- 1Department of Orthopaedics, Third Xiangya Hospital of Central South University, Changsha, Hunan, China
- 2Institute of Basic Medicine and Cancer (IBMC), Chinese Academy of Sciences, Hangzhou, Zhejiang, China
Three-dimensional (3D) bioprinting is an advanced tissue engineering technique that has received a lot of interest in the past years. We aimed to highlight the characteristics of articles on 3D bioprinting, especially in terms of research hotspots and focus. Publications related to 3D bioprinting from 2007 to 2022 were acquired from the Web of Science Core Collection database. We have used VOSviewer, CiteSpace, and R-bibliometrix to perform various analyses on 3,327 published articles. The number of annual publications is increasing globally, a trend expected to continue. The United States and China were the most productive countries with the closest cooperation and the most research and development investment funds in this field. Harvard Medical School and Tsinghua University are the top-ranked institutions in the United States and China, respectively. Dr. Anthony Atala and Dr. Ali Khademhosseini, the most productive researchers in 3D bioprinting, may provide cooperation opportunities for interested researchers. Tissue Engineering Part A contributed the largest publication number, while Frontiers in Bioengineering and Biotechnology was the most attractive journal with the most potential. As for the keywords in 3D bioprinting, Bio-ink, Hydrogels (especially GelMA and Gelatin), Scaffold (especially decellularized extracellular matrix), extrusion-based bioprinting, tissue engineering, and in vitro models (organoids particularly) are research hotspots analyzed in the current study. Specifically, the research topics “new bio-ink investigation,” “modification of extrusion-based bioprinting for cell viability and vascularization,” “application of 3D bioprinting in organoids and in vitro model” and “research in personalized and regenerative medicine” were predicted to be hotspots for future research.
1 Introduction
Three-dimensional (3D) bioprinting is a technology that enables the 3D printing of various cells, biocompatible materials, and supporting components into complex 3D functional living tissues (Murphy and Atala, 2014). This technique allows to print cell-embedded biomaterials in a layer-by-layer manner using computer-controlled automated dispensing systems (Matai et al. 2020). This method enables single or multiple cells to be arranged in a specified form in biomaterials, thereby realizing the construction of functional 3D tissues with cells (Derby, 2012; Tasoglu and Demirci, 2013; Ozbolat, 2015).
The advantage of 3D bioprinting technology lies in its high printing resolution, which allows for precise allocation of cells, matrix, biomolecules, and biological materials to mimic the natural tissue structure (Jana and Lerman, 2015). Traditional tissue engineering techniques can only control volume characteristics through fiber bonding, freeze-drying, solvent casting and electrospinning, et al. but cannot customize pore size, shape, network, internal structure, and topological structure. Besides, traditional tissue engineering methods also cannot easily achieve specific requirements for porosity. With the assistance of computer-aided design (CAD) technology, 3D bioprinting can efficiently and economically produce complex 3D structures ranging from nanoscale to microscale (Huang et al. 2017). Due to the superior performance of the 3D bioprinting technology, it has been a research hotspot in various fields of tissue engineering, such as transplant and regenerative medicine, as well as disease model construction. For instance, several types of bio-ink and printing processes have been developed for applications in tissue engineering (Liang et al. 2022; Luo et al. 2022; Murab et al. 2022; Sonaye et al. 2022; Thangadurai et al. 2022). In addition, 3D bioprinting has been widely used in artificial organ fabrication and tissue regeneration (Ramadan and Zourob, 2020; Jain et al. 2022; Panda et al. 2022; Pourmasoumi et al. 2022; Wang et al. 2023). Moreover, owing to its unique advantages, 3D bioprinting plays a key role in the construction of common disease in vitro models and organoids (Fan et al. 2019; Guagliano et al. 2022; Joddar et al. 2022; Jubelin et al. 2022; Neufeld et al. 2022; Shakir et al. 2022).
Over the past 20 years, there have been continuous breakthroughs in 3D bioprinting research, especially in the modification of bio-ink and printing technology, as well as their applications. Therefore, it is necessary to discuss the progress in this field. Bibliometrics is an interdisciplinary science of quantitative analysis of all knowledge using mathematical and statistical means, which can estimate the structure and development of specific scientific disciplines (Tang et al. 2021b; Tang et al. 2021c; Ding et al. 2022). Although there have been related studies published in the field of 3D bioprinting (Naveau et al. 2017; Santoni et al. 2022), the exponentially growing number of articles requires the latest bibliometric analysis to help researchers in this field understand the latest hot topics.
To further understand the development of 3D bioprinting, in this study we propose to use bibliometric methods to highlight the characteristics of articles on 3D bioprinting, especially in terms of research hotspots and focus. We hope our research can provide a more comprehensive understanding for researchers in the field of 3D bioprinting about the current state and future research development trends.
2 Materials and methods
2.1 Data collection
As an influential citation-based database, the Web of Science Core Collection (WoSCC) database has been widely used in bibliometric studies (Zhang et al. 2020; Zhu et al. 2020; Cheng et al. 2022). We performed a comprehensive online search in this database on the last day of November 2022 to reduce bias caused by database updates. We use “topic” as the search scope, which mainly includes the title, abstract, keyword, and other relevant elements sections of the article. The search formula was as follows: [#1: TS = (“bioprint*” OR “bio-print*”); #2: TS = (3D OR “3 dimensions” OR “three dimensions” OR “three dimensional” OR “3 dimensional”); Final dataset: #1 AND #2]. Language was limited to English. No time and publication type restrictions were used in this search. A total of 4,823 search results were retrieved in the initial search. Then, two researchers (ZYD and NT) manually examined the information of the retrieved documents, such as titles, abstracts, and the full text. Publications not related to 3D bioprinting were excluded (Supplementary Table S1). Finally, a total of 3,327 manuscripts were identified and included for further analysis. The flow chart is illustrated in Supplementary Figure S1.
2.2 Data analysis and visualization
Several software are frequently used in bibliometric analysis, including VOSviewer, CiteSpace, and HistCite (Zhang et al. 2021a). Two software and one online site were applied to the following analysis in this study.
VOSviewer 1.6.18 is a widely used software for constructing knowledge-maps based on a co-occurrence matrix (Farzanegan et al. 2017). The general information was standardized before analysis. Standardized author keywords that were manually paraphrased by the authors reduced the bias better. For example, we included “three-dimensional bioprinting” under “3d bioprinting” (Romero and Portillo-Salido, 2019). In terms of national information, we classified Taiwan as China (Gao et al. 2019). This bibliometric tool provided co-occurrence/co-citation/co-authorship maps of the restricted data. Generally, the larger the size of the diverse nodes/words, the more times they occur. The color line between two nodes represents the degree of connection. The thicker the line, the more widespread the cooperation (Shi et al. 2021). The color of the nodes indicates different average publication years.
CiteSpace 6.1. R2 is another bibliometric software for creating visualization maps based on the data retrieved from the database (Cheng et al. 2022; Feng et al. 2022). Several knowledge-based visual maps were constructed, such as citation bursts map, timeline view, and dual-map overlay. The basic parameter settings were as follows: years per slice (1), pruning (minimal spanning tree and pruning sliced nets), and inclusive standards (top N = 50), others were set as default (Zhang et al. 2021a). In the co-occurrence of countries/regions and institutions maps, node colors changed from blue to red representing the publication years from 2007 to 2022.
Moreover, an online platform (https://bibliometric.com/app_v0) was used for the comparison analysis of the annual number of papers among the top 10 countries. GraphPad Prism 8 and Microsoft Office Excel 365 were respectively used to draw a column chart and provide a descriptive analysis for annual publications. Impact factor (IF) scores were extracted from 2021 Journal Citation Reports (JCR).
3 Results
3.1 Publication outputs and trends
Based on the search strategy, a total of 3,327 papers were identified. The number of annual publications regarding 3D bioprinting is illustrated in Figure 1. From 2007 to 2022, the number of publications per year showed a rapid growth trend, reaching over 100 publications for the first time in 2015. To evaluate the change trend, a power function (y = 0.2085 × 2.7219, R2 = 0.9873) of the trend was created, where X represents the year and Y indicates the amount of annual publications.
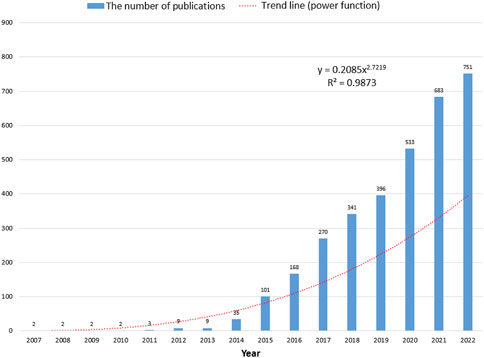
FIGURE 1. The corresponding number of annual publications regarding 3D bioprinting from 2007 to 2022.
