- Department of Orthopedics, The First People’s Hospital of Huzhou, First Affiliated Hospital of Huzhou University, Huzhou, Zhejiang, China
The entry of subcutaneous extracellular matrix proteins into the circulation is a key step in hemostasis initiation after vascular injury. However, in cases of severe trauma, extracellular matrix proteins are unable to cover the wound, making it difficult to effectively initiate hemostasis and resulting in a series of bleeding events. Acellular-treated extracellular matrix (ECM) hydrogels are widely used in regenerative medicine and can effectively promote tissue repair due to their high mimic nature and excellent biocompatibility. ECM hydrogels contain high concentrations of extracellular matrix proteins, including collagen, fibronectin, and laminin, which can simulate subcutaneous extracellular matrix components and participate in the hemostatic process. Therefore, it has unique advantages as a hemostatic material. This paper first reviewed the preparation, composition and structure of extracellular hydrogels, as well as their mechanical properties and safety, and then analyzed the hemostatic mechanism of the hydrogels to provide a reference for the application and research, and development of ECM hydrogels in the field of hemostasis.
1 Introduction
Uncontrolled bleeding is a major challenge in trauma care and surgery. Rapid and effective hemostasis is essential to improve care quality and save lives (Yang et al., 2019; Fan et al., 2021). Conventional hemostatic materials, such as tourniquets, gauze, and bandages, have shown limited efficacy in controlling bleeding (Chen et al., 2020). Moreover, gauze or bandages need to be completely removed (Leonhardt et al., 2019) after hemostasis because they are non-biodegradable, resulting in secondary injury, delayed healing, and additional pain. Therefore, there has been widespread interest in developing novel hemostatic materials and techniques. The ideal hemostatic material should have the following characteristics (Ellis-Behnke, 2011; Zhong et al., 2021): 1) the ability to quickly form thrombus; 2) It should be biocompatible, biodegradable, and conducive to accelerating wound healing; 3) stable, cost-effective, and safe.
As a novel polymer material, a hydrogel is a three-dimensional network structure with high water content (Zhang et al., 2021). The hydrogel can be applied to various irregular wounds and intraluminal injuries (Deng et al., 2017; Palomino-Durand et al., 2019) due to its injectability and fluidity, which is crucial for rapid and effective hemostasis. Furthermore, the excellent biodegradability and biocompatibility ensure the safety of hydrogel-based biomaterials for in vivo application and enhance their ability to promote wound healing (Labay et al., 2019). Therefore, hydrogel-based hemostatic materials have unique advantages.
Hemostasis refers to the quick stopping of bleeding (Zhang et al., 2020; D'Andrea et al., 2009; Wang et al., 2021; Zheng et al., 2020). Bleeding caused by small vessel injuries usually stops automatically within a few minutes. This phenomenon is called physiologic hemostasis. Physiologic hemostasis is one of the important protective mechanisms of the body, which include three steps: first, the injured small blood vessel immediately constricts to seal the vessel and reduce bleeding. Second, the subcutaneous matrix promotes platelet aggregation and adhesion, forming a soft hemostatic plug to fill the wound. Third, by activating the blood coagulation system, soluble fibrinogen in the plasma is converted into insoluble fibrin polymer, forming a firm mixture composed of fibrin and platelets, effectively stopping bleeding (Wang et al., 2021). However, physiological hemostasis only works in case of minor trauma (Zheng et al., 2020). Injury to an artery or viscera makes it difficult for the contracted vessels to cover the wound surface, preventing the subendothelial matrix from playing its role effectively. The subcutaneous extracellular matrix is essential for hemostasis because it contains numerous extracellular matrix proteins and can promote hemostasis in different ways (Watson, 2009; Bergmeier and Hynes, 2012; Wang et al., 2016a). Therefore, it is a novel idea to use an exogenous extracellular matrix to promote wound hemostasis when bleeding caused by trauma exceeds the ability of self-hemostasis.
ECM hydrogel has attracted much attention in the field of tissue repair and regenerative medicine in recent years due to its good cytocompatibility, biodegradability, and ability to induce tissue regeneration (Zhang et al., 2021). Native hydrogels contain a host of ECM proteins that can mimic the subcutaneous matrix to promote hemostasis. Regeneration and hemostasis can cooperate to provide tissue specific therapies. For example, When liver, kidney, or spleen tissue is injured and bleeding due to surgery or trauma, as shown in Figure 1, the corresponding ECM hydrogel of the liver, kidney, or spleen can be injected to halt bleeding and promote tissue regeneration. This review further introduces the preparation, composition, structure of ECM hydrogels, as well as their mechanical property and safety, and analyzes the role of various extracellular matrix components in hemostasis. It can provide a reference for future hemostatic material research.
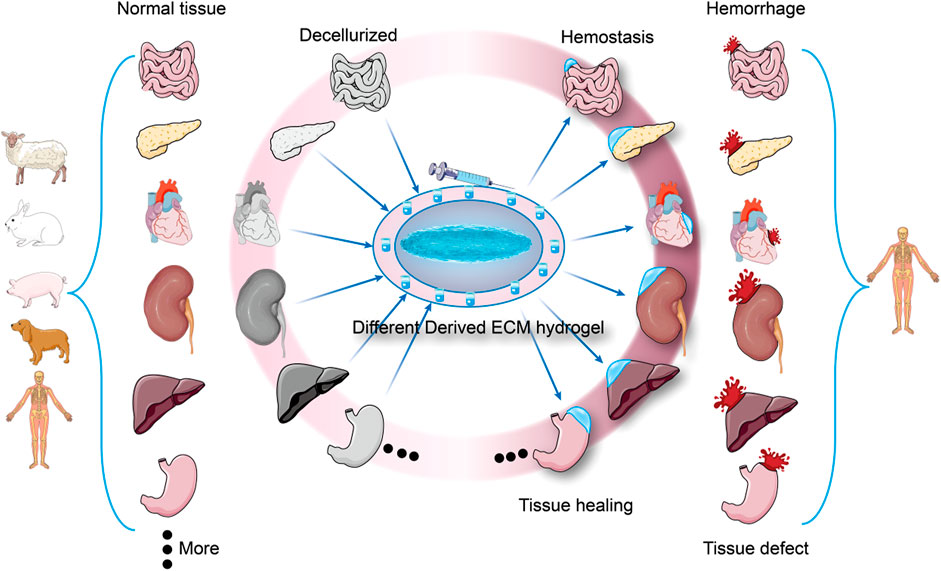
FIGURE 1. Different derived ECM hydrogels are used for hemostasis and tissue healing. Each tissue has a unique extracellular matrix structure, and when trauma results in tissue damage and bleeding, ECM hydrogels from the same tissue source can be used to stop bleeding and promote tissue healing (Specialized hemostasis and tissue healing).
2 Overview of extracellular matrix hydrogels
2.1 Source of extracellular matrix materials
The extracellular matrix can originate from cell-derived matrix, various tissues, and organs of mammals. ECM derived from in vitro cultured cell constructs provides a promising alternative for creating tissue engineered scaffolds (Cheng et al., 2014). For example, osteogenic ECMs can be constructed in vitro by culturing specific cells such as mesenchymal stem cells (Zeitouni et al., 2012), chondrocytes (Lau et al., 2012) or osteoblasts (Tour et al., 2011) under osteogenic medium. The extracellular matrix of animal origin is primarily xenogeneic, commonly derived from pig tissues and organs, but also from human cadavers and rarely from autogenic sources (Zhang et al., 2021). The sources of autologous and allogeneic tissue are minimal. Various tissues from different animals, such as the bladder (Kao et al., 2020) and heart (Seif-Naraghi et al., 2013) of pigs and the shin bone of cattle (Sawkins et al., 2013), have been widely used to overcome the shortage of human tissue to create decellularized ECM (dECM). Human-derived ECM can be sourced from cadavers, diseased or injured tissues and organs of patients, and donated tissues from human tissue banks. Obtaining autologous tissue-derived extracellular matrix requires surgical intervention (Fuller et al., 2013), decellularization, and detoxification procedures, which makes the process time-consuming.
2.2 Preparation of extracellular matrix hydrogels
Decellularization of biomaterials removes cellular components while maintaining the original structure, composition, biochemical, and mechanical properties of natural ECMs. Researchers have developed various decellularization methods, including physical, chemical, and enzyme treatments and combinations of these methods. For example, Sellaro et al. utilized mechanical agitation, trypsin/Ethylene diamine tetraacetic acid (EDTA), sodium deoxycholic (SDC), and Triton X-100 to create porcine liver ECM (Sellaro et al., 2010). However, each of the techniques mentioned above has its benefits and limitations. The physical method causes minimal damage to the tissue structure, but it is difficult to remove cellular components effectively (Burk et al., 2014). Chemical agents can effectively eliminate cellular components while destroying extracellular matrix proteins (Kasimir et al., 2003). Protein composition and content significantly affect the formation of extracellular matrix hydrogels. Therefore, it is crucial to consider the effect of chemical reagents on extracellular matrix proteins during the decellularization process, as shown in Table 1.
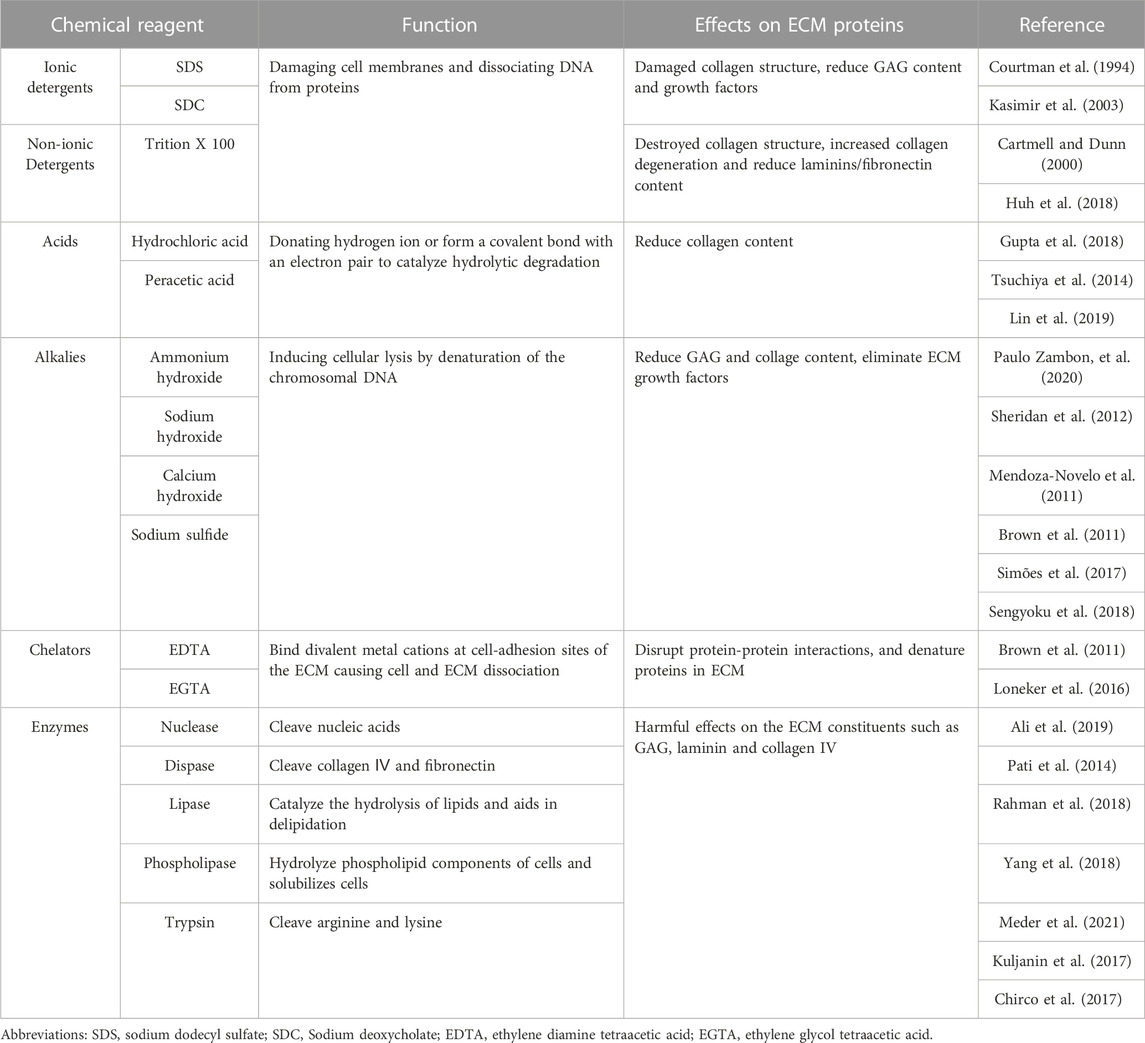
TABLE 1. The function and effection of acellular agents on ECM proteins in the process of decellularization.
