- 1Electronic Technology Department, Universidad Rey Juan Carlos, Madrid, Spain
- 2Department of Biomechanical Engineering, University of Twente, Enschede, Netherlands
Assistive ankle-foot orthoses (AAFOs) are powerful solutions to assist or rehabilitate gait on humans. Existing AAFO technologies include passive, quasi-passive, and active principles to provide assistance to the users, and their mechanical configuration and control depend on the eventual support they aim for within the gait pattern. In this research we analyze the state-of-the-art of AAFO and classify the different approaches into clusters, describing their basis and working principles. Additionally, we reviewed the purpose and experimental validation of the devices, providing the reader with a better view of the technology readiness level. Finally, the reviewed designs, limitations, and future steps in the field are summarized and discussed.
1 Introduction
Locomotion is a primary task for human beings and an essential component for a rich quality of life. There might be diverse (neurological or muscular) causes that limit the locomotion ability in humans, especially the efficiency and effectiveness of gait. Among all multi-body segments and muscles involved in walking, those related to the ankle joint are major contributors to perform the required mechanical work (Vaughan et al., 1999; Moltedo et al., 2018; Conner et al., 2022).
Over the last decades, wearable assistive ankle-foot orthoses (AAFOs) have been developed and applied to assist ankle motion in humans. The main aim of these devices is to either reinforce and enhance the mobility in able-bodied subjects (Moltedo et al., 2018), or to restore, assist or rehabilitate lost functions of people with motor disorders (Shorter et al., 2013; Alam et al., 2014; Moltedo et al., 2018; Bayón et al., 2023).
Despite the end goal to be achieved with the AAFO, a major distinction between these devices can be made according to their working principle. Passive AAFOs are those devices that rely on passive elements such as dampers or springs to store and release energy during gait, containing no control or electronics. Quasi-passive (or semi-active) AAFOs use computer control to adjust the performance of a passive element, and sometimes also hold a small motor to modulate their stiffness. Finally, active AAFOs, also called robotic exoskeletons, come with electric or pneumatic actuators connected to a power source to deliver assistive torques or forces to the users.
There are previous reviews that analyzed to some extent the application of articulated AAFOs and their influence on gait. These studies either focused on a single working principle such as passive (Choo and Chang, 2021) or active (Shorter et al., 2013; Moltedo et al., 2018), or concentrated on a single gait impairment (Alam et al., 2014). However, a more extensive comparison of different working principles (passive, quasi-passive, and active), and the effects when being used on both healthy and impaired subjects with different walking limitations is missing.
The aim of this paper is to assess the mechanical designs of devices that focus on providing assistance at the ankle level, and evaluate the gait effects generated by these devices when being tested. This research effort is intended to:
• Understand and describe the actuation mechanisms adopted within the three groups of AAFOs (passive, quasi-passive and active).
• Classify different robotic AAFOs with regard to their mechanical configuration, purpose and effects when evaluated in healthy and impaired subjects.
• Summarize the novelties and limitations of the reviewed systems to be considered in future designs.
In order to contextualize this work, we start with discussing the biomechanics of gait, highlighting the importance of the ankle-complex joint and giving an overview of the most common pathological gait patterns associated with ankle muscles’ weakness and the demanded assistances. In Section 3, we classify different reviewed designs for AAFOs into clusters, reviewing their mechanical configuration and control. Subsequently, in Section 4 and Section 5 we expose the extracted results on how the reviewed devices are applied in terms of the type of assistance provided and the effects generated on final users. Finally, we discuss the outcomes by providing input on advantages and disadvantages of the exposed mechanisms as well as proposing trends for future research.
2 Human gait
Human gait is a very complex activity that involves the coordination of various systems such as the musculoskeletal, nervous, and cardiovascular systems (Vaughan et al., 1999). One of the main characteristics of healthy human gait is the high repeatability, and as such, it is normally segmented in cyclic gait patterns. A gait cycle starts with an initial heel contact with the ground and finishes with the following heel contact of the same limb (Figure 1). Two main phases take place within a gait cycle: the stance phase, in which the ipsilateral limb is in contact with the ground; and the swing phase, in which the ipsilateral foot raises from the ground and the limb advances for the preparation of the following heel strike (Vaughan et al., 1999).
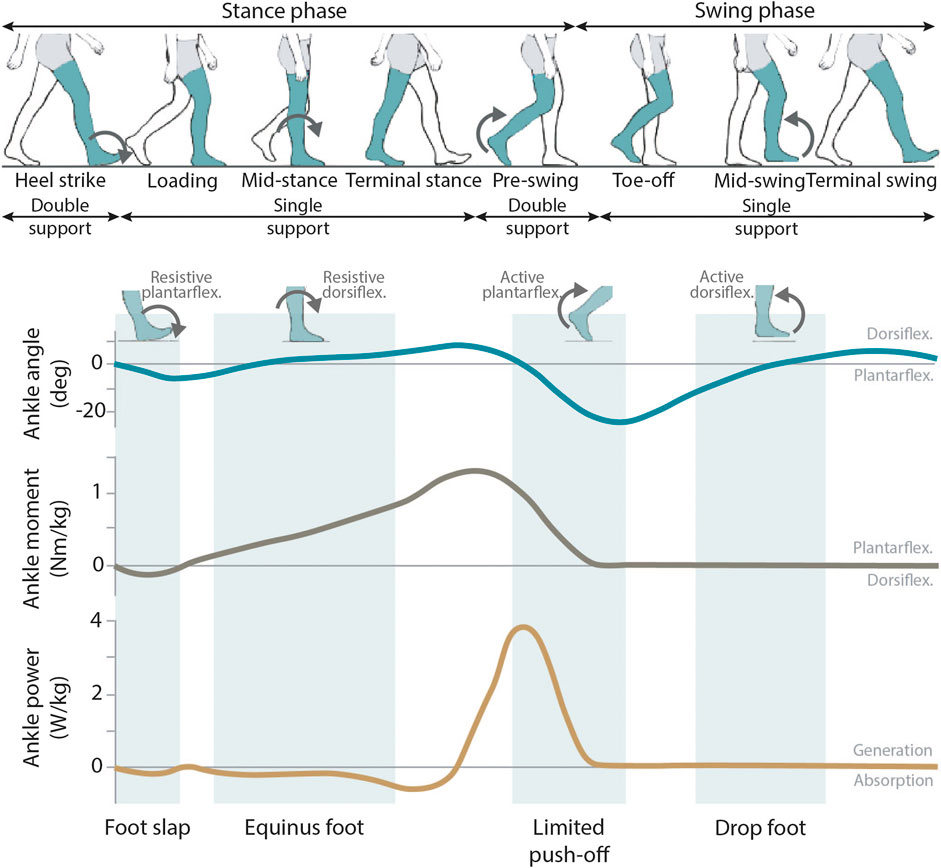
FIGURE 1. General overview of the human gait pattern. The ankle angle, ankle moment and ankle power normal patterns are shown along the gait cycle. In these graphs, the areas where the most common pathological gait patterns associated to the ankle-complex joint can be encountered are marked in green. For each of these common impairments, the required ankle assistance is shown.
2.1 Importance of the ankle-complex joint
The ankle-complex joint has an important and clear role during the full gait cycle (Shorter et al., 2013; Conner et al., 2022). At the beginning of the stance phase (initial heel contact), the muscles and tendons around the ankle are responsible for decelerating both the foot rotation before it enters in full contact with the ground, and the forward progression of the body. During mid-stance, the ankle-complex joint helps in maintaining balance and stability as all the user’s weight is solely supported by the ipsilateral limb (while the contralateral limb is in the swing phase). Finally, with the forward tibia progression during stance and the contraction of ankle plantarflexor muscles, energy is stored to be later delivered at the moment of toe-off as plantarflexor torque. This accounts for a large exerted force that is the main source of forward propulsion power during gait (Shorter et al., 2013) (Figure 1).
2.2 Pathological gait patterns
The role of the ankle-complex joint is compromised in many people with neurological disorders such as stroke, incomplete spinal cord injury or cerebral palsy. This causes a diminished walking ability and the appearance of pathological gait patterns (Beyaert et al., 2015; Armand et al., 2016; Wirz and van Hedel, 2018). The impairments at the ankle level can be related to reduced strength, muscle dysfunction, spasticity, poor selective control or limited range of motion (ROM). Due to the importance of the ankle during gait and in order to limit the consequences of its impaired function, AAFOs are the foremost used type of assistive devices to support walking limitations (Conner et al., 2022; Bayón et al., 2023). For a proper design of AAFO devices, it is key to get a good understanding of the effects of the different pathological gait in the subjects’ walking patterns (Shorter et al., 2013; Conner et al., 2022) (Figure 1).
Weakness in the ankle dorsiflexors (primarily represented by the tibialis anterior) may affect gait in both swing and initial stance phases (Rodda and Graham, 2001; Shorter et al., 2013): during swing, the most common gait deviation is the drop-foot, reflected by an insufficient toe clearance. This deficit is normally compensated by increased knee and hip flexion and hip abduction during swing. Additionally, during initial stance and just after initial heel contact, dorsiflexors’ weakness can also prevent the controlled deceleration of the foot, which is often known as foot-slap.
An impaired function of ankle plantarflexors (with the gastrocnemius and soleus muscles as main contributors) mostly affect the stance phase of gait. Especially, the existence of spasticity or contracture in the gastrocnemius and/or soleus results in equinus pattern (Rodda and Graham, 2001; Brockett and Chapman, 2016), characterized by an abnormal (increased) ankle plantarflexion through most of the stance phase. Additionally, ankle plantarflexors dysfunction also causes a limited push-off power due to the inability of the affected muscles to concentrically contract at the end of the stance phase (Brockett and Chapman, 2016). In general, weakness of ankle platarflexors affects stability, reduces walking speed, shortens step length, and increases the energy cost of walking.
2.3 Type of ankle assistances
Although the ankle-complex joint has three degrees of freedom (DOF), the primary plane during locomotion and where most of the motion is performed is the sagittal plane (Figure 1). Ideally, AAFOs designed to assist walking in neurological disorders should be able to accommodate dorsi- and plantarflexion assistances depending on the user’s needs. This is translated into different assistive strategies related to the targeted gait impairment: 1) avoiding excessive ankle plantarflexion during swing that causes drop-foot, 2) controlling deceleration of the foot at initial heel contact to inhibit foot-slap, 3) preserving stability during mid-stance while preventing equinus pattern, or 4) generating an assistive plantarflexion torque at the instant of push-off. The last one is also a common strategy used to enhance gait in able-bodied subjects.
The accomplishment of all the above-mentioned actions within a design is a real challenge, especially because adding extra weight to a device located at the ankle (distal joint) could hamper the performance of the user increasing their energy cost of walking (Bayón et al., 2023). Moreover, if the purpose of the AAFO device is to be used during daily-life activities, the design should allow some adaptability to the challenging mobility tasks and ground variations encountered in everyday use (e.g., stairs, ramps, uneven terrains…) (Bayón et al., 2023).
3 Mechanical designs for AAFOs
3.1 Passive AAFOs
Passive AAFOs are those that do not require any external power supply to generate the assistance. They have the main benefit of being lightweight compared with active AAFOs, so their use is closer to daily basis. To provide assistance, passive AAFOs need to be articulated, and normally they rely on passive elements such as springs, dampers, or elastic bands (conventional non-assistive passive AFOs are out of the scope of this manuscript). In most cases, the design parameters of the passive elements (e.g., the stiffness of the spring) can be optimized only in very specific situations. Thus, these devices have the main limitation of hindering users’ performance for tasks they are not designed for. Regardless of the passive element that is being used, a relevant classification of passive AAFOs can be done based on their working principle and the mechanical configuration of the device (see Supplementary Material).
3.1.1 Continuous adjustable dynamic response
The importance of tuning the mechanical properties of an articulated AAFO has gained importance in the last few years, and in that regard, adjustable dynamic response AAFOs (ADR-AAFOs) (Figure 2) have been introduced in the market as a potential solution (Ultraflex Systems, 2009; Becker Orthopedic, 2018; Ottobock, 2018; Fior and Gentz, 2021). ADR-AAFOs allow for a variable ankle ROM and selective dorsi- and plantarflexion resistance during both stance and swing phases, making these devices suitable for assisting the full gait cycle. Normally, the working principle of the ADR-AAFOs is based on the use of two springs, one for dorsiflexion and one for plantarflexion. However, this can be enhanced by adding additional springs in parallel that activate at different instants (Kobayashi et al., 2017), simulating the effects of a variable stiffness actuator (Vanderborght et al., 2013). The torques of different designs can be adjusted either by replacing the assistive springs or by modifying the spring preload. Nevertheless, once the spring stiffness has been selected, the provoked torque affects the user along the whole gait cycle, eliminating the possibility for online modulations.