3.2 Most prolific countries/regions, funding agencies and institutions
All publications regarding 3D bioprinting were published by 2,733 institutions in 83 countries/regions in total. The top 10 prolific countries and institutions are listed in Table 1. Figure 2A illustrates the annual number of publications of the top 10 countries from 2007 to 2022. The most productive country is United States (n = 1,069), followed by China (n = 733), South Korea (n = 288), Germany (n = 210), and India (n = 166). As shown in Table 1, the United States has the highest centrality of 0.34 among all the countries, which is much higher than that of other countries/regions. The international cooperation analysis is shown in Figure 2B, in which the line between two counties indicates a cooperative relationship. The United States has the most cooperation with other countries in 3D bioprinting. The overlay visualization map among the countries is illustrated in Figure 2C. Countries/regions with a minimum of 10 publications were identified. The top 10 funding agencies, of which four are based in the United States, are summarized in Figure 2D. The National Natural Science Foundation of China is the most frequent funding source.
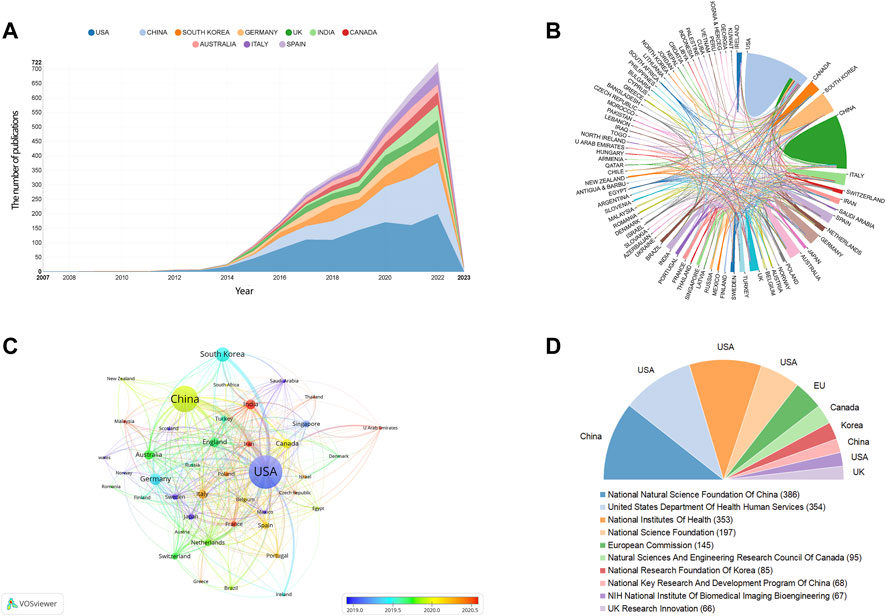
FIGURE 2. The distribution of counties/regions and funding agencies in 3D bioprinting research. (A) The number of publications per year of the top 10 countries. (B) The international cooperation analysis. The line between two counties indicates cooperative relationship. (C) The overlay visualization of co-authorship. The size of notes/words represent the total publications of a countries. The color line between two nodes represents the degree of co-authorship. The color of the nodes indicated different average publication year. (D) The top ten frequent funding agencies and corresponding countries.
The co-occurrences of institutions are presented in Figure 3A and the top 10 prolific institutions are listed in Table 1. Harvard Medical School (n = 86) is the institution with the largest number of publications, followed by Tsinghua University (n = 75), Chinese Academy of Sciences (n = 74), Zhejiang University (n = 69), and Wake Forest School of Medicine (n = 56). University of California, Los Angeles, has the highest centrality of 0.18. Of the top 10 most prolific institutions, four were from China and four were from United States. Moreover, an institution co-authorship analysis is shown in Figure 3B. Based on the color gradient, the newer average publication year of institutions, such as Chinese Academy of Sciences, Shanghai Jiao Tong University, and Yonsei University, was assigned the color red.
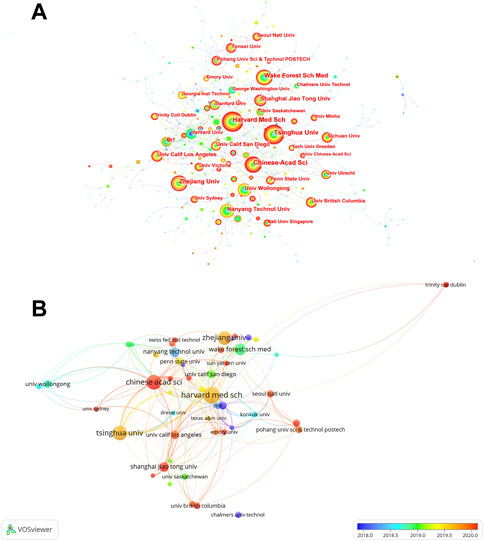
FIGURE 3. The distribution of institutions in 3D bioprinting research. (A) Co-occurrences of institutions. The notes represent corresponding institution. The size of notes/words represent the total outputs of one institution. The value between lines indicates the cooperation degree among two connected institutions. (B) Overlay visualization map of co-authorship.
3.3 Analysis of influential authors and Co-cited authors
The top 25 most prolific authors are shown in Figure 4A. Among them, the information of the top 10 most productive authors is illustrated in Figure 4B and Table 2. Atala A (n = 63) contributed the largest number of papers, followed by Lee SJ (n = 53), Gatenholm P (n = 41), Zhang YS (n = 39), and Yoo JJ (n = 39). These and the rest of the authors are listed in Table 2. Figure 4B illustrates the annual publications of these authors. Published articles are mainly from 2014 to 2022. The visualization of the top 25 prolific author relationships was shown in Figure 4C. We can notice more collaborations between Atlas A, Lee SJ, Yoo JJ, Kim J, Lee J, Zhang YS, Khademhosseini A, Cho DW, Park SA and Jiang J, but there almost no collaboration among other highly productive authors.
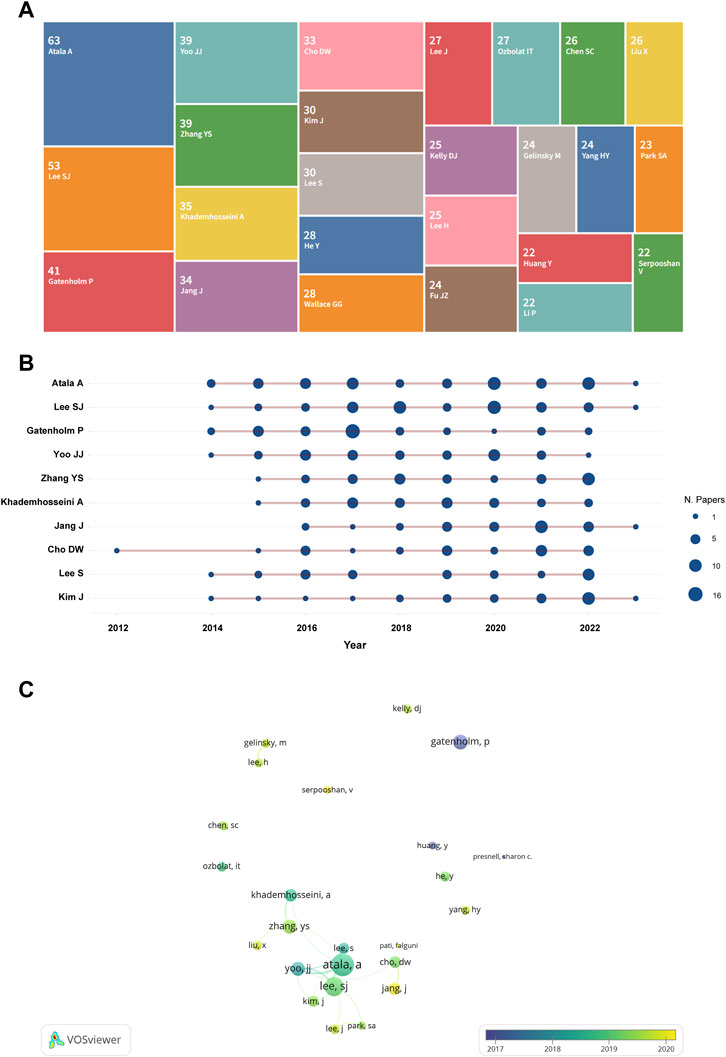
FIGURE 4. The overlay visualization of authors in 3D bioprinting research. (A) Tree map of the top 25 productive authors. The number above the name represent the amount of the publications. (B) Top 10 authors’ outputs over time. The size of the deep blue circle indicates the number of papers (N. papers). (C) The visualization of the top 25 prolific author relationships.
