- 1Department of Biomedical Engineering, Universidad de Los Andes, Bogotá, Colombia
- 2Department of Chemical and Food Engineering, Universidad de Los Andes, Bogotá, Colombia
Microfluidics is an interdisciplinary field that encompasses both science and engineering, which aims to design and fabricate devices capable of manipulating extremely low volumes of fluids on a microscale level. The central objective of microfluidics is to provide high precision and accuracy while using minimal reagents and equipment. The benefits of this approach include greater control over experimental conditions, faster analysis, and improved experimental reproducibility. Microfluidic devices, also known as labs-on-a-chip (LOCs), have emerged as potential instruments for optimizing operations and decreasing costs in various of industries, including pharmaceutical, medical, food, and cosmetics. However, the high price of conventional prototypes for LOCs devices, generated in clean room facilities, has increased the demand for inexpensive alternatives. Polymers, paper, and hydrogels are some of the materials that can be utilized to create the inexpensive microfluidic devices covered in this article. In addition, we highlighted different manufacturing techniques, such as soft lithography, laser plotting, and 3D printing, that are suitable for creating LOCs. The selection of materials and fabrication techniques will depend on the specific requirements and applications of each individual LOC. This article aims to provide a comprehensive overview of the numerous alternatives for the development of low-cost LOCs to service industries such as pharmaceuticals, chemicals, food, and biomedicine.
1 Introduction
Labs-on-a-chip (LOCs), also known as microfluidic devices, have revolutionized biomedical and chemical analysis by providing efficient, portable, and highly miniaturized solutions (Najjar et al., 2022; Izadifar et al., 2023; Zhao et al., 2023). The microfluidic design of these devices enables precise control of fluid flows and processes, leading to improved accuracy and repeatability of results (Karthik et al., 2022; Verma & Pandya, 2022). However, their high material costs and specific manufacturing processes, which need cleanroom settings, make them inaccessible to many laboratories (Pandey et al., 2017; Walsh et al., 2017).
Silicon and glass are the most common materials utilized in the manufacture of microfluidic devices (Wang Y et al., 2021; Yagyu et al., 2022). Silicon is a popular choice due to its chemical compatibility, resistance, and design flexibility (da Ponte et al., 2021). While glass is transparent, biocompatible, and electrically insulating (Orazi et al., 2022; Shubhava et al., 2022). However, both materials are expensive to manufacture and require specialized equipment and cleanroom facilities to ensure their purity and reliability (Elvira et al., 2022; Leung et al., 2022).
To overcome these obstacles, this review presents a complete analysis of different materials and methods that can be employed to build low-cost LOCs. The aim of this review is to make the benefits of microfluidic devices accessible to a broader range of researchers and to encourage the field’s continued development. Alternatives to silicon and glass will be presented, including alternative materials and fabrication techniques that can be used to produce microfluidic devices at a reasonable cost (Campbell et al., 2021; Niculescu et al., 2021; Scott & Ali, 2021).
2 Materials
2.1 Paper (cellulose and cellulose derivatives)
Paper is a cellulosic material that has become increasingly popular for fabricating microfluidic devices due to its advantageous properties (Martinez et al., 2007; Tsagkaris et al., 2021; Zhuang et al., 2022). Its distinct characteristics, such as low cost and wide availability, make it an attractive option for mass production. (Gao B et al., 2019; Kumawat et al., 2022; Zhu Y et al., 2022). There are numerous fabrication techniques available for paper, such as laser cutting, flexographic printing, screen printing, wax-based printing, and alkyl ketene dimer (AKD) printing, which make it viable and cost effective (Olmos et al., 2019; Parween et al., 2020; Ardakani et al., 2022; Lin et al., 2022; Fiore et al., 2023).
Paper’s hydrophilicity enables fluid manipulation without pumps, and its ability to be functionalized provides additional advantages (Yetisen et al., 2013). Recent advances in surface modification techniques, such as molecular imprinting, electrochemistry, or immunoassay, have allowed precise modification of paper’s surface chemical properties, resulting in precise fluid flow control and enhanced functionality (Cate et al., 2015; Boonkaew et al., 2021; Zhu L et al., 2022; Wang et al., 2023). These advances have led to the development of point-of-care tests with integrated detection functionalities that exhibit fluorescent, electrochemical, and microfluidic colorimetric paper chips (Cincotto et al., 2019; Ma et al., 2020; Tran et al., 2021). However, paper’s weak mechanical strength restricts its application in high-pressure environments, and its vulnerability to degradation limits its use in harsh conditions (Yetisen et al., 2013; Carrell et al., 2019). Moreover, irregular porosity in the paper can cause inaccuracies in regulating fluid flow, leading to reduced precision in the obtained results Nishat et al., 2021; Qin et al., 2021). To address these challenges, there has been a recent trend in using synthetic microfluidic paper composed of polymers. This innovative material offers several advantages over traditional paper, including improved consistency, more favorable surface chemistry, predictable pore size, and greater control over physical properties (Hansson et al., 2016; Zhou et al., 2021).
In line with this trend, Lin et al. (2022) have developed a novel and cost-effective technique for creating microfluidic paper-based analytical devices (μPADs). These μPADs can perform multiplexed enzyme-linked immunosorbent assays (ELISAs) for the detection of two cancer biomarkers, namely, alpha fetoprotein (AFP) and carcinoembryonic antigen (CEA). The fabrication process of these μPADs involves using nitrocellulose (NC) membranes as a substrate and polyurethane acrylate (PUA) as a barrier material to define flow channels and reaction zones. The PUA is applied to the NC membrane using screen-printing and then cured under UV light to form a hydrophobic barrier. This process allows the formation of precise and consistent channels, which are essential for accurate fluid flow regulation and the detection of the cancer biomarkers (Lin et al., 2022).
2.2 Hydrogels
Hydrogels are a versatile class of materials composed of cross-linked polymeric chains that have the remarkable ability to store a significant amount of water (Sharma & Tiwari, 2020; Bento et al., 2023; Tevlek et al., 2023). One of their most notable characteristics is their high degree of porosity, which makes them highly responsive to external stimuli such as temperature, pH, and ionic strength (García-Torres et al., 2022; Camman et al., 2023). Numerous hydrogels possess a notably high permeability, facilitating cell proliferation. Additionally, these hydrogels exhibit optical transparency, allowing visualization of internal microfluidic device components. These features render hydrogels particularly well-suited for use in lab-on-a-chip and organs-on-a-chip systems, especially in the context of biosensing and drug delivery applications (Hou et al., 2017; Nie et al., 2020; Ma et al., 2022; Sood et al., 2022; Cao et al., 2023).
Hydrogels can be made from natural, synthetic, or hybrid materials, depending on the specific application (Mahinroosta et al., 2018; Ho et al., 2022). Natural materials such as gelatin, chitosan, silk, and collagen provide better biocompatibility, biodegradability, and mimicry of the extracellular matrix. However, their cross-linking behavior can limit their functionality (Kato et al., 2021; Vera et al., 2021; Zhou et al., 2022). In contrast, synthetic materials such as polyethylene glycol, polyvinyl alcohol, poly (n-isopropyl acrylamide), and poly (hydroxyethyl methacrylate) have superior mechanical strength and shape memory but lack biocompatibility (Bashir et al., 2020; Nie et al., 2020; Vera et al., 2021). Ongoing research focuses on developing new hydrogel materials and improving their properties to address existing limitations and enable their use in more complex applications (Park et al., 2021; Clancy et al., 2022). In line with this rationale, Grebenyuk et al. (2023) have designed a hydrogel for precise microvessel printing at scales below the diffusion limit of living tissues, enabling the culture of large-scale engineered tissues in vitro over long periods, while avoiding hypoxia or necrosis. The formulation uses a photopolymer based on polyethylene glycol diacrylate (PEGDA), which incorporates the photocrosslinker pentaerythritol triacrylate (PETA) to increase the polymer’s density via crosslinking. Additionally, Triton-X 100 is used as an inert filler to maintain sufficient porosity for rapid molecule diffusion (Grebenyuk et al., 2023).
2.3 Polydimethylsiloxane (PDMS)
Polydimethylsiloxane (PDMS) is the most commonly used elastomer due to its compatibility with biological samples, optical clarity, and ease of fabrication for valves and pumps (de Almeida Monteiro Melo Ferraz et al., 2020; Dabaghi et al., 2021; Yandrapalli et al., 2021; Crevillen et al., 2022; Mistretta et al., 2022; Natsuhara et al., 2022). However, one of the major drawbacks of PDMS is its tendency to swell in the presence of certain solvents, particularly hydrocarbons, which can causee device failure (Raj & Chakraborty, 2020; Miranda et al., 2021). Additionally, PDMS has adsorptive properties for certain molecules that can affect the accuracy of experiments or their analyses (Akther et al., 2020; Grant et al., 2021). Water evaporation through the channel walls is a concern because it can lead to alterations in solution concentration, negatively affecting experimental results (Schneider et al., 2021b; Xia et al., 2022). The hydrophobicity of PDMS can make it difficult to fill microfluidic channels with aqueous solutions, resulting in fluid flow and analyte detection difficulties (Akther et al., 2020; Hu H et al., 2020).
2.4 Thermoplastics
In recent years, there has been a noticeable trend towards utilizing thermoplastic materials for fabricating microfluidic devices. This trend has gained traction due to the distinct advantages of thermoplastics, including lower costs and faster production times, rendering them a viable option for scaling up production (Becker & Gärtner, 2000; Tsao, 2016). Among the polymers that have gained significant usage in microfluidics are Polycarbonate (PC), Polyethylene terephthalate (PET), Polyimide (PI), Polypropylene (PP), Polystyrene (PS), Cyclic olefin copolymers (COC), and Poly (methyl methacrylate) (PMMA) (Denecke et al., 2022; Lee et al., 2022; Ortegón et al., 2022; Persson et al., 2022; Matsuura & Takata, 2023; Thaweeskulchai & Schulte, 2023).
Polystyrene (PS) is a highly stable and non-polar linear polymer that contains benzene as a pendant group (Bhavsar et al., 2018; Shakeri et al., 2022a). Its exceptional properties, including high mechanical and thermal stability, chemical resistance, biocompatibility, and optical transparency, make it an attractive material for microfluidic device fabrication (de Oliveira et al., 2021; Zhou et al., 2021). Furthermore, PS substrates can have their optical properties adjusted by incorporating various dopants in their structure, making it possible to tailor the optical properties of PS substrates to suit specific applications in microfluidics (Bhavsar et al., 2018; Sivakumar & Lee, 2020). However, it is important to note that PS can undergo degradation or physical and chemical changes upon exposure to light of wavelengths below 380 nm (Bhavsar et al., 2018).
Cyclic olefin copolymers (COC) in particular, are a type of thermoplastic material composed of cyclic olefin monomers and linear olefins (Bruijns et al., 2020; Agha et al., 2022). COC possesses numerous advantageous properties, including low water absorption, excellent electrical insulation, long-term surface treatment stability, and resistance to a broad range of acids and solvents (Shakeri et al., 2022b; Guan et al., 2022). It is an ideal material for use in diverse applications, such as biological, membrane, and semiconductor fields (Etxeberria et al., 2022; Xia et al., 2023). COC is remarkable for its stiffness, optical clarity, and heat deflection temperature, which varies between 70°C and 170°C, depending on the COC grade (Bruijns et al., 2019; Agha et al., 2022). Microchannels in COC can be fabricated using a variety of processes, including micromilling, injection molding, and heat embossing (Gang et al., 2019; Shakeri et al., 2022b; Li et al., 2022; Qin Y et al., 2022).
PMMA, in particular, is an inexpensive thermoplastic polymer with excellent optical properties, such as high transparency and refractive index (Kulsharova et al., 2018; Vo & Chen, 2022). Because it is easy to manipulate, it is a suitable material for the fabrication of microfluidic devices (Horowitz et al., 2020; Persson et al., 2022; Madadi et al., 2023; Zolti et al., 2023). PMMA is also biocompatible and can be sterilized easily by various sterilization methods, such as autoclaving (Guzzi et al., 2020; Khot et al., 2020) and chemical sterilization (Nguyen et al., 2019; Ameri et al., 2022), making it useful for biotechnological and biomedical applications. However, PMMA has some limitations, including high hydrophobicity and low mechanical strength (Amirabad et al., 2022; Shakeri et al., 2022a; Kulkarni & Goel, 2022).