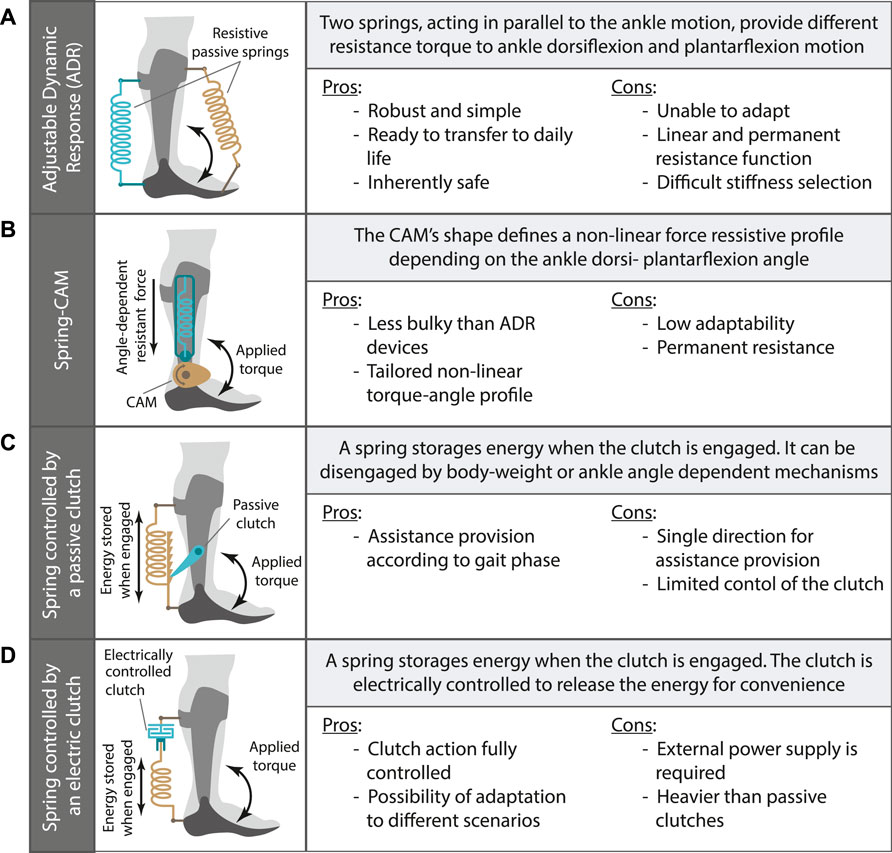
FIGURE 2. Clusters classification for the main mechanical configuration of passive and quasi-passive AAFOs. The working principle and main pros and cons are given for: (A) continuous adjustable dynamic response AAFO; (B) Spring-cam AAFO; (C) clutch-dependent AAFO; (D) Electrically-controlled clutch dependent AAFO.
The target users’ group of ADR-AAFOs is very wide (Kerkum et al., 2015; Wren et al., 2015; Kobayashi et al., 2019; Meyns et al., 2020), but all the designs have the main common intention of dynamically bringing the users into an upright position and improving their stability while walking and standing. This gain on stability may be related to the support of one or more ankle impairments encountered within the gait cycle (Figure 1).
3.1.2 Spring-cam
Spring-cam mechanisms can be used to compensate for diverse walking impairments by customizing individualized torque-angle curves according to the cam shape and the spring stiffness (Figure 2). The cam profile changes the moment arm, which effectively results in a characteristic torque-deflection mechanism that could be also considered to have variable stiffness around the ankle (Vanderborght et al., 2013). Thus, they provide personalized dorsi- and plantarflexion torques to fit the user’s needs. An example of a spring-cam prototype is presented in (Sekiguchi et al., 2020), where authors designed a solution to store energy during dorsiflexion (mid and late stance) that is later released to compensate for push-off (plantarflexion). This mechanism was incorporated in the AAFO device developed in (Yamamoto et al., 2005).
In patients suffering from equinus pattern, the self generated activity of the human has a large effect on the ankle motion. Rodriguez et al. (2018) proposed a spring-cam AAFO to compensate for the increased human stiffness in these patients. The proposed solution used a negative stiffness mechanism composed by a pre-loaded gas-spring and a cam follower.
3.1.3 Clutch-dependent
Clutch-dependent AAFOs (Figure 2) offer the possibility of selecting the periods within the gait cycle in which the stiffness of the passive element should be present. These clutches can be passively controlled, and a common way of doing so is based on either the user’s body weight (Hirai et al., 2006; Yandell et al., 2019; Wang et al., 2020; Liu et al., 2021; van Hoorn et al., 2022) or the ankle angle during gait (Collins et al., 2015; Leclair et al., 2018; Pardoel and Doumit, 2019).
Body-weight dependent clutches for AAFOs are normally located at the bottom of the user’s shoe, and they use the weight of the user during the stance phase to passively control the storage and release of energy. The core of a body-weight dependent clutch can be either compliant (e.g., pneumatic) (Hirai et al., 2006), a mechanism (Wang et al., 2020; Liu et al., 2021), or consisting of a slider-spring system (Yandell et al., 2019; van Hoorn et al., 2022). Several of these approaches have been designed for supporting push-off (Yandell et al., 2019; Wang et al., 2020; Liu et al., 2021; van Hoorn et al., 2022) by engaging an elastic spring when the ankle is dorsiflexing during the stance phase and releasing the stored energy when the ankle starts to plantarflex during push off, without hindering the ankle motion during swing. Other designs had the goal of preventing drop-foot by locking the ankle joint in a neutral position during the swing phase (Hirai et al., 2006). In this last case, the ankle motion is only constrained during swing and not during the stance phase (Hirai et al., 2006).
Ankle angle dependent clutches for AAFOs use the motion of the ankle joint during gait to control the storage and release of energy. These mechanisms are normally more complicated than body-weight clutches as, during a gait cycle, the ankle goes through plantarflexion twice, and thereby, this motion is not directly related to stance and swing phase (as happened with the body-weight). In this case, the pawl-ratchet principle is often used to engage and disengage the mechanism. Angle dependent clutches can be connected to passive elements such as springs (Collins et al., 2015), or to pneumatic artificial muscles (PAMs) that do not require an external power source (Leclair et al., 2018; Pardoel and Doumit, 2019). Devices based on this approach normally have the final goal of empowering human gait by assisting push-off.
3.1.4 Others
Oil dampers can also be used as hydraulically resistant shock absorbers. When the ankle joint moves to plantarflexion during initial stance the resistance produced by an oil damper reduces the rate of angular change. Following this working principle, Yamamoto et al. (2005) developed a passive AAFO to satisfy the requirements for patients with hemiplegia by improving the insufficient eccentric contraction of the dorsiflexors at initial stance (preventing foot-slap).
3.2 Quasi-passive AAFOs
Passive AAFOs are less adaptable than active (powered) devices due to their simplified design and their inability to control their functions. A trade-off between both solutions are the quasi-passive (or semi-active) devices, where sensors and small motors with low energy consumption are used to modulate the stiffness of passive elements (Supplementary Material). This enhances the adaptability of the devices to changes in gait conditions or environments, allowing the passive element to work in a closed-loop to some extent.
3.2.1 Electrically-controlled clutch
Similar to passive AAFOs, there are designs for quasi-passive devices that rely on the action of a clutch. However, in this case, the clutch is normally electrically controlled, and sometimes a small motor is also used to combine both passive energy storage and additional power supply (Figure 2). Such is the case for the design of Zhang et al. (2015), where an electromagnetic clutch was used to command the storage of energy in a compression spring. A DC motor added additional power for energy storage, which offered, in a sense, the inherent variable stiffness (Vanderborght et al., 2013). This device was intended to absorb heel strike impact and enhance push-off.
Another electromagnetically driven clutch-dependent AAFO designed to capture heel-strike energy loss and recycle it into push-off propulsion was recently presented by Wang C. et al. (2022). The clutch and the elastic energy storage mechanism are coupled together by a stiff assistance spring.
Finally, Dezman et al. (2018) presented the modification of an originally passive AAFO making it quasi-passive through an electrically controlled ratchet-pawl clutch. By including the active clutch, the authors could solve the problems with timing of engagement and disengagement specific to each user, being more reliable than with the original passive clutch.
3.2.2 Others
Other quasi-passive designs have been proposed in the literature with the same aim of improving the possibilities of passive AAFOs by adjusting the stiffness or damping. As these designs vary quite a lot from each other, we provide a short description of four of them without following a specific classification.
The first solution, called SmartAFO (Oba et al., 2019), used a quasi-passive technology for the mitigation of drop-foot and foot-slap during gait. In particular, the AAFO used a magnetorheological fluid damper to selectively modulate a variable impedance around the ankle joint. Equipped with an elastic link, the system allows energy storage and release according to its coil current.
Kumar et al. (2020) developed a quasi-passive AAFO for stiffness auto-tuning and adaptability across different walking conditions. In this solution, a stepper motor is used to vary the stiffness of a passive spring in real-time, while electromyography data of the user is being recorded for closing the control loop.
Diller et al. (2016) designed a lightweight, low-power electroadhesive clutch to demonstrate its usage for adjustable spring stiffness in AAFOs. The authors placed several clutched springs in parallel to discretely produce six different levels of stiffness. However, the main drawback of electroadhesive clutches is their failure because of dielectric breakdown, which can expend a significant amount of electrical energy and cause the clutch to disengage.
Finally, the quasi-passive AAFO designed in (Allen et al., 2021) is a dielectric elastomer actuator (DEA) that connects the foot near the toes to the shank under the knee. DEAs are a kind of artificial muscle that, when charged with a constant voltage, produce electrostatic forces. The DEA passively keeps the foot dorsiflexed to the maximum angle recorded during normal gait, making it suitable for drop-foot patients. This device automatically adapts to the user’s gait due to the gait phase detection ability of this AAFO.
3.3 Active AAFOs
There are certain conditions of motion control and assistance that cannot be accomplished with passive elements due to their limited adaptability and control. Active AAFOs have the advantage of using external power supplies and actuators to address these limitations. Differences between active AAFOs’ designs can be found looking to their mechanical configuration and the control strategies used to assist ankle function (see Supplementary Material).
3.3.1 Mechanical configuration of active orthoses
3.3.1.1 Rigid transmission
The straightforward configuration is composed of a rigid transmission between the actuator and the orthosis structure (Choi et al., 2018; de Miguel-Fernandez et al., 2022b; a; Moosavian et al., 2021; Huo et al., 2019; Kim et al., 2020; Li et al., 2011; Shorter et al., 2011; Yeung et al., 2017; Yeung et al., 2018; Yeung et al., 2021) (Figure 3). This configuration aims at ensuring the proper delivery of the torques and forces generated by the actuators. However, as a disadvantage, it is not inherently compliant, as it does not allow adaptations to avoid possible misalignment. Moreover, the movement of the mechanism is restricted to the one commanded by the controller.