The density maps of co-cited authors based on co-citations are shown in Figure 5, co-cited authors with ≥250 co-citations were included. The authors with the most co-citations are Murphy SV (n = 1,139), followed by Ozbolat IT (n = 806), Skardal A (n = 691), Kolesky DB (n = 650), and Pati F (n = 589). These and the rest of the top 10 co-cited authors are listed in Table 2.
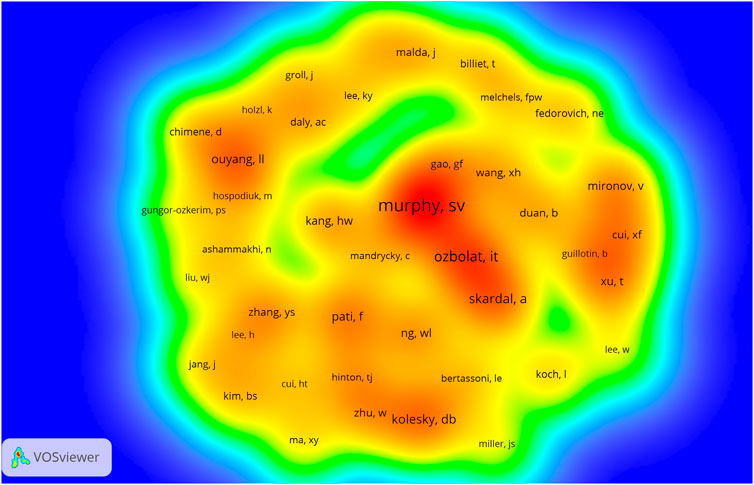
FIGURE 5. The density visualization of co-cited authors in 3D bioprinting research. The size of the circle/word are positively related to the number of co-citations.
3.4 Most active journals
The articles included in the analysis were published in 752 journals. Of these, 33 published a minimum of 20 papers and were included and visualized in Figure 6A. The top 10 most prolific journals and their basic information are shown in Table 3. The journal that published the greatest number of papers is Tissue Engineering Part A (n = 225), followed by Biofabrication (n = 191), Advanced Healthcare Materials (n = 82), International Journal of Bioprinting (n = 81), and Frontiers in Bioengineering and Biotechnology (n = 65). All the top 10 most productive journals had an IF (2021) of >4. Seven of these journals were categorized in the Q1 JCR division.
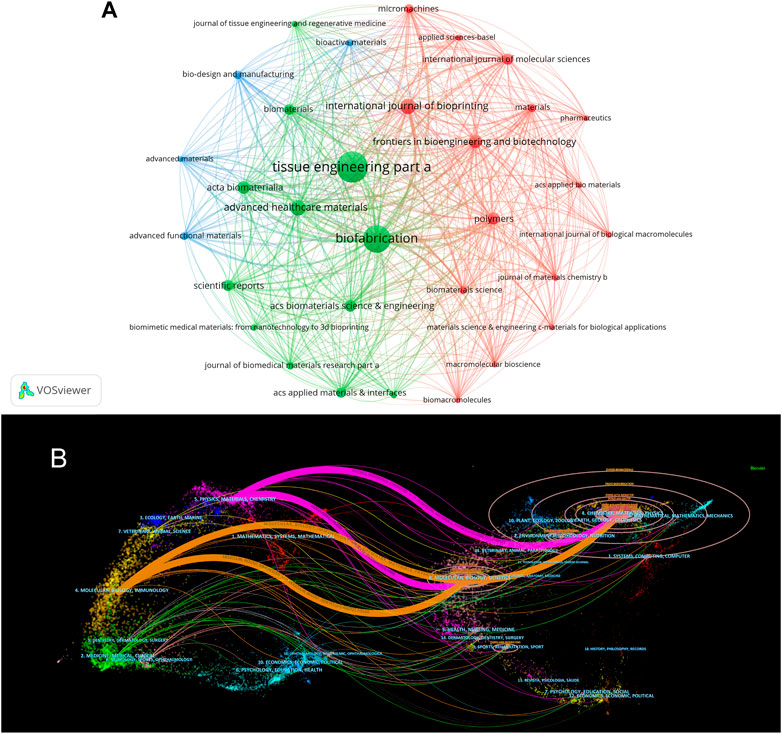
FIGURE 6. The visualization of journals in 3D bioprinting research. (A) Network visualization map of journals analysis. (B) Dual-map overlay of journals. The citing journals are on the left, the cited journals are on the right. The coloured path represents citation association of journals.
The dual-map overlay of journals created using CiteSpace is shown in Figure 6B. Four citation paths were recognized, indicating that the studies published in Molecular/Biology/Immunology or Physics/Materials/Chemistry journals were primarily cited by the research published in Molecular/Biology/Genetics or Chemistry/Materials/Physics journals.
3.5 Co-cited references and reference burst
Table 4 summarizes the top 10 most co-cited references related to 3D bioprinting research. The publication year of these papers was between 2014 and 2019. Two of these papers’ co-cited references were cited over 300 times. Nature Biotechnology and Biotechnology Advances both have two publications in the list. Of the top ten most cited references, six are review articles and four are research articles. The six review articles mainly summarize the applications and advances of bioinks (Hölzl et al. 2016; Hospodiuk et al. 2017; Gungor-Ozkerim et al. 2018) and 3D bioprinting technology (Murphy and Atala, 2014; Mandrycky et al. 2016; Ozbolat and Hospodiuk, 2016). Regarding the four research articles, Kolesky DB firstly proposed a bioprinting method that can be used to manufacture 3D tissue structures filled with vascular systems, multiple types of cells, and extracellular matrix, this structure opens new avenues for basic research in drug screening and wound healing, angiogenesis, and stem-cell niches (Kolesky et al. 2014). This team also proposed a new 3D bioprinting method that can create thick human tissue filled with engineered extracellular matrix, embedded vascular systems, and multiple types of cells, the proposal of this new method greatly promotes the development of using 3D bioprinting technology to construct human tissues for in vitro and in vivo applications (Kolesky et al. 2016). In 2006, Atala A led his team to develop an integrated tissue–organ printer (ITOP) that can fabricate stable, human-scale tissue constructs of any shape, they also demonstrated the use of the 3D bioprinter to manufacture mandibles, cranial bones, cartilage, and skeletal muscle. ITOP may be the prototype of various types of 3D bioprinters currently available, and its emergence has made it possible to construct more complex tissues and solid organs (Kang et al. 2016). In 2019, (Lee et al. 2019) proposed a method named FRESH (Freeform Reversible Embedding of Suspended Hydrogels) to 3D bioprint collagen, and used this method to construct heart tissue with patient-specific anatomical structures. This technology achieved gelation through pH control and has higher resolution (20-μm), making an important step in the study of using 3D bioprinting to construct organ tissues.
Analysis of the references is helpful to understand the development of a field. Figure 7 illustrates the top 30 references with the strongest citation bursts, all of which begun in 2014. Notably, the paper with the greatest number of co-citations (n = 470) and the highest strength of citation bursts (Strength = 120.73) was published in Nature Biotechnology by Murphy SV et al. in 2014 (Murphy and Atala, 2014). The second top co-cited publication was that by Kang HW et al. published in Nature Biotechnology (Kang et al. 2016). The third most co-cited paper was published in Biomaterials by Ozbolat and Hospodiuk (2016). Among all thirty articles, 6 are reviews and 24 are original research. In the top ten references with the strongest citation bursts, three of them overlap with the top 10 most co-cited references (Kolesky et al. 2014; Murphy and Atala, 2014; Lee et al. 2019), and two of the remaining seven articles are review papers mainly about engineering hydrogels for biofabrication (Malda et al. 2013), printing and prototyping of tissues and scaffolds (Derby, 2012). In the remaining five studies, Most of the studies are about different bioinks, including alginate/gelatin hydrogels (Duan et al. 2013), carbohydrate glass (Miller et al. 2012), decellularized extracellular matrix (Pati et al. 2014) and soft protein/polysaccharide hydrogels (Hinton et al. 2015). The development and improvement of bioinks are important components of 3D bioprinting research, as confirmed by early research directions. Besides, in addition to improving the biological ink matrix, research on cells is also very important. Noor et al. (2019) induced patient cells to become pluripotent stem cells and differentiated them into cardiac and endothelial cells. They used these two types of cells, along with collagenous nanofibers hydrogel, and 3D bioprinting technology to construct a cellularized human hearts with a natural architecture, this method demonstrated the potential for designing personalized tissues and organs.