The formation of extracellular matrix-derived hydrogels after decellularization is based on the self-assembly of collagen and is influenced by glycosaminoglycans, proteoglycans, and various proteins (Saldin et al., 2017; Zhang et al., 2021). The powdered decellularized extracellular matrix (dECM) is first dissolved into a homogeneous solution by enzymatic hydrolysis and acid dissolution. The formation of cross-linking gel can then be induced by adjusting the temperature, pH, or addition of cross-linking agent (Uriel et al., 2008). After the ECM powder is digested into a solution with pepsin, it contains dispersed collagen, glycosaminoglycan, proteoglycan, and ECM protein monomer. Under the suitable temperature and pH conditions or the addition of cross-linking agent, intramolecular and intermolecular cross-links of the three-dimensional helical structure of collagen monomers can be generated by covalent bonding to improve the tension and stability of collagen fibers and making ECM gel solution. Small intestinal submucosa (Mao et al., 2022), bladder (Kobayashi et al., 2020), fat (Tan et al., 2017), heart (Seif-Naraghi et al., 2013), cornea (Yazdanpanah et al., 2021a), dermis (Wolf et al., 2012), central nervous system (Tukmachev et al., 2016), umbilical cord (Výborný et al., 2019) and pancreas (Sackett et al., 2018) have been used to prepare ECM hydrogels successfully.
2.3 Constitutive structure of ECM hydrogels
Extracellular matrix-derived hydrogels are naturally occurring substances. After acellular treatment, the extracellular matrix retains the intrinsic structural and chemical integrity of the original tissue, which consists mainly of protein and non-protein components such as collagen, elastin, fibronectin, laminin, glycosaminoglycan, and hyaluronic acid (Halper, 2021). The ECM of each tissue is produced by its resident cells through interactions with other cell types. These cells secrete molecules that develop unique tissue structures and biochemical properties, creating an ideal microenvironment for their function. Therefore, the ECM formed by different tissues and organs after acellular treatment differs in composition, structure, and content as show in Table 2. Collagen is the main component of ECM hydrogel. Davidov et al. (2021) performed a quantitative analysis of hydrogels’ composition; artery-derived hydrogels contained approximately 64% collagen, while heart muscle, pancreas, and fat-derived hydrogels contained 20% more collagen than artery-derived hydrogels, ranging from 84% to 91%. Fibronectin (FN) is a multidomain glycoprotein present in most extracellular matrices and is involved in cell adhesion, migration, metastasis, proliferation, and differentiation (Xiao et al., 2018). Laminin is a high molecular weight multifunctional protein found in the extracellular matrix. Laminin-mediated interactions are crucial for cellular architecture formation through cell adhesion, spreading, and migration (Higaki et al., 2002). The ECM is also composed of many glycosaminoglycans (GAGs) mixtures that bind growth factors and improve water retention while giving the ECM some gel-like properties. The amount of GAG left in the tissue after decellularization largely depends on the method of decellularization. For example, ion stain removers are often used during decellularization to remove GAG from the ECM (Ebrahimi Sadrabadi et al., 2021). Therefore, ECM-derived hydrogels have a very complex composition in which different components play different roles in hemostasis.
2.4 The safety of ECM hydrogels
ECM hydrogels are widely used as scaffold materials in regenerative medicine due to their unique biological activity and good biocompatibility (Saldin et al., 2017; Zhang et al., 2021). ECM components are less vulnerable to rejection because their structure and function are highly conserved and nearly identical across species. ECM hydrogels, which eliminate many cell components compared to allograft and xenograft, can effectively reduce the potential for adverse host reactions after implantation. When compared to other synthetic polymers, ECM components of hydrogels are retained after decellularization, providing a better microenvironment for cell attachment and cell-ECM interaction (Badylak and Gilbert, 2008; Saldin et al., 2017).
ECM scaffolds are manufactured using various tissues derived from cells, animals, or humans. The dECM scaffold derived from cells has several advantages. For example, cultured cells can be screened for pathogens and then kept free of pathogens for ECM. In addition, after acellular treatment, the cell-derived matrix has improved plasticity and optimal porosity due to its loose structure (Zhu et al., 2021). Importantly, they can generate autologous ECM scaffolds from autologous cells, thereby avoiding the adverse host reactions induced by allogeneic or heterogeneous materials and circumventing the limited availability of autologous tissue. However, cell-derived dECM typically has limited mechanical properties (Guan et al., 2022).
Pigs are the primary source of animal extracellular matrix components. Compared with other animals, porcine organs are readily available in larger quantities and are comparable in size and function to human organs. Therefore, pigs have always been the preferred source of cellular scaffolds of tissues and organs (Zhang et al., 2021). Many commercialized porcine extracellular matrix products (Prima™ Plus, Hancock® II, Mosaic®, Freestyle®, Permacol®, Strattice™, MatriStem®, Oasis®, and CuffPatch®) (Table 2) are employed in tissue regeneration. For example, Oasis®, derived from acellular porcine small intestinal submucosa, is an acellular product primarily used in the treatment of chronic wounds (Holmes et al., 2013). Extracellular matrix hydrogels are prepared based on extracellular matrix scaffolds. Traverse et al. (2019) conducted a first-in-man, single-arm, multicenter trial to demonstrate the safety, feasibility, and preliminary efficacy of percutaneous trans-endocardial delivery of VentriGel (an extracellular matrix hydrogel derived from decellularized porcine myocardium) in early and late MI (Myocardial infarction) patients with left ventricular (LV) dysfunction, which is the first demonstration of using a decellularized ECM hydrogel in any tissue in patients. Interestingly, ECM hydrogel can be used as an embolic agent to embolize arteries and promote vascular healing. Animal experiments have shown no signs of lymphadenopathy, pulmonary emboli, or stroke, suggesting that ECM-based nanocomposite hydrogel was safe even when used in blood vessels (Hu et al., 2020). Porcine endogenous retroviruses (PERV) are present in the pig genome and could pose a safety hazard (Kimsa et al., 2014). However, the risk is minimal because the source pigs will be housed in specific pathogen-free, biosecure conditions and are regularly monitored (Cooper et al., 2018).
Various human tissues and organs, such as cardiac tissue (Johnson et al., 2014), pancreas (Sackett et al., 2018), and adipose (Chen et al., 2021), are utilized to produce dECM. Gao et al. developed a human cardiac tissue-derived scaffold using decellularization, which improved the functional behavior of cardiac progenitor cells from patients with congenital heart disease, including cell adhesion, survival, and proliferation (Gao et al., 2022). Human-derived ECM materials are not controllable and are easily affected by donor age, degree of damage, and storage period (Johnson et al., 2014). However, those materials can effectively prevent the transmission of xenogenetic diseases.
3 Hemostatic mechanism of hydrogel
3.1 Physical barrier
Temperature-sensitive hydrogels are of interest for achieving effective hemostasis and wound closure because they are suitable for wounds of various shapes (Liu et al., 2018; Cao et al., 2020). ECM hydrogels have good temperature sensitivity, existing in a liquid state at 4°C and a gel state at 37°C. Fully gelated ECMs usually appear as irregular nanofiber scaffolds with interconnected pores on SEM images (Freytes et al., 2008; Wolf et al., 2012). The thermal characteristics of hydrogels can be used to stop bleeding in wounds, especially irregular wounds. The liquid hydrogel can cover irregular wounds at low temperatures; when the temperature rises to body temperature, the hydrogel transforms into a gel. The microstate showed irregular fibrous reticular scaffolds that mechanically sealed the vascular breach and formed a physical barrier as shown in Figure 2. The hydrogel is injectable and can be injected into deep tissue wounds for hemostasis (Pourshahrestani et al., 2020). Compared to natural tissues, hydrogels exhibit poor mechanical properties (Grover et al., 2014; Ahearne and Coyle, 2016), making them susceptible to deformation. Increasing hydrogel mechanical strength can effectively facilitate wound sealing and prevent blood loss. There are two main methods to improve the mechanical properties of ECM hydrogel. The first approach is to increase the initial concentration of the extracellular matrix. The second approach is to use cross-linking techniques to improve the mechanical properties of hydrogels.
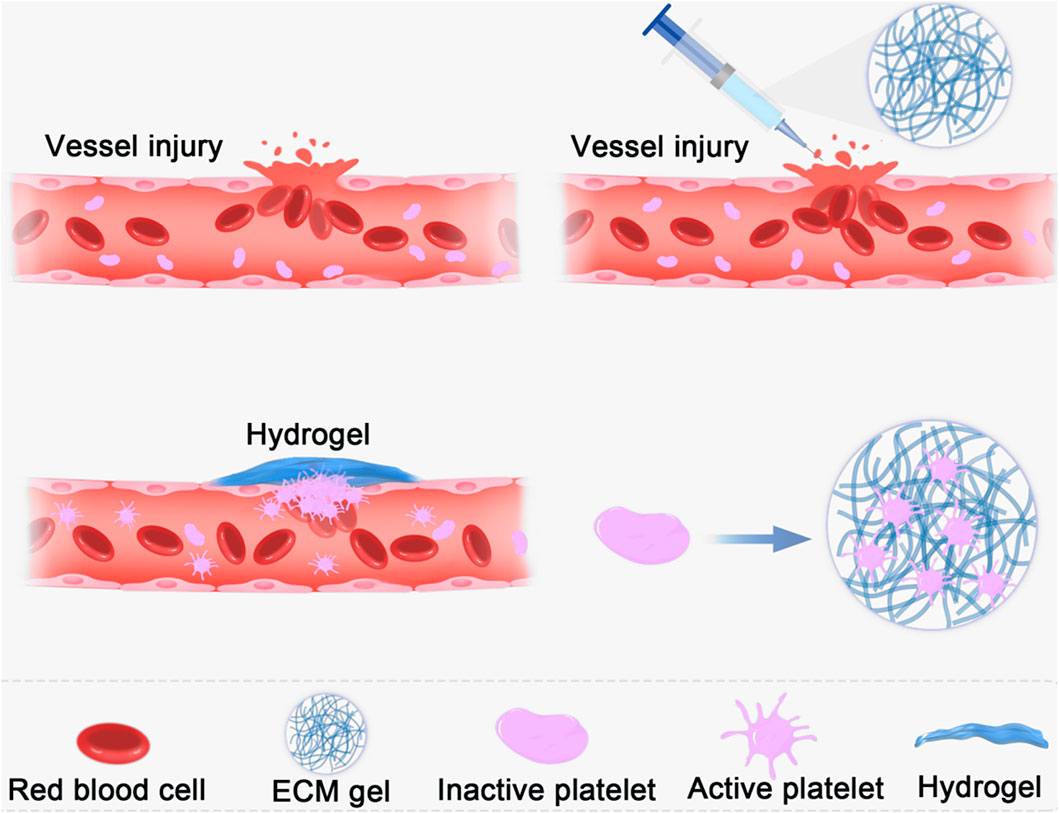
FIGURE 2. Schematic diagram of the use of ECM hydrogel showed irregular fibrous reticular scaffolds that mechanically sealed the vascular breach and formed a physical barrier.