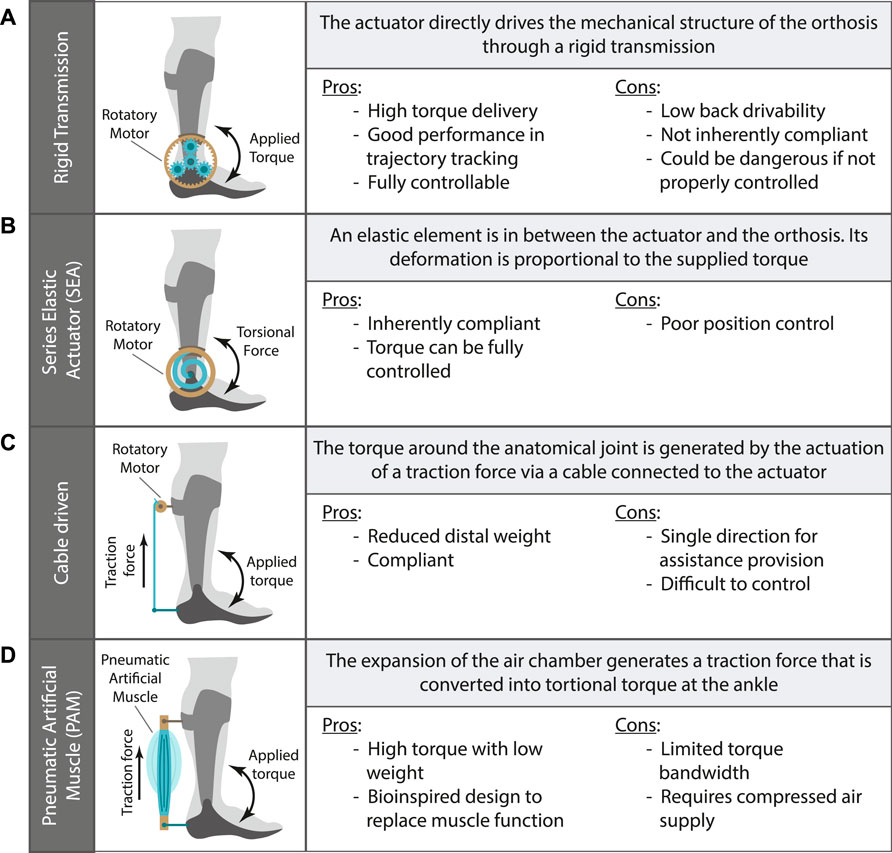
FIGURE 3. Clusters classification for the main mechanical configuration of active AAFOs. The working principle and main pros and cons are given for: (A) AAFO with rigid transmission; (B) AAFO with elastic element -SEA- in the transmission; (C) AAFO driven by a cable; (D) AAFO with a pneumatic artificial muscle -PAM-.
3.3.1.2 Series elastic actuator
To improve the adaptability, comfort, and efficiency of active AAFOs, some research groups have opted for using compliant elements in the torque transmission. These elements allow certain adaptations to the user and reduce the movement restrictions imposed by the orthoses. In this regard, a common approach is the use of elastic elements in series with the actuators to achieve the mentioned compliance (Blaya and Herr, 2004; Oymagil et al., 2007; Kim et al., 2011; Ward et al., 2011; Roy et al., 2013; Meijneke et al., 2014; Dzeladini et al., 2016; Forrester et al., 2016; Mooney and Herr, 2016; van Dijk et al., 2017; Tamburella et al., 2020; Bayón et al., 2022; Durandau et al., 2022) (see Figure 3). This configuration, also called Series Elastic Actuator (SEA), not only enables a less restrictive mechanism, but also allows the direct control of the exerted torque or force by controlling the deformation of the elastic element. Moreover, the SEA configuration allows for the implementation of a variable stiffness control to better adapt to natural changes of muscle tension (Vanderborght et al., 2013).
3.3.1.3 Cable driven
A different approach to obtain a compliant mechanism while reducing the inertia of the device is the use of cables or wires to transmit the force to the human segments (Figure 3) (Mooney et al., 2014a; Mooney et al., 2014b; Witte et al., 2015; Bae et al., 2015; Jackson and Collins, 2015; Awad et al., 2017a; Awad et al., 2017b; Steele et al., 2017; Zhang et al., 2017; Bae et al., 2018a; Bae et al., 2018b; Lerner et al., 2018; McCain et al., 2019; Bougrinat et al., 2019; Jackson and Collins, 2019; Gasparri et al., 2019; Lerner et al., 2019; Siviy et al., 2020; Orekhov et al., 2020; Xia et al., 2020; Gomez-Vargas et al., 2021; Conner et al., 2021; Harvey et al., 2021; Orekhov et al., 2021; Acosta-Sojo and Stirling, 2022; Wang W. et al., 2022; Peng et al., 2022; Fang et al., 2022; Fang and Lerner, 2022; Chen J. et al., 2022; Slade et al., 2022). These devices allow the placement of the major part of their weight in a proximal location (e.g., the pelvis), reducing the mass placed in the distal segments and therefore the inertia added by the device. In addition, some of them do not require a fixed rotatory axis at the AAFO [e.g., (Bae et al., 2015; Mooney and Herr, 2016)], negating the need of a precise alignment between the human ankle and the AAFO joint.
3.3.1.4 Pneumatic artificial muscles
Following a bioinspired approach, PAMs were developed to generate forces when they get deformed by pressurized air. Some researchers opted for the use of PAM actuators for active AAFOs (Cain et al., 2007; Gordon and Ferris, 2007; Sawicki and Ferris, 2008; Kinnaird and Ferris, 2009; Kao et al., 2010; Galle et al., 2013; Galle et al., 2014; Galle et al., 2015; Koller et al., 2015; Takahashi et al., 2015; Galle et al., 2017; Koller et al., 2017; Malcolm et al., 2018) (Figure 3). These actuators enable a high torque supply with a lightweight configuration. However, they are also characterized by slow dynamics while providing assistance and they require a pneumatic pump to power them.
3.3.2 Control of active orthoses
The performance of active AAFOs is determined by the control strategy followed to supply the intended assistance. In this regard, the control algorithm of these devices can be analysed following the bio-inspired hierarchical paradigm previously reported (Tucker et al., 2015; Baud et al., 2021). This paradigm divides the control of robotic exoskeletons into three levels, similarly to the control of human movement by the nervous system. The high-level control corresponds to the volitional control of the movement performed by supraspinal structures. The mid-level control is responsible for the generation of cyclic movement patterns by the spinal Central Pattern Generators. In the lowest level, the afferent information is integrated by the nervous system to properly generate the efferent signal to activate and deactivate agonist and antagonist muscles to effectively perform the movement.
In the context of wearable robotic devices, particularly active AAFOs, these neural controllers are translated into control algorithms. The high-level controllers are responsible for the intention detection and the context extraction, being independent of the device itself. For this reason, we do not report the high-level control in this manuscript. The mid-level controllers, responsible for the movement pattern generation, and the low-level controllers, responsible for closing the feedback loop, are described below.
3.3.2.1 Low-level control
Depending on the low-level control of active AAFOs, the deliverance of the assistance and thus the actuator behaviour can be performed following different paradigms. The most used approach is based on torque or force closed-loops (van Dijk et al., 2017; Choi et al., 2018; Lerner et al., 2018; Kim et al., 2020; Orekhov et al., 2020; Yeung et al., 2021; Peng et al., 2022). These controllers have the advantage of being directly involved in the delivery of the robotic assistance by counteracting the muscular weakness of the impaired users. Other approaches use impedance (Forrester et al., 2016) or position (Huo et al., 2019) controllers to command the assistance (see Supplementary Table S4 in the Supplementary Material).
3.3.2.2 Mid-level control
Mid-level controllers are also required to coordinate an AAFO’s action with the current user’s gait state, thus ensuring that the assistance is delivered at the correct timing (Supplementary Table S4 in the Supplementary Material). This coordination is crucial to avoid instabilities and maximize the benefits of wearing the active device. Mid-level controllers have been previously assessed in a general way for partial and unilateral robotic exoskeletons (Lora-Millan et al., 2022), but without focusing on ankle devices. The most common control strategy to achieve a proper coordination is based on the detection of several gait events that divide the gait into different phases. For each gait phase, an impedance model can be applied to set the assistance level (Forrester et al., 2016; Kim et al., 2020). Additionally, the detection of gait events can also be used to trigger the execution of assistive torque patterns (Mooney et al., 2014a; Acosta-Sojo and Stirling, 2022).
Since these strategies are limited to the instants of detection of the key events, some authors prefer to characterize the full step with a monotonically increasing signal, called continuous gait phase, that is reset once the step is over. Thereby, it enables a more versatile control strategy not limited to key instants. The gait phase can be calculated based on the duration of previous steps and the time elapsed from the last heel-strike (Bougrinat et al., 2019) or can be estimated in real-time by using adaptive frequency oscillators (van Dijk et al., 2017).
Finally, a third option would be to generate reference trajectories to be followed by the device from data directly measured on the subject. These references can be based on movement information from the assisted joint (Lerner et al., 2019; Harvey et al., 2021) or on the muscular activity that generates such movements (Koller et al., 2015; McCain et al., 2019).
4 Type of assistance provided
Although the reviewed literature (Supplementary Tables in Supplementary Material) has revealed different mechanical designs for passive, quasi-passive, and active devices, a possible combined classification of all of them can be done according to their goal: 1) to empower healthy walking, 2) to assist pathological gait, or 3) for rehabilitation purposes. Additionally, within each general purpose, we can identify the type of walking assistance that every device is intended for. These results for all analyzed devices (a total of 54) are presented in Table 1. Although the majority of these devices were designed for a unique target population (healthy or impaired walking subjects), some of them considered the gait assistance for both populations (Leclair et al., 2018; Yandell et al., 2019; Wang C. et al., 2022; Bayón et al., 2022).
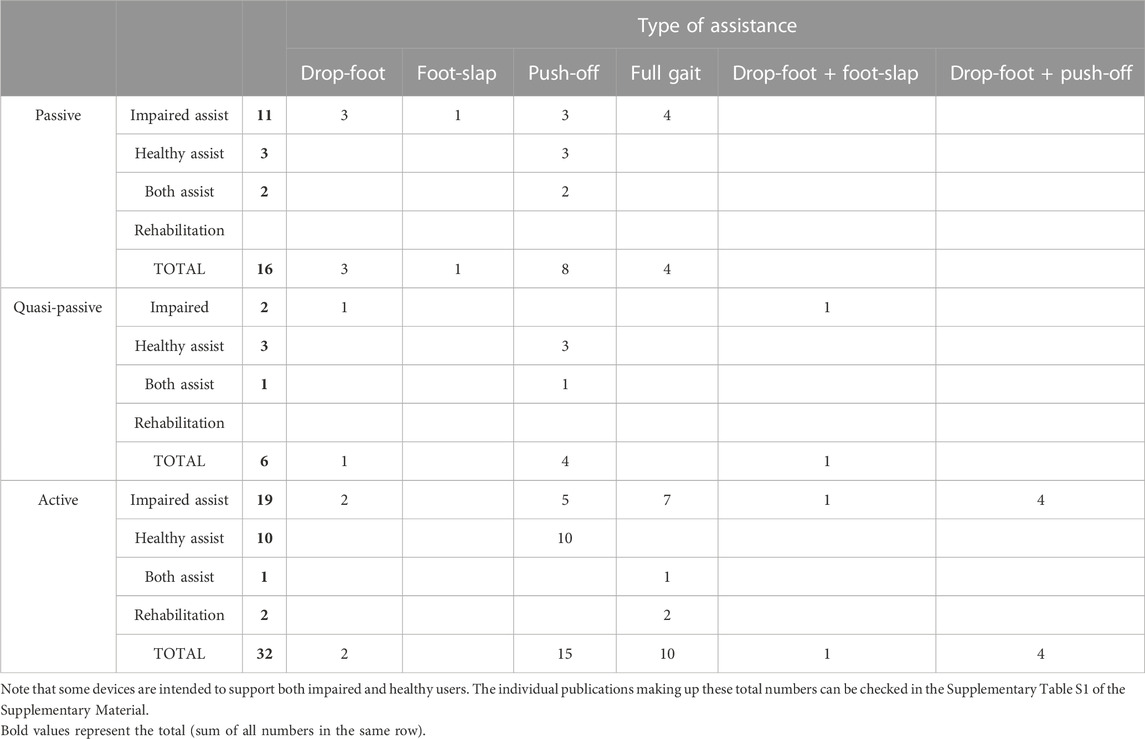
TABLE 1. Distribution of type of assistance and purpose (empowerment/assistive or rehabilitation) across passive, quasi-passive and active devices.
Assisting paretic ankle functions is the most common objective of AAFOs, being the purpose of 36 out of 54 reviewed devices (considering passive, quasi-passive, and active AAFOs). The main difference between devices’ approaches relies on the strategy adopted to assist the impaired walking. Some devices (12 out of 36) were designed to provide assistance during the whole gait cycle, e.g., (Bae et al., 2018a; Meyns et al., 2020). The rest of them were aimed to assist concrete gait functions, thus focusing on push-off [11 out of 36, e.g., (Takahashi et al., 2015; Leclair et al., 2018)], drop-foot [6 out of 36, e.g., (Rodriguez et al., 2018; Kim et al., 2020)] or foot-slap [1 of 36, e.g., (Kim et al., 2011; Yamamoto et al., 2022)]. Other authors assisted more than one gait function at the same time, for instance, drop-foot and foot-slap [2 out of 36, e.g., (Kim et al., 2011)] or drop-foot and push-off [4 out of 36, e.g., (Xia et al., 2020)].