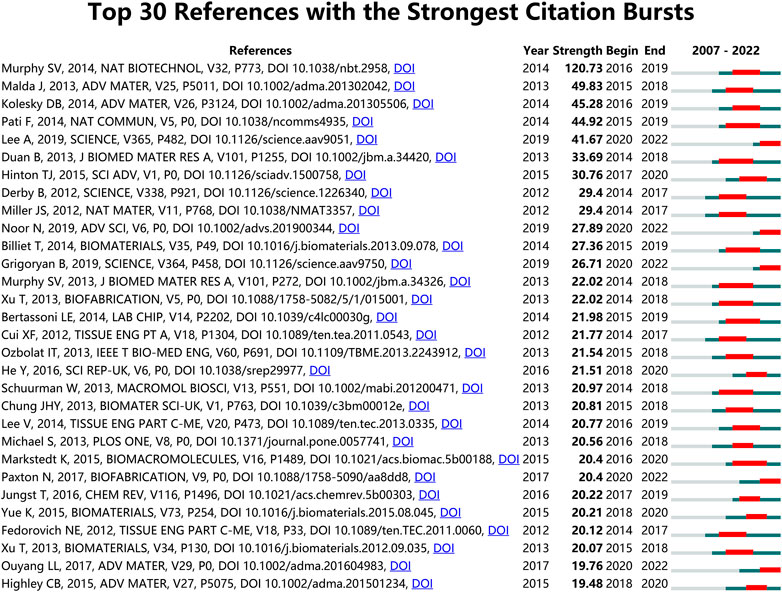
FIGURE 7. Top 30 references with the strongest citation bursts in 3D bioprinting research. Ranked by strength. The bars in red stands for a burst period for the references.
Analysis of Co-occurring Keywords and Related Genes; Keywords can further represent the hot issues in a related research field during a certain period, and recently erupted keywords can represent current research hotspots (Zhang et al. 2020; Wang H. et al. 2022). A total of 5,053 author keywords were identified, of which 49 with a minimum of 20 occurrences were extracted to create an overlay visualization map (Figure 8A). The top 20 author keywords based on the number of occurrences are shown in Figure 8B. 3D Bioprinting, Tissue Engineering, Bioprinting, 3D Printing, Bio-ink, Hydrogels, Biomaterials, Biofabrication, Regenerative Medicine, and Scaffold were ranked in the top ten in the occurrences of author keywords. As shown in Table 5, Cartilage Tissue Engineering, Cell Viability, Extrusion, Bioprinting, and Vascularization were the top five author keywords with the highest number of average citations. The top 30 author keywords of the average publication year are listed in Supplementary Table S2. Of these, “Decellularized Extracellular Matrix,” “In Vitro Models,” “Personalized Medicine,” “GelMA,” and “Wound Healing” were the top five author keywords with the newest average publication year. It is not surprised that keywords such as “3D bioprinting,” “Bio-ink,” “Tissue engineering” and “hydrogels,” et al. have become high-frequency keywords in recent times. Interesting to note is that according to the timeline, “Decellularized Extracellular Matrix,” “In Vitro Models,” “Personalized Medicine” and “Tumor microenvironment” have become the latest high-frequency keywords. Therefore, we speculate that these may be the hot research directions in the field of 3D bioprinting in the near future.
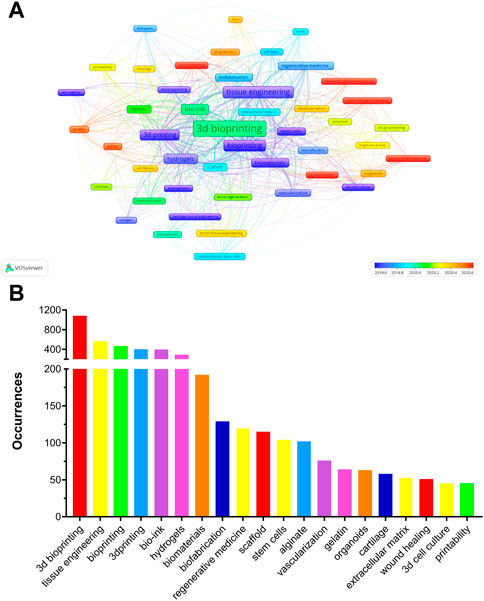
FIGURE 8. (A) The overlay of author keywords in 3D bioprinting research. The size of notes/words represent occurrences. The colour of the nodes indicated average publication year based on the strip of colour. (B) Top 20 author keywords with most frequent occurrences in 3D bioprinting research.
Moreover, the results of thematic evolution analysis are shown in Figure 9A. The top 10 author keywords with the most occurrences in the corresponding period were identified. A three-field plot was applied to illustrate the evolution of three periods in the field of 3D bioprinting. As indicated in Figure 9B, the nodes indicate co-cited references in the timeline view map. All the co-cited references could be clustered into nine specific clusters based on the author keywords (Modularity Q value = 0.69, Weighted mean silhouette value = 0.86), including “#0 3D bioprinting,” “#1 interface,” “#2 tissue engineering,” “#3 cell printing,” “#4 implant development,” “#5 vasculogenesis,” “#6 bioartificial organ,” “#7 biomaterials,” and “#8 micro/nano.” Tissue engineering plays a very important role in the field of 3D bioprinting without doubt. “Vasculogenesis” is a new research direction in the field of 3D bioprinting in recent years. Due to the dependency of cells on oxygen and nutrients, vascularization during the 3D bioprinting process is a key focus for constructing engineered tissues and organs (Anthon and Valente, 2022). Moreover, the top 20 author keywords with the strongest citation bursts are shown in Figure 10. Freeform fabrication was once the hottest topic of interest in this research field, with the strongest citation burst. Chondrocyte, osteogenic differentiation, alginate hydrogel, and composite scaffold are four keywords with citation burst in the recent 4 years, which could represent the current research hotspots in this field. The weighted mean silhouette value was 0.86 and the modularity Q was 0.69, indicating the rationality of this clustering method.
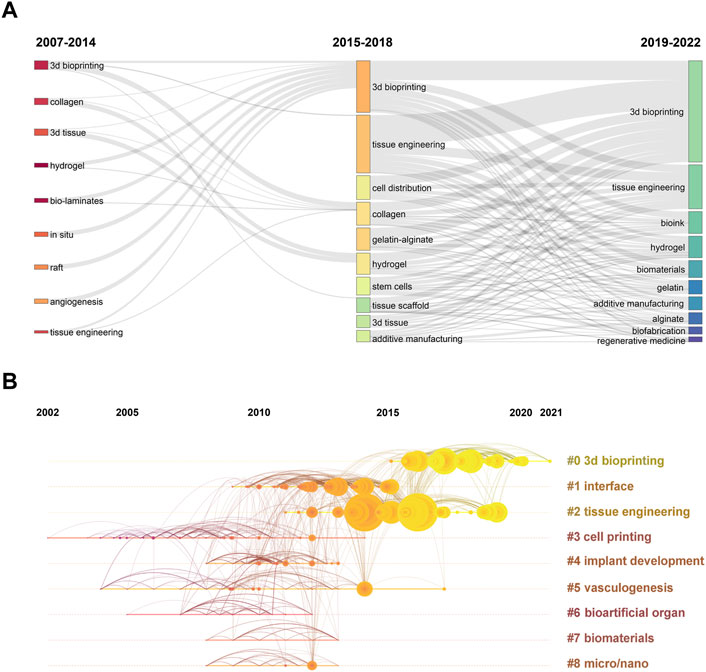
FIGURE 9. (A) Three-field plot of author keywords in 3D bioprinting research. The thick of the notes represent the relative co-occurrence frequency in a specific period. (B) The timeline view of co-cited reference in 3D bioprinting research. Years from 2002 to 2021 are arranged horizontally at the top, 9 clusters based on author keyword were identified and listed on the right. The larger the size of the circle, the more studies on the theme.
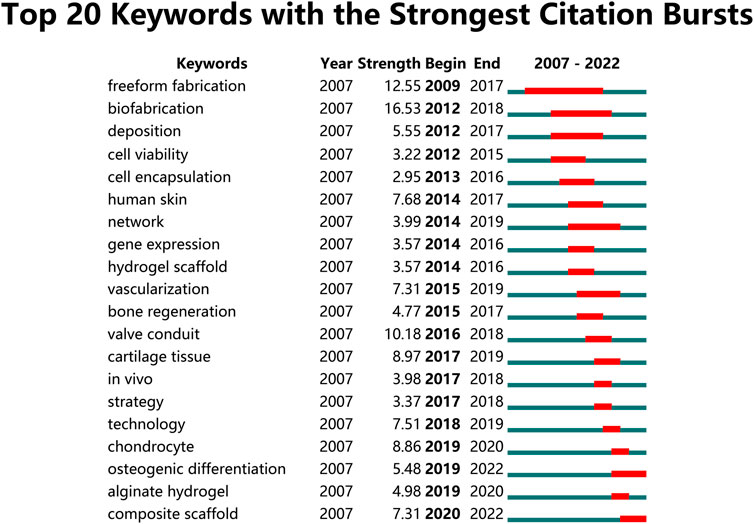
FIGURE 10. Top 20 author keywords with the strongest citation bursts in 3D bioprinting research. Ranked by begin year of citation burst. The bars in red stands for a burst period for the keywords.