3.1.1 Effect of concentration on mechanical properties
Extracellular matrix hydrogels have different mechanical properties closely connected to their composition. Davidov et al. compared the mechanical properties of ECM hydrogels derived from the porcine liver, pancreas, artery, and heart (Davidov et al., 2021). It was found that arterial hydrogels exhibit the highest mechanical properties, while pancreatic hydrogels exhibit the lowest. The mechanical properties of hydrogels vary with the species of origin. The porcine myocardial matrix is significantly higher than the Human myocardial matrix by measuring storage and loss modulus with a parallel plate rheometer (Johnson et al., 2014). The mechanical properties of hydrogels vary with the species of origin Matrix, and mechanical properties can be effectively enhanced by increasing the concentration of its extracellular matrix. Tissue fibrosis typically increases various extracellular matrix components. Normal heart tissue has an elastic modulus of 10–15 kPa, while fibrotic tissue can be 2 to 10 times stiffer. Healthy lung tissue is relatively soft, ranging from 1 to 5 kPa, and can stiffen above 10 kPa in pulmonary fibrosis (Hewawasam et al., 2023).
Hydrogels prepared with a high concentration of extracellular matrix typically have greater mechanical strength. Medberry et al. (2013) prepared brain-ECM, spinal cord-ECM, and urinary bladder-ECM hydrogels. As the temperature rapidly increased from 10°C to 37°C, the maximum storage modulus, maximum loss modulus, and the time to complete gelation increased for the three hydrogels with increasing ECM concentration. Dermal-ECM hydrogels were evaluated for their structural, mechanical, and in vitro cell response characteristics, which were found to depend on the ECM concentration (Wolf et al., 2012). These findings suggest that the ECM concentration can influence and control the physical properties of an ECM hydrogel.
3.1.2 Effect of crosslinking technology on mechanical properties
The application of crosslinking agents has been investigated to enhance the mechanical properties of hydrogels (Pilipchuk et al., 2013; Ahearne and Coyle, 2016; Parthiban et al., 2021). Crosslinking agents are introduced to modify various biomaterials and improve their mechanical properties by considering their composition and structural characteristics. Cell compatibility is a crucial evaluation factor, for example, using glutaraldehyde (GA) as a crosslinking agent might cause cell toxicity (Wang et al., 2014). Therefore, it is vital to consider the reaction of crosslinking agents on cells while enhancing mechanical features, as shown in Table 3.
3.2 Simulate physiologic hemostasis
The inner wall of most blood vessels is covered by a continuous layer of endothelial cells that seals the subcutaneous extracellular matrix components and provides an anti-thrombotic surface for the body. Moreover, it actively secretes platelet activation inhibitors, such as nitric oxide and prostacyclin (Hein et al., 2009). Which regulate blood circulation and prevent thrombosis (Gimbrone et al., 2000). However, in the case of trauma, the ruptured vascular wall is difficult to effectively play the role of hemostasis, leading to a series of bleeding and even death events. Exogenous ECM hydrogels mimic the significant components of the extracellular matrix, including collagen, laminin, fibronectin, and vitronectin, which induce platelet adhesion and activation and promote hemostasis and thrombosis. Due to the different molecular environments of the original tissue ECM, these hydrogels have varying compositions and contents, which play different roles in hemostasis (Figure 3). According to the research of Cai et al., 2021 as shown in Figure 4, extracellular matrix hydrogels derived from porcine dermal were used in liver, kidney, and vascular trauma models of Sprague-Dawley rats and the pathological section suggests thrombosis, which was found that ECM hydrogels effectively played a hemostatic role.
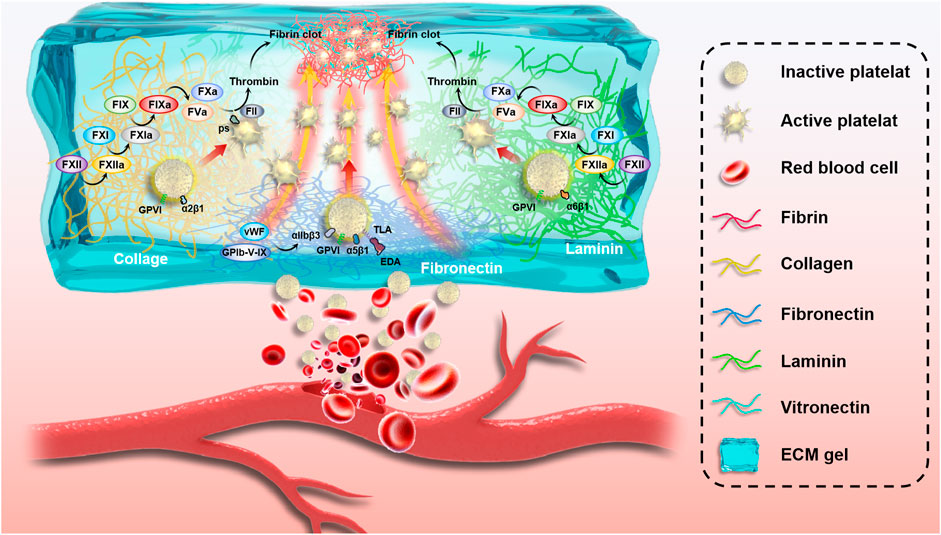
FIGURE 3. Schematic of the complex mechanism of blood vessel hemostasis. Vessel wall rupture makes it difficult for ECM proteins to play a hemostatic role, resulting in a series of bleeding events and even death. Exogenous ECM hydrogels mimic extracellular matrix main ingredients, including collagen, laminin, fibronectin, and vitronectin, which induce platelet adhesion and activation and promote hemostasis and thrombosis.
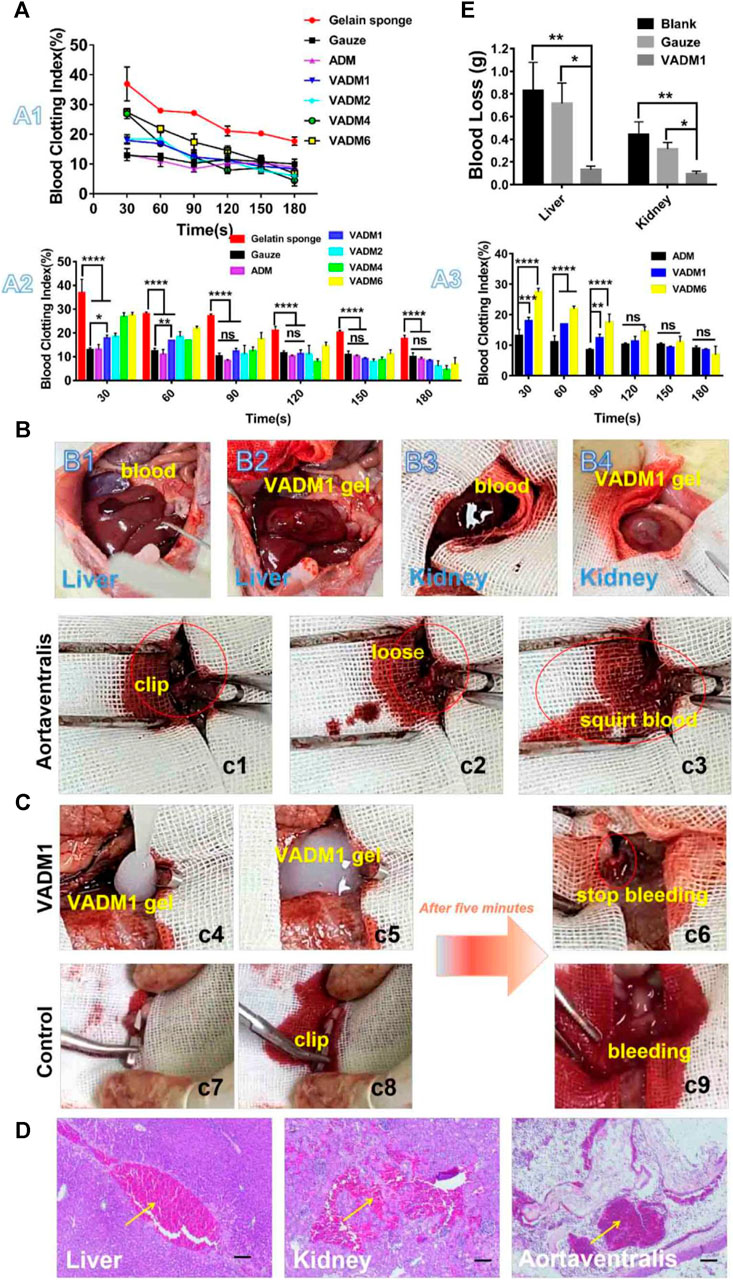
FIGURE 4. In vitro and in vivo hemostasis test. (A) In vitro dynamic whole-blood clotting evaluation (A1, A2, and A3) of the ADM, VADM1, VADM2, VADM4, and VADM6 hydrogels, with gelatin sponge and gauze used as the control. (B) Photographs captured during the liver laceration model and renal tissue-defect model. (B1) A wound with a length of 1 cm in the liver. (B2) VADM1 hydrogel formed in situ on the trauma injury. (B3) A small portion of the kidney tissue was removed with surgical scissors. (B4) VADM1 hydrogel formed in situ on the defective tissue. (C) Photographs captured during a hemostasis test on the abdominal aorta (highlighted by red circles and ellipses). (c1) Clipped abdominal aorta. (c2,c3) Arterial spurts can be observed after release of the artery clip. (c4) VADM1 hydrogel applied to the wound. (c5) Abdominal aorta surrounded by VADM1 hydrogel. (c6) Artery clip was removed after 5 min, and the bleeding was stopped completely. The abdominal aorta was clogged with hydrogel. (c7–c9) Arterial spurts still occur on the control group after 5 min, when the artery clip was released. (D) H&E stained micrographs, showing signifificant accumulation of red cells (yellow arrow) within the incision site. Scale bar ¼ 100 μm. (E) Blood loss from liver and kidney incisions. Three replicates of each sample were tested and the data were shown as mean ± SD. VADM:acellular dermal matrix hydrogel blended with vancomycin. Reprinted from (Cai et al., 2021) with permission from Elsevier Publisher, Ltd.
3.2.1 The role of collagen in hemastasis
Collagen is the main component of extracellular matrix hydrogel and is also a potent platelets activator. Its hemostatic mechanism is the effect on platelets to shorten the thrombosis time. Glycoprotein VI (GPVI) and α2β1 integrin are the collagen receptors on platelets (Sarratt et al., 2005). Collagen interacts directly with platelets via GPVI, mediates platelet activation, and integrin α2β1, which supports platelet adhesion to collagen (Jarvis et al., 2008; Attwood et al., 2013). Furthermore, collagen induces exposure of procoagulant phospholipids on platelets via GPVI(Manon-Jensen et al., 2016). The exposure of phospholipids provides an assembly site for coagulation factors, resulting in thrombin production required for platelet-fibrin thrombus. Simultaneously, collagen activates the intrinsic coagulation pathway by binding to factor XII(FXII, a coagulation factor) (van der Meijden et al., 2009). Therefore, collagen can promote blood coagulation through various pathways and plays a leading role in the process of ECM hemostasis.
3.2.2 The role of laminin in hemastasis
Laminin is a heterotrimeric glycoprotein found in almost all ECM tissues, especially in the basement membrane of the vasculature. Platelet recruitment by VWF (von Willebrand Factor) enables integrin α6β1 and GPVI to interact with laminin, supporting integrin activation and resulting in stable adhesion and platelet aggregates formation (Inoue et al., 2006). This mechanism of platelet adhesion and activation is similar to platelet-collagen interaction; integrin α2β1 binding to collagen facilitates the interaction between GPVI and collagen. Further studies by Inoue et al. (2008) discovered that immobilized laminin promotes platelet recruitment under shear flow in a GPIbα-vWF dependent manner. White-Adams et al. demonstrated that laminin can activate FXII and that surface-associated laminin alone can trigger fibrin- and platelet-rich clots formation under shear (White-Adams et al., 2010). Therefore, the process of platelet recruitment, activation, and adhesion on laminin is mechanically similar to the interaction between platelets and collagen under shearing, and both can activate FXII, suggesting that laminin and collagen may jointly promote hemostasis.
Laminin is a weak platelet agonist, with a 10-fold lower affinity for the interaction between laminin and GPVI than collagen binding (Watson, 2009). Because superficial injury does not expose fibrous collagen, laminin-mediated platelet adhesion activation may be more effective. When the vessel wall is severely damaged, the exposed collagen in the deeper layers of the extracellular matrix collaborates with the superficial laminin to promote hemostasis. Moreover, laminin-111 can induce fibronectin assembly after adhesion to platelets via integrin α6β1. Therefore, laminin can indirectly affect hemostasis and thrombosis by regulating fibronectin deposition in thrombus (Cho and Mosher, 2006). As an important component of extracellular matrix hydrogel, laminin can collaborate with other hydrogel components to exert a hemostatic effect.
3.2.3 The role of fibronectin in hemastasis
Fibronectin (FN) is a dimeric protein composed of two approximately 250 kDa subunits that have many biological functions and is involved in cell migration, adhesion, proliferation, hemostasis, and tissue repair (Wang et al., 2008; Tong et al., 2016). FN is present as plasma Fibronectin (pFN) and cell Fibronectin (cFN) in extracellular connective tissue matrix and extracellular fluid (Roberts et al., 2020). pFN is produced by hepatocytes and endothelial cells in the liver and exists as a soluble non-complex molecule in the blood, whereas cFN is secreted and synthesized by fibroblast and mesenchymal cells and exists in the ECM as an insoluble polymer. Fibronectin is an essential component of tissue ECM, and it exists in two forms: as a soluble form in plasma and as an insoluble polymerized form. The main difference between the two forms of existence is that cFN has an extra domain A (EDA) and an extra domain B (EDB) compared to pFN(Wang et al., 2008).
The fibrillar cFN in the ECM is a strong prothrombotic surface that promotes platelet adhesion, aggregation, and coagulation. Fibrillar cFN effectively supports platelet adhesion, and adherent platelets can be activated to form a thrombus. This process depends on the integration of α5β1 and αIIbβ3, together with the GPIb-V-IX complexes, GPVI, and TLR4 (Maurer et al., 2015). Integrins α5β1 and αIIbβ3 ensure the initial phase of platelet adhesion to fibrillar cFN. Integrins can effectively promote platelet activation once platelets are attached to fibrillar cFn (McCarty et al., 2004; Lickert et al., 2022). GPIb-V-IX complex is a membrane protein component on the surface of platelets that plays a key role in platelet thrombosis initiation and coagulation. The binding of von Willebrand factor (VWF) to the platelet membrane glycoprotein (GP) Ib-IX-V complex initiates a signaling cascade that activates αIIbβ3 and causes platelet aggregation (Liu et al., 2005). As a fibronectin receptor, activated αIIbβ3 can further promote platelet adhesion and activation. Maurer et al. (2015) decreased thrombus formation on fibrillar cFN by antagonizing the binding of VWF and GPIb-V-IX complexes. GPVI is not only a collagen and laminin agonist but also a ligand and agonist for fibronectin, which, together with integrins, promotes platelet adhesion activation (Perrella et al., 2021). TLR4 is an EDA-binding receptor involved in platelet aggregation on fibrillar cFN, and the volume of thrombus on fibrillar cFN was reduced by using TLR4 blockers (Maurer et al., 2015; Prakash et al., 2015). Fibronectin can bind to various platelet receptors and play an important role in hemostasis and thrombosis.
3.2.4 The role of vitronectin in hemastasis
Vitronectin (VN) is a multifunctional 75-kDa glycoprotein present in plasma, extracellular matrix, and the α-granules of platelets (Bergmeier and Hynes, 2012). Vitronectin can not only improve platelet adhesion and aggregation during thrombus formation, but it also promotes thrombus stability. Ekmekci et al. (2002) discovered high vitronectin levels in growing thrombus, suggesting that it is actively involved in thrombosis after vascular injury. Vitronectin has two fibrin binding sites that have the potential to link fibrin monomers to polymers, binding them to fibrin clots to promote further platelet adhesion and aggregation (Schvartz et al., 2002). Platelets are covered by VN after initial binding to fibrin, and VN incorporated into fibrin clots enhances platelet adhesion and aggregation via the homotypic binding of VN molecules present on platelet surfaces and in clots. Wu et al. (2004) perfused whole blood onto a fibrin network made from purified fibrinogen, resulting in approximately 20% of the surface being covered with platelets binding purified polymeric VN to the fibrin network, resulting in a 2-fold increase in platelet surface coverage and enhanced platelet aggregate formation.
Plasminogen activator inhibitor-1 (PAI-1) can prevent fibrinolysis by inhibiting the conversion of plasminogen to plasmin. VN can bind to the β-sheet-A subunit of PAI-1, stabilize its structure, inhibit its spontaneous inactivation, and prolong its role in the fibrinolytic system, reducing the fibrinolysis of thrombi (Eitzman et al., 1995; Hess et al., 1995). Multiple protein components of ECM hydrogel could effectively induce platelet adhesion and aggregation to achieve hemostasis; vitronectin further promotes thrombogenesis in platelets by binding to fibrin sites. Moreover, thrombus stability was effectively enhanced by stabilizing the PAI-I structure and inhibiting thrombus degradation.
4 Conclusion and future perspectives
This study discusses the manufacturing methods, composition, safety, mechanical properties, and role of extracellular matrix proteins in the hemostasis of extracellular matrix hydrogels. With continued research and development, ECM hydrogel will be the most competitive material in the field of hemostasis. ECM hydrogels have developed a mature preparation and validation system to promote tissue regeneration, including material acquisition, acellular treatment, hydrogel formation, in vitro cytocompatibility experiment, in vivo regeneration experiment, and clinical trials. These experimental and theoretical findings facilitate the investigation of ECM hydrogels for hemostasis. Although ECM hydrogel has the potential to be hemostatic, significant experimental studies are required before clinical application. The formation and hemostatic function of the hydrogel primarily depend on extracellular matrix proteins. Protein contents vary between hydrogels from different sources and tissues, and the application of acellular reagents may destroy extracellular matrix proteins. Therefore, the hemostatic effects of hydrogels from various sources and tissues can be compared to select appropriate hemostatic agents. Optimizing the decellularization method and reducing the destructive effect of chemical reagents on ECM are essential processes. Combining tissue regeneration and hemostasis will be the focus of future research on ECM hydrogel. The primary focus of research is to promote tissue healing while enhancing the hemostatic effect. Although the hemostatic effect can be improved by introducing cross-linking agent or increasing extracellular matrix protein, hydrogel’s regeneration and repair effect is easily influenced. In conclusion, extracellular matrix hydrogels have shown immense potential in the field of hemostasis. However, further research and exploration are necessary fully realize its potential.
Author contributions
DC contributed to conception and design of the study. WW organized the database. All authors contributed to the article and approved the submitted version.
Funding
The author(s) disclosed receipt of the following financial support for the research, authorship, and/or publication of this article: This study was supported by Zhejiang Province Public Welfare Technology Application Research Project (CN), China (Grant No. LGF20H060009).
Conflict of interest
The authors declare that the research was conducted in the absence of any commercial or financial relationships that could be construed as a potential conflict of interest.
Publisher’s note
All claims expressed in this article are solely those of the authors and do not necessarily represent those of their affiliated organizations, or those of the publisher, the editors and the reviewers. Any product that may be evaluated in this article, or claim that may be made by its manufacturer, is not guaranteed or endorsed by the publisher.
References
Adam Young, D., Bajaj, V., and Christman, K. L. (2014). Award winner for outstanding research in the PhD category, 2014 Society for Biomaterials annual meeting and exposition, Denver, Colorado, April 16-19, 2014: Decellularized adipose matrix hydrogels stimulate in vivo neovascularization and adipose formation. J. Biomed. Mater. Res. Part A. 102 (6), 1641–1651. doi:10.1002/jbm.a.35109