When authors pursued to improve healthy performance (20 out of 54), they always opted for complementing the push-off function of the ankle, regardless whether they followed passive, e.g., (Yandell et al., 2019; Wang et al., 2020), quasi-passive, e.g., (Wang C. et al., 2022) or active approaches, e.g., (Mooney and Herr, 2016; Koller et al., 2017).
Finally, some AAFOs were designed to be used for rehabilitation practices. This objective represents the least common purpose, being only the function of 2 out of 54 devices (Li et al., 2011; Yeung et al., 2021). These two devices are active AAFOs designed under the rigid transmission paradigm. They are able to act over the whole gait cycle, actuating in both dorsi- and plantarflexion movements.
5 Human-robot-environment interaction
5.1 Adaptability of the devices
Adapting to different gait phases, speeds, and terrains is of a great significance for any AAFO. Limiting these adaptations would imply a reduced versatility of the device as well as a hindrance towards the user’s improvement. Moreover, adaptability of the AAFOs does not only refer to adaptations to the environment or walking condition, but also the required adaptation to the user’s progression while using the device.
Passive AAFOs that do not utilize clutches have inherent limitations in terms of adaptability, as the open-loop function of passive elements limits their ability to adapt to different walking conditions and tasks. These passive devices are not capable of providing assistance to either dorsi- or plantarflexion without hindering the other. The problem is resolved to some extent by using body-weight dependent clutches [e.g., (Yandell et al., 2019; van Hoorn et al., 2022)]. These types of clutches can detect the switch between different phases of gait regardless of the speed of the user, but their effectiveness has not been tested yet when walking over different terrains.
Quasi-passive AAFOs can more accurately switch the function of the passive elements to better react to changes in gait phases (Wang C. et al., 2022). Some of them can adjust the stiffness of the AAFO joint to better match the stiffness of the biological ankle (Allen et al., 2021), or directly adjust the assistance provided by the device on the subject (Diller et al., 2016; Oba et al., 2019; Allen et al., 2021).
Finally, active AAFOs are able to control dorsi- and plantarflexion separately, thereby, they offer more possibilities for adaptation irrespective of whether their main purpose is to support drop-foot [e.g., (Blaya and Herr, 2004; Awad et al., 2017b; Kim et al., 2020)], foot-slap [e.g., (Kim et al., 2011; Choi et al., 2018; Chen B. et al., 2022)], push-off [e.g., (Ward et al., 2011; Choi et al., 2018; Acosta-Sojo and Stirling, 2022)] or full gait [e.g., (Lerner et al., 2018; Siviy et al., 2020; de Miguel-Fernandez et al., 2022b)]. In fact, apart of being able to adapt to walking velocities, they also have the ability to control their actuator to be active during some phases of the gait and transparent during other phases (Bayón et al., 2022).
5.2 Effects on users
Regarding the subjects involved in the experiments, three main validation strategies have been reported across the reviewed articles (Figure 4). Some authors just reported technical validations regarding the proper functioning of the device and the assistance generated in terms of force and torque [e.g., (Diller et al., 2016; Choi et al., 2018; Leclair et al., 2018)] but without directly testing in subjects. When subjects were involved in the experiment, we can distinguish between those that involved healthy [e.g., (Malcolm et al., 2018; Oba et al., 2019; Wang et al., 2020)] or impaired subjects [e.g., (Sekiguchi et al., 2020; Fang et al., 2022)]. Remarkably, the AAFOs considered in this manuscript are more extensively validated with healthy than with impaired walking subjects. This may be due to two main reasons: 1) some AAFOs are directly developed to empower healthy subjects and increase their endurance while walking; 2) healthy subjects are considered as a first step for the validation of AAFOs, even if they are designed to assist paretic patients.
When reporting validations with subjects, authors focused on the assessment of three main aspects: 1) the lower-limbs kinematics, related to functional aspects of gait; 2) the muscular adaptations that occur when users received the assistance provided by the AAFO; and 3) the metabolic cost of walking.
5.2.1 Effects on kinematics
Kinematic analysis is the most common assessment when impaired walking subjects are involved (17 out of 22 validations with impaired subjects reported it, Figure 4). Although quasi-passive AAFOs have not been validated in this sense, passive and active devices have shown their feasibility in assisting and improving impaired gait kinematics. Reported results included improvements of the ROM, e.g., (Blaya and Herr, 2004; Wren et al., 2015; Kim et al., 2020; Sekiguchi et al., 2020), reduction of gait deficits such as drop-foot, e.g., (Roy et al., 2013; Yeung et al., 2017), foot-slap, e.g., (Kim et al., 2011), or inter-limb asymmetry, e.g., (Bae et al., 2015; Awad et al., 2017b). Additionally, others also showed kinetics effects as an increase of the torque on the paretic side (McCain et al., 2019).
Remarkably, kinematics are being less assessed in evaluations with healthy subjects (not impaired), only accounting for 20 out of 52 of the reported validations with humans (Figure 4). Those that performed kinematic analysis in healthy subjects focused on increasing gait speed (Slade et al., 2022) or stability (Bayón et al., 2022; Gonzalez et al., 2022), for example.
5.2.2 Effects on EMG
Effects in muscular activity is the most common outcome reported when healthy subjects are involved in the experiment (21 out of 52 validations, Figure 4). From these reported assessments, 5 out of 21 were with passive devices, 3 out of 21 with quasi-passive, and 13 out of 21 with active. Generally, the evaluations using EMG with healthy reported a reduction of activity of muscles involved in the ankle movement [e.g., soleus (Yandell et al., 2019; Chen J. et al., 2022), gastrocnemius (Bougrinat et al., 2019; Acosta-Sojo and Stirling, 2022) or tibialis anterior (Rodriguez et al., 2018; Weerasingha et al., 2018)].
When impaired subjects were involved, EMG effects were reported only for active devices [e.g., (Orekhov et al., 2020; Fang and Lerner, 2021)], with main reductions in soleus activity.
5.2.3 Effects on energy expenditure
Several AAFOs have been focused on increasing the endurance and reducing the mechanical power of healthy or impaired subjects while walking (Mooney and Herr, 2016; Koller et al., 2017). In this sense, the most common assessment is to evaluate the metabolic consumption of the user walking while being assisted by the tested AAFO.
When healthy subjects were involved, the energy expenditure was assessed for all kinds of devices [e.g., passive (Collins et al., 2015), quasi-passive (Wang C. et al., 2022) and active (Shepherd et al., 2022)]. Irrespective of the device used, a reduction in the metabolic cost of walking was observed in most cases. However, when assisting impaired subjects, this evaluation was only done for active AAFOs (Jackson and Collins, 2019; Orekhov et al., 2020). Also, these studies reported a reduction in the energy consumption.
6 Discussion
In this manuscript, passive, quasi-passive, and active AAFOs have been identified and further categorized based on their working principle and mechanical configuration. As a result, three clusters were created to enclose passive devices, one for quasi-passive devices and four for active devices. Additionally, we discussed the possibilities of these devices to provide assistance, to adapt to the environment, and their effects when being tested with final users.
There is an extreme difficulty in quantitatively comparing devices’ performances and the effectiveness of the different solutions among each other. The primary reason is the varying and limited number of test subjects involved in the evaluations. The majority of designs have shown their effects only on a small number of healthy subjects, and evaluations on impaired subjects are even more scarce. Moreover, researchers do not have a defined consensus or a set of criteria for evaluating their devices following similar validation procedures, which makes it even more challenging to quantitatively compare the performances. Therefore, we focus here on discussing differences in designs and preferred devices’ applications, identifying the advantages and disadvantages of each cluster individually, and proposing a set of criteria that would be useful to consider in the future to ensure consistent evaluations.
6.1 Design-related advantages and disadvantages
AAFOs are always intended to assist ankle motion based on the specific user’s needs, which is done by the provision of assistive ankle torques. The main reason to select an AAFO design over others is the ability of the device to tailor the assistance to address those specific needs.
The principal design-related benefit of passive and quasi-passive devices compared with active ones is their reduced weight, as they do not use heavy actuators or batteries. From these, ADR-AAFOs are the passive devices closer to the market. They support the ankle during the entire gait cycle, providing bidirectional torques by means of passive springs (Ultraflex Systems, 2009; Becker Orthopedic, 2018; Ottobock, 2018; Fior and Gentz, 2021). However, these devices are reported to be still bulky, and it is difficult to adjust their spring stiffness to the specific user’s pathological gait (Kerkum et al., 2014; Kobayashi et al., 2017). Moreover, the fact of providing a constant stiffness along the whole gait cycle without a dedicated timing for the storage and release of energy, may hinder the subject’s residual capabilities. This, together with the lack of evidence that proves their ability to adapt to different scenarios and mobility tasks encountered in everyday life, can be considered as the big limitations for these solutions (Kerkum et al., 2015; Firouzeh et al., 2021; Bayón et al., 2023).
Spring-cam passive designs (Rodriguez et al., 2018; Sekiguchi et al., 2020) tried to introduce a concrete benefit over the ADRs: the possibility of customizing a non-linear torque-angle curve depending on the user’s needs. This advantage allows the adoption of alternative tailored designs to counteract for diverse pathological gaits and the allowance of some adaptation to different environments and settings. Although still lighter than active solutions, these devices generally are a bit heavier than other passive ones like the ADRs.
With the appearance of passive clutch-dependent devices (Hirai et al., 2006; Collins et al., 2015; Leclair et al., 2018; Pardoel and Doumit, 2019; Yandell et al., 2019; Wang et al., 2020; Liu et al., 2021; van Hoorn et al., 2022) researchers made the next effort to gain more control over the timing of the delivered assistance within the gait cycle. This potential benefit has been further exploited by quasi-passive AAFOs with the aim of reaching higher torque levels and kinematic compatibility than with passive ones (Zhang et al., 2015; Dezman et al., 2018; Kumar et al., 2020; Wang C. et al., 2022). The shortcoming for clutch-based solutions is that normally, they provide assistance only on a single direction (plantarflexion or dorsiflexion).
Still, (quasi) passive devices in general have not been extensively tested to prove their good performance in adapting to different users and walking terrains. On the contrary, active AAFOs have the major benefit of being able to provide large levels of assistance fully controllable in amplitude and timing, which enables them to adapt to different velocities or varying ground conditions (Shorter et al., 2011; Yeung et al., 2017; Lerner et al., 2018; Huo et al., 2019; Meijneke et al., 2021; Chen J. et al., 2022). However, their application is restricted to the performance of their controllers and actuators. For example, most of the devices that have a rigid transmission use position control rather than torque control. These devices are able to precisely control the final kinematics of the device, and therefore of the joint, but without considering the exerted torque on the subject, which can be dangerous if it is not properly controlled. They are mainly applied to patients who do not have control over their limbs and who are not able to oppose the movement (Choi et al., 2018; Weerasingha et al., 2018; Huo et al., 2019; Lee et al., 2021). To alleviate this strict position tracking, some researchers used impedance control to allow some deviation from predefined trajectories (Lee et al., 2021) or by using low-transmission brushless DC motors, which have low back-drivability, allowing users to have safer interaction with the AAFO (de Miguel-Fernandez et al., 2022a; de Miguel-Fernandez et al., 2022b; Nabipour and Moosavian, 2021).
The stiffer the actuator, the more delicate and accurate the controller should be to track the user’s motion or intention. Therefore, most of the active devices use compliant actuation that decreases the demands of the controllers due to the inherent adjustable behavior of the actuator. Starting from the geared actuator, the straightforward compliant system is formed by adding an elastic element, i.e., a spring, in series with the actuator (Kim et al., 2011; van Dijk et al., 2017; Durandau et al., 2022). These SEA actuators reduce the restriction imposed by the low back-drivability of the rigid transmission, being a compromise solution between maximal torque and weight that can fit well to assist impaired patients. However, depending on the device implementation, their size and bulkiness could not be yet suitable for daily use, so improving these is an open challenge.
An important issue about the assistance provided by active AAFOs is the strategy followed to deliver a timed assistance without disturbing the subject. In this sense, non-compliant devices with a rigid power transmission can only rely on control strategies that synchronize the subject’s gait with the action of the AAFO. However, the nature of compliant mechanisms allows for a slight absorption of the hampering effect of imprecise controllers, which permits the transparent behaviour of the AAFO when the assistive torque is not given.