4 Discussion
4.1 General information
The development of the annual output and the number of citations are useful indicators for the identification of trends in scientific research areas. By analysing the publication of global scientific publications, we can better understand the characteristics and current status of global scientific publications (Qin et al. 2020). The growing research fronts and future research interests in the field of 3D bioprinting over the past nearly 20 years are identified in this study. The data extracted from WoSCC database showed that 12,728 authors from 2,733 institutions in 83 countries/regions published 3,327 papers related to 3D bioprinting in 752 journals between 1 January 2007, and 30 November 2022.
Based on the trend line fitting the results shown in Figure 1, the numbers of published papers showed a rapid growth trend. The number of articles published each year did not exceed 100 before 2014, as the 3D bioprinting technology had just been proposed, while there were still many technical problems that had not been solved. With the development and commercialization of 3D bioprinters and bio-ink, an increasing number of research studies have been carried out in the field of 3D bioprinting, which explains the explosive growth of 3D bioprinting-related research after 2014 (Nishiyama et al. 2009; Guillemot et al. 2010; Chang et al. 2011; Mironov et al. 2011). Based on this growth trend, we believe that 3D bioprinting attracted more research attention and interest these years and predict that the number of papers in this field will further increase.
4.2 Countries and institutions
Based on the analyses of countries/regions and institutions shown in Figure 2, the United States and China were the two most productive countries. Furthermore, researchers from these two countries had more communication and cooperation in the 3D bioprinting field. This result was also found in 3D bioprinting-related fields, such as tissue engineering (Santisteban-Espejo et al. 2018), biomaterials in osteogenesis (Wang J. et al. 2022), nanocomposite hydrogels (Zhao et al. 2022) and stem cell precision medicine (Liu et al. 2022). It has been reported that research outputs are positively correlated with the gross domestic product (Tang et al. 2021c; Ding et al. 2022). Furthermore, China and the United States also spend the most research and development funds in this field, which can explain why the number of papers they publish is 1.3-fold higher than the total of any other country in the remainder top 10 countries. South Korea and Germany also had a number of publications exceeding 200, and researchers from the two countries also had close communication with the United States and China, suggesting that communication and cooperation is an important way to promote the development of this field.
Similar to the country distribution, most of the ten most prolific publishing institutions were in the United States and China. Harvard Medical School, Tsinghua University, Chinese Academy of Sciences, Zhejiang University, and Wake Forest School of Medicine, the best scientific research institutions in the United States and China, ranked in the top five in the list of the most prolific institutions. Obviously, excellent scientific research institutions are in a leading position in most fields and lead the way. University of California-Los Angeles has a top centrality of 0.18, suggesting that it may play an essential role in the 3D bioprinting field. International cooperation between different institutions is active as well, which is critical to the prosperity of this field (Feng et al. 2022).
4.3 Authors and Co-cited authors
Recognizing the influential scholars in a specific field can provide younger researchers with guidelines and direction. Among the authors with the most publications shown in Figure 4 and Table 2, Dr. Anthony Atala from Wake Forest School of Medicine was the leader with 63 articles and 113.14 times average citations per paper. As the director of the Wake Forest Institute for Regenerative Medicine, he and his team have developed 15 clinically-used technology-based applications, including muscle, urethra, cartilage, reproductive tissues, and skin. Furthermore, thay have developed specialized 3D printers to engineer tissues and achieved progress in organoids and body-on-a-chip systems (Atala et al. 2012; De Filippo et al. 2015; Kang et al. 2016; Wang et al. 2018; Jorgensen et al. 2020; Murphy et al. 2020). As the world’s leading expert in 3D bioprinting, Dr. Anthony has created many “world first” records in the 3D bioprinting field, such as developing a 3D bioprinter (the Integrated Tissue and Organ Printing System) to print living tissue structures over 14 years (Kang et al. 2016), and a novel hybrid strategy based on 3D bioprinting to fabricate endothelialized myocardium (Zhang et al. 2016). Another researcher is equally noteworthy, Dr. Ali Khademhosseini from the department of bioengineering, University of California-Los Angeles and Terasaki Institute for Biomedical Innovation, who has 35 articles published but 122.97 times average citations per paper and 27 H-index. Dr. Khademhosseini has made great contributions in the field of 3D bioprinting, including perfusable vascular constructs using direct 3D bioprinting (Jia et al. 2016), oxygenated cell-laden gelatin methacryloyl constructs using 3D bioprinting (Erdem et al. 2020) and a gelatin methacryloyl (GelMA)-based bio-ink for 3D bioprinting (Adib et al. 2020). Certainly, Dr. Khademhosseini and his team have made great progress in applying bioengineering solutions to precision medicine. Notably, all the top 10 authors have achievements each year, therefore, we infer that the 3D bioprinting field has excellent prospects. The collaboration among the top 25 highly productive authors is not as extensive as expected, which may be due to their different countries and research institutions or the impact of the COVID-19, et al.
As for the co-cited authors shown in Figure 5 and Table 2, Dr. Sean V Murphy from Wake Forest Institute for Regenerative Medicine and Dr. Ibrahim T Ozbolat from the Department of Engineering Science and Mechanics, Penn State University, have published several highly cited review papers on 3D bioprinting (Ozbolat and Yu, 2013; Dababneh and Ozbolat, 2014; Murphy and Atala, 2014; Ozbolat and Hospodiuk, 2016; Hospodiuk et al. 2017). These review articles help other scholars quickly and accurately understand the field of 3D bioprinting. In short, the top 10 authors with the most influential publications and the most co-citations have been leading the entire discipline forward.
4.4 Influential journals
Journals are an important vector for the dissemination of academic research results. We summarized the co-citation visualization network of the most influential journals in the field of 3D bioprinting, making it easier for researchers to choose the most suitable journals to submit papers (Cheng et al. 2022). As shown in Figure 6 and Table 3, Tissue Engineering Part A, Biofabrication, Advanced Healthcare Materials, International Journal of Bioprinting, and Frontiers in Bioengineering and Biotechnology take up the top five positions. Tissue Engineering Part A published the most 3D bioprinting research. This is an authority journal in tissue engineering that mainly focuses on the fundamental and applied experimental aspects for the development of therapeutic strategies to repair or regenerate tissue and organ function. It is worth noting that Frontiers in Bioengineering and Biotechnology is the journal mainly focusing on bioengineering and biotechnology. We believe that this journal will occupy a more important position in the field of 3D bioprinting in the future. Meanwhile, journals in JCR Q1 division accounted for 70% of the top 10 journals, indicating that these journals attract the interest of many researchers and play essential roles in the 3D bioprinting research field.
The dual-map overlay of journals stands for the topic distribution of academic journals (Chen and Leydesdorff, 2014). Figure 6 shows four citation paths, Physics/Materials/Chemistry co-cited journals to Chemistry/Materials/Physics and Molecular/Biology/Genetics, Molecular/Biology/immunology co-cited journals to Chemistry/Materials/Physics and Molecular/Biology/Genetics. It represents the theme distribution of the corresponding journal. The citing journals are on the left and the cited journals on the right. The saffron and purple paths reflect the relationships between the citing and cited journals, respectively (Zhang et al. 2021a). This result indicates that 3D bioprinting is an important interdisciplinary field, and that the cooperation between Chemistry/Materials/Physics and Molecular/Biology/Genetics/immunology is the current mainstream research direction.
4.5 The top 10 most Co-cited references
A co-cited reference means that an article was cited as a reference in different articles (Shen et al. 2022). The knowledge base for this study is a compilation of references cited by the included papers. Therefore, it is not the same as a highly cited paper.
According to the references listed in Table 4, most papers (60%) are reviews. Nature Biotechnology published the most co-cited reference papers by Dr. Sean V Murphy and Dr. Anthony Atala in 2014 with a total of 470 co-cited times until now (Murphy and Atala, 2014). This review article provides a comprehensive review of the applications of 3D bioprinting in tissue and organ engineering, mainly focusing on the strategy for printing organizational structure, different types of bioprinters and their impact on printing tissue structures, the process of printing tissue, limitations of current techniques, and challenges for future research. Certainly, the two top researchers initiated the comprehensive development of 3D bioprinting with this article.