Ahearne, M., and Coyle, A. (2016). Application of UVA-riboflavin crosslinking to enhance the mechanical properties of extracellular matrix derived hydrogels. J. Mech. Behav. Biomed. Mater. 54, 259–267. doi:10.1016/j.jmbbm.2015.09.035
Ali, M., Pr, A. K., Yoo, J. J., Zahran, F., Atala, A., and Lee, S. J. (2019). A photo-crosslinkable kidney ECM-derived bioink accelerates renal tissue formation. Adv. Healthc. Mater. 8 (7), e1800992. doi:10.1002/adhm.201800992
Attwood, S. J., Simpson, A. M., Hamaia, S. W., Bihan, D., Roy, D., Farndale, R. W., et al. (2013). Measurement of the interaction between recombinant I-domain from integrin alpha 2 beta 1 and a triple helical collagen peptide with the GFOGER binding motif using molecular force spectroscopy. Int. J. Mol. Sci. 14 (2), 2832–2845. doi:10.3390/ijms14022832
Badylak, S. F., and Gilbert, T. W. (2008). Immune response to biologic scaffold materials. Seminars Immunol. 20 (2), 109–116. doi:10.1016/j.smim.2007.11.003
Barber, F. A., Herbert, M. A., and Coons, D. A. (2006). Tendon augmentation grafts: Biomechanical failure loads and failure patterns. Arthrosc. J. Arthrosc. Relat. Surg. official Publ. Arthrosc. Assoc. N. Am. Int. Arthrosc. Assoc. 22 (5), 534–538. doi:10.1016/j.arthro.2005.12.021
Bergmeier, W., and Hynes, R. O. (2012). Extracellular matrix proteins in hemostasis and thrombosis. Cold Spring Harb. Perspect. Biol. 4 (2), a005132. doi:10.1101/cshperspect.a005132
Brown, B. N., Freund, J. M., Han, L., Rubin, J. P., Reing, J. E., Jeffries, E. M., et al. (2011). Comparison of three methods for the derivation of a biologic scaffold composed of adipose tissue extracellular matrix. Tissue Eng. Part C. Methods 17 (4), 411–421. doi:10.1089/ten.TEC.2010.0342
Brunner, M., Schneider, I., Günther, K., Grützmann, R., and Matzel, K. E. (2019). Permacol™ collagen paste for cryptoglandular and Crohn's anal fistula. Tech. coloproctology 23 (2), 135–141. doi:10.1007/s10151-019-01932-z
Burk, J., Erbe, I., Berner, D., Kacza, J., Kasper, C., Pfeiffer, B., et al. (2014). Freeze-thaw cycles enhance decellularization of large tendons. Tissue Eng. Part C Methods 20 (4), 276–284. doi:10.1089/ten.TEC.2012.0760
Cai, D., Chen, S., Wu, B., Chen, J., Tao, D., Li, Z., et al. (2021). Construction of multifunctional porcine acellular dermal matrix hydrogel blended with vancomycin for hemorrhage control, antibacterial action, and tissue repair in infected trauma wounds. Mater. today. Bio. 12, 100127. doi:10.1016/j.mtbio.2021.100127
Cao, J., Su, M., Hasan, N., Lee, J., Kwak, D., Kim, D. Y., et al. (2020). Nitric oxide-releasing thermoresponsive pluronic F127/alginate hydrogel for enhanced antibacterial activity and accelerated healing of infected wounds. Pharmaceutics 12 (10), 926. doi:10.3390/pharmaceutics12100926
Cartmell, J. S., and Dunn, M. G. (2000). Effect of chemical treatments on tendon cellularity and mechanical properties. J. Biomed. Mater. Res. 49 (1), 134–140. doi:10.1002/(sici)1097-4636(200001)49:1<134::aid-jbm17>3.0.co;2-d
Chaimov, D., Baruch, L., Krishtul, S., Meivar-Levy, I., Ferber, S., and Machluf, M. (2017). Innovative encapsulation platform based on pancreatic extracellular matrix achieve substantial insulin delivery. J. Control. release official J. Control. Release Soc. 257, 91–101. doi:10.1016/j.jconrel.2016.07.045
Chen, F. M., and Liu, X. (2016). Advancing biomaterials of human origin for tissue engineering. Prog. Polym. Sci. 53, 86–168. doi:10.1016/j.progpolymsci.2015.02.004
Chen, K. Y., Chen, Y. C., Lin, T. H., Yang, C. Y., Kuo, Y. W., and Lei, U. (2020). Hemostatic enhancement via chitosan is independent of classical clotting pathways-A quantitative study. Polymers 12 (10), 2391. doi:10.3390/polym12102391
Chen, Z., Zhang, B., Shu, J., Wang, H., Han, Y., Zeng, Q., et al. (2021). Human decellularized adipose matrix derived hydrogel assists mesenchymal stem cells delivery and accelerates chronic wound healing. J. Biomed. Mater. Res. Part A 109 (8), 1418–1428. doi:10.1002/jbm.a.37133
Cheng, C. W., Solorio, L. D., and Alsberg, E. (2014). Decellularized tissue and cell-derived extracellular matrices as scaffolds for orthopaedic tissue engineering. Biotechnol. Adv. 32 (2), 462–484. doi:10.1016/j.biotechadv.2013.12.012
Cheng, M. H., Uriel, S., Moya, M. L., Francis-Sedlak, M., Wang, R., Huang, J. J., et al. (2010). Dermis-derived hydrogels support adipogenesis in vivo. J. Biomed. Mater. Res. Part A. 92 (3), 852–858. doi:10.1002/jbm.a.32410
Chirco, K. R., Worthington, K. S., Flamme-Wiese, M. J., Riker, M. J., Andrade, J. D., Ueberheide, B. M., et al. (2017). Preparation and evaluation of human choroid extracellular matrix scaffolds for the study of cell replacement strategies. Acta biomater. 57, 293–303. doi:10.1016/j.actbio.2017.05.011
Cho, J., and Mosher, D. F. (2006). Characterization of fibronectin assembly by platelets adherent to adsorbed laminin-111. J. thrombosis haemostasis JTH 4 (5), 943–951. doi:10.1111/j.1538-7836.2006.01862.x
Christ, T., Grubitzsch, H., Claus, B., and Konertz, W. (2014). Long-term follow-up after aortic valve replacement with Edwards Prima Plus stentless bioprostheses in patients younger than 60 years of age. J. Thorac. Cardiovasc. Surg. 147 (1), 264–269. doi:10.1016/j.jtcvs.2012.10.032
Cooper, D. K. C., Gaston, R., Eckhoff, D., Ladowski, J., Yamamoto, T., Wang, L., et al. (2018). Xenotransplantation-the current status and prospects. Br. Med. Bull. 125 (1), 5–14. doi:10.1093/bmb/ldx043
Courtman, D. W., Pereira, C. A., Kashef, V., McComb, D., Lee, J. M., and Wilson, G. J. (1994). Development of a pericardial acellular matrix biomaterial: Biochemical and mechanical effects of cell extraction. J. Biomed. Mater. Res. 28 (6), 655–666. doi:10.1002/jbm.820280602
Crapo, P. M., and Wang, Y. (2010). Small intestinal submucosa gel as a potential scaffolding material for cardiac tissue engineering. Acta biomater. 6 (6), 2091–2096. doi:10.1016/j.actbio.2009.10.048
D'Andrea, G., Chetta, M., and Margaglione, M. (2009). Inherited platelet disorders: Thrombocytopenias and thrombocytopathies. Blood Transfus. = Trasfus. del sangue 7 (4), 278–292. doi:10.2450/2009.0078-08
Davidov, T., Efraim, Y., Hayam, R., Oieni, J., Baruch, L., and Machluf, M. (2021). Extracellular matrix hydrogels originated from different organs mediate tissue-specific properties and function. Int. J. Mol. Sci. 22 (21), 11624. doi:10.3390/ijms222111624
Demant, M., Lauritzen, E., Lang, C. L., Bredgaard, R., and Gramkow, C. (2022). A case of disintegrated Strattice™ 4 years after immediate breast reconstruction. Ann. R. Coll. Surg. Engl. 104 (3), e57–e59. doi:10.1308/rcsann.2021.0107
Deng, Y., Ren, J., Chen, G., Li, G., Wu, X., Wang, G., et al. (2017). Injectable in situ cross-linking chitosan-hyaluronic acid based hydrogels for abdominal tissue regeneration. Sci. Rep. 7 (1), 2699. doi:10.1038/s41598-017-02962-z
DeQuach, J. A., Mezzano, V., Miglani, A., Lange, S., Keller, G. M., Sheikh, F., et al. (2010). Simple and high yielding method for preparing tissue specific extracellular matrix coatings for cell culture. PloS one 5 (9), e13039. doi:10.1371/journal.pone.0013039
Ebrahimi Sadrabadi, A., Baei, P., Hosseini, S., and Baghaban Eslaminejad, M. (2021). Decellularized extracellular matrix as a potent natural biomaterial for regenerative medicine. Adv. Exp. Med. Biol. 1341, 27–43. doi:10.1007/5584_2020_504
Eitzman, D. T., Fay, W. P., Lawrence, D. A., Francis-Chmura, A. M., Shore, J. D., Olson, S. T., et al. (1995). Peptide-mediated inactivation of recombinant and platelet plasminogen activator inhibitor-1 in vitro. J. Clin. investigation 95 (5), 2416–2420. doi:10.1172/JCI117937
Ekmekci, H., Sonmez, H., Ekmekci, O. B., Ozturk, Z., Domanic, N., and Kokoglu, E. (2002). Plasma vitronectin levels in patients with coronary atherosclerosis are increased and correlate with extent of disease. J. thrombosis thrombolysis 14 (3), 221–225. doi:10.1023/a:1025000810466
Ellis-Behnke, R. (2011). At the nanoscale: Nanohemostat, a new class of hemostatic agent. Wiley Interdiscip. Rev. Nanomedicine nanobiotechnology. 3 (1), 70–78. doi:10.1002/wnan.110
Fan, X., Li, M., Yang, Q., Wan, G., Li, Y., Li, N., et al. (2021). Morphology-controllable cellulose/chitosan sponge for deep wound hemostasis with surfactant and pore-foaming agent. Mater. Sci. Eng. C, Mater. Biol. Appl. 118, 111408. doi:10.1016/j.msec.2020.111408
Fernández-Pérez, J., and Ahearne, M. (2019). The impact of decellularization methods on extracellular matrix derived hydrogels. Sci. Rep. 9 (1), 14933. doi:10.1038/s41598-019-49575-2
Freytes, D. O., Martin, J., Velankar, S. S., Lee, A. S., and Badylak, S. F. (2008). Preparation and rheological characterization of a gel form of the porcine urinary bladder matrix. Biomaterials 29 (11), 1630–1637. doi:10.1016/j.biomaterials.2007.12.014
Fu, W., Xu, P., Feng, B., Lu, Y., Bai, J., Zhang, J., et al. (2019). A hydrogel derived from acellular blood vessel extracellular matrix to promote angiogenesis. J. biomaterials Appl. 33 (10), 1301–1313. doi:10.1177/0885328219831055
Fu, Y., Fan, X., Tian, C., Luo, J., Zhang, Y., Deng, L., et al. (2016). Decellularization of porcine skeletal muscle extracellular matrix for the formulation of a matrix hydrogel: A preliminary study. J. Cell. Mol. Med. 20 (4), 740–749. doi:10.1111/jcmm.12776
Fuller, M. K., Faulk, D. M., Sundaram, N., Mahe, M. M., Stout, K. M., von Furstenberg, R. J., et al. (2013). Intestinal stem cells remain viable after prolonged tissue storage. Cell. tissue Res. 354 (2), 441–450. doi:10.1007/s00441-013-1674-y
Gaetani, R., Aude, S., DeMaddalena, L. L., Strassle, H., Dzieciatkowska, M., Wortham, M., et al. (2018). Evaluation of different decellularization protocols on the generation of pancreas-derived hydrogels. Tissue Eng. Part C. Methods 24 (12), 697–708. doi:10.1089/ten.TEC.2018.0180
Gao, L., Li, X., Tan, R., Cui, J., and Schmull, S. (2022). Human-derived decellularized extracellular matrix scaffold incorporating autologous bone marrow stem cells from patients with congenital heart disease for cardiac tissue engineering. Bio-medical Mater. Eng. 33 (5), 407–421. doi:10.3233/BME-211368
Getova, V. E., van Dongen, J. A., Brouwer, L. A., and Harmsen, M. C. (2019). Adipose tissue-derived ECM hydrogels and their use as 3D culture scaffold. Artif. cells, nanomedicine, Biotechnol. 47 (1), 1693–1701. doi:10.1080/21691401.2019.1608215
Ghuman, H., Mauney, C., Donnelly, J., Massensini, A. R., Badylak, S. F., and Modo, M. (2018). Biodegradation of ECM hydrogel promotes endogenous brain tissue restoration in a rat model of stroke. Acta biomater. 80, 66–84. doi:10.1016/j.actbio.2018.09.020
Gimbrone, M. A., Topper, J. N., Nagel, T., Anderson, K. R., and Garcia-Cardeña, G. (2000). Endothelial dysfunction, hemodynamic forces, and atherogenesisa. Ann. N. Y. Acad. Sci. 902, 230–240. doi:10.1111/j.1749-6632.2000.tb06318.x
Grover, G. N., Rao, N., and Christman, K. L. (2014). Myocardial matrix-polyethylene glycol hybrid hydrogels for tissue engineering. Nanotechnology 25 (1), 014011. doi:10.1088/0957-4484/25/1/014011
Guan, Y., Yang, B., Xu, W., Li, D., Wang, S., Ren, Z., et al. (2022). Cell-derived extracellular matrix materials for tissue engineering. Tissue Eng. Part B, Rev. 28 (5), 1007–1021. doi:10.1089/ten.TEB.2021.0147
Gupta, S. K., Mishra, N. C., and Dhasmana, A. (2018). Decellularization methods for scaffold fabrication. Methods Mol. Biol. Clift. N.J.). 1577, 1–10. doi:10.1007/7651_2017_34
Halper, J. (2021). Basic components of connective tissues and extracellular matrix: Fibronectin, fibrinogen, laminin, elastin, fibrillins, fibulins, matrilins, tenascins and thrombospondins. Adv. Exp. Med. Biol. 1348, 105–126. doi:10.1007/978-3-030-80614-9_4
Hein, T. W., Qamirani, E., Ren, Y., and Kuo, L. (2009). C-Reactive protein impairs coronary arteriolar dilation to prostacyclin synthase activation: Role of peroxynitrite. J. Mol. Cell. Cardiol. 47 (2), 196–202. doi:10.1016/j.yjmcc.2009.04.015
Hess, S., Kanse, S. M., Kost, C., and Preissner, K. T. (1995). The versatility of adhesion receptor ligands in haemostasis: Morpho-regulatory functions of vitronectin. Thrombosis haemostasis 74 (1), 258–265. doi:10.1055/s-0038-1642687
Hewawasam, R. S., Blomberg, R., Šerbedžija, P., and Magin, C. M. (2023). Chemical modification of human decellularized extracellular matrix for incorporation into phototunable hybrid-hydrogel models of tissue fibrosis. ACS Appl. Mater. interfaces 15 (12), 15071–15083. doi:10.1021/acsami.2c18330
Higaki, Y., Schullery, D., Kawata, Y., Shnyreva, M., Abrass, C., and Bomsztyk, K. (2002). Synergistic activation of the rat laminin gamma1 chain promoter by the gut-enriched Kruppel-like factor (GKLF/KLF4) and Sp1. Nucleic acids Res. 30 (11), 2270–2279. doi:10.1093/nar/30.11.2270
Holmes, C., Wrobel, J. S., Maceachern, M. P., and Boles, B. R. (2013). Collagen-based wound dressings for the treatment of diabetes-related foot ulcers: A systematic review. Diabetes, metabolic syndrome Obes. targets Ther. 6, 17–29. doi:10.2147/DMSO.S36024
Hu, J., Altun, I., Zhang, Z., Albadawi, H., Salomao, M. A., Mayer, J. L., et al. (2020). Bioactive-tissue-derived nanocomposite hydrogel for permanent arterial embolization and enhanced vascular healing. Adv. Mater. Deerf. Beach, Fla.) 32 (33), e2002611. doi:10.1002/adma.202002611
Huh, M. I., Lee, K. P., Kim, J., Yi, S., Park, B. U., and Kim, H. K. (2018). Generation of femtosecond laser-cut decellularized corneal lenticule using hypotonic trypsin-EDTA solution for corneal tissue engineering.J. Ophthalmol. 2018, 1–12. doi:10.1155/2018/2590536
Inoue, O., Suzuki-Inoue, K., McCarty, O. J., Moroi, M., Ruggeri, Z. M., Kunicki, T. J., et al. (2006). Laminin stimulates spreading of platelets through integrin α6β1–dependent activation of GPVI. Blood 107 (4), 1405–1412. doi:10.1182/blood-2005-06-2406
Inoue, O., Suzuki-Inoue, K., and Ozaki, Y. (2008). Redundant mechanism of platelet adhesion to laminin and collagen under flow: Involvement of von Willebrand factor and glycoprotein ib-IX-V. J. Biol. Chem. 283 (24), 16279–16282. doi:10.1074/jbc.C700241200
Jamieson, W. R., Riess, F. C., Raudkivi, P. J., Metras, J., Busse, E. F., Goldstein, J., et al. (2011). Medtronic mosaic porcine bioprosthesis: Assessment of 12-year performance. J. Thorac. Cardiovasc. Surg. 142 (2), 302–307.e2. doi:10.1016/j.jtcvs.2010.08.090
Jarvis, G. E., Raynal, N., Langford, J. P., Onley, D. J., Andrews, A., Smethurst, P. A., et al. (2008). Identification of a major GpVI-binding locus in human type III collagen. Blood 111 (10), 4986–4996. doi:10.1182/blood-2007-08-108472
Jeffords, M. E., Wu, J., Shah, M., Hong, Y., and Zhang, G. (2015). Tailoring material properties of cardiac matrix hydrogels to induce endothelial differentiation of human mesenchymal stem cells. ACS Appl. Mater. interfaces 7 (20), 11053–11061. doi:10.1021/acsami.5b03195
Johnson, T. D., Dequach, J. A., Gaetani, R., Ungerleider, J., Elhag, D., Nigam, V., et al. (2014). Human versus porcine tissue sourcing for an injectable myocardial matrix hydrogel. Biomaterials Sci. 2, 735–744. doi:10.1039/C3BM60283D
Johnson, T. D., Hill, R. C., Dzieciatkowska, M., Nigam, V., Behfar, A., Christman, K. L., et al. (2016). Quantification of decellularized human myocardial matrix: A comparison of six patients. Clin. Appl. 10 (1), 75–83. doi:10.1002/prca.201500048
Kao, C. Y., Nguyen, H. Q., and Weng, Y. C. (2020). Characterization of porcine urinary bladder matrix hydrogels from sodium dodecyl sulfate decellularization method. Polymers 12 (12), 3007. doi:10.3390/polym12123007
Kasimir, M. T., Rieder, E., Seebacher, G., Silberhumer, G., Wolner, E., Weigel, G., et al. (2003). Comparison of different decellularization procedures of porcine heart valves. Int. J. Artif. organs 26 (5), 421–427. doi:10.1177/039139880302600508
Kellaway, S. C., Roberton, V., Jones, J. N., Loczenski, R., Phillips, J. B., and White, L. J. (2023). Engineered neural tissue made using hydrogels derived from decellularised tissues for the regeneration of peripheral nerves. Acta biomater. 157, 124–136. doi:10.1016/j.actbio.2022.12.003
Khazaal, J., Ragagni, M., Parker, J., Timek, T., Murphy, E., Heiser, J., et al. (2022). Freestyle aortic bioprostheses in patients 60 Years old and younger. Seminars Thorac. Cardiovasc. Surg. 34 (3), 870–877. doi:10.1053/j.semtcvs.2021.08.003
Kim, K., and Kim, M. S. (2016). An injectable hydrogel derived from small intestine submucosa as a stem cell carrier. J. Biomed. Mater. Res. Part B, Appl. biomaterials 104 (8), 1544–1550. doi:10.1002/jbm.b.33504
Kim, S., Min, S., Choi, Y. S., Jo, S. H., Jung, J. H., Han, K., et al. (2022). Tissue extracellular matrix hydrogels as alternatives to Matrigel for culturing gastrointestinal organoids. Nat. Commun. 13 (1), 1692. doi:10.1038/s41467-022-29279-4
Kimsa, M. C., Strzalka-Mrozik, B., Kimsa, M. W., Gola, J., Nicholson, P., Lopata, K., et al. (2014). Porcine endogenous retroviruses in xenotransplantation--molecular aspects. Viruses 6 (5), 2062–2083. doi:10.3390/v6052062
Kobayashi, M., Kadota, J., Hashimoto, Y., Fujisato, T., Nakamura, N., Kimura, T., et al. (2020). Elastic modulus of ECM hydrogels derived from decellularized tissue affects capillary network formation in endothelial cells. Int. J. Mol. Sci. 21 (17), 6304. doi:10.3390/ijms21176304
Kuljanin, M., Brown, C. F. C., Raleigh, M. J., Lajoie, G. A., and Flynn, L. E. (2017). Collagenase treatment enhances proteomic coverage of low-abundance proteins in decellularized matrix bioscaffolds. Biomaterials 144, 130–143. doi:10.1016/j.biomaterials.2017.08.012
Labay, C., Hamouda, I., Tampieri, F., Ginebra, M. P., and Canal, C. (2019). Production of reactive species in alginate hydrogels for cold atmospheric plasma-based therapies. Sci. Rep. 9 (1), 16160. doi:10.1038/s41598-019-52673-w
Lau, T. T., Lee, L. Q., Vo, B. N., Su, K., and Wang, D. A. (2012). Inducing ossification in an engineered 3D scaffold-free living cartilage template. Biomaterials 33 (33), 8406–8417. doi:10.1016/j.biomaterials.2012.08.025
Leonhardt, E. E., Kang, N., Hamad, M. A., Wooley, K. L., and Elsabahy, M. (2019). Absorbable hemostatic hydrogels comprising composites of sacrificial templates and honeycomb-like nanofibrous mats of chitosan. Nat. Commun. 10 (1), 2307. doi:10.1038/s41467-019-10290-1
Liang, R., Knight, K., Easley, D., Palcsey, S., Abramowitch, S., and Moalli, P. A. (2017). Towards rebuilding vaginal support utilizing an extracellular matrix bioscaffold. Acta biomater. 57, 324–333. doi:10.1016/j.actbio.2017.05.015
Lickert, S., Kenny, M., Selcuk, K., Mehl, J. L., Bender, M., Früh, S. M., et al. (2022). Platelets drive fibronectin fibrillogenesis using integrin αIIbβ3. Sci. Adv. 8 (10), eabj8331. doi:10.1126/sciadv.abj8331
Lin, C. Y., Liu, T. Y., Chen, M. H., Sun, J. S., and Chen, M. H. (2016). An injectable extracellular matrix for the reconstruction of epidural fat and the prevention of epidural fibrosis. Biomed. Mater. (Bristol, Engl. 11 (3), 035010. doi:10.1088/1748-6041/11/3/035010
Lin, H. J., Wang, T. J., Li, T. W., Chang, Y. Y., Sheu, M. T., Huang, Y. Y., et al. (2019). Development of decellularized cornea by organic acid treatment for corneal regeneration. Tissue Eng. Part A 25 (7-8), 652–662. doi:10.1089/ten.TEA.2018.0162
Liu, J., Pestina, T. I., Berndt, M. C., Jackson, C. W., and Gartner, T. K. (2005). Botrocetin/VWF-induced signaling through GPIb-IX-V produces TxA2 in an αIIbβ3-and aggregation-independent manner. Blood 106 (8), 2750–2756. doi:10.1182/blood-2005-04-1667
Liu, X., Gan, H., Hu, C., Sun, W., Zhu, X., Meng, Z., et al. (2018). Silver sulfadiazine nanosuspension-loaded thermosensitive hydrogel as a topical antibacterial agent. Int. J. nanomedicine 14, 289–300. doi:10.2147/IJN.S187918
Loneker, A. E., Faulk, D. M., Hussey, G. S., D'Amore, A., and Badylak, S. F. (2016). Solubilized liver extracellular matrix maintains primary rat hepatocyte phenotype in-vitro. J. Biomed. Mater. Res. Part A. 104 (4), 957–965. doi:10.1002/jbm.a.35636
López-Martínez, S., Campo, H., de Miguel-Gómez, L., Faus, A., Navarro, A. T., Díaz, A., et al. (2021a). A natural xenogeneic endometrial extracellular matrix hydrogel toward improving current human in vitro models and future in vivo applications. Front. Bioeng. Biotechnol. 9, 639688. doi:10.3389/fbioe.2021.639688
López-Martínez, S., Rodríguez-Eguren, A., de Miguel-Gómez, L., Francés-Herrero, E., Faus, A., Díaz, A., et al. (2021b). Bioengineered endometrial hydrogels with growth factors promote tissue regeneration and restore fertility in murine models. Acta biomater. 135, 113–125. doi:10.1016/j.actbio.2021.08.025
Manon-Jensen, T., Kjeld, N. G., and Karsdal, M. A. (2016). Collagen-mediated hemostasis. J. thrombosis haemostasis JTH. 14 (3), 438–448. doi:10.1111/jth.13249
Mao, X., Yao, L., Li, M., Zhang, X., Weng, B., Zhu, W., et al. (2022). Enhancement of tendon repair using tendon-derived stem cells in small intestinal submucosa via M2 macrophage polarization. Cells 11 (17), 2770. doi:10.3390/cells11172770
Maurer, E., Schaff, M., Receveur, N., Bourdon, C., Mercier, L., Nieswandt, B., et al. (2015). Fibrillar cellular fibronectin supports efficient platelet aggregation and procoagulant activity. Thrombosis haemostasis 114 (6), 1175–1188. doi:10.1160/TH14-11-0958
McCarty, O. J., Zhao, Y., Andrew, N., Machesky, L. M., Staunton, D., Frampton, J., et al. (2004). Evaluation of the role of platelet integrins in fibronectin-dependent spreading and adhesion. J. thrombosis haemostasis JTH. 2 (10), 1823–1833. doi:10.1111/j.1538-7836.2004.00925.x
Medberry, C. J., Crapo, P. M., Siu, B. F., Carruthers, C. A., Wolf, M. T., Nagarkar, S. P., et al. (2013). Hydrogels derived from central nervous system extracellular matrix. Biomaterials 34 (4), 1033–1040. doi:10.1016/j.biomaterials.2012.10.062
Meder, T., Prest, T., Skillen, C., Marchal, L., Yupanqui, V. T., Soletti, L., et al. (2021). Nerve-specific extracellular matrix hydrogel promotes functional regeneration following nerve gap injury. NPJ Regen. Med. 6 (1), 69. doi:10.1038/s41536-021-00174-8
Mendoza-Novelo, B., Avila, E. E., Cauich-Rodríguez, J. V., Jorge-Herrero, E., Rojo, F. J., Guinea, G. V., et al. (2011). Decellularization of pericardial tissue and its impact on tensile viscoelasticity and glycosaminoglycan content. Acta biomater. 7 (3), 1241–1248. doi:10.1016/j.actbio.2010.11.017
Okada, M., Payne, T. R., Oshima, H., Momoi, N., Tobita, K., and Huard, J. (2010). Differential efficacy of gels derived from small intestinal submucosa as an injectable biomaterial for myocardial infarct repair. Biomaterials 31 (30), 7678–7683. doi:10.1016/j.biomaterials.2010.06.056
Ozpinar, E. W., Frey, A. L., Arthur, G. K., Mora-Navarro, C., Biehl, A., Snider, D. B., et al. (2021). Dermal extracellular matrix-derived hydrogels as an in vitro substrate to study mast cell maturation. Tissue Eng. Part A 27 (15-16), 1008–1022. doi:10.1089/ten.TEA.2020.0142
Palomino-Durand, C., Lopez, M., Cazaux, F., Martel, B., Blanchemain, N., and Chai, F. (2019). Influence of the Soluble⁻Insoluble ratios of cyclodextrins polymers on the viscoelastic properties of injectable Chitosan⁻Based hydrogels for biomedical application. Polymers 11 (2), 214. doi:10.3390/polym11020214
Parthiban, S. P., Athirasala, A., Tahayeri, A., Abdelmoniem, R., George, A., and Bertassoni, L. E. (2021). BoneMA-synthesis and characterization of a methacrylated bone-derived hydrogel for bioprinting ofin-vitrovascularized tissue constructs. Biofabrication 13 (3), 035031. doi:10.1088/1758-5090/abb11f
Pati, F., Jang, J., Ha, D. H., Won Kim, S., Rhie, J. W., Shim, J. H., et al. (2014). Printing three-dimensional tissue analogues with decellularized extracellular matrix bioink. Nat. Commun. 5, 3935. doi:10.1038/ncomms4935
Paulo Zambon, J., Atala, A., and Yoo, J. J. (2020). Methods to generate tissue-derived constructs for regenerative medicine applications. Methods (San Diego, Calif.) 171, 3–10. doi:10.1016/j.ymeth.2019.09.016
Peloso, A., Urbani, L., Cravedi, P., Katari, R., Maghsoudlou, P., Fallas, M. E., et al. (2016). The human pancreas as a source of protolerogenic extracellular matrix scaffold for a new-generation bioartificial endocrine pancreas. Ann. Surg. 264 (1), 169–179. doi:10.1097/SLA.0000000000001364
Perrella, G., Nagy, M., Watson, S. P., and Heemskerk, J. W. M. (2021). Platelet GPVI (glycoprotein VI) and thrombotic complications in the venous system. Arteriosclerosis, thrombosis, Vasc. Biol. 41 (11), 2681–2692. doi:10.1161/ATVBAHA.121.316108
Pilipchuk, S. P., Vaicik, M. K., Larson, J. C., Gazyakan, E., Cheng, M. H., and Brey, E. M. (2013). Influence of crosslinking on the stiffness and degradation of dermis-derived hydrogels. J. Biomed. Mater. Res. Part A. 101 (10), 2883–2895. doi:10.1002/jbm.a.34602
Poon, C. J., Pereira E Cotta, M. V., Sinha, S., Palmer, J. A., Woods, A. A., Morrison, W. A., et al. (2013). Preparation of an adipogenic hydrogel from subcutaneous adipose tissue. Acta biomater. 9 (3), 5609–5620. doi:10.1016/j.actbio.2012.11.003
Pourshahrestani, S., Zeimaran, E., Kadri, N. A., Mutlu, N., and Boccaccini, A. R. (2020). Polymeric hydrogel systems as emerging biomaterial platforms to enable hemostasis and wound healing. Adv. Healthc. Mater. 9 (20), 2000905. doi:10.1002/adhm.202000905
Prakash, P., Kulkarni, P. P., Lentz, S. R., and Chauhan, A. K. (2015). Cellular fibronectin containing extra domain A promotes arterial thrombosis in mice through platelet Toll-like receptor 4. Blood 125 (20), 3164–3172. doi:10.1182/blood-2014-10-608653
Rahman, S., Griffin, M., Naik, A., Szarko, M., and Butler, P. E. M. (2018). Optimising the decellularization of human elastic cartilage with trypsin for future use in ear reconstruction. Sci. Rep. 8 (1), 3097. doi:10.1038/s41598-018-20592-x
Ramzan, F., Ekram, S., Frazier, T., Salim, A., Mohiuddin, O. A., and Khan, I. (2022). Decellularized human umbilical tissue-derived hydrogels promote proliferation and chondrogenic differentiation of mesenchymal stem cells. Bioeng. (Basel, Switz. 9 (6), 239. doi:10.3390/bioengineering9060239
Roberts, A. L., Mavlyutov, T. A., Perlmutter, T. E., Curry, S. M., Harris, S. L., Chauhan, A. K., et al. (2020). Fibronectin extra domain A (FN-EDA) elevates intraocular pressure through Toll-like receptor 4 signaling. Sci. Rep. 10 (1), 9815. doi:10.1038/s41598-020-66756-6
Sackett, S. D., Tremmel, D. M., Ma, F., Feeney, A. K., Maguire, R. M., Brown, M. E., et al. (2018). Extracellular matrix scaffold and hydrogel derived from decellularized and delipidized human pancreas. Sci. Rep. 8 (1), 10452. doi:10.1038/s41598-018-28857-1
Sadeghian, A., Kharaziha, M., and Khoroushi, M. (2023). Dentin extracellular matrix loaded bioactive glass/GelMA support rapid bone mineralization for potential pulp regeneration. Int. J. Biol. Macromol. 234, 123771. doi:10.1016/j.ijbiomac.2023.123771
Saldin, L. T., Cramer, M. C., Velankar, S. S., White, L. J., and Badylak, S. F. (2017). Extracellular matrix hydrogels from decellularized tissues: Structure and function. Acta biomater. 49, 1–15. doi:10.1016/j.actbio.2016.11.068
Sarratt, K. L., Chen, H., Zutter, M. M., Santoro, S. A., Hammer, D. A., and Kahn, M. L. (2005). GPVI and α2β1 play independent critical roles during platelet adhesion and aggregate formation to collagen under flow. Blood 106 (4), 1268–1277. doi:10.1182/blood-2004-11-4434
Sawkins, M. J., Bowen, W., Dhadda, P., Markides, H., Sidney, L. E., Taylor, A. J., et al. (2013). Hydrogels derived from demineralized and decellularized bone extracellular matrix. Acta biomater. 9 (8), 7865–7873. doi:10.1016/j.actbio.2013.04.029
Schvartz, I., Seger, D., Maik-Rachline, G., Kreizman, T., and Shaltiel, S. (2002). Truncated vitronectins: Binding to immobilized fibrin and to fibrin clots, and their subsequent interaction with cells. Biochem. biophysical Res. Commun. 290 (2), 682–689. doi:10.1006/bbrc.2001.6273
Seif-Naraghi, S. B., Singelyn, J. M., Salvatore, M. A., Osborn, K. G., Wang, J. J., Sampat, U., et al. (2013). Safety and efficacy of an injectable extracellular matrix hydrogel for treating myocardial infarction. Sci. Transl. Med. 5 (173), 173ra25. doi:10.1126/scitranslmed.3005503
Sellaro, T. L., Ranade, A., Faulk, D. M., McCabe, G. P., Dorko, K., Badylak, S. F., et al. (2010). Maintenance of human hepatocyte function in vitro by liver-derived extracellular matrix gels. Tissue Eng. Part a. 16 (3), 1075–1082. doi:10.1089/ten.TEA.2008.0587
Sengyoku, H., Tsuchiya, T., Obata, T., Doi, R., Hashimoto, Y., Ishii, M., et al. (2018). Sodium hydroxide based non-detergent decellularizing solution for rat lung. Organogenesis 14 (2), 94–106. doi:10.1080/15476278.2018.1462432
Seo, Y., Jeong, S., Chung, J. J., Kim, S. H., Choi, N., and Jung, Y. (2020). Development of an anisotropically organized brain dECM hydrogel-based 3D neuronal culture platform for recapitulating the brain microenvironment in vivo. ACS biomaterials Sci. Eng. 6 (1), 610–620. doi:10.1021/acsbiomaterials.9b01512
Sheridan, W. S., Duffy, G. P., and Murphy, B. P. (2012). Mechanical characterization of a customized decellularized scaffold for vascular tissue engineering. J. Mech. Behav. Biomed. Mater. 8, 58–70. doi:10.1016/j.jmbbm.2011.12.003
Shojarazavi, N., Mashayekhan, S., Pazooki, H., Mohsenifard, S., and Baniasadi, H. (2021). Alginate/cartilage extracellular matrix-based injectable interpenetrating polymer network hydrogel for cartilage tissue engineering. J. biomaterials Appl. 36 (5), 803–817. doi:10.1177/08853282211024020
Simões, I. N., Vale, P., Soker, S., Atala, A., Keller, D., Noiva, R., et al. (2017). Acellular urethra bioscaffold: Decellularization of whole urethras for tissue engineering applications. Sci. Rep. 7, 41934. doi:10.1038/srep41934
Simsa, R., Rothenbücher, T., Gürbüz, H., Ghosheh, N., Emneus, J., Jenndahl, L., et al. (2021). Brain organoid formation on decellularized porcine brain ECM hydrogels. PloS one 16 (1), 0245685. doi:10.1371/journal.pone.0245685
Singelyn, J. M., and Christman, K. L. (2011). Modulation of material properties of a decellularized myocardial matrix scaffold. Macromol. Biosci. 11 (6), 731–738. doi:10.1002/mabi.201000423
Tan, Q. W., Zhang, Y., Luo, J. C., Zhang, D., Xiong, B. J., Yang, J. Q., et al. (2017). Hydrogel derived from decellularized porcine adipose tissue as a promising biomaterial for soft tissue augmentation. J. Biomed. Mater. Res. Part A. 105 (6), 1756–1764. doi:10.1002/jbm.a.36025
Tong, Y. F., Liu, Y., Hu, Z. X., and Li, Z. C. (2016). Protocatechuic aldehyde inhibits TNF-α-induced fibronectin expression in human umbilical vein endothelial cells via a c-Jun N-terminal kinase dependent pathway. Exp. Ther. Med. 11 (1), 277–282. doi:10.3892/etm.2015.2896
Tour, G., Wendel, M., and Tcacencu, I. (2011). Cell-derived matrix enhances osteogenic properties of hydroxyapatite. Tissue Eng. Part A 17 (1-2), 127–137. doi:10.1089/ten.TEA.2010.0175
Traverse, J. H., Henry, T. D., Dib, N., Patel, A. N., Pepine, C., Schaer, G. L., et al. (2019). First-in-Man study of a cardiac extracellular matrix hydrogel in early and late myocardial infarction patients. JACC. Basic Transl. Sci. 4 (6), 659–669. doi:10.1016/j.jacbts.2019.07.012
Tremmel, D. M., Sackett, S. D., Feeney, A. K., Mitchell, S. A., Schaid, M. D., Polyak, E., et al. (2022). A human pancreatic ECM hydrogel optimized for 3-D modeling of the islet microenvironment. Sci. Rep. 12 (1), 7188. doi:10.1038/s41598-022-11085-z
Tsuchiya, T., Balestrini, J. L., Mendez, J., Calle, E. A., Zhao, L., and Niklason, L. E. (2014). Influence of pH on extracellular matrix preservation during lung decellularization. Tissue Eng. Part C. Methods. 20 (12), 1028–1036. doi:10.1089/ten.TEC.2013.0492
Tukmachev, D., Forostyak, S., Koci, Z., Zaviskova, K., Vackova, I., Vyborny, K., et al. (2016). Injectable extracellular matrix hydrogels as scaffolds for spinal cord injury repair. Tissue Eng. Part A 22 (3-4), 306–317. doi:10.1089/ten.TEA.2015.0422
Ungerleider, J. L., Johnson, T. D., Rao, N., and Christman, K. L. (2015). Fabrication and characterization of injectable hydrogels derived from decellularized skeletal and cardiac muscle. Methods (San Diego, Calif.) 84, 53–59. doi:10.1016/j.ymeth.2015.03.024
Uriel, S., Huang, J. J., Moya, M. L., Francis, M. E., Wang, R., Chang, S. Y., et al. (2008). The role of adipose protein derived hydrogels in adipogenesis. Biomaterials 29 (27), 3712–3719. doi:10.1016/j.biomaterials.2008.05.028
Valfrè, C., Rizzoli, G., Zussa, C., Ius, P., Polesel, E., Mirone, S., et al. (2006). Clinical results of Hancock II versus Hancock standard at long-term follow-up. J. Thorac. Cardiovasc. Surg. 132 (3), 595–601.e2. doi:10.1016/j.jtcvs.2006.03.062
van der Meijden, P. E., Munnix, I. C., Auger, J. M., Govers-Riemslag, J. W., Cosemans, J. M., Kuijpers, M. J., et al. (2009). Dual role of collagen in factor XII-dependent thrombus formation. Blood 114 (4), 881–890. doi:10.1182/blood-2008-07-171066
van Dongen, J. A., Getova, V., Brouwer, L. A., Liguori, G. R., Sharma, P. K., Stevens, H. P., et al. (2019). Adipose tissue-derived extracellular matrix hydrogels as a release platform for secreted paracrine factors. J. tissue Eng. Regen. Med. 13 (6), 973–985. doi:10.1002/term.2843
Ventura, R. D., Padalhin, A. R., Kim, B., Park, M., and Lee, B. T. (2020). Evaluation of bone regeneration potential of injectable extracellular matrix (ECM) from porcine dermis loaded with biphasic calcium phosphate (BCP) powder. Mater. Sci. Eng. C, Mater. Biol. Appl. 110, 110663. doi:10.1016/j.msec.2020.110663
Výborný, K., Vallová, J., Kočí, Z., Kekulová, K., Jiráková, K., Jendelová, P., et al. (2019). Genipin and EDC crosslinking of extracellular matrix hydrogel derived from human umbilical cord for neural tissue repair. Sci. Rep. 9 (1), 10674. doi:10.1038/s41598-019-47059-x
Wang, F., Shi, W., Li, H., Wang, H., Sun, D., Zhao, L., et al. (2020). Decellularized porcine cornea-derived hydrogels for the regeneration of epithelium and stroma in focal corneal defects. ocular Surf. 18 (4), 748–760. doi:10.1016/j.jtos.2020.07.020
Wang, Q., Chen, J., Wang, D., Shen, M., Ou, H., Zhao, J., et al. (2021). Rapid hemostatic biomaterial from a natural bath sponge skeleton. Mar. drugs 19 (4), 220. doi:10.3390/md19040220
Wang, S. Y., Sang, J. W., Ding, W., Qin, T. W., Bai, L., Zhang, J., et al. (2019). The cytoptrotection of small intestinal submucosa-derived gel in HL-1 cells during hypoxia/reoxygenation-induced injury. J. tissue Eng. Regen. Med. 13 (8), 1346–1361. doi:10.1002/term.2878
Wang, W., Zhang, X., Chao, N. N., Qin, T. W., Ding, W., Zhang, Y., et al. (2016a). Preparation and characterization of pro-angiogenic gel derived from small intestinal submucosa. Acta biomater. 29, 135–148. doi:10.1016/j.actbio.2015.10.013
Wang, X. Q., Chen, L., Pan, R., Zhao, J., Liu, Y., and He, R. Q. (2008). An earthworm protease cleaving serum fibronectin and decreasing HBeAg in HepG2.2.15 cells. BMC Biochem. 9, 30. doi:10.1186/1471-2091-9-30
Wang, Y., Gallant, R. C., and Ni, H. (2016b). Extracellular matrix proteins in the regulation of thrombus formation. Curr. Opin. Hematol. 23 (3), 280–287. doi:10.1097/MOH.0000000000000237
Wang, Z., Zhang, Y., Zhang, J., Huang, L., Liu, J., Li, Y., et al. (2014). Exploring natural silk protein sericin for regenerative medicine: An injectable, photoluminescent, cell-adhesive 3D hydrogel. Sci. Rep. 4, 7064. doi:10.1038/srep07064
Wassenaar, J. W., Gaetani, R., Garcia, J. J., Braden, R. L., Luo, C. G., Huang, D., et al. (2016). Evidence for mechanisms underlying the functional benefits of a myocardial matrix hydrogel for post-MI treatment. J. Am. Coll. Cardiol. 67 (9), 1074–1086. doi:10.1016/j.jacc.2015.12.035
Watson, S. P. (2009). Platelet activation by extracellular matrix proteins in haemostasis and thrombosis. Curr. Pharm. Des. 15 (12), 1358–1372. doi:10.2174/138161209787846702
White-Adams, T. C., Berny, M. A., Patel, I. A., Tucker, E. I., Gailani, D., Gruber, A., et al. (2010). Laminin promotes coagulation and thrombus formation in a factor XII-dependent manner. J. thrombosis haemostasis JTH 8 (6), 1295–1301. doi:10.1111/j.1538-7836.2010.03850.x
Wolf, M. T., Daly, K. A., Brennan-Pierce, E. P., Johnson, S. A., Carruthers, C. A., D'Amore, A., et al. (2012). A hydrogel derived from decellularized dermal extracellular matrix. Biomaterials 33 (29), 7028–7038. doi:10.1016/j.biomaterials.2012.06.051
Wu, J., Brazile, B., McMahan, S. R., Liao, J., and Hong, Y. (2019). Heart valve tissue-derived hydrogels: Preparation and characterization of mitral valve chordae, aortic valve, and mitral valve gels. J. Biomed. Mater. Res. Part B, Appl. biomaterials 107 (5), 1732–1740. doi:10.1002/jbm.b.34266
Wu, Y. P., Bloemendal, H. J., Voest, E. E., Logtenberg, T., de Groot, P. G., Gebbink, M. F., et al. (2004). Fibrin-incorporated vitronectin is involved in platelet adhesion and thrombus formation through homotypic interactions with platelet-associated vitronectin. Blood 104 (4), 1034–1041. doi:10.1182/blood-2003-12-4293
Xiao, J., Yang, W., Xu, B., Zhu, H., Zou, J., Su, C., et al. (2018). Expression of fibronectin in esophageal squamous cell carcinoma and its role in migration. BMC cancer 18 (1), 976. doi:10.1186/s12885-018-4850-3
Yang, J. L., Yao, X., Qing, Q., Zhang, Y., Jiang, Y. L., Ning, L. J., et al. (2018). An engineered tendon/ligament bioscaffold derived from decellularized and demineralized cortical bone matrix. J. Biomed. Mater. Res. Part A. 106 (2), 468–478. doi:10.1002/jbm.a.36261
Yang, X., Liu, W., Xi, G., Wang, M., Liang, B., Shi, Y., et al. (2019). Fabricating antimicrobial peptide-immobilized starch sponges for hemorrhage control and antibacterial treatment. Carbohydr. Polym. 222, 115012. doi:10.1016/j.carbpol.2019.115012
Yazdanpanah, G., Jiang, Y., Rabiee, B., Omidi, M., Rosenblatt, M. I., Shokuhfar, T., et al. (2021b). Fabrication, rheological, and compositional characterization of thermoresponsive hydrogel from cornea. Tissue Eng. Part C. Methods 27 (5), 307–321. doi:10.1089/ten.TEC.2021.0011
Yazdanpanah, G., Shah, R., Raghurama R Somala, S., Anwar, K. N., Shen, X., An, S., et al. (2021a). In-situ porcine corneal matrix hydrogel as ocular surface bandage. ocular Surf. 21, 27–36. doi:10.1016/j.jtos.2021.04.004
Zeitouni, S., Krause, U., Clough, B. H., Halderman, H., Falster, A., Blalock, D. T., et al. (2012). Human mesenchymal stem cell-derived matrices for enhanced osteoregeneration. Sci. Transl. Med. 4 (132), 132ra55. doi:10.1126/scitranslmed.3003396
Zhang, D., Tan, Q. W., Luo, J. C., and Lv, Q. (2018). Evaluating the angiogenic potential of a novel temperature-sensitive gel scaffold derived from porcine skeletal muscle tissue. Biomed. Mater. (Bristol, Engl. 13 (5), 055003. doi:10.1088/1748-605X/aac275
Zhang, H., Pan, D., Wu, X., Su, W., Tang, X., Zhao, D., et al. (2020). Platelet protease activated receptor 1 is involved in the hemostatic effect of 20(S)-Protopanaxadiol by regulating calcium signaling. Front. Pharmacol. 11, 549150. doi:10.3389/fphar.2020.549150
Zhang, W., Du, A., Liu, S., Lv, M., and Chen, S. (2021). Research progress in decellularized extracellular matrix-derived hydrogels. Regen. Ther. 18, 88–96. doi:10.1016/j.reth.2021.04.002
Zhao, Y., Fan, J., and Bai, S. (2019). Biocompatibility of injectable hydrogel from decellularized human adipose tissue in vitro and in vivo. J. Biomed. Mater. Res. Part B, Appl. biomaterials 107 (5), 1684–1694. doi:10.1002/jbm.b.34261
Zheng, C., Zeng, Q., Pimpi, S., Wu, W., Han, K., Dong, K., et al. (2020). Research status and development potential of composite hemostatic materials. J. Mater. Chem. B 8 (25), 5395–5410. doi:10.1039/d0tb00906g
Zhong, Y., Hu, H., Min, N., Wei, Y., Li, X., and Li, X. (2021). Application and outlook of topical hemostatic materials: A narrative review. Ann. Transl. Med. 9 (7), 577. doi:10.21037/atm-20-7160
Keywords: extracellular matrix hydrogel, hemostasis, hemostatic mechanism, extracellular matrix components (ECM), hemorrhage
Citation: Cai D and Weng W (2023) Development potential of extracellular matrix hydrogels as hemostatic materials. Front. Bioeng. Biotechnol. 11:1187474. doi: 10.3389/fbioe.2023.1187474
Received: 16 March 2023; Accepted: 02 June 2023;
Published: 13 June 2023.
Edited by:
Marta S. Carvalho, University of Lisbon, PortugalReviewed by:
Vipuil Kishore, Florida Institute of Technology, United StatesDiana Soares Da Costa, University of Minho, Portugal
Copyright © 2023 Cai and Weng. This is an open-access article distributed under the terms of the Creative Commons Attribution License (CC BY). The use, distribution or reproduction in other forums is permitted, provided the original author(s) and the copyright owner(s) are credited and that the original publication in this journal is cited, in accordance with accepted academic practice. No use, distribution or reproduction is permitted which does not comply with these terms.
*Correspondence: Wei Weng, Y2QxOTk0MjAyMkAxMjYuY29t