The trend of utilizing polymers for microfluidic device fabrication is evident in the pursuit of novel materials that can be effectively employed in microfluidic devices. For example, Kaya et al. (2022) have introduced programmable polymer magnetic composites that incorporate droplets of solid-liquid phase change material, each containing a single magnetic dipole particle. These composites can be reprogrammed into four different states: superparamagnetic, artificial spin ice, spin glass, and ferromagnetic. Also, they possess high remanence characteristics along with Curie temperatures below the composite’s degradation temperature (Kaya et al., 2022). The development of such innovative materials offers new tools that can be utilized in microfluidics to develop advanced devices and enhance the design of labs-on-a-chip.
2.5 Surface functionalization techniques
Various surface functionalization techniques have been developed to enhance the properties of polymers used in microfluidics devices, such as PDMS and PMMA (Shakeri et al., 2021). Plasma treatment is among these techniques, which has been shown to improve the wettability and adhesion to other materials (Sui et al., 2021; Gizer et al., 2023). In addition, techniques like chemical vapor deposition (CVD) and graft polymer coating can improve the surface’s chemical and mechanical stability (Dabaghi et al., 2019; Fan et al., 2019). Furthermore, protein adsorption and layer-by-layer (LBL) deposition teachniques can produce a biologically active surface and a multilayered coating, thereby enhancing the functionality of the devices (Babaei et al., 2022; Siddique et al., 2021; Z. Li et al., 2021). These techniques have significantly improved the properties of surfaces and expanded their potential applications in various fields, including biomedical research and point-of-care diagnostics (Y. He et al., 2022; Khemthongcharoen et al., 2021; Tang et al., 2021).
3 Manufacturing techniques
Conventional manufacturing techniques used for microfluidic devices require a clean room and generate high costs, which hinder their widespread adoption (Walsh et al., 2017; Shakeri et al., 2021). Consequently, there has been a growing interest in developing low-cost techniques to fabricate microfluidic devices, facilitating broader access and expediting integration into various applications (Li et al., 2022; Akhtar et al., 2023; Li et al., 2023).
In general, two types of techniques can be used to manufacture microfluidic devices at a low cost: subtractive and additive techniques (Niculescu et al., 2021; Scott & Ali, 2021; Ching et al., 2023). In subtractive techniques, material is removed from a substrate to create microchannels and other features, whereas in additive techniques, material is added layer by layer to build a three-dimensional structure (Bhatia & Sehgal, 2021; Kulkarni, Salve, et al., 2021; Mesquita et al., 2022). Common subtractive techniques include hot embossing and laser ablation, while common additive techniques include 3D printing, soft lithography, and inkjet printing (Bavendiek et al., 2020; Schneider et al., 2021a; Grebenyuk et al., 2023).
These techniques offer various advantages, including low-cost, rapid prototyping, and the ability to manufacture microfluidic devices with complex geometries (Chen et al., 2020; Santana et al., 2020). However, there are limitations to these methods that must be considered. This contribution will examine the advantages and disadvantages of these low-cost techniques.
3.1 Soft lithography
Soft lithography is a highly popular technique for developing microfluidic devices, which utilizes soft and elastomeric materials such as polydimethylsiloxane (PDMS) to create microfluidic patterns (Whitesides et al., 2001; Phiphattanaphiphop et al., 2020; Duc et al., 2021; Šakalys et al., 2021).
Figure 1 illustrates the key phases of the soft lithography method, beginning with the design of the microfluidic device using computer-aided design (CAD) software (Bressan et al., 2019; Sommonte et al., 2022). Subsequently, a patterned substrate known as the “master” is created using expensive techniques, which offer excellent resolution. These techniques include photolithography (250 nm), nanoimprint lithography (15 nm), x-ray lithography (15 nm), or electron beam lithography (10 nm), but are primarily used in large-scale manufacturing facilities (Maldonado & Peckerar, 2016; Gale et al., 2018; Thuau et al., 2018; Matsumoto et al., 2020; Phiphattanaphiphop et al., 2022). Consequently, there has been a growing interest in employing low-cost manufacturing techniques, including micromilling (25 μm), 3D printing (5–100 μm), and laser cutting (25 μm) (Gale et al., 2018; Rusling, 2018; Hamilton et al., 2021; Šakalys et al., 2021; Preetam et al., 2022; Qin S et al., 2022).
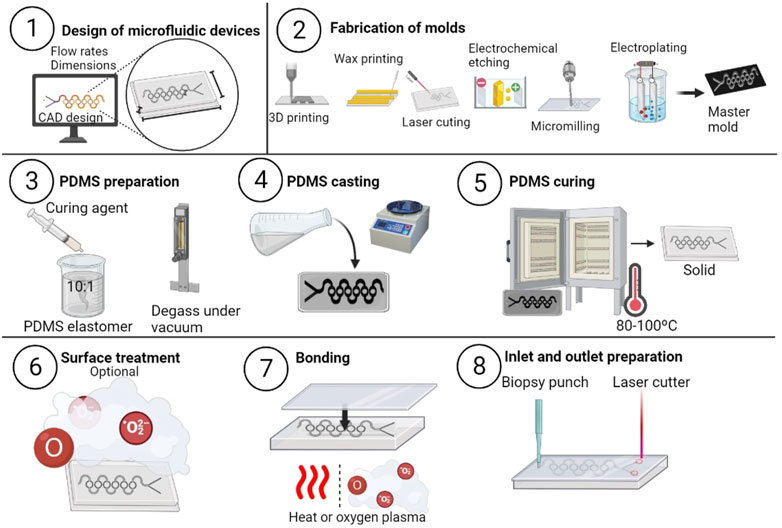
FIGURE 1. Soft lithography method to produce microfluidic devices. The procedure consists of the steps listed below: 1) Using CAD software, design the microfluidic device in consideration of the device’s specifications. 2) Create the master mold using a variety of methods, such as 3D printing, wax printing, laser cutting, micro milling, or electrochemical etching. 3) Create the PDMS solution by combining the PDM and curing agent with the PDMS. 4) Using a spin coater or by hand, evenly coat the mold with the PDMS mixture. 5) Cure the PDMS layer in an oven at temperatures between 80°C and 100°C for a few hours. The PDMS should then be removed from the mold and sized and shaped as desired. 6) Treat the surface of the PDMS device. Attach the PDMS device to the substrate’s lid using oxygen plasma or a thermal bonding technique. Utilize a biopsy punch or a laser cutter to create the required inlets and outlets in the PDMS.
After producing the master, a mixture of PDMS elastomer and curing agent is prepared and poured into the mold (Kamei et al., 2015; Mukherjee et al., 2019). The mixture is spread evenly using a spin coater or by hand and then baked between 75°C and 150°C for several hours until it solidifies (Jang et al., 2016; B. Parker et al., 2016). The cured PDMS is then removed from the mold and cut to the desired dimensions and shape. To ensure excellent adhesion and wetting of the PMDS, the receiving substrate is treated with a surface modifier, such as oxygen plasma treatment, UV/ozone treatment, chemical modification, or physical abrasion (Long et al., 2017; Borók et al., 2021; Mondal et al., 2021; Darboui et al., 2022).
Finally, inlets and outlets are cut into the PDMS using a biopsy punch or laser cutter (Mamidanna et al., 2017; Lee et al., 2018). Soft lithography is a promising and cost-effective method for fabricating microfluidic devices, making it a valuable tool for researchers and small-scale manufacturing facilities (Heo et al., 2020; Šakalys et al., 2021; Nguyen et al., 2022).
3.2 Laser ablation
Due to their precise material removal capabilities, lasers have gained significant interest in recent years for producing microfluidic devices (Ghosh et al., 2019; Mansour et al., 2022; Wei et al., 2023). Laser energy can be focused on the substrate to initiate thermal degradation by optically amplifying the light (Ravi-Kumar et al., 2019; Ho et al., 2022). The laser pulses break polymer bonds, causing an increase in temperature and the expulsion of the material (Vargas et al., 2019; Ouyang et al., 2022). The wavelength of the laser is crucial for determining the quality of the resulting microfluidic devices (Roth et al., 2018; Scott & Ali, 2021) and can be categorized into two types: long or ultra-short wavelengths (Wang et al., 2017; Niculescu et al., 2021). Long-wavelength lasers can achieve widths up to 100 μm (Sarma & Joshi, 2020), while ultra-short wavelength lasers can produce channels with widths as small as 10 μm (Meineke et al., 2016; Roth et al., 2019).
As depicted in Figure 2, laser ablation is a multistep process. The microfluidic device is initially designed using CAD software (Isiksacan et al., 2016; Guo et al., 2021). The design is then used to control the movement of the laser, which removes substrate material. The laser can remove material in a variety of patterns, including straight lines, curved lines, and complex shapes (Wlodarczyk et al., 2019; Hu X et al., 2020). The depth of engraving is determined by the laser’s speed and power, both controlled by software (Hubeatir et al., 2018; Mansour et al., 2022). The laser moves back and forth over the substrate to create the desired microfluidic channels by removing material in a controlled manner (Yao & Fan, 2021; Katla et al., 2023).
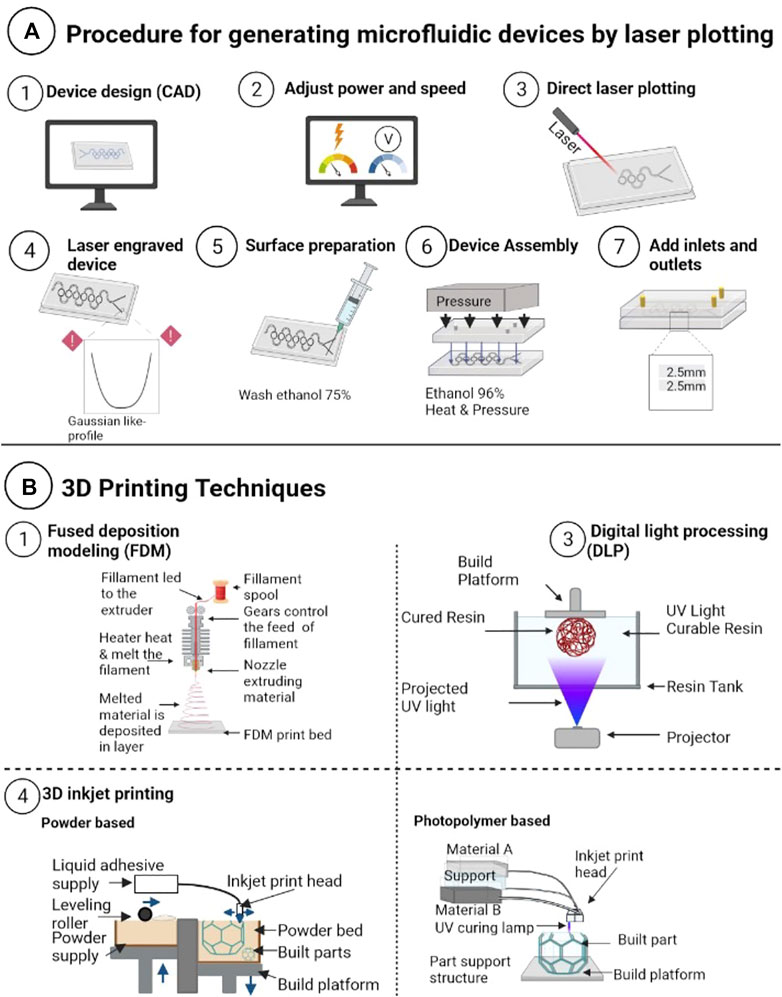
FIGURE 2. (A): 3D printing techniques adapted from Prabhakar et al. (2021), and Padash et al. (2020), including: 1) Fused Deposition Modeling (FDM), which involves heating and extruding material through a nozzle to create layers of the microfluidic device. 2) Stereolithography (SLA) uses a UV laser to solidify resin and generate the device. 3)Digital Light Processing (DLP), which selectively shines light onto the resin using a digital projector. (B): Laser engraving process for creating a microfluidic device, which includes the following steps: 1) CAD software is used to design the device. 2) Adjust the laser’s speed and power to achieve the desired microchannel depth. 3) Using laser engraving, which has the disadvantage of producing a Gaussian profile. 4) To remove residues, wash the surface with 75% ethanol. 5) Using 96% ethanol and heat, join the device’s lid to the bottom layer. 6) Gluing the device’s inputs and outputs.
Laser ablation offers several advantages, including its low-cost and rapid manufacturing process, which can be applied to various materials, including polymers, glass, metals, and ceramics (Malecha et al., 2019; Remiszewska et al., 2019; Wlodarczyk et al., 2019). Polymers such as PMMA are frequently utilized due to their thermal stability and versatility. However, as confirmed by microscopy techniques, this method has the disadvantage of causing a Gaussian degradation, resulting in diagonal walls with a grainy texture in the channel walls (Figure 2) (Matellan & del Río Hernández, 2018; Gao K et al., 2019; Guo et al., 2022).
3.3 3D printing
In recent years, 3D printing has gained popularity as a method for fabricating microfluidic devices due to its capacity to create precise, intricate structures (Markoski et al., 2021; Shan et al., 2021; Wang A et al., 2021; Wang H et al., 2021). This method offers a wide range of material options, including metals, ceramics, and polymers such as PDMS, polycarbonate (PC), PMMA, and acrylonitrile butadiene styrene (ABS) (Litti et al., 2021; Abdalkader et al., 2022; Fei et al., 2022; Li et al., 2023).
Numerous 3D printing techniques exist, such as Selective Laser Sintering (SLS), Electron Beam Melting (EBM), stereolithography (SLA), and Multi Jet Fusion (MJF) (Roy et al., 2019; Berger et al., 2021; Hwang et al., 2022; Uçak et al., 2022). However, due to the high equipment and material costs, these methods may not be suitable for smaller-scale production and research facilities with limited resources (Mehta & Rath, 2021; Griffin & Pappas, 2023). As a result, alternative low-cost techniques have been developed to enable the 3D printing of microfluidic devices, such as Fused Deposition Modeling (FDM), Digital Light Processing (DLP), and Inkjet 3D printing (i3Dp), (Kanitthamniyom et al., 2021; Vasilescu et al., 2021; Duarte et al., 2022). These techniques provide the advantages of 3D printing, such as design flexibility and production speed, without the high costs of more advanced methods (Au et al., 2016; Prabhakar et al., 2021).
Inkjet 3D printing (i3Dp) is an exceptionally precise additive manufacturing technique that utilizes a printhead to deposit photocrosslinkable resin onto a build platform in a layer-by-layer process, resulting in a continuous pattern (Mehta & Rath, 2021; Gonzalez et al., 2022). This process is versatile, efficient, and exceptionally precise, with a resolution of around 10 μm (Zhou et al., 2020; Mehta & Rath, 2021; Gonzalez et al., 2022; Su et al., 2023). It is important to note that i3DP has two main types: powder-based and photopolymer-based. In powder-based i3Dp, a polymeric sticking solution is used to agglomerate powder particles, while the photopolymer-based i3Dp deposits small droplets of both the build and support materials in a layer-by-layer process to fabricate an object (Padash et al., 2020; Wang & Chen, 2020; Prabhakar et al., 2021).
FDM is the most popular low-cost technique for manufacturing microfluidic devices due to its affordability (Ballacchino et al., 2021; Mader et al., 2021; Mehta et al., 2021). This method has a resolution around 100 μm (Zhou et al., 2020). It creates microfluidic devices by heating and extruding thermoplastic polymeric materials through a nozzle and depositing successive layers onto a cooled surface in an X-Y plane (Lynh & Pin-Chuan, 2018; Zhang et al., 2020). Numerous researchers favor it due to its relative simplicity, user-friendliness, and quicker production time (Gaal et al., 2017; Bauer & Kulinsky, 2018; Quero et al., 2022).
DLP is a 3D printing method that uses photopolymerization to create microfluidic devices with a resolution of around 5 μm (Amoyav et al., 2020; van der Linden et al., 2020; Zhou et al., 2020; Bucciarelli et al., 2022). It employs a digital projector to selectively shine light on the resin, allowing for faster printing because an entire layer of resin can be exposed at once (Catterton et al., 2021; Tabriz et al., 2022). Due to the high pixel density of the digital projector, this method typically has a higher resolution; however, some other 3D printing technologies produce smoother and more accurate surfaces due to the precision of the laser beam (Rey-Joly Maura et al., 2021; Musgrove et al., 2022; Chen et al., 2023).
DLP printers are distinguished by their remarkable efficiency and cost-effectiveness when compared to similar methods. Notably, they are considerably more affordable than Stereolithography (SLA) techniques, which often require expensive equipment and materials (Tully & Meloni, 2020; Yoo et al., 2021). However, the use of a single resin in the production of microfluidic devices presents a significant challenge in achieving varying material properties across different components of the device. Grayscale digital light processing (g-DLP) has emerged as a promising technique to overcome this limitation. A prime example of this has been demonstrated by Yue et al. (2023), who have developed a photocurable resin using g-DLP that offers high stretchability and a broad range of modulus values. By utilizing three different monomers and incorporating aliphatic urethane diacrylate (AUD) as a crosslinker, the resin can form hydrogen bonds with both isobornyl acrylate (IBOA) and 2-hydroxyethyl acrylate (2-HEA) to achieve moduli values ranging from 0.016 to 478 MPa, with a stretchability of up to 1,500% in its soft state (Yue et al., 2023).
The choice of 3D printing technique to produce microfluidic devices is contingent on the application and available resources. Factors such as cost, resolution, and surface accuracy must be considered when selecting the most appropriate technique (Waheed et al., 2016; Mehta & Rath, 2021; Gonzalez et al., 2022).
4 Discussion
Labs-on-a-chip (LOCs) devices have been made possible by microfluidic technology, which enables the miniaturization and optimization of processes. The industry has been focusing on their development (Mistretta et al., 2022; Najjar et al., 2022). However, traditional techniques for producing LOCs are expensive, limiting their availability and creating a need for more efficient and cost-effective alternatives (Ren et al., 2013; Elvira et al., 2022). Consequently, several low-cost microfluidic device manufacturing techniques have emerged to replace traditional manufacturing techniques requiring clean room facilities (Leung et al., 2022; Mistretta et al., 2022).
This article compares soft lithography, laser plotting, and 3D printing, three low-cost techniques for manufacturing microfluidic devices. Soft lithography is an affordable technique that offers high resolution and versatile geometries (Leung et al., 2022; Mesquita et al., 2022). Laser plotting is a rapid method (Scott & Ali, 2021; Šakalys et al., 2021), while 3D printing provides design flexibility and fast production (Niculescu et al., 2021; Griffin & Pappas, 2023).
In addition, the review examines the suitability of diverse materials, including paper, polymers, and hydrogels, for producing low-cost LOCs. Paper microfluidics devices are inexpensive, user-friendly, and portable but have limitations in mechanical strength and reusability (Cate et al., 2015; Prabhakar et al., 2021). Because of their high compatibility with biological samples and ease of fabrication, PDMS and PMMA are frequently employed in microfluidic device production. However, they have drawbacks, such as PDMS’s tendency to swell when exposed to certain organic solvents and PMMA’s low mechanical strength. Hydrogels have modifiable mechanical properties and can mimic the extracellular matrix (ECM), but their mechanical strength and long-term stability are limited (Hsu et al., 2018; Qin et al., 2021).
The material chosen for the device is determined by its specific requirements and intended application, as each material has its own advantages and disadvantages. The development of low-cost manufacturing techniques for LOCs will increase their availability and accelerate their incorporation into various applications. This review offers an overview of the potential routes to enhance LOC accessibility for a wider range of researchers and support the ongoing growth of microfluidics.
Author contributions
CR, VA-P, MV, and AM-O wrote the original draft. JO, LR, and JC reviewed and edited the manuscript, and overall supervised the project’s execution. All authors approved the submitted manuscript.
Acknowledgments
The authors would like to thank the Department of Biomedical Engineering and the Department of Chemical and Food Engineering of the Universidad de Los Andes for their support in the development of this review. The authors also would like to thank the Vice Presidency of Research & Creation’s Publication Fund at Universidad de los Andes for its financial support.
Conflict of interest
The authors declare that the research was conducted in the absence of any commercial or financial relationships that could be construed as a potential conflict of interest.
Publisher’s note
All claims expressed in this article are solely those of the authors and do not necessarily represent those of their affiliated organizations, or those of the publisher, the editors and the reviewers. Any product that may be evaluated in this article, or claim that may be made by its manufacturer, is not guaranteed or endorsed by the publisher.
References
Abdalkader, R., Konishi, S., and Fujita, T. (2022). The development of biomimetic aligned skeletal muscles in a fully 3d printed microfluidic device. Biomimetics 7 (1), 2. doi:10.3390/biomimetics7010002
Agha, A., Waheed, W., Alamoodi, N., Mathew, B., Alnaimat, F., Abu-Nada, E., et al. (2022). A review of cyclic olefin copolymer applications in microfluidics and microdevices. Macromol. Mater. Eng. 307 (8), 2200053. doi:10.1002/mame.202200053
Akhtar, A. S., Soares, R. R. G., Pinto, I. F., and Russom, A. (2023). A portable and low-cost centrifugal microfluidic platform for multiplexed colorimetric detection of protein biomarkers. Anal. Chim. Acta 1245, 340823. doi:10.1016/J.ACA.2023.340823
Akther, F., Yakob, S. B., Nguyen, N.-T., and Ta, H. T. (2020). Surface modification techniques for endothelial cell seeding in PDMS microfluidic devices. Biosensors 10 (11), 182. doi:10.3390/bios10110182
Ameri, A. R., Imanparast, A., Passandideh-Fard, M., and Mousavi Shaegh, S. A. (2022). A whole-thermoplastic microfluidic chip with integrated on-chip micropump, bioreactor and oxygenator for cell culture applications. Anal. Chim. Acta 1221, 340093. doi:10.1016/j.aca.2022.340093
Amirabad, L. M., Tahriri, M., Zarrintaj, P., Ghaffari, R., and Tayebi, L. (2022). Preparation and characterization of TiO 2 -coated polymerization of methyl methacrylate (PMMA) for biomedical applications: In vitro study. Asia-Pacific J. Chem. Eng. 17 (3), e2761. doi:10.1002/apj.2761
Amoyav, B., Goldstein, Y., Steinberg, E., and Benny, O. (2020). 3D printed microfluidic devices for drug release assays. Pharmaceutics 13 (1), 13. doi:10.3390/PHARMACEUTICS13010013
Ardakani, F., Shojaeifard, Z., and Hemmateenejad, B. (2022). Point-of-need determination of blood typing using a three-dimensional origami microfluidic paper based analytical device. Microchem. J. 181, 107796. doi:10.1016/j.microc.2022.107796
Au, A. K., Huynh, W., Horowitz, L. F., and Folch, A. (2016). 3D-Printed microfluidics. Angew. Chem. Int. Ed. 55 (12), 3862–3881. doi:10.1002/anie.201504382
Babaei, M., Bonakdar, S., and Nasernejad, B. (2022). Selective biofunctionalization of 3D cell-imprinted PDMS with collagen immobilization for targeted cell attachment. Sci. Rep. 12 (1), 12837. doi:10.1038/s41598-022-17252-6
Ballacchino, G., Weaver, E., Mathew, E., Dorati, R., Genta, I., Conti, B., et al. (2021). Manufacturing of 3D-printed microfluidic devices for the synthesis of drug-loaded liposomal formulations. Int. J. Mol. Sci. 22 (15), 8064. doi:10.3390/ijms22158064
Bashir, S., Hina, M., Iqbal, J., Rajpar, A. H., Mujtaba, M. A., Alghamdi, N. A., et al. (2020). Fundamental concepts of hydrogels: Synthesis, properties, and their applications. Polymers 12 (11), 2702. doi:10.3390/POLYM12112702
Bauer, M., and Kulinsky, L. (2018). Fabrication of a lab-on-chip device using material extrusion (3D printing) and demonstration via malaria-ab ELISA. Micromachines 9 (1), 27. doi:10.3390/mi9010027
Bavendiek, J., Maurer, P., Gräber, S., Pasch, A., Schomburg, W. K., and Jahnen-Dechent, W. (2020). Rapid calcification propensity testing in blood using a temperature controlled microfluidic polymer chip. PLOS ONE 15 (4), e0230493. doi:10.1371/journal.pone.0230493
Becker, H., and Gärtner, C. (2000). Polymer microfabrication methods for microfluidic analytical applications. Electrophoresis 21 (1), 12–26. doi:10.1002/(SICI)1522-2683(20000101)21:1<12::AID-ELPS12>3.0.CO;2-7
Bento, S. A., Gaspar, M. C., Coimbra, P., de Sousa, H. C., and Braga, E. M. M. (2023). A review of conventional and emerging technologies for hydrogels sterilization. Int. J. Pharm. 634, 122671. doi:10.1016/J.IJPHARM.2023.122671
Berger, J., Aydin, M. Y., Stavins, R., Heredia, J., Mostafa, A., Ganguli, A., et al. (2021). Portable pathogen diagnostics using microfluidic cartridges made from continuous liquid interface production additive manufacturing. Anal. Chem. 93 (29), 10048–10055. doi:10.1021/acs.analchem.1c00654
Bhatia, A., and Sehgal, A. K. (2021). Additive manufacturing materials, methods and applications: A review. Mater. Today Proc. 2021, 379. doi:10.1016/j.matpr.2021.04.379
Bhavsar, S., Patel, G. B., and Singh, N. L. (2018). Investigation of optical properties of aluminium oxide doped polystyrene polymer nanocomposite films. Phys. B Condens. Matter 533, 12–16. doi:10.1016/j.physb.2017.12.055
Boonkaew, S., Jang, I., Noviana, E., Siangproh, W., Chailapakul, O., and Henry, C. S. (2021). Electrochemical paper-based analytical device for multiplexed, point-of-care detection of cardiovascular disease biomarkers. Sensors Actuators B Chem. 330, 129336. doi:10.1016/j.snb.2020.129336
Borók, A., Laboda, K., and Bonyár, A. (2021). PDMS bonding technologies for microfluidic applications: A review. Biosensors 11 (8), 292. doi:10.3390/BIOS11080292
Bressan, L. P., Adamo, C. B., Quero, R. F., de Jesus, D. P., and da Silva, J. A. F. (2019). A simple procedure to produce FDM-based 3D-printed microfluidic devices with an integrated PMMA optical window. Anal. Methods 11 (8), 1014–1020. doi:10.1039/C8AY02092B
Bruijns, B., Tiggelaar, R., and Gardeniers, H. (2020). A microfluidic approach for biosensing DNA within forensics. Appl. Sci. 10 (20), 7067. doi:10.3390/app10207067
Bruijns, B., Veciana, A., Tiggelaar, R., and Gardeniers, H. (2019). Cyclic olefin copolymer microfluidic devices for forensic applications. Biosensors 9 (3), 85. doi:10.3390/bios9030085
Bucciarelli, A., Paolelli, X., de Vitis, E., Selicato, N., Gervaso, F., Gigli, G., et al. (2022). VAT photopolymerization 3D printing optimization of high aspect ratio structures for additive manufacturing of chips towards biomedical applications. Addit. Manuf. 60, 103200. doi:10.1016/J.ADDMA.2022.103200
Camman, M., Joanne, P., Brun, J., Marcellan, A., Dumont, J., Agbulut, O., et al. (2023). Anisotropic dense collagen hydrogels with two ranges of porosity to mimic the skeletal muscle extracellular matrix. Biomater. Adv. 144, 213219. doi:10.1016/J.BIOADV.2022.213219
Campbell, S. B., Wu, Q., Yazbeck, J., Liu, C., Okhovatian, S., and Radisic, M. (2021). Beyond polydimethylsiloxane: Alternative materials for fabrication of organ-on-a-chip devices and microphysiological systems. ACS Biomaterials Sci. Eng. 7 (7), 2880–2899. doi:10.1021/acsbiomaterials.0c00640
Cao, U. M. N., Zhang, Y., Chen, J., Sayson, D., Pillai, S., and Tran, S. D. (2023). Microfluidic organ-on-A-chip: A guide to biomaterial choice and fabrication. Int. J. Mol. Sci. 24 (4), 3232. doi:10.3390/IJMS24043232
Carrell, C., Kava, A., Nguyen, M., Menger, R., Munshi, Z., Call, Z., et al. (2019). Beyond the lateral flow assay: A review of paper-based microfluidics. Microelectron. Eng. 206, 45–54. doi:10.1016/J.MEE.2018.12.002
Cate, D. M., Adkins, J. A., Mettakoonpitak, J., and Henry, C. S. (2015). Recent developments in paper-based microfluidic devices. Anal. Chem. 87 (1), 19–41. doi:10.1021/AC503968P
Catterton, M. A., Ball, A. G., and Pompano, R. R. (2021). Rapid fabrication by digital light processing 3d printing of a slipchip with movable ports for local delivery to ex vivo organ cultures. Micromachines 12 (8), 993. doi:10.3390/mi12080993
Chen, Q., Nguyen, T., Duong Bang, D., Sommonte, F., Denora, N., and Lamprou, D. A. (2023). Combining 3D printing and microfluidic techniques: A powerful synergy for nanomedicine. Pharmaceuticals 16 (1), 69. doi:10.3390/PH16010069
Chen, Y. Y., Kingston, B. R., and Chan, W. C. W. (2020). Transcribing in vivo blood vessel networks into in vitro perfusable microfluidic devices. Adv. Mater. Technol. 5 (6), 2000103. doi:10.1002/admt.202000103
Ching, T., Nie, X., Chang, S.-Y., Toh, Y.-C., and Hashimoto, M. (2023). Techniques and materials for the fabrication of microfluidic devices. Princ. Hum. Organs-on-Chips 2023, 1–36. doi:10.1016/B978-0-12-823536-2.00014-6
Cincotto, F. H., Fava, E. L., Moraes, F. C., Fatibello-Filho, O., and Faria, R. C. (2019). A new disposable microfluidic electrochemical paper-based device for the simultaneous determination of clinical biomarkers. Talanta 195, 62–68. doi:10.1016/J.TALANTA.2018.11.022
Clancy, A., Chen, D., Bruns, J., Nadella, J., Stealey, S., Zhang, Y., et al. (2022). Hydrogel-based microfluidic device with multiplexed 3D in vitro cell culture. Sci. Rep. 12 (1), 17781–17813. doi:10.1038/s41598-022-22439-y
Crevillen, A. G., Mayorga-Martinez, C. C., Vaghasiya, J. v., and Pumera, M. (2022). 3D-Printed SARS-CoV-2 RNA genosensing microfluidic system. Adv. Mater. Technol. 7 (6), 2101121. doi:10.1002/admt.202101121
da Ponte, R. M., Gaio, N., van Zeijl, H., Vollebregt, S., Dijkstra, P., Dekker, R., et al. (2021). Monolithic integration of a smart temperature sensor on a modular silicon-based organ-on-a-chip device. Sensors Actuators A Phys. 317, 112439. doi:10.1016/J.SNA.2020.112439
Dabaghi, M., Saraei, N., Fusch, G., Rochow, N., Brash, J. L., Fusch, C., et al. (2019). An ultra-thin, all PDMS-based microfluidic lung assist device with high oxygenation capacity. Biomicrofluidics 13 (3), 034116. doi:10.1063/1.5091492
Dabaghi, M., Shahriari, S., Saraei, N., Da, K., Chandiramohan, A., Selvaganapathy, P. R., et al. (2021). Surface modification of PDMS-based microfluidic devices with collagen using polydopamine as a spacer to enhance primary human bronchial epithelial cell adhesion. Micromachines 12 (2), 132. doi:10.3390/mi12020132
Darboui, M., Askari Moghadam, R., and Parichehr, R. (2022). Microfluidics chip inspired by fish gills for blood cells and serum separation. Sensors Actuators A Phys. 346, 113839. doi:10.1016/J.SNA.2022.113839
de Almeida Monteiro Melo Ferraz, M., Nagashima, J. B., Venzac, B., le Gac, S., and Songsasen, N. (2020). 3D printed mold leachates in PDMS microfluidic devices. Sci. Rep. 10 (1), 1–9. doi:10.1038/s41598-020-57816-y
de Oliveira, K. G., Estrela, P. F. N., Mendes, G. de M., dos Santos, C. A., Silveira-Lacerda, E. de P., and Duarte, G. R. M. (2021). Rapid molecular diagnostics of COVID-19 by RT-LAMP in a centrifugal polystyrene-toner based microdevice with end-point visual detection. Analyst 146 (4), 1178–1187. doi:10.1039/D0AN02066D
Denecke, K. M., McBain, C. A., Hermes, B. G., Teertam, S. K., Farooqui, M., Virumbrales-Muñoz, M., et al. (2022). Microfluidic model to evaluate astrocyte activation in penumbral region following ischemic stroke. Cells 11 (15), 2356. doi:10.3390/cells11152356
Duarte, L. C., Pereira, I., Maciel, L. I. L., Vaz, B. G., and Coltro, W. K. T. (2022). 3D printed microfluidic mixer for real-time monitoring of organic reactions by direct infusion mass spectrometry. Anal. Chim. Acta 1190, 339252. doi:10.1016/j.aca.2021.339252
Duc, P., Vignes, M., Hugon, G., Sebban, A., Carnac, G., Malyshev, E., et al. (2021). Human neuromuscular junction on micro-structured microfluidic devices implemented with a custom micro electrode array (MEA). Lab a Chip 21 (21), 4223–4236. doi:10.1039/D1LC00497B
Elvira, K. S., Gielen, F., Tsai, S. S. H., and Nightingale, A. M. (2022). Materials and methods for droplet microfluidic device fabrication. Lab a Chip 22 (5), 859–875. doi:10.1039/D1LC00836F
Etxeberria, L., Messelmani, T., Badiola, J. H., Llobera, A., Fernandez, L., Vilas-Vilela, J. L., et al. (2022). Validation of HepG2/C3A cell cultures in cyclic olefin copolymer based microfluidic bioreactors. Polymers 14 (21), 4478. doi:10.3390/polym14214478
Fan, W., Chen, X., Ge, Y., Jin, Y., Jin, Q., and Zhao, J. (2019). Single-cell impedance analysis of osteogenic differentiation by droplet-based microfluidics. Biosens. Bioelectron. 145, 111730. doi:10.1016/j.bios.2019.111730
Fei, G., Nie, L., Zhong, L., Shi, Q., Hu, K., Parra-Cabrera, C., et al. (2022). Photocurable resin-silica composites with low thermal expansion for 3D printing microfluidic components onto printed circuit boards. Mater. Today Commun. 31, 103482. doi:10.1016/J.MTCOMM.2022.103482
Fiore, L., Mazzaracchio, V., Serani, A., Fabiani, G., Fabiani, L., Volpe, G., et al. (2023). Microfluidic paper-based wearable electrochemical biosensor for reliable cortisol detection in sweat. Sensors Actuators B Chem. 379, 133258. doi:10.1016/j.snb.2022.133258
Gaal, G., Mendes, M., de Almeida, T. P., Piazzetta, M. H. O., Gobbi, Â. L., Riul, A., et al. (2017). Simplified fabrication of integrated microfluidic devices using fused deposition modeling 3D printing. Sensors Actuators B Chem. 242, 35–40. doi:10.1016/j.snb.2016.10.110
Gale, B., Jafek, A., Lambert, C., Goenner, B., Moghimifam, H., Nze, U., et al. (2018). A review of current methods in microfluidic device fabrication and future commercialization prospects. Inventions 3 (3), 60. doi:10.3390/inventions3030060
Gang, M. G., Chae, K. W., Kim, W.-B., Jung, Y. H., Jun, M. B. G., and Min, B.-K. (2019). Wettability modification of cyclic olefin copolymer surface and microchannel using micromilling process. J. Manuf. Process. 37, 168–176. doi:10.1016/j.jmapro.2018.10.037
Gao, B., Li, X., Yang, Y., Chu, J., and He, B. (2019). Emerging paper microfluidic devices. Analyst 144 (22), 6497–6511. doi:10.1039/C9AN01275C
Gao, K., Liu, J., Fan, Y., and Zhang, Y. (2019). Ultra-low-cost fabrication of polymer-based microfluidic devices with diode laser ablation. Biomed. Microdevices 21 (4), 83. doi:10.1007/s10544-019-0433-6
García-Torres, J., Colombi, S., Macor, L. P., and Alemán, C. (2022). Multitasking smart hydrogels based on the combination of alginate and poly(3,4-ethylenedioxythiophene) properties: A review. Int. J. Biol. Macromol. 219, 312–332. doi:10.1016/J.IJBIOMAC.2022.08.008
Ghosh, R., Gopalakrishnan, S., Savitha, R., Renganathan, T., and Pushpavanam, S. (2019). Fabrication of laser printed microfluidic paper-based analytical devices (LP-µPADs) for point-of-care applications. Sci. Rep. 9 (1), 7896–7911. doi:10.1038/s41598-019-44455-1
Gizer, S. G., Bhethanabotla, V. R., Ayyala, R. S., and Sahiner, N. (2023). Low-pressure plasma treated polycarbonate and polymethyl methacrylate (PMMA) sheets with different surface patterns to change their surface properties. Surfaces Interfaces 37, 102646. doi:10.1016/j.surfin.2023.102646
Gonzalez, G., Roppolo, I., Pirri, C. F., and Chiappone, A. (2022). Current and emerging trends in polymeric 3D printed microfluidic devices. Addit. Manuf. 55, 102867. doi:10.1016/J.ADDMA.2022.102867
Grant, J., Özkan, A., Oh, C., Mahajan, G., Prantil-Baun, R., and Ingber, D. E. (2021). Simulating drug concentrations in PDMS microfluidic organ chips. Lab a Chip 21 (18), 3509–3519. doi:10.1039/D1LC00348H
Grebenyuk, S., Abdel Fattah, A. R., Kumar, M., Toprakhisar, B., Rustandi, G., Vananroye, A., et al. (2023). Large-scale perfused tissues via synthetic 3D soft microfluidics. Nat. Commun. 14 (1), 193. doi:10.1038/s41467-022-35619-1
Griffin, K., and Pappas, D. (2023). 3D printed microfluidics for bioanalysis: A review of recent advancements and applications. TrAC Trends Anal. Chem. 158, 116892. doi:10.1016/j.trac.2022.116892
Guan, T., Yuket, S., Cong, H., Carton, D. W., and Zhang, N. (2022). Permanent hydrophobic surface treatment combined with solvent vapor-assisted thermal bonding for mass production of cyclic olefin copolymer microfluidic chips. ACS Omega 7 (23), 20104–20117. doi:10.1021/acsomega.2c01948
Guo, W., Chen, Z., Feng, Z., Li, H., Zhang, M., Zhang, H., et al. (2022). Fabrication of concave microwells and their applications in micro-tissue engineering: A review. Micromachines 13 (9), 1555. doi:10.3390/mi13091555
Guo, W., Tang, L., Zhou, B., and Fung, Y. (2021). Fundamental studies of rapidly fabricated on-chip passive micromixer for modular microfluidics. Micromachines 12 (2), 153. doi:10.3390/mi12020153
Guzzi, F., Candeloro, P., Coluccio, M. L., Cristiani, C. M., Parrotta, E. I., Scaramuzzino, L., et al. (2020). A disposable passive microfluidic device for cell culturing. Biosensors 10 (3), 18. doi:10.3390/bios10030018
Hamilton, A., Perris, J., Convery, N., Mulvihill, D. M., and Gadegaard, N. (2021). Flexible inserts for injection molding of complex micro-structured polymer components. Macromol. Mater. Eng. 306 (9), 2100223. doi:10.1002/MAME.202100223
Hansson, J., Yasuga, H., Haraldsson, T., and Van Der Wijngaart, W. (2016). Synthetic microfluidic paper: High surface area and high porosity polymer micropillar arrays. Lab a Chip 16 (2), 298–304. doi:10.1039/C5LC01318F
He, Y., Yu, Y., Yang, Y., Gu, Y., Mao, T., Shen, Y., et al. (2022). Design and aligner-assisted fast fabrication of a microfluidic platform for quasi-3D cell studies on an elastic polymer. Bioact. Mater. 15, 288–304. doi:10.1016/j.bioactmat.2021.12.010
Heo, B., Fiola, M., Yang, J. H., and Koh, A. (2020). A low-cost, composite collagen-PDMS material for extended fluid retention in the skin-interfaced microfluidic devices. Colloid Interface Sci. Commun. 38, 100301. doi:10.1016/j.colcom.2020.100301
Ho, T. C., Chang, C. C., Chan, H. P., Chung, T. W., Shu, C. W., Chuang, K. P., et al. (2022). Hydrogels: Properties and applications in biomedicine. Molecules 27 (9), 2902. doi:10.3390/MOLECULES27092902
Horowitz, L. F., Rodriguez, A. D., Dereli-Korkut, Z., Lin, R., Castro, K., Mikheev, A. M., et al. (2020). Multiplexed drug testing of tumor slices using a microfluidic platform. Npj Precis. Oncol. 4 (1), 12. doi:10.1038/s41698-020-0117-y
Hou, X., Zhang, Y. S., Santiago, G. T. De, Alvarez, M. M., Ribas, J., Jonas, S. J., et al. (2017). Interplay between materials and microfluidics. Nat. Rev. Mater. 2 (5), 17016–17115. doi:10.1038/natrevmats.2017.16
Hsu, M. N., Wei, S. C., Guo, S., Phan, D. T., Zhang, Y., and Chen, C. H. (2018). Smart hydrogel microfluidics for single-cell multiplexed secretomic analysis with high sensitivity. Small 14 (49), 1802918. doi:10.1002/SMLL.201802918
Hu, H., Li, S., Ying, C., Zhang, R., Li, Y., Qian, W., et al. (2020). Hydrophilic PDMS with a sandwich-like structure and no loss of mechanical properties and optical transparency. Appl. Surf. Sci. 503, 144126. doi:10.1016/j.apsusc.2019.144126
Hu, X., Yang, F., Guo, M., Pei, J., Zhao, H., and Wang, Y. (2020). Fabrication of polyimide microfluidic devices by laser ablation based additive manufacturing. Microsyst. Technol. 26 (5), 1573–1583. doi:10.1007/s00542-019-04698-4
Hubeatir, K., Al-Kafaji, M. M., and Omran, H. J. (2018). A review: Effect of different laser types on material EngravingProcess. J. Mat. Sci. 6 (4), 210–217.
Hwang, J.-Y., Seo, J., and Ji, C.-H. (2022). Electromagnetic omnidirectional scanning micromirror with multi jet fusion printed structures for smart factory applications. Addit. Manuf. 55, 102868. doi:10.1016/j.addma.2022.102868
Isiksacan, Z., Guler, M. T., Aydogdu, B., Bilican, I., and Elbuken, C. (2016). Rapid fabrication of microfluidic PDMS devices from reusable PDMS molds using laser ablation. J. Micromechanics Microengineering 26 (3), 035008. doi:10.1088/0960-1317/26/3/035008
Izadifar, Z., Cotton, J., Chen, C., Bustos, N. A., Horvath, V., Stejskalova, A., et al. (2023). Mucus production, host-microbiome interactions, hormone sensitivity, and innate immune responses modeled in human endo- and Ecto-Cervix Chips. Available at: https://doi.org/10.1101/2023.02.22.529436.
Jang, L. W., Razu, M. E., Jensen, E. C., Jiao, H., and Kim, J. (2016). A fully automated microfluidic micellar electrokinetic chromatography analyzer for organic compound detection. Lab a Chip 16 (18), 3558–3564. doi:10.1039/C6LC00790B
Kamei, K., Mashimo, Y., Koyama, Y., Fockenberg, C., Nakashima, M., Nakajima, M., et al. (2015). 3D printing of soft lithography mold for rapid production of polydimethylsiloxane-based microfluidic devices for cell stimulation with concentration gradients. Biomed. Microdevices 17 (2), 36–38. doi:10.1007/s10544-015-9928-y
Kanitthamniyom, P., Hon, P. Y., Zhou, A., Abdad, M. Y., Leow, Z. Y., Yazid, N. B. M., et al. (2021). A 3D-printed magnetic digital microfluidic diagnostic platform for rapid colorimetric sensing of carbapenemase-producing Enterobacteriaceae. Microsystems Nanoeng. 7 (1), 47. doi:10.1038/s41378-021-00276-9
Karthik, V., Karuna, B., Kumar, P. S., Saravanan, A., and Hemavathy, R. v. (2022). Development of lab-on-chip biosensor for the detection of toxic heavy metals: A review. Chemosphere 299, 134427. doi:10.1016/J.CHEMOSPHERE.2022.134427
Katla, S. K., Zhou, W., Tavakoli, H., Méndez, E. L. P., and Li, X. (2023). Portable in situ temperature-dependent spectroscopy on a low-cost microfluidic platform integrated with a battery-powered thermofoil heater. VIEW 2023, 20220053. doi:10.1002/VIW.20220053
Kato, M., Fukushi, M., Yamada, M., Utoh, R., and Seki, M. (2021). Microengineering of collagen hydrogels integrated into microfluidic devices for perfusion culture of mammalian cells. MATEC Web Conf. 333, 07006. doi:10.1051/MATECCONF/202133307006
Kaya, K., Iseri, E., and van der Wijngaart, W. (2022). Soft metamaterial with programmable ferromagnetism. Microsystems Nanoeng. 8 (1), 127. doi:10.1038/s41378-022-00463-2
Khemthongcharoen, N., Uawithya, P., Yookong, N., Chanasakulniyom, M., Jeamsaksiri, W., Sripumkhai, W., et al. (2021). Microfluidic system evaluation for the semi-automatic detection of MOG-IgG in serum samples. Sens. Bio-Sensing Res. 34, 100458. doi:10.1016/j.sbsr.2021.100458
Khot, M. I., Levenstein, M. A., de Boer, G. N., Armstrong, G., Maisey, T., Svavarsdottir, H. S., et al. (2020). Characterising a PDMS based 3D cell culturing microfluidic platform for screening chemotherapeutic drug cytotoxic activity. Sci. Rep. 10 (1), 15915. doi:10.1038/s41598-020-72952-1
Kulkarni, M. B., and Goel, S. (2022). Recent advancements in integrated microthermofluidic systems for biochemical and biomedical applications – a review. Sensors Actuators A Phys. 341, 113590. doi:10.1016/j.sna.2022.113590
Kulkarni, M. B., Salve, M., and Goel, S. (2021). Miniaturized thermal monitoring module with CO₂ laser ablated microfluidic device for electrochemically validated DNA amplification. IEEE Trans. Instrum. Meas. 70, 1–8. doi:10.1109/TIM.2021.3097861
Kulsharova, G., Dimov, N., Marques, M. P. C., Szita, N., and Baganz, F. (2018). Simplified immobilisation method for histidine-tagged enzymes in poly(methyl methacrylate) microfluidic devices. New Biotechnol. 47, 31–38. doi:10.1016/j.nbt.2017.12.004
Kumawat, N., Soman, S. S., Vijayavenkataraman, S., and Kumar, S. (2022). Rapid and inexpensive process to fabricate paper based microfluidic devices using a cut and heat plastic lamination process. Lab a Chip 22 (18), 3377–3389. doi:10.1039/D2LC00452F
Lee, J.-B., Kim, H., Kim, S., and Sung, G. (2022). Fabrication and evaluation of tubule-on-a-chip with RPTEC/HUVEC Co-culture using injection-molded polycarbonate chips. Micromachines 13 (11), 1932. doi:10.3390/mi13111932
Lee, S., Kim, H., Lee, W., and Kim, J. (2018). Finger-triggered portable PDMS suction cup for equipment-free microfluidic pumping. Micro Nano Syst. Lett. 6 (1), 1. doi:10.1186/s40486-018-0063-4
Leung, C. M., de Haan, P., Ronaldson-Bouchard, K., Kim, G. A., Ko, J., Rho, H. S., et al. (2022). A guide to the organ-on-a-chip. Nat. Rev. Methods Prim. 2 (1), 33–29. doi:10.1038/s43586-022-00118-6
Li, F., Wang, W., Li, H., Yang, M., Wu, J., Zhang, L., et al. (2023). A low-cost portable microfluidic chemiluminometer for point-of-care testing of adiponectin for early risk assessment of diabetes. Sensors Actuators B Chem. 380, 133384. doi:10.1016/J.SNB.2023.133384
Li, Q., Niu, K., Wang, D., Xuanb, L., and Wang, X. (2022). Low-cost rapid prototyping and assembly of an open microfluidic device for a 3D vascularized organ-on-a-chip. Lab. Chip 22, 2682–2694. The Royal Society of Chemistry. doi:10.1039/D1LC00767J
Li, Z., Yeom, B., and Kim, S.-J. (2021). Preprogrammed microfluidic system for parallel anti-reflection coating by layer-by-layer assembly. Lab a Chip 21 (23), 4629–4636. doi:10.1039/D1LC00556A
Lin, D., Li, B., Fu, L., Qi, J., Xia, C., Zhang, Y., et al. (2022). A novel polymer-based nitrocellulose platform for implementing a multiplexed microfluidic paper-based enzyme-linked immunosorbent assay. Microsystems Nanoeng. 8 (1), 53. doi:10.1038/s41378-022-00385-z
Litti, L., Trivini, S., Ferraro, D., and Reguera, J. (2021). 3D printed microfluidic device for magnetic trapping and SERS quantitative evaluation of environmental and biomedical analytes. ACS Appl. Mater. Interfaces 13 (29), 34752–34761. doi:10.1021/acsami.1c09771
Long, H. P., Lai, C. C., and Chung, C. K. (2017). Polyethylene glycol coating for hydrophilicity enhancement of polydimethylsiloxane self-driven microfluidic chip. Surf. Coatings Technol. 320, 315–319. doi:10.1016/J.SURFCOAT.2016.12.059
Lynh, H. D., and Pin-Chuan, C. (2018). Novel solvent bonding method for creation of a three-dimensional, non-planar, hybrid PLA/PMMA microfluidic chip. Sensors Actuators A Phys. 280, 350–358. doi:10.1016/j.sna.2018.08.002
Ma, X., Hao, G., Zhang, Z., Li, J., Yang, X., and Chen, L. (2020). Environmentally friendly ratiometric fluorescent microfluidic paper chip for rapid detection of difenoconazole. Sci. Sin. Chim. 50 (3), 393–405. doi:10.1360/SSC-2019-0140
Ma, Z., Li, B., Peng, J., and Gao, D. (2022). Recent development of drug delivery systems through microfluidics: From synthesis to evaluation. Pharmaceutics 14 (2), 434. doi:10.3390/PHARMACEUTICS14020434
Madadi, M., Madadi, A., Zareifar, R., and Nikfarjam, A. (2023). A simple solvent-assisted method for thermal bonding of large-surface, multilayer pmma microfluidic devices. Sensors Actuators A Phys. 349, 114077. doi:10.1016/j.sna.2022.114077
Mader, M., Rein, C., Konrat, E., Meermeyer, S. L., Lee-Thedieck, C., Kotz-Helmer, F., et al. (2021). Fused deposition modeling of microfluidic chips in transparent polystyrene. Micromachines 12 (11), 1348. doi:10.3390/mi12111348
Mahinroosta, M., Jomeh Farsangi, Z., Allahverdi, A., and Shakoori, Z. (2018). Hydrogels as intelligent materials: A brief review of synthesis, properties and applications. Mater. Today Chem. 8, 42–55. doi:10.1016/J.MTCHEM.2018.02.004
Maldonado, J. R., and Peckerar, M. (2016). X-ray lithography: Some history, current status and future prospects. Microelectron. Eng. 161, 87–93. doi:10.1016/j.mee.2016.03.052
Malecha, K., Jasińska, L., Grytsko, A., Drzozga, K., Słobodzian, P., and Cabaj, J. (2019). Monolithic microwave-microfluidic sensors made with low temperature Co-fired ceramic (LTCC) technology. Sensors 19 (3), 577. doi:10.3390/s19030577
Mamidanna, A., Lefky, C., and Hildreth, O. (2017). Drop-on-demand printed microfluidics device with sensing electrodes using silver and PDMS reactive inks. Microfluid. Nanofluidics 21 (11), 172. doi:10.1007/s10404-017-2010-8
Mansour, H., Soliman, E. A., El-Bab, A. M. F., and Abdel-Mawgood, A. L. (2022). Development of epoxy resin-based microfluidic devices using CO2 laser ablation for DNA amplification point-of-care (POC) applications. Int. J. Adv. Manuf. Technol. 120 (7–8), 4355–4372. doi:10.1007/s00170-022-08992-w
Markoski, A., Wong, I. Y., and Borenstein, J. T. (2021). 3D printed monolithic device for the microfluidic capture, perfusion, and analysis of multicellular spheroids. Front. Med. Technol. 3, 646441. doi:10.3389/FMEDT.2021.646441
Martinez, A. W., Phillips, S., Butte, M., and Whitesides, G. (2007). Patterned paper as a platform for inexpensive, low-volume, portable bioassays. Angew. Chem. Int. Ed. 46 (8), 1318–1320. doi:10.1002/anie.200603817
Matellan, C., and del Río Hernández, A. E. (2018). Cost-effective rapid prototyping and assembly of poly(methyl methacrylate) microfluidic devices. Sci. Rep. 8 (1), 6971. doi:10.1038/s41598-018-25202-4
Matsumoto, H., Okabe, T., and Taniguchi, J. (2020). Microchannel fabrication via ultraviolet-nanoimprint lithography and electron-beam lithography using an ultraviolet-curable positive-tone electron-beam resist. Microelectron. Eng. 226, 111278. doi:10.1016/J.MEE.2020.111278
Matsuura, K., and Takata, K. (2023). Blood cell separation using polypropylene-based microfluidic devices based on deterministic lateral displacement. Micromachines 14 (2), 238. doi:10.3390/mi14020238
Mehta, V., and Rath, S. N. (2021). 3D printed microfluidic devices: A review focused on four fundamental manufacturing approaches and implications on the field of healthcare. Bio-Design Manuf. 4 (2), 311–343. doi:10.1007/S42242-020-00112-5
Mehta, V., Vilikkathala Sudhakaran, S., and Rath, S. N. (2021). Facile route for 3D printing of transparent PETg-based hybrid biomicrofluidic devices promoting cell adhesion. ACS Biomaterials Sci. Eng. 7 (8), 3947–3963. doi:10.1021/acsbiomaterials.1c00633
Meineke, G., Hermans, M., Klos, J., Lenenbach, A., and Noll, R. (2016). A microfluidic opto-caloric switch for sorting of particles by using 3D-hydrodynamic focusing based on SLE fabrication capabilities. Lab a Chip 16 (5), 820–828. doi:10.1039/C5LC01478F
Mesquita, P., Gong, L., and Lin, Y. (2022). Low-cost microfluidics: Towards affordable environmental monitoring and assessment. Front. Lab a Chip Technol. 1. doi:10.3389/frlct.2022.1074009
Miranda, I., Souza, A., Sousa, P., Ribeiro, J., Castanheira, E. M. S., Lima, R., et al. (2021). Properties and applications of PDMS for biomedical engineering: A review. J. Funct. Biomaterials 13 (1), 2. doi:10.3390/jfb13010002
Mistretta, M., Gangneux, N., and Manina, G. (2022). Microfluidic dose–response platform to track the dynamics of drug response in single mycobacterial cells. Sci. Rep. 12 (1), 19578–19618. doi:10.1038/s41598-022-24175-9
Mondal, P., Saundarkar, S., Khantwal, N., Tiwari, P., and Srivastava, A. K. (2021). Fabrication of microfluidic channel of polydimethylsiloxane using X-ray lithography and its surface nanostructuring. J. Micromanufacturing 5 (2), 107–115. doi:10.1177/25165984211015760
Mukherjee, P., Nebuloni, F., Gao, H., Zhou, J., and Papautsky, I. (2019). Rapid prototyping of soft lithography masters for microfluidic devices using dry film photoresist in a non-cleanroom setting. Micromachines 10 (3), 192. doi:10.3390/MI10030192
Musgrove, H. B., Catterton, M. A., and Pompano, R. R. (2022). Applied tutorial for the design and fabrication of biomicrofluidic devices by resin 3D printing. Anal. Chim. Acta 1209, 339842. doi:10.1016/J.ACA.2022.339842
Najjar, D., Rainbow, J., Sharma Timilsina, S., Jolly, P., de Puig, H., Yafia, M., et al. (2022). A lab-on-a-chip for the concurrent electrochemical detection of SARS-CoV-2 RNA and anti-SARS-CoV-2 antibodies in saliva and plasma. Nat. Biomed. Eng. 6 (8), 968–978. doi:10.1038/s41551-022-00919-w
Natsuhara, D., Misawa, S., Saito, R., Shirai, K., Okamoto, S., Nagai, M., et al. (2022). A microfluidic diagnostic device with air plug-in valves for the simultaneous genetic detection of various food allergens. Sci. Rep. 12 (1), 12852–12914. doi:10.1038/s41598-022-16945-2
Nguyen, T., Jung, S. H., Lee, M. S., Park, T.-E., Ahn, S., and Kang, J. H. (2019). Robust chemical bonding of PMMA microfluidic devices to porous PETE membranes for reliable cytotoxicity testing of drugs. Lab a Chip 19 (21), 3706–3713. doi:10.1039/C9LC00338J
Nguyen, T., Sarkar, T., Tran, T., Moinuddin, S. M., Saha, D., and Ahsan, F. (2022). Multilayer soft photolithography fabrication of microfluidic devices using a custom-built wafer-scale PDMS slab aligner and cost-efficient equipment. Micromachines 13 (8), 1357. doi:10.3390/mi13081357
Niculescu, A. G., Chircov, C., Bîrcă, A. C., and Grumezescu, A. M. (2021). Fabrication and applications of microfluidic devices: A review. Int. J. Mol. Sci. 22 (4), 2011. doi:10.3390/IJMS22042011
Nie, J., Fu, J., and He, Y. (2020). Hydrogels: The next generation body materials for microfluidic chips? Small 16 (46), 2003797. doi:10.1002/SMLL.202003797
Nishat, S., Jafry, A. T., Martinez, A. W., and Awan, F. R. (2021). Paper-based microfluidics: Simplified fabrication and assay methods. Sensors Actuators B Chem. 336, 129681. doi:10.1016/J.SNB.2021.129681
Olmos, C. M., Vaca, A., Rosero, G., Peñaherrera, A., Perez, C., de Sá Carneiro, I., et al. (2019). Epoxy resin mold and PDMS microfluidic devices through photopolymer flexographic printing plate. Sensors Actuators B Chem. 288, 742–748. doi:10.1016/j.snb.2019.03.062
Orazi, L., Siciliani, V., Pelaccia, R., Oubellaouch, K., and Reggiani, B. (2022). Ultrafast laser micromanufacturing of microfluidic devices. Procedia CIRP 110, 122–127. doi:10.1016/J.PROCIR.2022.06.023
Ortegón, S., Peñaranda, P. A., Rodríguez, C. F., Noguera, M. J., Florez, S. L., Cruz, J. C., et al. (2022). Magnetic torus microreactor as a novel device for sample treatment via solid-phase microextraction coupled to graphite furnace atomic absorption spectroscopy: A route for arsenic pre-concentration. Molecules 27 (19), 6198. doi:10.3390/molecules27196198
Ouyang, Z., Long, J., Wu, J., Lin, J., Xie, X., Tan, G., et al. (2022). Preparation of high-quality three-dimensional microstructures on polymethyl methacrylate surfaces by femtosecond laser micromachining and thermal-induced micro-leveling. Opt. Laser Technol. 145, 107499. doi:10.1016/J.OPTLASTEC.2021.107499
Padash, M., Enz, C., and Carrara, S. (2020). Microfluidics by additive manufacturing for wearable biosensors: A review. Sensors 20 (15), 4236. doi:10.3390/s20154236
Pandey, C. M., Augustine, S., Kumar, S., Kumar, S., Nara, S., Srivastava, S., et al. (2017). Microfluidics based point-of-care diagnostics. Biotechnol. J. 13 (1), 1700047. doi:10.1002/biot.201700047
Park, D., Lee, J., Lee, Y., Son, K., Choi, J. W., Jeang, W. J., et al. (2021). Aspiration-mediated hydrogel micropatterning using rail-based open microfluidic devices for high-throughput 3D cell culture. Sci. Rep. 11 (1), 1–10. doi:10.1038/s41598-021-99387-6
Parker, B., Samanipour, R., Ahmadi, A., and Kim, K. (2016). Rapid fabrication of circular channel microfluidic flow-focusing devices for hydrogel droplet generation. Micro & Nano Lett. 11 (1), 41–45. doi:10.1049/MNL.2015.0329
Parween, S., Bhatnagar, I., Bhosale, S., Paradkar, S., Michael, I. J., Rao, C. M., et al. (2020). Cross-linked chitosan biofunctionalized paper-based microfluidic device towards long term stabilization of blood typing antibodies. Int. J. Biol. Macromol. 163, 1233–1239. doi:10.1016/j.ijbiomac.2020.07.075
Persson, H., Park, S., Mohan, M., Cheung, K. K., Simmons, C. A., and Young, E. W. K. (2022). Rapid assembly of PMMA microfluidic devices with PETE membranes for studying the endothelium. Sensors Actuators B Chem. 356, 131342. doi:10.1016/j.snb.2021.131342
Phiphattanaphiphop, C., Leksakul, K., Phatthanakun, R., and Khamlor, T. (2020). A novel microfluidic chip-based sperm-sorting device constructed using design of experiment method. Sci. Rep. 10 (1), 17143–17213. doi:10.1038/s41598-020-73841-3
Phiphattanaphiphop, C., Leksakul, K., Wanta, T., Khamlor, T., and Phattanakun, R. (2022). Antibody-conjugated magnetic beads for sperm sexing using a multi-wall carbon nanotube microfluidic device. Micromachines 13 (3), 426. doi:10.3390/MI13030426
Prabhakar, P., Sen, R. K., Dwivedi, N., Khan, R., Solanki, P. R., Srivastava, A. K., et al. (2021). 3D-Printed microfluidics and potential biomedical applications. Front. Nanotechnol. 3, 609355. doi:10.3389/fnano.2021.609355
Preetam, S., Nahak, B. K., Patra, S., Toncu, D. C., Park, S., Syväjärvi, M., et al. (2022). Emergence of microfluidics for next generation biomedical devices. Biosens. Bioelectron. X 10, 100106. doi:10.1016/j.biosx.2022.100106
Qin, S., Ou, G., Wang, B., Li, Z., Hu, R., Li, Y., et al. (2022). Photolithography-free fabrication of photoresist-mold for rapid prototyping of microfluidic PDMS devices. Chin. Chem. Lett. 33 (2), 987–989. doi:10.1016/J.CCLET.2021.07.045
Qin, X., Liu, J., Zhang, Z., Li, J., Yuan, L., Zhang, Z., et al. (2021). Microfluidic paper-based chips in rapid detection: Current status, challenges, and perspectives. TrAC Trends Anal. Chem. 143, 116371. doi:10.1016/J.TRAC.2021.116371
Qin, Y., Kreutz, J. E., Schneider, T., Yen, G. S., Shah, E. S., Wu, L., et al. (2022). A reinforced PDMS mold for hot embossing of cyclic olefin polymer in the fabrication of microfluidic chips. Lab a Chip 22 (23), 4729–4734. doi:10.1039/D2LC00857B
Quero, R. F., Costa, B. M. de C., da Silva, J. A. F., and de Jesus, D. P. (2022). Using multi-material fused deposition modeling (FDM) for one-step 3D printing of microfluidic capillary electrophoresis with integrated electrodes for capacitively coupled contactless conductivity detection. Sensors Actuators B Chem. 365, 131959. doi:10.1016/j.snb.2022.131959
Raj, M. K., and Chakraborty, S. (2020). PDMS microfluidics: A mini review. J. Appl. Polym. Sci. 137 (27), 48958. doi:10.1002/app.48958
Ravi-Kumar, S., Lies, B., Zhang, X., Lyu, H., and Qin, H. (2019). Laser ablation of polymers: A review. Polym. Int. 68 (8), 1391–1401. doi:10.1002/PI.5834
Remiszewska, E., Malecha, K., Kruk, J., Jankowska-Śliwińska, J., Torbicz, W., Samluk, A., et al. (2019). Enzymatic method of urea determination in LTCC microfluidic system based on absorption photometry. Sensors Actuators B Chem. 285, 375–384. doi:10.1016/j.snb.2019.01.032
Ren, K., Zhou, J., and Wu, H. (2013). Materials for microfluidic chip fabrication. Accounts Chem. Res. 46 (11), 2396–2406. doi:10.1021/AR300314S
Rey-Joly Maura, C., Godinho, J., Amorim, M., Pinto, R., Marques, D., and Jardim, L. (2021). Precision and trueness of maxillary crowded models produced by 2 vat photopolymerization 3-dimensional printing techniques. Am. J. Orthod. Dentofac. Orthop. 160 (1), 124–131. doi:10.1016/j.ajodo.2020.06.033
Roth, G.-L., Esen, C., and Hellmann, R. (2018). Control of femtosecond laser generated microfluidic channels inside poly(methyl methacrylate). J. Laser Appl. 30 (3), 032016. doi:10.2351/1.5049352
Roth, G. L., Esen, C., and Hellmann, R. (2019). A new approach to seal polymer microfluidic devices using ultrashort laser pulses. J. Laser Micro Nanoeng. 14 (1), 49–53. doi:10.2961/JLMN.2019.01.0009
Roy, N. K., Behera, D., Dibua, O. G., Foong, C. S., and Cullinan, M. A. (2019). A novel microscale selective laser sintering (μ-SLS) process for the fabrication of microelectronic parts. Microsystems Nanoeng. 5 (1), 64. doi:10.1038/s41378-019-0116-8
Rusling, J. F. (2018). Developing microfluidic sensing devices using 3D printing. ACS Sensors 3 (3), 522–526. doi:10.1021/acssensors.8b00079
Šakalys, R., Kho, K. W., and Keyes, T. E. (2021). A reproducible, low cost microfluidic microcavity array SERS platform prepared by soft lithography from a 2 photon 3D printed template. Sensors Actuators B Chem. 340, 129970. doi:10.1016/j.snb.2021.129970
Santana, H. S., Palma, M. S. A., Lopes, M. G. M., Souza, J., Lima, G. A. S., Taranto, O. P., et al. (2020). Microfluidic devices and 3D printing for synthesis and screening of drugs and tissue engineering. Industrial Eng. Chem. Res. 59 (9), 3794–3810. doi:10.1021/acs.iecr.9b03787
Sarma, U., and Joshi, S. N. (2020). Machining of micro-channels on polycarbonate by using laser-induced plasma assisted ablation (LIPAA). Opt. Laser Technol. 128, 106257. doi:10.1016/J.OPTLASTEC.2020.106257
Schneider, S., Brás, E. J. S., Schneider, O., Schlünder, K., and Loskill, P. (2021a). Facile patterning of thermoplastic elastomers and robust bonding to glass and thermoplastics for microfluidic cell culture and organ-on-chip. Micromachines 12 (5), 575. doi:10.3390/mi12050575
Schneider, S., Gruner, D., Richter, A., and Loskill, P. (2021b). Membrane integration into PDMS-free microfluidic platforms for organ-on-chip and analytical chemistry applications. Lab a Chip 21 (10), 1866–1885. doi:10.1039/D1LC00188D
Scott, S. M., and Ali, Z. (2021). Fabrication methods for microfluidic devices: An overview. Micromachines 12 (3), 319. doi:10.3390/MI12030319
Shakeri, A., Jarad, N. A., Khan, S., and F Didar, T. (2022a). Bio-functionalization of microfluidic platforms made of thermoplastic materials: A review. Anal. Chim. Acta 1209, 339283. doi:10.1016/j.aca.2021.339283
Shakeri, A., Khan, S., and Didar, T. F. (2021). Conventional and emerging strategies for the fabrication and functionalization of PDMS-based microfluidic devices. Lab a Chip 21 (16), 3053–3075. doi:10.1039/D1LC00288K
Shakeri, A., Khan, S., Jarad, N. A., and Didar, T. F. (2022b). The fabrication and bonding of thermoplastic microfluidics: A review. Materials 15 (18), 6478. doi:10.3390/ma15186478
Shan, H., Lin, Q., Wang, D., Sun, X., Quan, B., Chen, X., et al. (2021). 3D printed integrated multi-layer microfluidic chips for ultra-high volumetric throughput nanoliposome preparation. Front. Bioeng. Biotechnol. 9, 773705. doi:10.3389/fbioe.2021.773705
Sharma, S., and Tiwari, S. (2020). A review on biomacromolecular hydrogel classification and its applications. Int. J. Biol. Macromol. 162, 737–747. doi:10.1016/J.IJBIOMAC.2020.06.110
Shubhava, S., Jayarama, A., Kannarpady, G. K., Kale, S., Prabhu, S., and Pinto, R. (2022). Chemical etching of glasses in hydrofluoric acid: A brief review. Mater. Today Proc. 55, 46–51. doi:10.1016/J.MATPR.2021.12.110
Siddique, A., Pause, I., Narayan, S., Kruse, L., and Stark, R. W. (2021). Endothelialization of PDMS-based microfluidic devices under high shear stress conditions. Colloids Surfaces B Biointerfaces 197, 111394. doi:10.1016/j.colsurfb.2020.111394
Sivakumar, R., and Lee, N. Y. (2020). Microfluidic device fabrication mediated by surface chemical bonding. Analyst 145 (12), 4096–4110. doi:10.1039/D0AN00614A
Sommonte, F., Weaver, E., Mathew, E., Denora, N., and Lamprou, D. A. (2022). In-house innovative “diamond shaped” 3D printed microfluidic devices for lysozyme-loaded liposomes. Pharmaceutics 14, 2484. doi:10.3390/PHARMACEUTICS14112484
Sood, A., Kumar, A., Dev, A., Gupta, V. K., and Han, S. S. (2022). Advances in hydrogel-based microfluidic blood–brain-barrier models in oncology research. Pharmaceutics 14 (5), 993. doi:10.3390/PHARMACEUTICS14050993
Su, R., Wang, F., and McAlpine, M. C. (2023). 3D printed microfluidics: Advances in strategies, integration, and applications. Lab a Chip 23 (5), 1279–1299. doi:10.1039/D2LC01177H
Sui, S., Li, L., Shen, J., Ni, G., Xie, H., Lin, Q., et al. (2021). Plasma treatment of polymethyl methacrylate to improve surface hydrophilicity and antifouling performance. Polym. Eng. Sci. 61 (2), 506–513. doi:10.1002/pen.25595
Tabriz, A. G., Viegas, B., Okereke, M., Uddin, M. J., Lopez, E. A., Zand, N., et al. (2022). Evaluation of 3D printability and biocompatibility of microfluidic resin for fabrication of solid microneedles. Micromachines 13 (9), 1368. doi:10.3390/MI13091368
Tang, Q., Li, X., Lai, C., Li, L., Wu, H., Wang, Y., et al. (2021). Fabrication of a hydroxyapatite-PDMS microfluidic chip for bone-related cell culture and drug screening. Bioact. Mater. 6 (1), 169–178. doi:10.1016/j.bioactmat.2020.07.016
Tevlek, A., Kecili, S., Ozcelik, O. S., Kulah, H., and Tekin, H. C. (2023). Spheroid engineering in microfluidic devices. ACS Omega 8 (4), 3630–3649. doi:10.1021/acsomega.2c06052
Thaweeskulchai, T., and Schulte, A. (2023). Basic guide to multilayer microfluidic fabrication with polyimide tape and diode laser. Micromachines 14 (2), 324. doi:10.3390/mi14020324
Thuau, D., Laval, C., Dufour, I., Poulin, P., Ayela, C., and Salmon, J. B. (2018). Engineering polymer MEMS using combined microfluidic pervaporation and micro-molding. Microsystems Nanoeng. 4 (1), 15–18. doi:10.1038/s41378-018-0017-2
Tran, T. D., Nguyen, P. T., Le, T. N., and Kim, M. il. (2021). DNA-copper hybrid nanoflowers as efficient laccase mimics for colorimetric detection of phenolic compounds in paper microfluidic devices. Biosens. Bioelectron. 182, 113187. doi:10.1016/J.BIOS.2021.113187
Tsagkaris, A. S., Migliorelli, D., Uttl, L., Filippini, D., Pulkrabova, J., and Hajslova, J. (2021). A microfluidic paper-based analytical device (μPAD) with smartphone readout for chlorpyrifos-oxon screening in human serum. Talanta 222, 121535. doi:10.1016/J.TALANTA.2020.121535
Tsao, C. W. (2016). Polymer microfluidics: Simple, low-cost fabrication process bridging academic lab research to commercialized production. Micromachines 7 (12), 225. doi:10.3390/MI7120225
Tully, J. J., and Meloni, G. N. (2020). A scientist’s guide to buying a 3D printer: How to choose the right printer for your laboratory. Anal. Chem. 92 (22), 14853–14860. doi:10.1021/ACS.ANALCHEM.0C03299
Uçak, N., Çiçek, A., and Aslantas, K. (2022). Machinability of 3D printed metallic materials fabricated by selective laser melting and electron beam melting: A review. J. Manuf. Process. 80, 414–457. doi:10.1016/j.jmapro.2022.06.023
van der Linden, P. J. E. M., Popov, A. M., and Pontoni, D. (2020). Accurate and rapid 3D printing of microfluidic devices using wavelength selection on a DLP printer. Lab a Chip 20 (22), 4128–4140. doi:10.1039/D0LC00767F
Vargas, M. J. T., Nieuwoudt, M., Yong, R. M., Vanholsbeeck, F., Williams, D. E., and Simpson, M. C. (2019). Excellent quality microchannels for rapid microdevice prototyping: Direct CO2 laser writing with efficient chemical postprocessing. Microfluid. Nanofluidics 23 (11), 124–213. doi:10.1007/s10404-019-2291-1
Vasilescu, S. A., Khorsandi, S., Ding, L., Bazaz, S. R., Nosrati, R., Gook, D., et al. (2021). A microfluidic approach to rapid sperm recovery from heterogeneous cell suspensions. Sci. Rep. 11 (1), 7917. doi:10.1038/s41598-021-87046-9
Vera, D., García-Díaz, M., Torras, N., Álvarez, M., Villa, R., and Martinez, E. (2021). Engineering tissue barrier models on hydrogel microfluidic platforms. ACS Appl. Mater. Interfaces 13 (12), 13920–13933. doi:10.1021/ACSAMI.0C21573
Verma, N., and Pandya, A. (2022). Challenges and opportunities in micro/nanofluidic and lab-on-a-chip. Prog. Mol. Biol. Transl. Sci. 186 (1), 289–302. doi:10.1016/BS.PMBTS.2021.07.016
Vo, T. N. A., and Chen, P.-C. (2022). Maximizing interfacial bonding strength between PDMS and PMMA substrates for manufacturing hybrid microfluidic devices withstanding extremely high flow rate and high operation pressure. Sensors Actuators A Phys. 334, 113330. doi:10.1016/j.sna.2021.113330
Waheed, S., Cabot, J. M., Macdonald, N. P., Lewis, T., Guijt, R. M., Paull, B., et al. (2016). 3D printed microfluidic devices: Enablers and barriers. Lab a Chip 16 (11), 1993–2013. doi:10.1039/C6LC00284F
Walsh, D. I., Kong, D. S., Murthy, S. K., and Carr, P. A. (2017). Enabling microfluidics: From clean rooms to makerspaces. Trends Biotechnol. 35 (5), 383–392. doi:10.1016/j.tibtech.2017.01.001
Wang, A., Wu, Z., Huang, Y., Zhou, H., Wu, L., Jia, C., et al. (2021). A 3d-printed microfluidic device for qpcr detection of macrolide-resistant mutations of mycoplasma pneumoniae. Biosensors 11 (11), 427. doi:10.3390/bios11110427
Wang, H., and Chen, L. (2020). Electrowetting-on-Dielectric based economical digital microfluidic chip on flexible substrate by inkjet printing. Micromachines 11 (12), 1113. doi:10.3390/mi11121113
Wang, H., Enders, A., Preuss, J. A., Bahnemann, J., Heisterkamp, A., and Torres-Mapa, M. L. (2021). 3D printed microfluidic lab-on-a-chip device for fiber-based dual beam optical manipulation. Sci. Rep. 11 (1), 14584–14612. doi:10.1038/s41598-021-93205-9
Wang, H., Zhang, Y. L., Wang, W., Ding, H., and Sun, H. B. (2017). On-chip laser processing for the development of multifunctional microfluidic chips. Laser & Photonics Rev. 11 (2), 1600116. doi:10.1002/LPOR.201600116
Wang, X., Zheng, X., Song, Z., Lin, D., Li, Q., Qi, J., et al. (2023). Electric yo-yo centrifugation combining with paper-based microfluidic immunoassay chip for inflammatory biomarkers detection in whole blood. Talanta 253, 123883. doi:10.1016/j.talanta.2022.123883
Wang, Y., Cao, X., Messina, W., Hogan, A., Ugwah, J., Alatawi, H., et al. (2021). Development of a mobile analytical chemistry workstation using a silicon electrochromatography microchip and capacitively coupled contactless conductivity detector. Micromachines 12 (3), 239. doi:10.3390/MI12030239
Wei, Y., Wang, T., Wang, Y., Zeng, S., Ho, Y. P., and Ho, H. P. (2023). Rapid prototyping of multi-functional and biocompatible Parafilm®-based microfluidic devices by laser ablation and thermal bonding. Micromachines 14 (3), 656. doi:10.3390/mi14030656
Whitesides, G. M., Ostuni, E., Takayama, S., Jiang, X., and Ingber, D. E. (2001). Soft lithography in biology and biochemistry. Ann. Rev. Biomed. Eng. 3, 335–373. doi:10.1146/annurev.bioeng.3.1.335
Wlodarczyk, K. L., Hand, D. P., and Maroto-Valer, M. M. (2019). Maskless, rapid manufacturing of glass microfluidic devices using a picosecond pulsed laser. Sci. Rep. 9 (1), 20215. doi:10.1038/s41598-019-56711-5
Xia, L., Zhou, W., Huang, J., Dong, J., Xiao, X., and Li, G. (2023). Size-resolved counting of circulating tumor cells on pinched flow-based microfluidic cytometry. ELECTROPHORESIS 44 (1–2), 82–88. doi:10.1002/elps.202200171
Xia, Y., Chu, X., Zhao, C., Wang, N., Yu, J., Jin, Y., et al. (2022). A glass–ultra-thin PDMS film–glass microfluidic device for digital PCR application based on flexible mold peel-off process. Micromachines 13 (10), 1667. doi:10.3390/mi13101667
Yagyu, H., Hamamoto, M., and Wang, Y. (2022). Analyzing the critical mixing time for the liquid-phase reduction synthesis of monodisperse gold nanoparticles using glass microfluidics. Microfluid. Nanofluidics 26 (2), 8–10. doi:10.1007/s10404-021-02517-9
Yandrapalli, N., Petit, J., Bäumchen, O., and Robinson, T. (2021). Surfactant-free production of biomimetic giant unilamellar vesicles using PDMS-based microfluidics. Commun. Chem. 4 (1), 100. doi:10.1038/s42004-021-00530-1
Yao, Y., and Fan, Y. (2021). CO2 Laser Fabrication of hydrogel-based open-channel microfluidic devices. Biomed. Microdevices 23 (4), 47. doi:10.1007/s10544-021-00584-x
Yetisen, A. K., Akram, M. S., and Lowe, C. R. (2013). Paper-based microfluidic point-of-care diagnostic devices. Lab a Chip 13 (12), 2210–2251. doi:10.1039/C3LC50169H
Yoo, S. Y., Kim, S. K., Heo, S. J., Koak, J. Y., and Kim, J. G. (2021). Dimensional accuracy of dental models for three-unit prostheses fabricated by various 3D printing technologies. Materials 14 (6), 1550. doi:10.3390/MA14061550
Yue, L., Macrae Montgomery, S., Sun, X., Yu, L., Song, Y., Nomura, T., et al. (2023). Single-vat single-cure grayscale digital light processing 3D printing of materials with large property difference and high stretchability. Nat. Commun. 14 (1), 1251. doi:10.1038/s41467-023-36909-y
Zhang, P., Wang, Z., Li, J., Li, X., and Cheng, L. (2020). From materials to devices using fused deposition modeling: A state-of-art review. Nanotechnol. Rev. 9 (1), 1594–1609. doi:10.1515/ntrev-2020-0101
Zhao, Y., Lv, X., Li, X., Rcheulishvili, N., Chen, Y., Li, Z., et al. (2023). Microfluidic actuated and controlled systems and application for lab-on-chip in Space Life Science. Space Sci. Technol. 3, 0008. doi:10.34133/space.0008
Zhou, L., Fu, J., and He, Y. (2020). A review of 3D printing technologies for soft polymer materials. Adv. Funct. Mater. 30 (28), 2000187. doi:10.1002/adfm.202000187
Zhou, M., Wu, S., Song, F., Chen, F., Wang, F., Xia, Q., et al. (2022). A facile and green approach toward precise fabrication of silk fibroin-based microfluidic devices using water as etchant. Eur. Polym. J. 179, 111584. doi:10.1016/J.EURPOLYMJ.2022.111584
Zhou, W., Dou, M., Timilsina, S. S., Xu, F., and Li, X. J. (2021). Recent innovations in cost-effective polymer and paper hybrid microfluidic devices. Lab a Chip 21 (14), 2658–2683. doi:10.1039/D1LC00414J
Zhu, L., Mei, X., Peng, Z., Yang, J., and Li, Y. (2022). A paper-based microfluidic sensor array combining molecular imprinting technology and carbon quantum dots for the discrimination of nitrophenol isomers. J. Hazard. Mater. 435, 129012. doi:10.1016/J.JHAZMAT.2022.129012
Zhu, Y., Tong, X., Wei, Q., Cai, G., Cao, Y., Tong, C., et al. (2022). 3D origami paper-based ratiometric fluorescent microfluidic device for visual point-of-care detection of alkaline phosphatase and butyrylcholinesterase. Biosens. Bioelectron. 196, 113691. doi:10.1016/J.BIOS.2021.113691
Zhuang, J., Zhao, Z., Lian, K., Yin, L., Wang, J., Man, S., et al. (2022). SERS-based CRISPR/Cas assay on microfluidic paper analytical devices for supersensitive detection of pathogenic bacteria in foods. Biosens. Bioelectron. 207, 114167. doi:10.1016/J.BIOS.2022.114167
Keywords: microfluidics, lab-on-a-chip, organ-on-a-chip, microfabrication, biochips, chip materials
Citation: Rodríguez CF, Andrade-Pérez V, Vargas MC, Mantilla-Orozco A, Osma JF, Reyes LH and Cruz JC (2023) Breaking the clean room barrier: exploring low-cost alternatives for microfluidic devices. Front. Bioeng. Biotechnol. 11:1176557. doi: 10.3389/fbioe.2023.1176557
Received: 28 February 2023; Accepted: 17 April 2023;
Published: 27 April 2023.
Edited by:
Alfredo De La Escosura-Muñiz, University of Oviedo, SpainReviewed by:
Cesar Fernandez-Sanchez, Spanish National Research Council (CSIC), SpainCopyright © 2023 Rodríguez, Andrade-Pérez, Vargas, Mantilla-Orozco, Osma, Reyes and Cruz. This is an open-access article distributed under the terms of the Creative Commons Attribution License (CC BY). The use, distribution or reproduction in other forums is permitted, provided the original author(s) and the copyright owner(s) are credited and that the original publication in this journal is cited, in accordance with accepted academic practice. No use, distribution or reproduction is permitted which does not comply with these terms.
*Correspondence: Luis H. Reyes, bGgucmV5ZXNAdW5pYW5kZXMuZWR1LmNv; Juan C. Cruz, amMuY3J1ekB1bmlhbmRlcy5lZHUuY28=