Two years later, Dr. Anthony Atala and his team published the second most co-cited publication in the same journal (Kang et al. 2016). The team presented an integrated tissue–organ printer that can fabricate stable, human-scale tissue constructs of any shape. They constructed mandible bone, calvarial bone, cartilage and skeletal muscle. This innovative 3D bioprinter that can produce human-scale tissue is the prototype of the most commercial 3D bioprinters.
The third most co-cited paper was a review article by Dr. Ibrahim T Ozbolat and Dr. Monika Hospodiuk in 2016 in Biomaterials (Ozbolat and Hospodiuk, 2016). Extrusion-based bioprinting (EBB) has made noteworthy progress from 2006 to 2016, and this article comprehensively reviewed this technology for the first time. Specifically, the authors discussed the current progress in EBB technology, and highlighted the future directions for upgrading the technology to produce applicable products for tissue engineering and regenerative medicine. The rest of the top 10 most co-cited articles were published between 2014 and 2019 (Table 4).
4.6 The top references with the strongest citation burst
References with high burst values in a particular time period indicate that they have received a lot of attention during the corresponding time span (Sun et al. 2022). The references with the top 30 strongest citation bursts are listed in Figure 7. As expected, the strongest citation burst reference was still the review article published by Dr. Sean V Murphy and Dr. Anthony Atala in 2014 in Nature Biotechnology with a 120.73 strength, proving once again the importance of this article in the field (Murphy and Atala, 2014). Dr. Jos Malda, et al. published a 25th anniversary article about engineering hydrogels for biofabrication in Advanced Materials in 2013 (Malda et al. 2013). As the second most cited burst reference, this article mainly focused on the important physicochemical aspects for the development and characterization of hydrogels for biofabrication, and discussed how they may impact the composition and properties of hydrogel bio-inks in the future.
The time of citation burst of all these 30 references started after 2014 and ended approximately in 2022, further proving that the 3D bioprinting technology-related research achieved rapid development after 2014 and became a hotspot in tissue engineering and regenerative medicine recently.
4.7 The hot topics and future trends of 3D bioprinting
Analysis of author keyword occurrences can reveal the research interest areas and hotspots in a specific field (Xiao et al. 2017). The author keywords with high occurrence are shown in Figure 8. 3D Bioprinting, Tissue Engineering, Bioprinting, Bio-ink, 3D Printing, Hydrogels, Biomaterials, Biofibrication, Regeneration Medicine, and Scaffold were listed in the top ten. These can be regarded as the hotspot research interest areas in 3D bioprinting. Besides, Cartilage Tissue Engineering, Cell Viability, Extrusion, Bioprinting and Vascularization have the top five highest average citations in Table 5.
Furthermore, the changing of research hotspots and directions are capable of predicting the future trends in the field with ease as shown in Figure 9A. When comparing the changes in research hotspots between the past 8 years and the recent 4 years, we can clearly see that there have been significant changes. Firstly, 3D bioprinting has gained increasing attention, while the hotspot of tissue engineering has slightly decreased. However, since 3D bioprinting is a branch of tissue engineering research, we can understand this shift in hot topics as 3D bioprinting gaining more attention in the field of tissue engineering. In addition, it is not surprising that bioink has emerged as a hot keyword in the past 4 years. As one of the most crucial components of 3D bioprinting technology, it is probably one of the hot trends for future research. The attention given to hydrogels has significantly increased, and as a material that is, highly suitable for bioink applications, it is widely recognized as a future development trend in 3D bioprinting. Biomaterials have also emerged as a hot keyword in the past 4 years, and we believe that the application of 3D bioprinting in biomaterials production will experience significant development in the coming years. Gelatin and alginate are two of the earliest discovered hydrogels, but their attention has decreased in the past 4 years as various other hydrogels with more advantages have been developed. It is undeniable that they are high-quality hydrogels and have been applied in various fields, but their future research will gradually decrease. Besides, as a part of the additive manufacturing and biofabrication field, the development of 3D bioprinting has promoted the advancement of additive manufacturing and biofabrication technology. Regenerative medicine, as the biggest application direction of 3D bioprinting, is probably a highly focused field in the future.
Analysis author keywords of the average publication year can also make certain predictions about the future development trends in the field as shown in Supplementary Table S2, Decellularized Extracellular Matrix, In Vitro Models, Personalized Medicine, Gelatin Methacryloyl, and Wound Healing are the top five latest author keywords.
Based on the bibliometric analysis, we make the prediction that bioink, hydrogels, tissue engineering, decellularized extracellular matrix (DECM), in vitro models, personalized medicine, regenerative medicine, GelMA, cell viability and vascularization will be the hot trends of 3D bioprinting in the present and nearly future. The following is our further analysis.
4.7.1 Bio-ink and hydrogels
Bio-ink was defined as materials which are capable to include cells and other bioactive components for the use in biofabrication (Heid and Boccaccini, 2020). Bio-inks cannot be confused with biomaterial-inks, which have always been considered the materials used in biofabrication. Bio-ink is a material that must be first printed, sterilized, and seeded with cells to enable scaffolding components or generate hybrid supports to improve the mechanical resistance of 3D printed specimens (Groll et al. 2018). Bio-inks always consist of cells and scaffolding material before printing, meaning that the cells are already evenly distributed in the scaffold during the printing process (Tiwari et al. 2021). The bio-inks must have the ability: i) to act as a platform for cells to adhere, grow, spread and proliferate; ii) provide adequate structural support during and after printing; and iii) protect cells from printing stress damage (Chimene et al. 2020; Zhang et al. 2021b). To further analyse the recent progress in bio-ink, we conducted a search using “bioink” OR “bio-ink” OR “bioinks” OR “bio-inks” as the keywords in the WOS core collection database. We obtained 1810 related articles (original research and reviews) and extracted all author keywords, then screened for all keywords related to bio-ink materials. Based on the analysis, we constructed a time-based co-occurrence network of the keywords (Supplementary Figure S2). As shown in Supplementary Figure S2, there have been significant advancements in bio-inks materials in recent years, with more and more materials being discovered for use in bioprinting. Before 2015, only a few types of bioinks were available for 3D bioprinting, including combined biopolymers and composite materials (Pataky et al. 2012; Malda et al. 2013; Wüst et al. 2014; Das et al. 2015; Kesti et al. 2015), fibrin (Cui and Boland, 2009; Cubo et al. 2016), oxidized alginate (Jia et al. 2014), and spherical cell aggregates (Jakab et al. 2004; Levato et al. 2014). From 2015 to 2018, a large number of new materials that can be used as bioinks have emerged. These include, but are not limited to, alginate (Axpe and Oyen, 2016), hyaluronic acid (Lee et al. 2018; Petta et al. 2018; Weis et al. 2018), collagen (Diamantides et al. 2017; Włodarczyk-Biegun and Del Campo, 2017), decellularized extracellular matrix (dECM) (Pati et al. 2014; Kim et al. 2017; Choudhury et al. 2018; Nam and Park, 2018), gelatin methacryloyl (GelMA) (Yue et al. 2015; Van Hoorick et al. 2019), silk fibroin (Chawla et al. 2018), spider silk (DeSimone et al. 2017) and agarose (Duarte Campos et al. 2015; Forget et al. 2017; López-Marcial et al. 2018). Besides, it is worth noting that gelatin is mostly used in combination with other materials in bioinks, and its main function is to maintain the shape of the printing scaffold before ink crosslinking. After 2018, more materials have been discovered to be suitable for use as bio-inks., including chitosan (Kołodziejska et al. 2021; Xu J. et al. 2022; Lazaridou et al. 2022), gellan gum (Cernencu and Ioniță, 2023), hydroxyapatite (Heid and Boccaccini, 2020), pectin (Mahendiran et al. 2021; Merli et al. 2022), cellulose (Ahlfeld et al. 2020; Zennifer et al. 2021) and polysaccharides (Naranda et al. 2021; Teixeira et al. 2022). Meanwhile, we also noticed that dECM, GelMA, and alginate have received more attention recently. In addition, more studies are combining two or three existing bio-inks to obtain a better bio-ink through complementary advantages and disadvantages, which is also one of the hot research directions in this field.
Based on the material requirements, hydrogels are gradually being used in bio-inks. Hydrogel biomaterials include alginate, gelatin, collagen, fibrin/fibrinogen, gellan gum, hyaluronic acid, agarose, chitosan, silk, decellularized extracellular matrix (dECM), poly (ethylene glycol), and Pluronic. Hydrogels have many attractive features for use as bio-inks. As they are biocompatible and typically biodegradable, most of them are easy for cells to adhere, grow, spread and proliferate on (Gungor-Ozkerim et al. 2018). Furthermore, different cross-linking methods enable hydrogels to be applied to various cells and tissues (Basu et al. 2016; Hospodiuk et al. 2017; Paxton et al. 2017; Vázquez-González and Willner, 2020; Xu et al. 2020). Although the abundance of hydrogels offers great potential for tissue engineering, their applications in 3D bioprinting are still limited due to the lack of bioprinting capabilities (Hospodiuk et al. 2017). Hydrogels do not contain specific proteins from the extracellular matrix of cells, so it is hard to simulate the “native environments” for specific cells. Furthermore, the physical properties of hydrogels may affect the normal biological function of cells. Then, the degradation of hydrogels and strength of scaffold should also be noticed (Hospodiuk et al. 2017; Osidak et al. 2020; Petta et al. 2020). Not surprisingly, bio-inks and hydrogels have been the most studied topic in 3D bioprinting. With the development of regenerative medicine and tissue engineering, research on new hydrogel materials with better material properties might become a research hotspot in the future.
4.7.2 Tissue engineering
Tissue engineering is one of the hottest research fields in recent years, and it has been widely applied to musculoskeletal tissue, oral tissue, cardiovascular tissue, urogenital tissue, ocular tissue, and so on (Gupta and Bit, 2022). Based on its important role in 3D bioprinting, we conducted a brief bibliometric analysis of tissue engineering research in the past 4 years. A total of 30,738 documents (article and review) and 39,849 author keywords were analyzed, and Frontiers in Bioengineering and Biotechnology has published the most research (785 articles). As shown in Supplementary Figure S3, we obtained the top 60 author keywords in tissue engineering research over the past 4 years and they were further analyzed by VOSviewer to create the density visualization map (Supplementary Figure S3A) and all the sixty keywords were subjected to cluster analysis, where the size of the node representing each keyword corresponds to its frequency of occurrence (Supplementary Figure S3B). We found that bone tissue engineering and cartilage tissue engineering were the most researched directions. Meanwhile, 3D bioprinting is also one of the hot research directions in tissue engineering, which is consistent with our previous analysis. From this, we can make further inference that the application of 3D bioprinting technology in bone and cartilage tissue engineering will continue to be a research hotspot in these two fields for some time to come, and technological advancements will bring more advantageous solutions for the repair of bone and cartilage injuries.
4.7.3 Extrusion and bioprinting
There are four major 3D bioprinting methods, namely, extrusion, inkjet, stereolithography, and laser-assisted printing (Gillispie et al. 2020). EBB extrudes or dispenses continuous strands or fibers of biomaterials to form 3D scaffold structures, and is the first of the demonstrated bioprinting modalities and the most widely used 3D bioprinting technology (Landers et al. 2002; Pati et al. 2015; Zhang et al. 2021c). EBB has plenty of advantages compared to other 3D printing methods: 1) a variety of biomaterials and cell types can be used (Pati et al. 2014); 2) the scaffold can be constructed layer by layer with appropriate physiological cell density according to needs (Mironov et al. 2009a); 3) it is less damaging to cells in the construction process (Skoog et al. 2014; Ozbolat and Hospodiuk, 2016); 4) has great potential for stem cell growth, differentiation, and function (Chen, 2019). However, EBB still has some limitations. First, the commonly used bio-inks are limited by the inability to obtain high cell densities comparable to those found in native tissues (Zhang et al. 2021c), while scaffold-free multicellular spheroids with extrusion bioprinting and single-cell-only bioprinting techniques may be able to solve this problem (Mironov et al. 2009b; Norotte et al. 2009; Yu et al. 2016; Jeon et al. 2019). Second, as the resolution and throughput limits the development of EBB technology, one has to sacrifice one of these two contradicting functional parameters. We believe this problem can be solved by microfluidics technology, airflow motor control, and a microvascular multi-nozzle printhead (Hansen et al. 2013; du Chatinier et al. 2021; Tang G. et al. 2021). Therefore, we infer that developing 3D bioprinting methods and technologies with more advantages may become one of the hot trends in future research.
4.7.4 DECM
DECM scaffold refers to biomaterials formed by human or animal organs/tissues with the removal of immunogenic cellular components using decellularized technologies (Hinderer et al. 2016). A dECM scaffold is mainly composed of extracellular matrix, which contains collagen, elastin, fibronectin, laminin, and matricellular proteins (Chen and Liu, 2016; Zhang et al. 2022). As dECM scaffolds maintain the physicochemical signals and biological performance after decellularization as well as provide mechanical support, they have been widely used in tissue engineering (Hoshiba, 2019; Kim et al. 2020). Despite this fact, not all dECM are suitable for 3D bioprinting; bioprintability, cell viability, mechanical and structural properties, as well as remodeling capability must be considered (Kim et al. 2020). The dECM still has lots of limitations, such as immunogenic properties, pro-remodeling response in host tissue, cellular cytoxicity from UV light and chemical agents in the process of cross-linking, and the integration of the cell-printed constructs in the body (Yeo et al. 2007; Wang et al. 2017; Schmitt et al. 2021). In general, the dECM scaffold has great prospects in 3D bioprinting, but several problems remain to be solved before practical application in the future.
4.7.5 In Vitro Models
In vitro models are important tools to study the occurrence and development of diseases (Kaur et al. 2022). Many disease models have been created using 3D bioprinting, such as tumor models (Neufeld et al. 2022), osteochondral unit models (Santos-Beato et al. 2022), multicellular cardiac fibrosis models (Picchio et al. 2022), as well as liver (Guagliano et al. 2022) and skin models (Phang et al. 2022). In recent years, organoid technology has rapidly developed as an in vitro model. Organoids are derived from pluripotent stem cells or isolated organ progenitors that differentiate to form an organ-like tissue exhibiting multiple cell types that self-organize to form a structure not unlike the organ in vivo (Lancaster and Knoblich, 2014; Rossi et al. 2018). Organoid technology is further developed because of the 3D bioprinting technology, allowing the distribution of cells in organoids to be adjusted as needed and better organ simulation (Chakraborty et al. 2022). Therefore, we believe that the combinatorial perspectives of tissue engineering, organoids, and 3D bioprinting are promising.
4.7.6 GelMA
GelMA is a hydrogel synthesized by the chemical reaction between the hydroxyl and amine groups of the amino acid residues and methacrylic anhydride (Van Den Bulcke et al. 2000). As GelMA exhibits great compatibility with various cells, biodegradability, and accessibility (Loessner et al. 2016), it is widely used in hard and soft tissue engineering (Li et al. 2018; Chimene et al. 2020; Unagolla and Jayasuriya, 2020; Rajabi et al. 2021). Besides, due to its similarity to the main component in the extracellular matrix, GelMA has been used in drug and gene delivery, as well as in wound healing applications (Yue et al. 2015; Ayoub et al. 2022; Hölzl et al. 2022; Moniruzzaman et al. 2022; Tozar et al. 2022). As one of the best 3D bioprinting materials, GelMA still has some limitations, such as low viscosity at room or higher temperature and rapid degradation in body tissue (Seyedmahmoud et al. 2019; Rajabi et al. 2021). Nonetheless, GelMA has very good prospects in 3D bioprinting.
4.7.7 Personalized and regenerative medicine
3D bioprinting has played a significant role in personalized medicine, especially in the field of cancer research. In the treatment of tumors, the most appropriate treatment plan should be adopted for different patients, and 3D bioprinting technology can achieve personalized tumor organoid and simulate the tumor microenvironment to the greatest extent. Studying the sensitivity of drugs in this personalized organoid and designing the optimal treatment plan is the best solution pursued in clinical treatment, while also reducing the use of animal models (Jung et al. 2022). In addition, 3D bioprinting technology also has great potential in drug screening. Studying the efficacy of new drugs by constructing different tissue organoids and disease models is one of the hot research directions. Meanwhile, utilizing 3D bioprinting technology to construct personalized tissue organs and apply them in transplant medicine also can be one of the development directions for the future of personalized medicine (Ma et al. 2018).
Regenerative medicine is an important discipline focused on the regeneration of complex tissue and organ systems (Tang et al. 2019). Currently, 3D bioprinting has achieved results in the regenerative medicine research of various organs, including skin tissue, heart tissue, bone tissue, cartilage tissue, liver tissue, lung tissue, nervous tissue, and pancreatic tissue, among others (Matai et al. 2020). However, so far, 3D bioprinting technology still has many limitations in the field of regenerative medicine, including the insolubility, stability, and promotion of cell growth of bioinks (Ravnic et al. 2017). Therefore, the application of 3D bioprinting technology in the field of regenerative medicine also might be a hot research direction for the future.
4.7.8 Cell viability and vascularization
As cells are subjected to various types of pressure during the 3D bioprinting process, cell viability is one of the most challenging issues (Kačarević et al. 2018; Xu H-Q. et al. 2022). The pressure generated during the 3D bioprinting process can affect cell signaling pathways and protein expression, which further impacts cell viability. Current methods mainly involve controlling pressure changes during the printing process, improving the shape and performance of the nozzle, adjusting overall parameters, and optimizing bioinks, among other techniques (Chang et al. 2008; Busch et al. 2015; Boularaoui et al. 2020). Therefore, improving cell viability as much as possible during the 3D bioprinting process is an urgent and important issue that needs to be addressed in this field of research in the near future.
Studies have shown that engineered tissues with a thickness greater than 1 mm have difficulty maintaining their normal cell viability without vascularization (Visconti et al. 2010; Laschke and Menger, 2012). Therefore, achieving vascularization in 3D bioprinting is crucial. Although there are currently several methods available for vascularization, including improved printing techniques, the use of bioinks that promote vascularization, and others, there is still a significant gap compared to normal tissue (Kong and Wang, 2023). Therefore, we believe that developing improved 3D bioprinting techniques for vascularization may be one of the future hotspots.
4.8 Limitations
All the bibliometric analysis articles have several inherent limitations and this study is no exception. First, all the data in this study were extracted from the WoSCC only. Although this database has been widely used in bibliometrics, papers only published in other databases were excluded from this study. Then, part of the data was analysed using software based on the strength of machine learning, which may lead to bias in data analysis. Additionally, Due to the time required for the publication of an article, new relevant literature may be published during this period, this is an unavoidable issue in current bibliometric research. Nonetheless, our research still provides a comprehensive overview of different directions in the field of 3D bioprinting and offers researchers more objective data, knowledge, and insight.
5 Conclusion
Using information visualization technology, this study fully summarizes the research progress, hotspots, and frontiers in the 3D bioprinting field. Exciting findings can provide a foundation for further research in this field and inspire the collaboration among potential partners and institutions. Research on 3D bioprinting has greatly developed and still has huge prospect in the future. The United States and China have absolute advantages in this field, and interested researchers may find cooperation opportunities in Harvard Medical School, Tsinghua University, Chinese Academy of Sciences, University of California-Los Angeles, and Wake Forest School of Medicine. Tissue Engineering Part A published the most articles in this field, and Dr. Anthony Atala was the most productive author. The academic exchange and cooperation between countries, institutions, and authors has promoted the development of this research field. Bio-inks and scaffolds have become a hotspot recently. The development of different hydrogels (especially GelMA) and dECM is expected to become an attractive direction in the next years. In addition, further studies on the modification of the EBB technique are key to promoting the progress of 3D bioprinting. Notably, the application of 3D bioprinting, especially in tissue engineering and in vitro models (organoids in particular), is the research Frontier in this field, and it is currently in an explosive period. Last but not least, the application of 3D bioprinting in personalized and regenerative medicine will continue to develop in the future, but the important issues of cell viability and vascularization need to be addressed first.
Data availability statement
The original contributions presented in the study are included in the article/Supplementary Material, further inquiries can be directed to the corresponding authors.
Author contributions
ZD and SW conceived the idea for the study, provided a retrieval strategy. ZD and NT performed the analysis and wrote the first draft of the manuscript that was iteratively improved by JH, XC and SW. All authors contributed to the article and approved the submitted version.
Funding
This work was supported by Science and Technology Innovation Leading Plan of High-Tech Industry in Hunan Province (2020SK2011) and Medical Research Development Fund Project (WS865C).
Conflict of interest
The authors declare that the research was conducted in the absence of any commercial or financial relationships that could be construed as a potential conflict of interest.
Publisher’s note
All claims expressed in this article are solely those of the authors and do not necessarily represent those of their affiliated organizations, or those of the publisher, the editors and the reviewers. Any product that may be evaluated in this article, or claim that may be made by its manufacturer, is not guaranteed or endorsed by the publisher.
Supplementary material
The Supplementary Material for this article can be found online at: https://www.frontiersin.org/articles/10.3389/fbioe.2023.1169893/full#supplementary-material
References
Adib, A. A., Sheikhi, A., Shahhosseini, M., Simeunović, A., Wu, S., Castro, C. E., et al. (2020). Direct-write 3D printing and characterization of a GelMA-based biomaterial for intracorporeal tissue engineering. Biofabrication 12 (4), 045006. doi:10.1088/1758-5090/ab97a1
Ahlfeld, T., Guduric, V., Duin, S., Akkineni, A. R., Schütz, K., Kilian, D., et al. (2020). Methylcellulose - a versatile printing material that enables biofabrication of tissue equivalents with high shape fidelity. Biomaterials Sci. 8 (8), 2102–2110. doi:10.1039/d0bm00027b
Anthon, S. G., and Valente, K. P. (2022). Vascularization strategies in 3D cell culture models: From scaffold-free models to 3D bioprinting. Int. J. Mol. Sci. 23 (23), 14582. doi:10.3390/ijms232314582
Atala, A., Kasper, F. K., and Mikos, A. G. (2012). Engineering complex tissues. Sci. Transl. Med. 4 (160), 160rv12. doi:10.1126/scitranslmed.3004890
Axpe, E., and Oyen, M. L. (2016). Applications of alginate-based bioinks in 3D bioprinting. Int. J. Mol. Sci. 17 (12), 1976. doi:10.3390/ijms17121976
Ayoub, A. A., Mahmoud, A. H., Ribeiro, J. S., Daghrery, A., Xu, J., Fenno, J. C., et al. (2022). Electrospun azithromycin-laden gelatin methacryloyl fibers for endodontic infection control. Int. J. Mol. Sci. 23 (22), 13761. doi:10.3390/ijms232213761
Basu, A., Kunduru, K. R., Doppalapudi, S., Domb, A. J., and Khan, W. (2016). Poly (lactic acid) based hydrogels. Adv. Drug Deliv. Rev. 107, 192–205. doi:10.1016/j.addr.2016.07.004
Boularaoui, S., Al Hussein, G., Khan, K. A., Christoforou, N., and Stefanini, C. (2020). An overview of extrusion-based bioprinting with a focus on induced shear stress and its effect on cell viability. Bioprinting 20, e00093. doi:10.1016/j.bprint.2020.e00093
Busch, R., Strohbach, A., Pennewitz, M., Lorenz, F., Bahls, M., Busch, M. C., et al. (2015). Regulation of the endothelial apelin/APJ system by hemodynamic fluid flow. Cell. Signal. 27 (7), 1286–1296. doi:10.1016/j.cellsig.2015.03.011
Cernencu, A. I., and Ioniță, M. (2023). The current state of the art in gellan-based printing inks in tissue engineering. Carbohydr. Polym. 309, 120676. doi:10.1016/j.carbpol.2023.120676
Chakraborty, J., Chawla, S., and Ghosh, S. (2022). Developmental biology-inspired tissue engineering by combining organoids and 3D bioprinting. Curr. Opin. Biotechnol. 78, 102832. doi:10.1016/j.copbio.2022.102832
Chang, C. C., Boland, E. D., Williams, S. K., and Hoying, J. B. (2011). Direct-write bioprinting three-dimensional biohybrid systems for future regenerative therapies. J. Biomed. Mater. Res. Part B, Appl. Biomaterials 98 (1), 160–170. doi:10.1002/jbm.b.31831
Chang, R., Nam, J., and Sun, W. (2008). Effects of dispensing pressure and nozzle diameter on cell survival from solid freeform fabrication-based direct cell writing. Tissue Eng. Part A 14 (1), 41–48. doi:10.1089/ten.2007.0004
Chawla, S., Midha, S., Sharma, A., and Ghosh, S. (2018). Silk-based bioinks for 3D bioprinting. Adv. Healthc. Mater. 7 (8), e1701204. doi:10.1002/adhm.201701204
Chen, C., and Leydesdorff, L. (2014). Patterns of connections and movements in dual-map overlays: A new method of publication portfolio analysis. J. Assoc. Inf. Sci. Technol. 65 (2), 334–351. doi:10.1002/asi.22968
Chen, D. X. (2019). Extrusion bioprinting of scaffolds for tissue engineering applications. Berlin, Germany: Springer, 1–13.
Chen, F.-M., and Liu, X. (2016). Advancing biomaterials of human origin for tissue engineering. Prog. Polym. Sci. 53, 86–168. doi:10.1016/j.progpolymsci.2015.02.004
Cheng, K., Guo, