediation of antibiotics, caprolactam, and sulphonate-based herbicides
Arsenite methyltransferase [EC:2.1.1.137] (Figure 3), involved in the first conversion step of organoarsenic compounds such as methylarsonic acid to dimethylarsinate (EAWAG-BBD reaction, reacID: r0805) (Shah and Damare, 2018), was found to be most abundant in A. cepistipes, which had an exclusive 7 copies while A. solidipes, A. ostoyae, A. borealis, and A. gallica had only 2 copies each. In addition, 1 copy of the arsenite methyltransferase gene was found in A. luteobubalina, D. tabescens, D. ectypa, S. commune, Dentipellis sp. KUC8613, and H. annosum, but not in A. mellea or G. necrorhizus. On average, ascomycetous white-rot fungi possessed one copy of arsenite methyltransferase, and among all studied ascomycetous fungi, F. oxysporum had the highest number (3 copies).
All armillarioid species except A. luteobubalina possessed 2 copies of caprolactam-degrading epsilon-lactone hydrolases [EC:3.1.1.83] (Figure 3). A. luteobubalina and other basidiomycetes had only 1 copy of epsilon-lactone hydrolase. They were also found to be almost non-existent in the ascomycetous white-rot species.
The gene copy number of sulfonate dioxygenase [EC:1.14.11.-] (Figure 2), a sulfonate-degrading enzyme, was higher in ascomycetous fungi and armillarioid species than in other basidiomycetes. Their number was evenly distributed in Armillaria but varied from 1 to 8 among ascomycetous fungi. Two basidiomycetous species, L. edodes and Dentipellis sp. KUC8613 were exceptions as their genomes contained 5 copies.
3.5 RNA-seq profiling of genes involved in the degradation of various aromatic compounds
Initial expression profiling of genes with mycoremediation potential was performed using raw RNA-Seq data from recently published in vitro stem invasion assays (Sahu et al., 2023) to analyse their expression under native plant-interactive and artificial, rich media conditions. The RSTO medium, which provides a broad spectrum of substrates, is essential for Armillaria species initiating fruiting body formation and a critical inoculum allowing the invasiveness of less virulent isolates in stem invasion experiments. Gene expression profiles were explored in single pairs of highly virulent and less virulent isolates of two conifer specific Armillaria species (A. borealis and A. ostoyae) and found that the majority of identified mycoremediation genes were expressed in both species, 95% in A. ostoyae and 87% in A. borealis (Supplementary File 1; Table 1).
For the comparative expression analysis of the benzoate-4-monooxygenase genes, the individual substrate and heme-binding sites of the genes were also included (Figure 4). Interestingly, 4 out of 5 genes having leucine or alanine at the 2nd and leucine at the 7th position in the substrate-binding sites–representing the most likely armillarioid-specific residues (Supplementary Figure 6)—showed an in planta response where genes were upregulated under stem-invasive conditions in all isolates of both species. Notably, 1 A. borealis gene (Ambor|1721289) appeared to contribute to virulence, as it was significantly overexpressed under plant-invasive conditions in the virulent isolate compared to the less-virulent one. In contrast, 7 other genes with altered substitutions at the 2nd and/or 7th positions, or significantly different replacements at other positions in the binding site were silent.
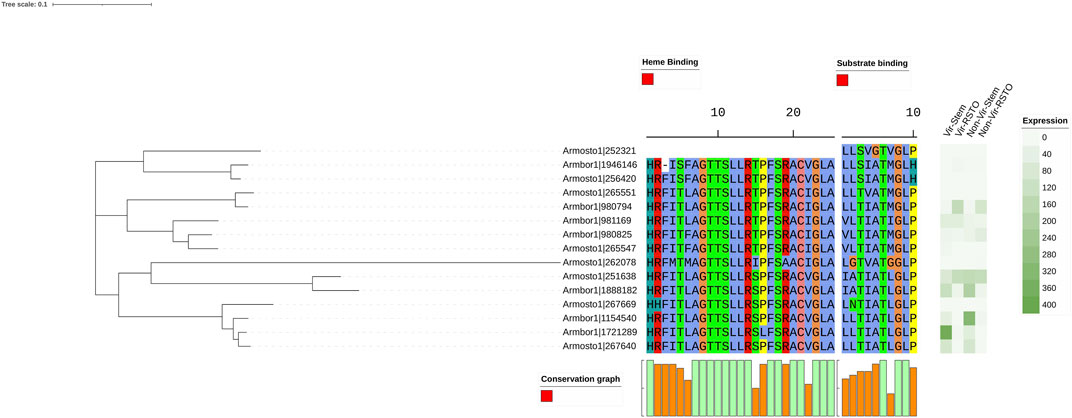
FIGURE 4. Heatmaps of gene expression and alignments of heme and substrate binding sites of the benzoate-4-monooxygenase genes. High and low virulent A. ostoyae and A. borealis isolate pairs were used in the in vitro stem invasion assays. “Vir” and “Non-Vir” refer to the high and low virulent isolates, respectively, and “Stem” and “RSTO” indicate mycelia isolated from the underbark tissue of stem segments and mycelia growing on rich media.
The homogentisate 1,2-dioxygenase genes were also relatively more abundant in Armillaria species. Their comparative gene expression profiling showed that 3 of the 8 genes, similarly to benzoate-4-monooxygenases, were also upregulated under plant-invasive conditions, while the rest was silent or expressed differently (Figure 5).
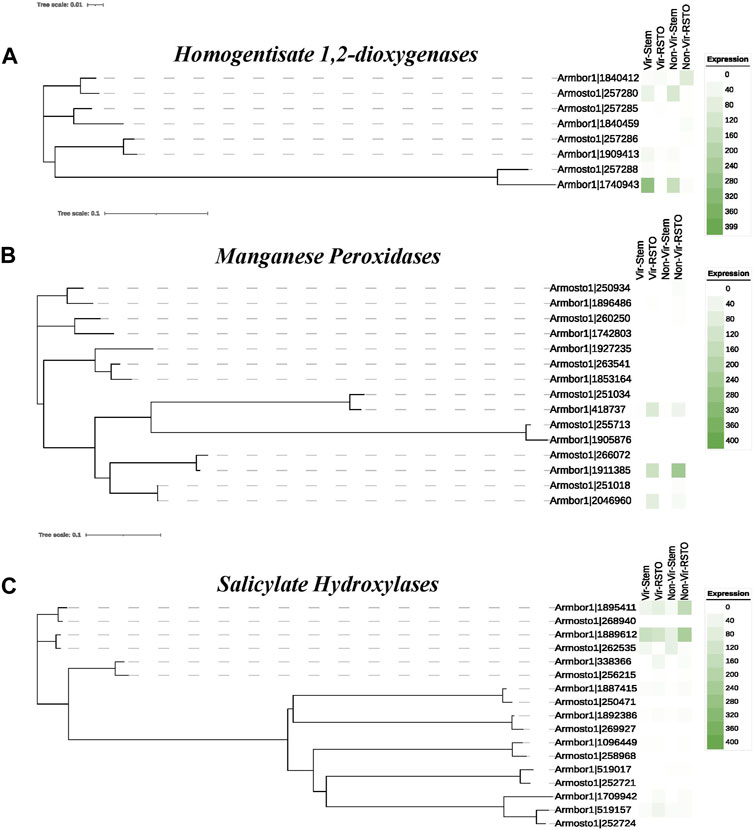
FIGURE 5. Heatmaps of the expression of HGD (A), MnP (B) and salicylate-hydroxylase (C) genes. High and low virulent A. ostoyae and A. borealis isolate pairs were used in the in vitro stem invasion assays. “Vir” and “Non-Vir” refer to the high and low virulent isolates, respectively, and “Stem” and “RSTO” indicate mycelia isolated from the underbark tissue of stem segments and mycelia growing on rich media.
Regarding the gene expression profiles of salicylate hydroxylases and MnPs, both involved in the degradation of PAHs and prevalent in Basidiomycota, they showed different expression patterns from benzoate monooxygenase and HGD genes (Figure 5) as their expression levels tended to increase on rich media.
4 Discussion
In the search for ecologically friendly and effective practical solutions to environmental pollution, mycoremediation proves to be a promising option that meets all the necessary criteria, such as cost efficiency, environmental friendliness, and effectiveness. Additionally, using fungi and fungal enzymes to treat various chemical wastes can offer greater flexibility and cost-effectiveness than typical chemical remediation techniques.
Our preliminary tests using an initial comparative genomics analysis to predict mycoremediation-related genes in Dentopellis sp. (KUC8613) identified 141 genes in the Dentopellis genome. Among these genes, 34 were significantly upregulated in a PAH-based mycoremediation experiment conducted by Park et al. (2019). The strong positive correlation (R = 0.86, p-value = 2.3e-07) observed for the upregulated enzyme repertoire within the expressed genome level counts suggested that the differential expression of these genes may indicate the genetic potential within the genomes and also implied that the presence and abundance of mycoremediation-related genes in a genome can be linked to enzymatic activities and capabilities required for efficient biodegradation. These findings prompted us to explore further and compare the gene repertoires in other fungal species, focusing on possible functional diversities and specializations within expanded bioremediation-related functions.
An extensive comparative genomics analysis of xenobiotics-degrading gene/protein copy numbers in 36 fungal species gave us more profound insights into the distribution and abundance of key enzymes involved in mycoremediation across diverse taxa. Our findings revealed that armillarioid species have a distinctive potential to degrade benzoic acid derivatives. It was reflected in the genetically diverse, increased repertoires of benzoate-degrading benzoate 4-monooxygenase genes in the armillarioid species (Supplementary Figure 4). Consequently, these data suggest that armillarioid species could efficiently treat environmental benzoate contaminations. However, benzoate 4-monooxygenase [EC 1.14.14.92], an oxidoreductase enzyme in high copy numbers in the genomes of armillarioids, offers only a single step in transforming benzoate to 4-hydroxybenzoate (Fuchs et al., 2011). The genes involved in further converting 4-hydroxybenzoate still need to be identified, and the complete degradation process should be adequately studied.
Based on the increased repertoire of NADPH2 dehydrogenase/xenobiotic reductase [EC:1.6.99.1] in the armillarioids (Supplementary Figure 5) it can also be speculated that they possess enhanced nitroglycerin-degrading potential. NADPH2 dehydrogenase might be involved in converting nitroglycerin to 1,2-dinitroglycerol. Pal and Christodoulatos (1995) used P. chrysosporium to study the nitroglycerin-degrading potential of fungi in a mixed batch reactor and observed that the fungus was highly efficient in degrading nitroglycerine and 2,4-dinitrotoluene. Our analysis shows that armillarioids have expanded nitroglycerine-degrading enzymes [EC:1.6.99.1]. Hence, armillariod species like A. cepistipes and A. ostoyae would be suitable candidates for in vitro screening experiments, besides other routinely used fungi like P. chrysosporium, Penicillium corylophilum, A. fumigatus, and G. candidum.
Maleyl acetate reductases are responsible for the breakdown of chloroaromatic compounds by directing maleyl acetate and its by-products to the 3-oxoadipate pathway (Seibert et al., 1993; Martins et al., 2015). BLAST analysis of armillarioid maleyl acetate dehydrogenase genes with the nr database of NCBI (National Centre for Biotechnology Information) indicated that their consensus sequence was 61% identical (E-value: 6e-145) with that of the maleyl acetate genes of Pseudomonas sp., showing that its presence in the fungal genomes may be the result of horizontal gene transfer.
A. cepistipes might be a promising candidate for arsenic biotransformation compared to other Armillaria and Desarmillaria species because of the high copy number of arsenite methyl transferases. A. cepistipes was previously found to be involved in removing heavy metals from the forest soil (Rigling et al., 2006), and also vanadium from media (Xu et al., 2019). Xu et al. (2019) studied the tolerance index of A. cepistipes, Amanita muscaria, Xerocomus badius, and B. adusta and compared them to one another. A. cepistipes showed acceptable tolerance towards sodium metavanadate (NaVO3) and vanadyl sulfate (VOSO4). However, the questions that remain to be answered are whether arsenite methyltransferases have a role in vanadium uptake and resistance and whether they also share a similar pathway for heavy metal accumulation.
Brunner et al. (2018) studied the plastic-degrading potential of A. cepistipes and A. ostoyae and various other fungal isolates. However, an experimental study of the possibility of fungi degrading polyurethane and polyethylene showed that none of the fungi could degrade the latter (Brunner et al., 2018). Therefore, it is clear from our study that the fungi we analyzed do not possess any genes with a potential role in polyethylene degradation.
Our comparative genomics approach provided a high-level overview of the mycoremediation potential of various fungal species that could efficiently degrade different kinds of pollutants. Although mycoremediation seems promising, various physical and chemical parameters play significant roles in determining the degradation efficiency of fungal strains when the operation is performed in vitro or in situ. For example, it was previously noted by Hefnawy et al. (2017) that a concentration of 0.05% direct blue dye inhibited the growth of A. flavus and Penicillium canescens, and they also found that concentrations beyond 0.01% were already toxic to the fungi, as was evidenced by decreased decolourization values. A similar consequence was observed for P. chrysosporium by Senthilkumar et al. (2014) when they increased the dye concentration; however, optimizing the media and introducing possible inducers such as lignin helped to significantly increase the tolerance. Factors such as temperature and pH were also observed to have crucial effects on biodegradation. For example, Hefnawy et al. (2017) found that A. flavus and P. canescens showed the best activity in the temperature and pH ranges of 30°C-35°C and 4-5, respectively. All these studies indicate that species with more significant biodegradation potential should be prioritized, and further intense research into optimizing the conditions of the preferred reaction pathways is crucial.
Bioremediation interventions primarily apply to the natural environment, but the application process can also be modeled and carried out in a controlled laboratory setting, where optimal conditions can be provided for the growth and activity of microorganisms or enzymes. Recent advancements in genetic and protein engineering have made laboratory procedures and bioengineering substantial in designing and developing more reliable solutions for mycoremediation. As a possible powerful alternative to employing native fungal cells, genetic engineering using the versatile CRISPR-Cas targeted genome editing technology could help to express the critically important genes or their edited versions in an environmentally or biotechnologically more suitable bacterial or fungal host (Jaiswal et al., 2019; Song et al., 2019; Singh et al., 2021). Importantly, the recently developed “HACKing” strategy may offer a highly reliable and efficient system for the co-expression of multiple genes in fungi used in environmental mycoremediation (Yue et al., 2023). Based on our current findings, testing the specialization and efficiency of the genetically diverse benzoate-monooxygenase homologs of the armillarioids in a heterologous system could be the first step toward a selective, targeted, and efficient biodegradation of certain aromatic, xenobiotic compounds in the environment.
5 Conclusion
Our study provides valuable insights into the importance of prior genome-wide analysis of the gene repertoires of various fungi potentially useable in biodegradation and biosorption techniques. We compared the potential biodegrading genes of armillarioid species to other ascomycetous and basidiomycetous fungi and identified the genes/enzymes that could be responsible for unique mycoremediation capabilities. The availability of such comparative data may offer novel and more reliable solutions in designing targeted mycoremediation applications to effectively treat specific environmental pollutants in natural environments. Comparative genomic analysis of 37 genomes showed that gene copy number patterns associated with biodegradation are generally conserved in both ascomycetous and basidiomycetous fungi, with notable exceptions at variable taxon levels. Results highlight the potential of white-rot fungi, particularly armillarioid species, in possible mycoremediation applications. Armillarioid species were found to have unique sets of genes, mainly involved in degrading aromatics, which clustered them separately from other white-rot species in a pPCA, suggesting that armillarioids have evolved unique strategies as well to degrade aromatics in their environment.
Our results demonstrate the benefit of using comparative genomics and transcriptomics data for the initial screening of fungal species and then identifying the most promising fungal candidates for a projected mycoremediation procedure. Future research could identify additional genes, explore further and elucidate the mechanisms underlying the relevant biodegradation pathways, leading to more sophisticated and efficient bioremediation procedures.
Data availability statement
The datasets presented in this study can be found in online repositories. The names of the repository/repositories and accession number(s) can be found in the article/Supplementary Material.
Author contributions
Conceptualization: SC, GS, and LK; formal analysis: SC, BI, and KH; funding acquisition: GS; investigation: SC, AtS, CT, OL, and BI; methodology: SC; project administration: GS and LK; resources: GS, LK, and CV; software: SC; supervision: GS, CV, and LK; validation: GS, AnS, and SC; visualisation: BI, AtS, and SC; writing—original draft preparation: SC, BI, and KH; Writing—review and editing: GS, CV, AnS, and LK. All authors contributed to the article and approved the submitted version.
Funding
This research was funded by the Hungarian Government and the European Union within the frames of the Széchenyi 2020 Programme (GINOP-2.3.2-15-2016-00052). The publication of this article was supported by the RRF-2.1.2-21-2022-00011 project, financed by the Government of Hungary within the framework of the Recovery and Resilience Facility.
Acknowledgments
The research was performed in collaboration with the Genomics and Bioinformatics Core Facility at the Szentágothai Research Centre of the University of Pécs.
Conflict of interest
The authors declare that the research was conducted in the absence of any commercial or financial relationships that could be construed as a potential conflict of interest.
Publisher’s note
All claims expressed in this article are solely those of the authors and do not necessarily represent those of their affiliated organizations, or those of the publisher, the editors and the reviewers. Any product that may be evaluated in this article, or claim that may be made by its manufacturer, is not guaranteed or endorsed by the publisher.
Supplementary material
The Supplementary Material for this article can be found online at: https://www.frontiersin.org/articles/10.3389/fbioe.2023.1189640/full#supplementary-material
References
Akhtar, N., and Mannan, M. A. (2020). Mycoremediation: expunging environmental pollutants. Biotechnol. Rep. 26, e00452. doi:10.1016/j.btre.2020.e00452
Alam, R., Mahmood, R. A., Islam, S., Ardiati, F. C., Solihat, N. N., Alam, M. B., et al. (2023). Understanding the biodegradation pathways of azo dyes by immobilized white-rot fungus, Trametes hirsuta D7, using UPLC-PDA-FTICR MS supported in silico simulations and toxicity assessment. Chemosphere 313, 137505. doi:10.1016/j.chemosphere.2022.137505
Ali, A., Guo, D., Mahar, A., Wang, P., Shen, F., Li, R., et al. (2017). Mycoremediation of potentially toxic trace elements - a biological tool for soil cleanup: a review. Pedosphere 27 (2), 205–222. doi:10.1016/S1002-0160(17)60311-4
Ansari, A. A., Naeem, M., Gill, S. S., and AlZuaibr, F. M. (2020). Phytoremediation of contaminated waters: an eco-friendly technology based on aquatic macrophytes application. Egypt J. Aqua Res. 46 (4), 371–376. doi:10.1016/j.ejar.2020.03.002
Aramaki, T., Blanc-Mathieu, R., Endo, H., Ohkubo, K., Kanehisa, M., Goto, S., et al. (2020). KofamKOALA: KEGG ortholog assignment based on profile HMM and adaptive score threshold. Bioinformatics 36 (7), 2251–2252. doi:10.1093/bioinformatics/btz859
Babič, J., Likozar, B., and Pavko, A. (2012). Optimization of ligninolytic enzyme activity and production rate with Ceriporiopsis subvermispora for application in bioremediation by varying submerged media composition and growth immobilization support. Int. J. Mol. Sci. 13 (9), 11365–11384. doi:10.3390/ijms130911365
Bailey, T. L., Johnson, J., Grant, C. E., and Noble, W. S. (2015). The MEME suite. Nucleic Acids Res. 43, W39–W49. doi:10.1093/nar/gkv416
Barh, A., Kumari, B., Sharma, S., Annepu, S. K., Kumar, A., Kamal, S., et al. (2019). Mushroom mycoremediation: kinetics and mechanism. Smart Bioremediation Technol. 2019, 1–22. doi:10.1016/B978-0-12-818307-6.00001-9
Barr, D. P., and Aust, S. D. (1994). Pollutant degradation by white rot fungi. Rev. Environ. Contam. Toxicol. 138, 49–72. doi:10.1007/978-1-4612-2672-7_3
Brunner, I., Fischer, M., Rüthi, J., Stierli, B., and Frey, B. (2018). Ability of fungi isolated from plastic debris floating in the shoreline of a lake to degrade plastics. PLoS One 13 (8), e0202047. doi:10.1371/journal.pone.0202047
Cajnko, M. M., Oblak, J., Grilc, M., and Likozar, B. (2021). Enzymatic bioconversion process of lignin: mechanisms, reactions and kinetics. Biores Tech. 340, 125655. doi:10.1016/j.biortech.2021.125655
Dao, A. T., Vonck, J., Janssens, T. K., Dang, H. T., Brouwer, A., and de Boer, T. E. (2019). Screening white-rot fungi for bioremediation potential of 2, 3, 7, 8-tetrachlorodibenzo-p-dioxin. Ind. Crops Prod. 128, 153–161. doi:10.1016/j.indcrop.2018.10.059
Davolos, D., Russo, F., Canfora, L., Malusà, E., Tartanus, M., Furmanczyk, E. M., et al. (2021). A genomic and transcriptomic study on the DDT-resistant Trichoderma hamatum FBL 587: first genetic data into mycoremediation strategies for DDT-polluted sites. Microorganisms 9 (8), 1680. doi:10.3390/microorganisms9081680
Fu, Y., and Viraraghavan, T. (2001). Fungal decolorization of dye wastewaters: a review. Biores. Technol. 79 (3), 251–262. doi:10.1016/S0960-8524(01)00028-1
Fuchs, G., Boll, M., and Heider, J. (2011). Microbial degradation of aromatic compounds—From one strategy to four. Nat. Rev. Microbiol. 9 (11), 803–816. doi:10.1038/nrmicro2652
Gao, J., Ellis, L. B. M., and Wackett, L. P. (2010). The university of Minnesota biocatalysis/biodegradation database: improving public access. Nucleic Acids Res. 38 (1), D488–D491. doi:10.1093/nar/gkp771
Glenn, J. K., and Gold, M. H. (1983). Decolorization of several polymeric dyes by the lignin-degrading basidiomycete Phanerochaete chrysosporium. Appl. Environ. Microbiol. 45 (6), 1741–1747. doi:10.1128/aem.45.6.1741-1747.1983
Gul, R., Sharma, P., Kumar, R., Umar, A., Ibrahim, A. A., Alhamami, M., et al. (2023). A sustainable approach to the degradation of dyes by fungal species isolated from industrial wastewaters: performance, parametric optimization, kinetics and degradation mechanism. Environ. Res. 216, 114407. doi:10.1016/j.envres.2022.114407
Hefnawy, M. A., Gharieb, M. M., Shaaban, M. T., and Soliman, A. M. (2017). Optimization of culture condition for enhanced decolorization of direct blue dye by Aspergillus flavus and Penicillium canescens. J. Appl. Pharm. Sci. 7 (2), 83–92. doi:10.7324/JAPS.2017.70210
Herrero, M., and Stuckey, D. C. (2015). Bioaugmentation and its application in wastewater treatment: a review. Chemosphere 140, 119–128. doi:10.1016/j.chemosphere.2014.10.033
Jaiswal, S., Singh, D. K., and Shukla, P. (2019). Gene editing and systems biology tools for pesticide bioremediation: a review. Front. Microbiol. 10, 87. doi:10.3389/fmicb.2019.00087
Jombart, T., Balloux, F., and Dray, S. (2010). Adephylo: new tools for investigating the phylogenetic signal in biological traits. Bioinformatics 26 (15), 1907–1909. doi:10.1093/bioinformatics/btq292
Kanehisa, M., Sato, Y., Kawashima, M., Furumichi, M., and Tanabe, M. (2016). KEGG as a reference resource for gene and protein annotation. Nucleic Acids Res. 44 (D1), D457–D462. doi:10.1093/nar/gkv1070
Kapahi, M., and Sachdeva, S. (2017). Mycoremediation potential of Pleurotus species for heavy metals: a review. Biores. Bioproc. 4 (1), 32. doi:10.1186/s40643-017-0162-8
Katoh, K., and Standley, D. M. (2013). MAFFT multiple sequence alignment software version 7: improvements in performance and usability. Mol. Biol. Evol. 30 (4), 772–780. doi:10.1093/molbev/mst010
Keck, A., Conradt, D., Mahler, A., Stolz, A., Mattes, R., and Klein, J. (2006). Identification and functional analysis of the genes for naphthalenesulfonate catabolism by Sphingomonas xenophaga BN6. Microbiol. (UK) 152 (7), 1929–1940. doi:10.1099/mic.0.28783-0
Kedves, O., Shahab, D., Champramary, S., Chen, L., Indic, B., Bóka, B., et al. (2021). Epidemiology, biotic interactions and biological control of Armillarioids in the Northern Hemisphere. Pathogens 10 (1), 76. doi:10.3390/pathogens10010076
Kogej, A., Likozar, B., and Pavko, A. (2010). Lead biosorption by self-immobilized Rhizopus nigricans pellets in a laboratory scale packed bed column: mathematical model and experiment. Food Technol. Biotechnol. 48 (3), 344–351.
Krijger, J. J., Thon, M. R., Deising, H. B., and Wirsel, S. G. (2014). Compositions of fungal secretomes indicate a greater impact of phylogenetic history than lifestyle adaptation. BMC Genom 15 (1), 722–819. doi:10.1186/1471-2164-15-722
Kulshreshtha, S., Mathur, N., and Bhatnagar, P. (2014). Mushroom as a product and their role in mycoremediation. AMB Expr. 4 (1), 29. doi:10.1186/s13568-014-0029-8
Lee, H., Jang, Y., Choi, Y. S., Kim, M. J., Lee, J., Lee, H., et al. (2014). Biotechnological procedures to select white rot fungi for the degradation of PAHs. J. Microbiol. Meth. 97, 56–62. doi:10.1016/j.mimet.2013.12.007
Letunic, I., and Bork, P. (2021). Interactive Tree Of Life (iTOL) v5: an online tool for phylogenetic tree display and annotation. Nucl. Acids. Res. 49 (W1), W293–W296. doi:10.1093/nar/gkab301
Liou, Y. F., Charoenkwan, P., Srinivasulu, Y. S., Vasylenko, T., Lai, S. C., Lee, H. C., et al. (2014). SCMHBP: prediction and analysis of heme binding proteins using propensity scores of dipeptides. BMC Bioinfo 15 (16), S4. doi:10.1186/1471-2105-15-S16-S4
Marchler-Bauer, A., Derbyshire, M. K., Gonzales, N. R., Lu, S., Chitsaz, F., Geer, L. Y., et al. (2015). CDD: NCBI’s conserved domain database. Nuc. Acids. Res. 43 (Database issue), D222-6. doi:10.1093/nar/gku1221
Martins, T. M., Hartmann, D. O., Planchon, S., Martins, I., Renaut, J., and Silva Pereira, C. (2015). The old 3-oxoadipate pathway revisited: new insights in the catabolism of aromatics in the saprophytic fungus Aspergillus nidulans. Fungal Genet. Biol. 74, 32–44. doi:10.1016/j.fgb.2014.11.002
Matsubara, M., Lynch, J. M., and De Leij, F. A. A. M. (2005). A simple screening procedure for selecting fungi with potential for use in the bioremediation of contaminated land. Enzyme Microb. Technol. 39 (7), 1365–1372. doi:10.1016/j.enzmictec.2005.04.025
McMullan, G., Meehan, C., Conneely, A., Kirby, N., Robinson, T., Nigam, P., et al. (2001). Microbial decolourisation and degradation of textile dyes. Appl. Microbiol. Biotechnol. 56 (1–2), 81–87. doi:10.1007/s002530000587
Muszewska, A., Stepniewska-Dziubinska, M. M., Steczkiewicz, K., Pawlowska, J., Dziedzic, A., and Ginalski, K. (2017). Fungal lifestyle reflected in serine protease repertoire. Sci. Rep. 7 (1), 9147–9212. doi:10.1038/s41598-017-09644-w
Pal, N., and Christodoulatos, C. (1995). Fungal degradation of 2,4-dinitrotoluene and nitroglycerin in batch and fixed-film bioreactors. J. Energ. Mat. 13 (3–4), 259–282. doi:10.1080/07370659508019388
Park, H., and Choi, I. G. (2020). Genomic and transcriptomic perspectives on mycoremediation of polycyclic aromatic hydrocarbons. Appl. Microbiol. Biotechnol. 104, 6919–6928. doi:10.1007/s00253-020-10746-1
Park, H., Min, B., Jang, Y., Kim, J., Lipzen, A., Sharma, A., et al. (2019). Comprehensive genomic and transcriptomic analysis of polycyclic aromatic hydrocarbon degradation by a mycoremediation fungus, Dentipellis sp. KUC8613. Appl. Microbiol. Biotechnol. 103, 8145–8155. doi:10.1007/s00253-019-10089-6
Patel, H., Gupte, S., Gahlout, M., and Gupte, A. (2014). Purification and characterization of an extracellular laccase from solid-state culture of Pleurotus ostreatus HP-1. 3 Biotech. 4 (1), 77–84. doi:10.1007/s13205-013-0129-1
Price, M. N., Dehal, P. S., and Arkin, A. P. (2010). FastTree 2–approximately maximum-likelihood trees for large alignments. PloS One 5 (3), e9490. doi:10.1371/journal.pone.0009490
Rabinovich, M. L., Bolobova, A. V., and Vasilchenko, L. G. (2004). Fungal decomposition of natural aromatic structures and xenobiotics: a review. Appl. Biochem. Microbiol. 40 (1), 1–17. doi:10.1023/B:ABIM.0000010343.73266.08
Rajhans, G., Barik, A., Sen, S. K., and Raut, S. (2021). Degradation of dyes by fungi: an insight into mycoremediation. BioTechnologia 102 (4), 445–455. doi:10.5114/bta.2021.111109
Rhodes, C. J. (2014). Mycoremediation (bioremediation with fungi)–growing mushrooms to clean the earth. Chem. Spec. Bioavail. 26 (3), 196–198. doi:10.3184/095422914X14047407349335
Rigling, D., Günthardt-Goerg, M. S., Blauenstein, H., and Frey, B. (2006). Accumulation of heavy metals into Armillaria rhizomorphs from contaminated soils. For. Snow Landsc. Res. 80 (2), 213–220.
Sahu, N., Indic, B., Wong-Bajracharya, J., Merényi, Z., Ke, H. M., Ahrendt, S., et al. (2023). Vertical and horizontal gene transfer shaped plant colonization and biomass degradation in the fungal genus. Armillaria Nat. Microbiol. 2023, 01448. doi:10.1038/s41564-023-01448-1
Sahu, N., Merényi, Z., Bálint, B., Kiss, B., Sipos, G., Owens, R. A., et al. (2021). Hallmarks of Basidiomycete soft- and white-rot in wood-decay -omics data of two Armillaria species. Microorganisms 9 (1), 149. doi:10.3390/microorganisms9010149
Seibert, V., Stadler-Fritzsche, K., and Schlömann, M. (1993). Purification and characterization of maleylacetate reductase from Alcaligenes eutrophus JMP134(PJP4). J. Bacteriol. 175 (21), 6745–6754. doi:10.1128/jb.175.21.6745-6754.1993
Senthilkumar, S., Perumalsamy, M., and Janardhana Prabhu, H. (2014). Decolourization potential of white-rot fungus Phanerochaete chrysosporium on synthetic dye bath effluent containing amido black 10B. J. Saudi Chem. Soc. 18 (6), 845–853. doi:10.1016/j.jscs.2011.10.010
Shah, S., and Damare, S. R. (2018). Differential protein expression in a marine-derived Staphylococcus sp. NIOSBK35 in response to arsenic(III). 3Biotech 8 (6), 287. doi:10.1007/s13205-018-1307-y
Singh, A. K., Bilal, M., Iqbal, H. M. N., Meyer, A. S., and Raj, A. (2021). Bioremediation of lignin derivatives and phenolics in wastewater with lignin modifying enzymes: status, opportunities and challenges. Sci. Total Environ. 777, 145988. doi:10.1016/j.scitotenv.2021.145988
Sipos, G., Prasanna, A. N., Walter, M. C., O'Connor, E., Bálint, B., Krizsán, K., et al. (2017). Genome expansion and lineage-specific genetic innovations in the forest pathogenic fungi Armillaria. Nat. Ecol. Evol. 1, 1931–1941. doi:10.1038/s41559-017-0347-8
Song, R., Zhai, Q., Sun, L., Huang, E., Zhang, Y., Zhu, Y., et al. (2019). CRISPR/Cas9 genome editing technology in filamentous fungi: progress and perspective. Appl. Microbiol. Biotechnol. 103, 6919–6932. doi:10.1007/s00253-019-10007-w
Stecher, G., Tamura, K., and Kumar, S. (2020). Molecular evolutionary genetics analysis (MEGA) for macOS. Mol. Biol. Evol. 37 (4), 1237–1239. doi:10.1093/molbev/msz312
Surendran, A., Siddiqui, Y., Saud, H. M., Ali, N. S., and Manickam, S. (2018). Inhibition and kinetic studies of lignin degrading enzymes of Ganoderma boninense by naturally occurring phenolic compounds. J. Appl. Microbiol. 125 (3), 876–887. doi:10.1111/jam.13922
Taiwo, A. M. (2011). Composting as a sustainable waste management technique in developing countries. J. Environ. Sci. Technol. 4 (2), 93–102. doi:10.3923/jest.2011.93.102
Tkavc, R., Matrosova, V. Y., Grichenko, O. E., Gostinčar, C., Volpe, R. P., Klimenkova, P., et al. (2018). Prospects for fungal bioremediation of acidic radioactive waste sites: characterization and genome sequence of Rhodotorula taiwanensis MD1149. Front. Microbiol. 8, 2528. doi:10.3389/fmicb.2017.02528
Tyagi, M., da Fonseca, M. M. R., and de Carvalho, C. C. C. R. (2011). Bioaugmentation and biostimulation strategies to improve the effectiveness of bioremediation processes. Biodegradation 22 (2), 231–241. doi:10.1007/s10532-010-9394-4
Wickham, H. (2016). Ggplot2: elegant graphics for data analysis, second edition. Switzerland: Springer. Use R!. doi:10.1007/978-3-319-24277-4
Xu, Y. H., Brandl, H., Osterwalder, S., Elzinga, E. J., and Huang, J.-H. (2019). Vanadium-basidiomycete fungi interaction and its impact on vanadium biogeochemistry. Environ. Int. 130, 104891. doi:10.1016/j.envint.2019.06.001
Yadav, P., Rai, S. N., Mishra, V., and Singh, M. P. (2021). Mycoremediation of environmental pollutants: a review with special emphasis on mushrooms. Environ. Sust. 4 (4), 605–618. doi:10.1007/s42398-021-00197-3
Yesilada, O., Birhanli, E., and Geckil, H. (2018). “Bioremediation and decolorization of textile dyes by white rot fungi and laccase enzymes,” in Mycoremediation and environmental sustainability. Editor R. Prasad (Cham: Fungal Biology; Springer International Publishing), 121–153. doi:10.1007/978-3-319-77386-6_5
Keywords: mycoremediation, biodegradation, armillarioids, white-rot, phylogenetic principal component analysis, benzoate 4-monooxygenase
Citation: Champramary S, Indic B, Szűcs A, Tyagi C, Languar O, Hasan KMF, Szekeres A, Vágvölgyi C, Kredics L and Sipos G (2023) The mycoremediation potential of the armillarioids: a comparative genomics analysis. Front. Bioeng. Biotechnol. 11:1189640. doi: 10.3389/fbioe.2023.1189640
Received: 19 March 2023; Accepted: 03 August 2023;
Published: 17 August 2023.
Edited by:
Feng Wang, Jiangsu University, ChinaReviewed by:
Blaž Likozar, National Institute of Chemistry, SloveniaMichael Dare Asemoloye, Tianjin University, China
Copyright © 2023 Champramary, Indic, Szűcs, Tyagi, Languar, Hasan, Szekeres, Vágvölgyi, Kredics and Sipos. This is an open-access article distributed under the terms of the Creative Commons Attribution License (CC BY). The use, distribution or reproduction in other forums is permitted, provided the original author(s) and the copyright owner(s) are credited and that the original publication in this journal is cited, in accordance with accepted academic practice. No use, distribution or reproduction is permitted which does not comply with these terms.
*Correspondence: György Sipos, c2lwb3MuZ3lvcmd5QHVuaS1zb3Byb24uaHU=