Devices based on PAMs are characterized by the best compromise between exerted force and lightweight (Kinnaird and Ferris, 2009; Koller et al., 2015; Galle et al., 2017). However, their slow dynamics only allow their use in subjects walking or moving at a slow velocity, i.e., impaired or elderly subjects. In addition, these devices are limited by their necessity of a compressed air supply to power them, which constrains their application to assist daily life activities.
Finally, AAFOs driven by cables have the potential of being the most lightweight active approach (Bae et al., 2018a; McCain et al., 2019; Slade et al., 2022). By placing the actuator in a proximal location (mostly around the pelvis), the extra inertia added to the distal segment of the limb is reduced. This feature would enable them to be used in daily life scenarios, although they have not been extensively tested in such conditions. As a drawback, cable-driven AAFOs are only able to assist the motion in the traction direction of the cable, normally the plantarflexion direction of the ankle. This would prevent the assistance during the full gait cycle, but could be enough to assist certain gait functions such as push-off. Alternatively, by adding more than one cable and actuator, the device could assist both dorsi- and plantarflexion (Rewalk Robotics, 2023).
6.2 Primary goals with AAFOs when assisting gait
By analyzing the experimental validations performed with the reviewed AAFOs (Figure 4), we can conclude that active devices have aroused more interest in the scientific community, dealing with a greater number of related articles. Moreover, none of the reviewed quasi-passive AAFOs have been finally validated with impaired subjects, even when they were designed to do so. This fact may imply that the readiness-level of quasi-passive solutions is lower than for purely passive or active devices and, therefore, further research effort should be done before this technology can be used in real-life scenarios by people with impairments.
The push-off assistance is the preferred goal for all three AAFOs categories (i.e., passive, quasi-passive, and active). In most cases, the target populations for this type of support are healthy (empowerment), or aging and people who suffer from muscle weaknesses (assistance). Most of these devices have only been validated with experiments on healthy subjects [except (Sekiguchi et al., 2020) for passive, and (Ward et al., 2011; Takahashi et al., 2015; Awad et al., 2017a; McCain et al., 2019; Tamburella et al., 2020; Gomez-Vargas et al., 2021; Harvey et al., 2021) for active]. A reason for the limited evaluation might be that most of these devices require subjects with good balance capabilities as the devices do not normally account for that. For future use of these devices in populations with impairments it is key that they account for balance loss, assisting the user in that regard, or at least not being detrimental.
In the case of passive and quasi-passive devices, the capacity to support push-off is normally assessed by reductions in muscular activity. All the reviewed (quasi) passive studies reported reductions of plantarflexors muscular activity. However, dorsiflexors like the tibialis anterior, were normally not assessed or even showed an increase of activity (Collins et al., 2015; Wang C. et al., 2022). Only (Kumar et al., 2020) reported a reduction in tibialis anterior and soleus at the same time, but their validation was only performed on a single healthy subject. These results suggest that extensive validations are still required for these devices.
Regarding active AAFOs, assisting push-off is the preferred objective for most cable-driven and PAM configurations. In this case, the validations focused on reducing the metabolic cost of walking in healthy subjects and improving kinematics and kinetics for paretic ankle propulsion. Based on these metrics, both approaches appeared to be valid alternatives that achieved their assistive purpose.
After push-off, the most common assistance is full-gait. For passive devices, ADRs and spring-cam are the approaches that allow for a double dorsi- and plantarflexion support (Kerkum et al., 2014; Wren et al., 2015; Kobayashi et al., 2017) but have their limitations. As mentioned before, the assistance provided is not timed within the gait cycle. This implies that instead of assisting the residual capabilities of the subject to perform a specific ankle motion, they provide stability while might restrict motion. Most of the ADR-AAFOs were tested with impaired subjects and some qualitative improvements in subjects’ kinematics were reported. Nevertheless, no quantitative extensive discussion over their effects on the users is published (e.g., to evaluate if the added mass of the device to provoke those improvements is justified). Therefore, in spite of these promising results, there is not solid evidence of their benefits when assisting the complete gait cycle.
Active AAFOs offer more possibilities to assist full gait but for some mechanical designs, this comes at the cost of a double configuration. Only for the usually employed SEAs or rigid transmission the actuation provided in both dorsi- and plantarflexion is intrinsic to their design. However, PAM-based or cable-driven devices could also allow for that following a double configuration, with the actuator placed in parallel to both the agonist and antagonist muscle groups. In spite of this potential solution that would extend the capabilities of these groups of devices, the double configuration has only been explored by cable-driven AAFOs [e.g., (Awad et al., 2017b; Gomez-Vargas et al., 2021; Rewalk Robotics, 2023)].
6.3 Comprehensive analysis and need for extensive evaluations
As mentioned earlier, due to the lack of consensus in the used experimental procedures between studies, it is very difficult to find common reported criteria for an objective comparison of the performance of different AAFOs.
When addressing the functional performance of their device, technological indicators that describe the physical capability of the AAFO are normally provided. In that regard, researchers tried to report results on, for example, joint kinematics, spatio-temporal parameters, interaction forces, adaptability to walking speed, and effects on muscular activity or energy consumption. However, not all these topics were addressed for all devices, and even more importantly, the topics contemplated were assessed following different methodologies or with different target populations. Therefore and in order to have a fair quantitative comparison, it is essential to make sets of bench-marking criteria that researchers can follow to evaluate their devices, which is the approach also proposed by Torricelli et al. (2020).
Additionally, there is also a lack of evaluations of the devices in diverse conditions that are decisive for representing daily-life situations. Currently, devices are mostly evaluated during flat overground walking, treadmill, or just technically. Experiments on non-standardized settings like uneven terrains, when maneuvering around, or just start and stop gait for instance, have not been sufficiently discussed. Thus, although it is possible to hypothesize, for example, that AAFOs with body-weight dependent clutches are superior to spring-cam or angle-dependent clutches in providing assistance while walking on a slope, no evidence is available for proving this claim.
The effect of the AAFOs on users’ balance or user’s safety further than locomotion support are important aspects that are scarcely considered in the literature. There are some studies with active AAFOs that investigated the capacity of the device on improving balance capabilities of the users (Bayón et al., 2022; Gonzalez et al., 2022), but they were not focused on assessing the mechanical configuration of the orthosis itself, but on evaluating a specific controller [except (Harvey et al., 2021)].
Finally, the users’ perceived experiences in terms of perceptual, emotional and cognitive aspects are also barely reported. A reason for that might be the small number of studies and evaluations with human beings at long term, which limits the inclusion of questionnaires or human-in-the-loop experiments with variations of the technical device that report experiences of final-users. These reports would be very useful, for example, to assess the perceived comfort, control (embodiment and agency) and required effort when using an specific AAFO.
Based on the above-mentioned points, the advantages and disadvantages reported in Section 6.1, and the data found on the literature (see Supplementary Table S2 in the Supplementary Material), we provide a subjective analysis of the clusters created in this article in terms of the readiness level we think they have in terms of functional performance and user experience (Tables 2, 3 respectively). The features included in these tables are categorized using the framework of (Torricelli et al., 2020), and can also be used as a potential set of criteria that researchers can utilize when developing new devices in order to ensure a future consistent evaluation.
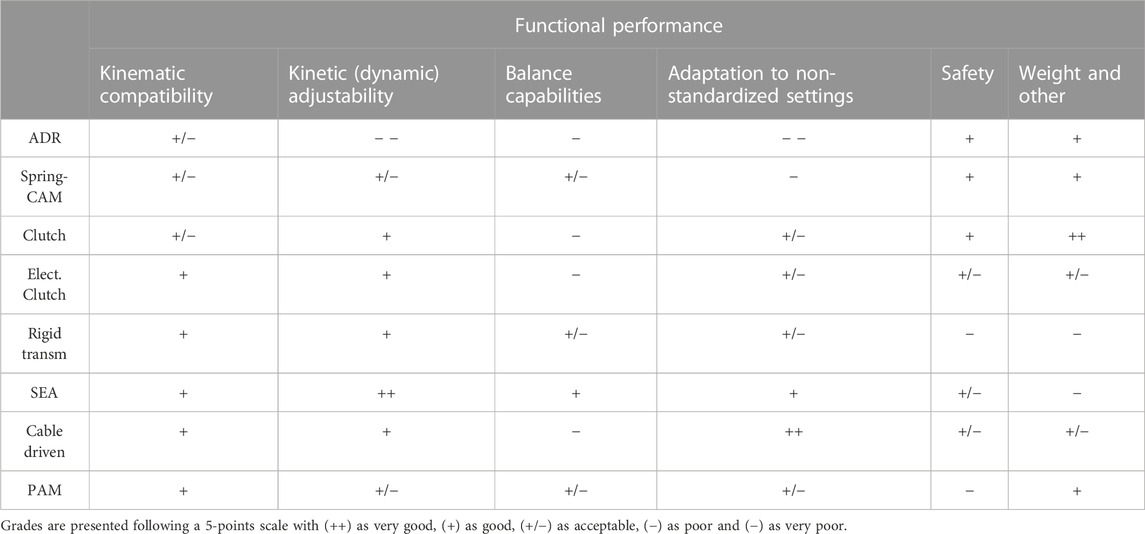
TABLE 2. Features for functional performance and subjective evaluation of readiness level for each cluster.
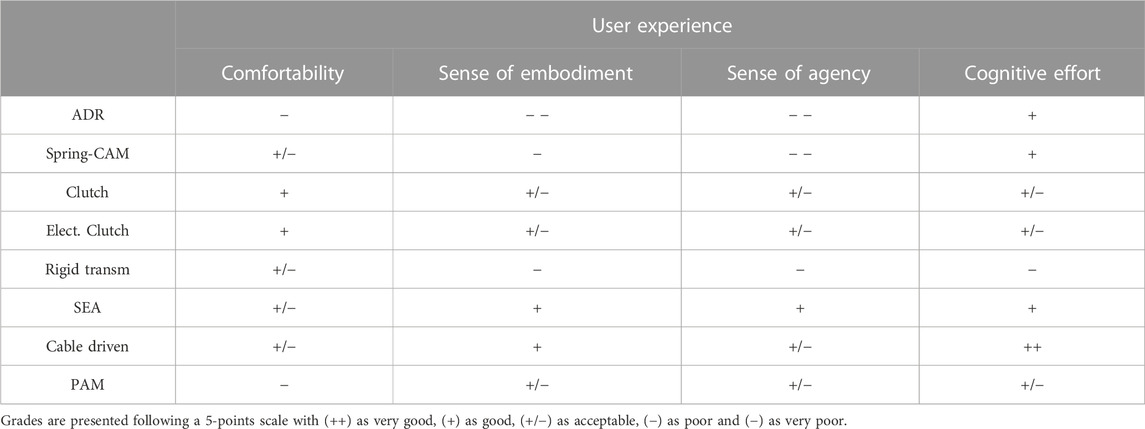
TABLE 3. Features for perceived users’ experiences with the AAFOs and subjective evaluation of readiness level for each cluster.
6.4 Future designs
There have been quite some advances in passive AAFOs in the last years, but still these devices are not robustly prepared to address all kinds of situations encountered in everyday life. Started from less than 10 years ago, quasi-passive solutions represent a compromise between passive and active devices, resulting in an increasing trend that opted for using low electrical power to activate clutches. A trend for these devices (started in 2016) was to use other types of actuation rather than just springs and dampers for applying the assistance. The electroadhesive and dielectric elastomer actuators are examples of this. However, most of the passive and quasi-passive approaches have the limitation of providing assistance only towards dorsi- or plantarflexion, but not both. A groundbreaking step for (quasi) passive designs would be to have a device able to provide a configurable assistance on both directions with minor modifications.
Active AAFOs have the potential of being fully controllable to assist several gait functions with a single design. These devices are now evolving to lighter and more compliant solutions that should be transparent for the user while they are not providing assistance. Following the tendency of passive devices to assist activities of daily living, active AAFOs should also be tested in unrestricted environments to assess their performance when assisting gait under more challenging and rapidly varying conditions (variations of terrain and gait velocity).
Author contributions
JL-M and MN performed the literature review, collected the information, drafted and wrote the manuscript. EA revised the manuscript and made substantial comments. CB designed the conceptualization and design of the study, contributed with the literature review, collected the information, drafted, wrote and reviewed the manuscript. All authors contributed to the article and approved the submitted version.
Funding
This work has been carried out with the financial support from the Dutch Research Council NWO, under the grant Veni-TTW-2020 with ref. number 18079, and partly with the financial support from the KNAW Ter Meulen grant of the Academy Medical Sciences Fund, Royal Netherlands Academy of Arts and Sciences, ref. number KNAWWF/1327/TMB202101.
Conflict of interest
The authors declare that the research was conducted in the absence of any commercial or financial relationships that could be construed as a potential conflict of interest.
Publisher’s note
All claims expressed in this article are solely those of the authors and do not necessarily represent those of their affiliated organizations, or those of the publisher, the editors and the reviewers. Any product that may be evaluated in this article, or claim that may be made by its manufacturer, is not guaranteed or endorsed by the publisher.
Supplementary material
The Supplementary Material for this article can be found online at: https://www.frontiersin.org/articles/10.3389/fbioe.2023.1188685/full#supplementary-material
References
Acosta-Sojo, Y., and Stirling, L. (2022). Individuals differ in muscle activation patterns during early adaptation to a powered ankle exoskeleton. Appl. Ergon. 98, 103593. doi:10.1016/j.apergo.2021.103593
Alam, M., Choudhury, I. A., and Mamat, A. B. (2014). Mechanism and design analysis of articulated ankle foot orthoses for drop-foot. Sci. World J. 2014, 1–14. doi:10.1155/2014/867869
Allen, D. P., Little, R., Laube, J., Warren, J., Voit, W., and Gregg, R. D. (2021). Towards an ankle-foot orthosis powered by a dielectric elastomer actuator. Mechatronics 76, 102551. doi:10.1016/J.MECHATRONICS.2021.102551
Armand, S., Decoulon, G., and Bonnefoy-Mazure, A. (2016). Gait analysis in children with cerebral palsy. EFORT Open Rev. 1, 448–460. doi:10.1302/2058-5241.1.000052
Awad, L. N., Bae, J., Kudzia, P., Long, A., Hendron, K., Holt, K. G., et al. (2017a). Reducing circumduction and hip hiking during hemiparetic walking through targeted assistance of the paretic limb using a soft robotic exosuit. Am. J. Phys. Med. Rehabil. 96, S157–S164. doi:10.1097/PHM.0000000000000800
Awad, L. N., Bae, J., O’Donnell, K., De Rossi, S. M. M., Hendron, K., Sloot, L. H., et al. (2017b). A soft robotic exosuit improves walking in patients after stroke. Sci. Transl. Med. 9, eaai9084. doi:10.1126/scitranslmed.aai9084
Bae, J., De Rossi, S. M. M., O’Donnell, K., Hendron, K. L., Awad, L. N., Teles Dos Santos, T. R., et al. (2015). “A soft exosuit for patients with stroke: Feasibility study with a mobile off-board actuation unit,” in IEEE International Conference on Rehabilitation Robotics. 11–14. doi:10.1109/ICORR.2015.7281188
Bae, J., Awad, L. N., Long, A., O’Donnell, K., Hendron, K. L., Holt, K. G., et al. (2018a). Biomechanical mechanisms underlying exosuit-induced improvements in walking economy after stroke. J. Exp. Biol. 221, jeb168815. doi:10.1242/jeb.168815
Bae, J., Siviy, C., Rouleau, M., Menard, N., Odonnell, K., Geliana, I., et al. (2018b). “A lightweight and efficient portable soft exosuit for paretic ankle assistance in walking after stroke,” in 2018 IEEE International Conference on Robotics and Automation (ICRA) (IEEE), 2820–2827. doi:10.1109/ICRA.2018.8461046
Baud, R., Manzoori, A. R., Ijspeert, A., and Bouri, M. (2021). Review of control strategies for lower-limb exoskeletons to assist gait. J. NeuroEng. Rehabil. 18, 119. doi:10.1186/s12984-021-00906-3
Bayón, C., Keemink, A. Q. L., van Mierlo, M., Rampeltshammer, W., van der Kooij, H., and van Asseldonk, E. H. F. (2022). Cooperative ankle-exoskeleton control can reduce effort to recover balance after unexpected disturbances during walking. J. NeuroEng. Rehabil. 19, 21. doi:10.1186/s12984-022-01000-y
Bayón, C., van Hoorn, M., Barrientos, A., Rocon, E., Trost, J. P., and van Asseldonk, E. H. F. (2023). Perspectives on ankle-foot technology for improving gait performance of children with cerebral palsy in daily-life: Requirements, needs and wishes. J. NeuroEng. Rehabil. 20, 44. doi:10.1186/s12984-023-01162-3
Beyaert, C., Vasa, R., and Frykberg, G. E. (2015). Gait post-stroke: Pathophysiology and rehabilitation strategies. Neurophysiol. Clin. 45, 335–355. doi:10.1016/j.neucli.2015.09.005
Blaya, J., and Herr, H. (2004). Adaptive control of a variable-impedance ankle-foot orthosis to assist drop-foot gait. IEEE Trans. Neural Syst. Rehabil. Eng. 12, 24–31. doi:10.1109/TNSRE.2003.823266
Bougrinat, Y., Achiche, S., and Raison, M. (2019). Design and development of a lightweight ankle exoskeleton for human walking augmentation. Mechatronics 64, 102297. doi:10.1016/j.mechatronics.2019.102297
Brockett, C. L., and Chapman, G. J. (2016). Biomechanics of the ankle. Orthop. Trauma 30, 232–238. doi:10.1016/J.MPORTH.2016.04.015
Cain, S. M., Gordon, K. E., and Ferris, D. P. (2007). Locomotor adaptation to a powered ankle-foot orthosis depends on control method. J. NeuroEng. Rehabil. 4, 48–13. doi:10.1186/1743-0003-4-48
Chen, B., Zi, B., Zhou, B., and Wang, Z. (2022a). Implementation of robotic ankle-foot orthosis with an impedance-based assist-as-needed control strategy. J. Mech. Robotics 14, 1–12. doi:10.1115/1.4053218
Chen, J., Han, J., and Zhang, J. (2022b). Design and evaluation of a mobile ankle exoskeleton with switchable actuation configurations. IEEE/ASME Trans. Mechatronics 27, 1846–1853. doi:10.1109/TMECH.2022.3175731
Choi, H., Park, Y. J., Seo, K., Lee, J., Lee, S. E., and Shim, Y. (2018). A multifunctional ankle exoskeleton for mobility enhancement of gait-impaired individuals and seniors. IEEE Robotics Autom. Lett. 3, 411–418. doi:10.1109/LRA.2017.2734239
Choo, Y. J., and Chang, M. C. (2021). Commonly used types and recent development of ankle-foot orthosis: A narrative review. Healthc. Switz. 9, 1046. doi:10.3390/healthcare9081046
Collins, S. H., Bruce Wiggin, M., and Sawicki, G. S. (2015). Reducing the energy cost of human walking using an unpowered exoskeleton. Nature 522, 212–215. doi:10.1038/nature14288
Conner, B. C., Orekhov, G., and Lerner, Z. (2021). Ankle exoskeleton assistance increases six-minute walk test performance in cerebral palsy. IEEE Open J. Eng. Med. Biol. 2, 320–323. doi:10.1109/ojemb.2021.3135826
Conner, B. C., Remec, N. M., Michaels, C. M., Wallace, C. W., Andrisevic, E., and Lerner, Z. F. (2022). Relationship between ankle function and walking ability for children and young adults with cerebral palsy: A systematic review of deficits and targeted interventions. Gait Posture 91, 165–178. doi:10.1016/j.gaitpost.2021.10.024
de Miguel-Fernandez, J., Mesa-Garrido, A., Pescatore, C., Rikhof, C., Lobo-Prat, J., and Font- Llagunes, J. M. (2022a). Relationship between ankle assistive torque and biomechanical gait metrics in individuals after stroke. preprint at TechRxiv, 0–9. doi:10.36227/techrxiv.20749789.v1
de Miguel-Fernandez, J., Pescatore, C., Mesa-Garrido, A., Rikhof, C., Prinsen, E., Font-Llagunes, J. M., et al. (2022b). Immediate biomechanical effects of providing adaptive assistance with an ankle exoskeleton in individuals after stroke. IEEE Robotics Autom. Lett. 7, 7574–7580. doi:10.1109/LRA.2022.3183799
Dezman, M., Babic, J., and Gams, A. (2018). “Qualitative assessment of a clutch-actuated ankle exoskeleton,” in International Conference on Robotics in Alpe-Adria Danube Region. doi:10.1007/978-3-319-61276-8_82
Diller, S., Majidi, C., and Collins, S. H. (2016). “A lightweight, low-power electroadhesive clutch and spring for exoskeleton actuation,” in IEEE International Conference on Robotics and Automation.
Durandau, G., Rampeltshammer, W. F., van der Kooij, H., and Sartori, M. (2022). Neuromechanical model-based adaptive control of bilateral ankle exoskeletons: Biological joint torque and electromyogram reduction across walking conditions. IEEE Trans. Robotics 38, 1380–1394. doi:10.1109/TRO.2022.3170239
Dzeladini, F., Wu, A. R., Renjewski, D., Arami, A., Burdet, E., van Asseldonk, E., et al. (2016). “Effects of a neuromuscular controller on a powered ankle exoskeleton during human walking,” in 2016 6th IEEE International Conference on Biomedical Robotics and Biomechatronics (BioRob) (IEEE), 617–622. doi:10.1109/BIOROB.2016.7523694
Fang, Y., and Lerner, Z. F. (2021). Feasibility of augmenting ankle exoskeleton walking performance with step length biofeedback in individuals with cerebral palsy. IEEE Trans. Neural Syst. Rehabil. Eng. 29, 442–449. doi:10.1109/TNSRE.2021.3055796
Fang, Y., and Lerner, Z. F. (2022). Bilateral vs. Paretic-Limb-Only ankle exoskeleton assistance for improving hemiparetic gait: A case series. IEEE Robotics Autom. Lett. 7, 1246–1253. doi:10.1109/LRA.2021.3139540
Fang, Y., Orekhov, G., and Lerner, Z. F. (2022). Improving the energy cost of incline walking and stair ascent with ankle exoskeleton assistance in cerebral palsy. IEEE Trans. Biomed. Eng. 69, 2143–2152. doi:10.1109/TBME.2021.3137447
Fior and Gentz (2021). NEURO SWING ankle joints – dynamic balance and stability. Tech. rep. Fior and Gentz.
Firouzeh, P., Sonnenberg, L. K., Morris, C., and Pritchard-Wiart, L. (2021). Ankle foot orthoses for young children with cerebral palsy: A scoping review. Disabil. Rehabil. 43, 726–738. doi:10.1080/09638288.2019.1631394
Forrester, L. W., Roy, A., Hafer-Macko, C., Krebs, H. I., and Macko, R. F. (2016). Task-specific ankle robotics gait training after stroke: A randomized pilot study. J. NeuroEng. Rehabil. 13, 51. doi:10.1186/s12984-016-0158-1
Galle, S., Malcolm, P., Derave, W., and De Clercq, D. (2013). Adaptation to walking with an exoskeleton that assists ankle extension. Gait Posture 38, 495–499. doi:10.1016/j.gaitpost.2013.01.029
Galle, S., Malcolm, P., Derave, W., and De Clercq, D. (2014). Enhancing performance during inclined loaded walking with a powered ankle–foot exoskeleton. Eur. J. Appl. Physiology 114, 2341–2351. doi:10.1007/s00421-014-2955-1
Galle, S., Malcolm, P., Derave, W., and De Clercq, D. (2015). Uphill walking with a simple exoskeleton: Plantarflexion assistance leads to proximal adaptations. Gait Posture 41, 246–251. doi:10.1016/j.gaitpost.2014.10.015
Galle, S., Malcolm, P., Collins, S. H., and De Clercq, D. (2017). Reducing the metabolic cost of walking with an ankle exoskeleton: Interaction between actuation timing and power. J. NeuroEng. Rehabil. 14, 35–16. doi:10.1186/s12984-017-0235-0
Gasparri, G. M., Luque, J., and Lerner, Z. F. (2019). Proportional joint-moment control for instantaneously adaptive ankle exoskeleton assistance. IEEE Trans. Neural Syst. Rehabil. Eng. 27, 751–759. doi:10.1109/TNSRE.2019.2905979
Gomez-Vargas, D., Ballen-Moreno, F., Barria, P., Aguilar, R., Azorín, J. M., Munera, M., et al. (2021). The actuation system of the ankle exoskeleton T-FLEX: First use experimental validation in people with stroke. Brain Sci. 11, 412. doi:10.3390/brainsci11040412
Gonzalez, S., Stegall, P., Cain, S. M., Siu, H. C., and Stirling, L. (2022). Assessment of a powered ankle exoskeleton on human stability and balance. Appl. Ergon. 103, 103768. doi:10.1016/j.apergo.2022.103768
Gordon, K. E., and Ferris, D. P. (2007). Learning to walk with a robotic ankle exoskeleton. J. Biomechanics 40, 2636–2644. doi:10.1016/j.jbiomech.2006.12.006
Harvey, T. A., Conner, B. C., and Lerner, Z. F. (2021). Does ankle exoskeleton assistance impair stability during walking in individuals with cerebral palsy? Ann. Biomed. Eng. 49, 2522–2532. doi:10.1007/s10439-021-02822-y
Hirai, H., Ozawa, R., Goto, S., Fujigaya, H., Yamasaki, S., Hatanaka, Y., et al. (2006). “Development of an ankle-foot orthosis with a pneumatic passive element,” in IEEE International Workshop on Robot and Human Interactive Communication, 220–225. doi:10.1109/ROMAN.2006.314421
Huo, W., Arnez-Paniagua, V., Ding, G., Amirat, Y., and Mohammed, S. (2019). Adaptive proxy-based controller of an active ankle foot orthosis to assist lower limb movements of paretic patients. Robotica 37, 2147–2164. doi:10.1017/S0263574719000250
Jackson, R. W., and Collins, S. H. (2015). An experimental comparison of the relative benefits of work and torque assistance in ankle exoskeletons. J. Appl. Physiol. 119, 541–557. doi:10.1152/japplphysiol.01133.2014
Jackson, R. W., and Collins, S. H. (2019). Heuristic-based ankle exoskeleton control for Co-adaptive assistance of human locomotion. IEEE Trans. Neural Syst. Rehabil. Eng. 27, 2059–2069. doi:10.1109/TNSRE.2019.2936383
Kao, P.-C., Lewis, C. L., and Ferris, D. P. (2010). Invariant ankle moment patterns when walking with and without a robotic ankle exoskeleton. J. Biomechanics 43, 203–209. doi:10.1016/j.jbiomech.2009.09.030
Kerkum, Y. L., Brehm, M. A., Buizer, A. I., Van Den Noort, J. C., Becher, J. G., and Harlaar, J. (2014). Defining the mechanical properties of a spring-hinged ankle foot orthosis to assess its potential use in children with spastic cerebral palsy. J. Appl. Biomech. 30, 728–731. doi:10.1123/jab.2014-0046
Kerkum, Y. L., Buizer, A. I., Noort, J. C. V. D., Becher, J. G., Harlaar, J., and Brehm, M. A. (2015). The effects of varying ankle foot orthosis stiffness on gait in children with spastic cerebral palsy who walk with excessive knee flexion. PLoS ONE 10, e0142878. doi:10.1371/journal.pone.0142878
Kim, J., Hwang, S., Sohn, R., Lee, Y., and Kim, Y. (2011). Development of an active ankle foot orthosis to prevent foot drop and toe drag in hemiplegic patients: A preliminary study. Appl. Bionics Biomech. 8, 377–384. doi:10.1155/2011/530375
Kim, S. J., Na, Y., Lee, D. Y., Chang, H., and Kim, J. (2020). Pneumatic AFO powered by a miniature custom compressor for drop foot correction. IEEE Trans. Neural Syst. Rehabil. Eng. 28, 1781–1789. doi:10.1109/TNSRE.2020.3003860
Kinnaird, C. R., and Ferris, D. P. (2009). Medial gastrocnemius myoelectric control of a robotic ankle exoskeleton. IEEE Trans. Neural Syst. Rehabil. Eng. 17, 31–37. doi:10.1109/TNSRE.2008.2008285
Kobayashi, T., Orendurff, M. S., Hunt, G., Lincoln, L. S., Gao, F., LeCursi, N., et al. (2017). An articulated ankle–foot orthosis with adjustable plantarflexion resistance, dorsiflexion resistance and alignment: A pilot study on mechanical properties and effects on stroke hemiparetic gait. Med. Eng. Phys. 44, 94–101. doi:10.1016/j.medengphy.2017.02.012
Kobayashi, T., Orendurff, M. S., Hunt, G., Gao, F., LeCursi, N., Lincoln, L. S., et al. (2019). The effects of alignment of an articulated ankle-foot orthosis on lower limb joint kinematics and kinetics during gait in individuals post-stroke. J. Biomechanics 83, 57–64. doi:10.1016/j.jbiomech.2018.11.019
Koller, J. R., Jacobs, D. A., Ferris, D. P., and Remy, C. D. (2015). Learning to walk with an adaptive gain proportional myoelectric controller for a robotic ankle exoskeleton. J. Neuroeng. Rehabil. 12, 97. doi:10.1186/s12984-015-0086-5
Koller, J. R., David Remy, C., and Ferris, D. P. (2017). Comparing neural control and mechanically intrinsic control of powered ankle exoskeletons. IEEE Int. Conf. Rehabil. Robotics 294, 294–299. doi:10.1109/ICORR.2017.8009262
Kumar, S., Zwall, M. R., Bolívar-Nieto, E. A., Gregg, R. D., and Gans, N. (2020). Tibial nerve schwannoma: An unexplained cause of lateral foot pain - a rare case report and review. IEEE Robotics Autom. Lett. 5, 1–6. doi:10.13107/jocr.2020.v10.i09.1880
Leclair, J., Pardoel, S., Helal, A., and Doumit, M. (2018). Development of an unpowered ankle exoskeleton for walking assist. Disabil. Rehabil. Assistive Technol. 15, 1–13. doi:10.1080/17483107.2018.1494218
Lee, M., Kim, J., Hyung, S., Lee, J., Seo, K., Park, Y. J., et al. (2021). A compact ankle exoskeleton with a multiaxis parallel linkage mechanism. IEEE/ASME Trans. Mechatronics 26, 191–202. doi:10.1109/TMECH.2020.3008372
Lerner, Z. F., Gasparri, G. M., Bair, M. O., Lawson, J. L., Luque, J., Harvey, T. A., et al. (2018). An untethered ankle exoskeleton improves walking economy in a pilot study of individuals with cerebral palsy. IEEE Trans. Neural Syst. Rehabil. Eng. 26, 1985–1993. doi:10.1109/TNSRE.2018.2870756
Lerner, Z. F., Harvey, T. A., and Lawson, J. L. (2019). A battery-powered ankle exoskeleton improves gait mechanics in a feasibility study of individuals with cerebral palsy. Ann. Biomed. Eng. 47, 1345–1356. doi:10.1007/s10439-019-02237-w
Li, D. Y., Becker, A., Shorter, K. A., Bretl, T., and Hsiao-Wecksler, E. T. (2011). Estimating system state during human walking with a powered ankle-foot orthosis. IEEE/ASME Trans. Mechatronics 16, 835–844. doi:10.1109/TMECH.2011.2161769
Liu, L., Wei, W., Zheng, K., Diao, Y., Wang, Z., Li, G., et al. (2021). “Design of an unpowered ankle-foot exoskeleton used for walking assistance,” in 43rd Annual International Conference of the IEEE Engineering in Medicine and Biology Society (EMBC), 4501–4504.
Lora-Millan, J. S., Moreno, J. C., and Rocon, E. (2022). Coordination between partial robotic exoskeletons and human gait: A comprehensive review on control strategies. Front. Bioeng. Biotechnol. 10, 842294. doi:10.3389/fbioe.2022.842294
Malcolm, P., Galle, S., Van Den Berghe, P., and De Clercq, D. (2018). Exoskeleton assistance symmetry matters: Unilateral assistance reduces metabolic cost, but relatively less than bilateral assistance. J. NeuroEng. Rehabil. 15, 74. doi:10.1186/s12984-018-0381-z
McCain, E. M., Dick, T. J. M., Giest, T. N., Nuckols, R. W., Lewek, M. D., Saul, K. R., et al. (2019). Mechanics and energetics of post-stroke walking aided by a powered ankle exoskeleton with speed-adaptive myoelectric control. J. NeuroEng. Rehabil. 16, 57. doi:10.1186/s12984-019-0523-y
Meijneke, C., Van Dijk, W., and Van Der Kooij, H. (2014). “Achilles: An autonomous lightweight ankle exoskeleton to provide push-off power,” in Proceedings of the IEEE RAS and EMBS International Conference on Biomedical Robotics and Biomechatronics, 918–923. doi:10.1109/biorob.2014.6913898
Meijneke, C., van Oort, G., Sluiter, V., van Asseldonk, E., Tagliamonte, N. L., Tamburella, F., et al. (2021). Symbitron exoskeleton: Design, control, and evaluation of a modular exoskeleton for incomplete and complete spinal cord injured individuals. IEEE Trans. neural Syst. Rehabil. Eng. a Publ. IEEE Eng. Med. Biol. Soc. 29, 330–339. doi:10.1109/TNSRE.2021.3049960
Meyns, P., Kerkum, Y. L., Brehm, M. A., Becher, J. G., Buizer, A. I., and Harlaar, J. (2020). Ankle foot orthoses in cerebral palsy: Effects of ankle stiffness on trunk kinematics, gait stability and energy cost of walking. Eur. J. Paediatr. Neurology 26, 68–74. doi:10.1016/j.ejpn.2020.02.009
Moltedo, M., Baček, T., Verstraten, T., Rodriguez-Guerrero, C., Vanderborght, B., and Lefeber, D. (2018). Powered ankle-foot orthoses: The effects of the assistance on healthy and impaired users while walking. J. NeuroEng. Rehabil. 15, 86. doi:10.1186/s12984-018-0424-5
Mooney, L. M., and Herr, H. M. (2016). Biomechanical walking mechanisms underlying the metabolic reduction caused by an autonomous exoskeleton. J. NeuroEng. Rehabil. 13, 4. doi:10.1186/s12984-016-0111-3
Mooney, L. M., J, R. E., Herr, H. M., Rouze, E. J., and Herr, H. M. (2014a). Autonomous exoskeleton reduces metabolic cost of human walking during load carriage. J. NeuroEng. Rehabil. 11, 80. doi:10.1186/1743-0003-11-80
Mooney, L. M., Rouse, E. J., and Herr, H. M. (2014b). “Autonomous exoskeleton reduces metabolic cost of walking,” in 2014 36th Annual International Conference of the IEEE Engineering in Medicine and Biology Society (IEEE), 3065–3068. doi:10.1109/EMBC.2014.6944270
Moosavian, S. A. A., Nabipour, M., Absalan, F., and Akbari, V. (2021). RoboWalk: Augmented human-robot mathematical modelling for design optimization. Math. Comput. Model. Dyn. Syst. 27, 373–404. doi:10.1080/13873954.2021.1879874
Nabipour, M., and Moosavian, S. A. A. (2021). Human model in the loop design optimization for RoboWalk wearable device. J. Mech. Sci. Technol. 35, 4685–4693. doi:10.1007/s12206-021-0935-z
Oba, T., Kadone, H., Hassan, M., and Suzuki, K. (2019). Robotic ankle–foot orthosis with a variable viscosity link using MR fluid. IEEE Trans. mechatronics 24, 495–504. doi:10.1109/TMECH.2019.2894406
Orekhov, G., Fang, Y., Luque, J., and Lerner, Z. (2020). Ankle exoskeleton assistance can improve over-ground walking economy in individuals with cerebral palsy. IEEE Trans. Neural Syst. Rehabil. Eng. 28, 461–467. doi:10.1109/TNSRE.2020.2965029
Orekhov, G., Fang, Y., Cuddeback, C. F., and Lerner, Z. F. (2021). Usability and performance validation of an ultra-lightweight and versatile untethered robotic ankle exoskeleton. J. NeuroEng. Rehabil. 18, 163–216. doi:10.1186/s12984-021-00954-9
Oymagil, A. M., Hitt, J. K., Sugar, T., and Fleeger, J. (2007). “Control of a regenerative braking powered ankle foot orthosis,” in 2007 IEEE 10th International Conference on Rehabilitation Robotics (IEEE), 28–34. doi:10.1109/ICORR.2007.4428402
Pardoel, S., and Doumit, M. (2019). Development and testing of a passive ankle exoskeleton. Biocybern. Biomed. Eng. 39, 902–913. doi:10.1016/j.bbe.2019.08.007
Peng, X., Acosta-Sojo, Y., Wu, M. I., and Stirling, L. (2022). Actuation timing perception of a powered ankle exoskeleton and its associated ankle angle changes during walking. IEEE Trans. Neural Syst. Rehabil. Eng. 30, 869–877. doi:10.1109/TNSRE.2022.3162213
Rodda, J., and Graham, H. K. (2001). Classification of gait patterns in spastic hemiplegia and spastic diplegia: A basis for a management algorithm. Eur. J. Neurol. 8, 98–108. doi:10.1046/j.1468-1331.2001.00042.x
Rodriguez, K., Groot, J. D., Baas, F., Stijntjes, M., Helm, F. V. D., Kooij, H. V. D., et al. (2018). “Passive ankle joint stiffness compensation by a novel ankle-foot-orthosis,” in IEEE International Conference on Biomedical Robotics and Biomechatronics (BioRob). doi:10.1109/BIOROB.2018.8487784
Roy, A., Krebs, H. I., Barton, J. E., Macko, R. F., and Forrester, L. W. (2013). “Anklebot-assisted locomotor training after stroke: A novel deficit-adjusted control approach,” in 2013 IEEE International Conference on Robotics and Automation (IEEE), 2175–2182. doi:10.1109/ICRA.2013.6630869
Sawicki, G. S., and Ferris, D. P. (2008). Mechanics and energetics of level walking with powered ankle exoskeletons. J. Exp. Biol. 211, 1402–1413. doi:10.1242/jeb.009241
Sekiguchi, Y., Owaki, D., Honda, K., Fukushi, K., Hiroi, N., Nozaki, T., et al. (2020). Ankle–foot orthosis with dorsiflexion resistance using spring-cam mechanism increases knee flexion in the swing phase during walking in stroke patients with hemiplegia. Gait Posture 81, 27–32. doi:10.1016/j.gaitpost.2020.06.029
Shepherd, M. K., Molinaro, D. D., Sawicki, G. S., and Young, A. J. (2022). Deep learning enables exoboot control to augment variable-speed walking. IEEE Robotics Autom. Lett. 7, 3571–3577. doi:10.1109/LRA.2022.3147565
Shorter, K. A., Kogler, G. F., Loth, E., Durfee, W. K., and Hsiao-Wecksler, E. T. (2011). A portable powered ankle-foot orthosis for rehabilitation. J. Rehabil. Res. Dev. 48, 459. doi:10.1682/JRRD.2010.04.0054
Shorter, K. A., Xia, J., Hsiao-Wecksler, E. T., Durfee, W. K., and Kogler, G. F. (2013). Technologies for powered ankle-foot orthotic systems: Possibilities and challenges. IEEE/ASME Trans. Mechatronics 18, 337–347. doi:10.1109/TMECH.2011.2174799
Siviy, C., Bae, J., Baker, L., Porciuncula, F., Baker, T., Ellis, T. D., et al. (2020). Offline assistance optimization of a soft exosuit for augmenting ankle power of stroke survivors during walking. IEEE Robotics Autom. Lett. 5, 828–835. doi:10.1109/LRA.2020.2965072
Slade, P., Kochenderfer, M. J., Delp, S. L., and Collins, S. H. (2022). Personalizing exoskeleton assistance while walking in the real world. Nature 610, 277–282. doi:10.1038/s41586-022-05191-1
Steele, K. M., Jackson, R. W., Shuman, B. R., and Collins, S. H. (2017). Muscle recruitment and coordination with an ankle exoskeleton. J. Biomechanics 59, 50–58. doi:10.1016/j.jbiomech.2017.05.010
Takahashi, K. Z., Lewek, M. D., and Sawicki, G. S. (2015). A neuromechanics-based powered ankle exoskeleton to assist walking post-stroke: A feasibility study. J. NeuroEng. Rehabil. 12, 23. doi:10.1186/s12984-015-0015-7
Tamburella, F., Tagliamonte, N. L., Pisotta, I., Masciullo, M., Arquilla, M., Van Asseldonk, E. H., et al. (2020). Neuromuscular controller embedded in a powered ankle exoskeleton: Effects on gait, clinical features and subjective perspective of incomplete spinal cord injured subjects. IEEE Trans. Neural Syst. Rehabil. Eng. 28, 1157–1167. doi:10.1109/TNSRE.2020.2984790
Torricelli, D., Rodriguez-Guerrero, C., Veneman, J. F., Crea, S., Briem, K., Lenggenhager, B., et al. (2020). Benchmarking wearable robots: Challenges and recommendations from functional, user experience, and methodological perspectives. Front. Robot. AI 13, 561774. doi:10.3389/frobt.2020.561774
Tucker, M. R., Olivier, J., Pagel, A., Bleuler, H., Bouri, M., Lambercy, O., et al. (2015). Control strategies for active lower extremity prosthetics and orthotics: A review. J. NeuroEng. Rehabil. 12, 1. doi:10.1186/1743-0003-12-1
Ultraflex Systems (2009). Unprecedented stability and motion: Ultraflex’s adjustable dynamic response (ADR). Tech. rep. Ultraflex.
van Dijk, W., Meijneke, C., and van der Kooij, H. (2017). Evaluation of the achilles ankle exoskeleton. IEEE Trans. Neural Syst. Rehabil. Eng. 25, 151–160. doi:10.1109/TNSRE.2016.2527780
van Hoorn, M., Hekman, E., Rocon, E., van Asseldonk, E., and Bayón, C. (2022). “Technical validation of a body-weight controlled clutch for ankle-foot orthoses of children with cerebral palsy,” in Proceedings - XLIII Jornadas de Automática, 2022, Logroño (La Rioja), 165–170. doi:10.17979/spudc.9788497498418.0165
Vanderborght, B., Albu-Schaeffer, A., Bicchi, A., Burdet, E., Caldwell, D. G., Carloni, R., et al. (2013). Variable impedance actuators: A review. Robotics Aut. Syst. 61, 1601–1614. doi:10.1016/j.robot.2013.06.009
Wang, X., Guo, S., Qu, B., Song, M., and Qu, H. (2020). Design of a passive gait-based ankle-foot exoskeleton with self-adaptive capability. Chin. J. Mech. Eng. 33, 49. (English Edition). doi:10.1186/s10033-020-00465-z
Wang, C., Dai, L., Shen, D., Wu, J., Wang, X., Tian, M., et al. (2022a). Design of an ankle exoskeleton that recycles energy to assist propulsion during human walking. IEEE Trans. Biomed. Eng. 69, 1212–1224. doi:10.1109/TBME.2021.3120716
Wang, W., Chen, J., Ding, J., Zhang, J., and Liu, J. (2022b). Improving walking economy with an ankle exoskeleton prior to human-in-the-loop optimization. Front. Neurorobotics 15, 797147–797212. doi:10.3389/fnbot.2021.797147
Ward, J., Sugar, T., Boehler, A., Standeven, J., and Engsberg, J. R. (2011). Stroke survivors’ gait adaptations to a powered ankle-foot orthosis. Adv. Robot. 25, 1879–1901. doi:10.1163/016918611X588907
Weerasingha, A. H., Pragnathilaka, A. D., Withanage, W. P., Ranaweera, R. K., and Gopura, R. A. (2018). “C-JAE: 3 DOF robotic ankle exoskeleton with compatible joint axes,” in MERCon 2018 - 4th International Multidisciplinary Moratuwa Engineering Research Conference, 270–275. doi:10.1109/MERCon.2018.8422004
Wirz, M., and van Hedel, H. J. (2018). Balance, gait, and falls in spinal cord injury. 1 edn, 159. Elsevier B.V. doi:10.1016/B978-0-444-63916-5.00024-0
Witte, K. A., Zhang, J., Jackson, R. W., and Collins, S. H. (2015). “Design of two lightweight, high-bandwidth torque-controlled ankle exoskeletons,” in Proceedings - IEEE International Conference on Robotics and Automation (Pittsburgh, PA, United States: Dept. Mechanical Engineering, Carnegie Mellon University), 1223–1228. 5000 Forbes Ave. doi:10.1109/ICRA.2015.7139347
Wren, T. A., Dryden, J. W., Mueske, N. M., Dennis, S. W., Healy, B. S., and Rethlefsen, S. A. (2015). Comparison of 2 orthotic approaches in children with cerebral palsy. Pediatr. Phys. Ther. 27, 218–226. doi:10.1097/PEP.0000000000000153
Xia, H., Kwon, J., Pathak, P., Ahn, J., Shull, P. B., and Park, Y.-L. (2020). “Design of A Multi-Functional soft ankle exoskeleton for foot-drop prevention, propulsion assistance, and inversion/eversion stabilization,” in 2020 8th IEEE RAS/EMBS International Conference for Biomedical Robotics and Biomechatronics (BioRob) (IEEE), 118–123. doi:10.1109/BioRob49111.2020.9224420
Yamamoto, S., Hagiwara, A., Mizobe, T., Yokoyama, O., and Yasui, T. (2005). Development of an ankle-foot orthosis with an oil damper. Prosthetics Orthot. Int. 29, 209–219. doi:10.1080/03093640500199455
Yamamoto, S., Motojima, N., Kobayashi, Y., Osada, Y., Tanaka, S., and Daryabor, A. (2022). Ankle-foot orthosis with an oil damper versus nonarticulated ankle-foot orthosis in the gait of patients with subacute stroke: A randomized controlled trial. J. NeuroEng. Rehabil. 19, 50. doi:10.1186/s12984-022-01027-1
Yandell, M. B., Tacca, J. R., and Zelik, K. E. (2019). Design of a low profile, unpowered ankle exoskeleton that fits under clothes: Overcoming practical barriers to widespread societal adoption. IEEE Trans. Neural Syst. Rehabil. Eng. 27, 712–723. doi:10.1109/TNSRE.2019.2904924
Yeung, L.-F., Ockenfeld, C., Pang, M.-K., Wai, H.-W., Soo, O.-Y., Li, S.-W., et al. (2017). “Design of an exoskeleton ankle robot for robot-assisted gait training of stroke patients,” in 2017 International Conference on Rehabilitation Robotics (ICORR) (IEEE), 211–215. doi:10.1109/ICORR.2017.8009248
Yeung, L. F., Ockenfeld, C., Pang, M. K., Wai, H. W., Soo, O. Y., Li, S. W., et al. (2018). Randomized controlled trial of robot-assisted gait training with dorsiflexion assistance on chronic stroke patients wearing ankle-foot-orthosis. J. NeuroEng. Rehabil. 15, 51–12. doi:10.1186/s12984-018-0394-7
Yeung, L.-F., Lau, C. C. Y., Lai, C. W. K., Soo, Y. O. Y., Chan, M.-L., and Tong, R. K. Y. (2021). Effects of wearable ankle robotics for stair and over-ground training on sub-acute stroke: A randomized controlled trial. J. NeuroEng. Rehabil. 18, 19. doi:10.1186/s12984-021-00814-6
Zhang, C., Zhu, Y., Fan, J., Zhao, J., and Yu, H. (2015). Design of a quasi-passive 3 DOFs ankle-foot wearable rehabilitation orthosis. Bio-Med. Mater. Eng. 26, S647–S654. doi:10.3233/BME-151356
Keywords: ankle-foot, orthosis, gait, passive, active
Citation: Lora-Millan JS, Nabipour M, van Asseldonk E and Bayón C (2023) Advances on mechanical designs for assistive ankle-foot orthoses. Front. Bioeng. Biotechnol. 11:1188685. doi: 10.3389/fbioe.2023.1188685
Received: 17 March 2023; Accepted: 27 June 2023;
Published: 07 July 2023.
Edited by:
Yang Liu, Hong Kong Polytechnic University, Hong Kong SAR, ChinaReviewed by:
Daniel Gomez-Vargas, National University of San Juan, ArgentinaWojciech Wolański, Silesian University of Technology, Poland
Maohua Lin, Florida Atlantic University, United States
Copyright © 2023 Lora-Millan, Nabipour, van Asseldonk and Bayón. This is an open-access article distributed under the terms of the Creative Commons Attribution License (CC BY). The use, distribution or reproduction in other forums is permitted, provided the original author(s) and the copyright owner(s) are credited and that the original publication in this journal is cited, in accordance with accepted academic practice. No use, distribution or reproduction is permitted which does not comply with these terms.
*Correspondence: Cristina Bayón, Yy5iYXlvbmNhbGRlcm9uQHV0d2VudGUubmw=
†These authors have contributed equally to this work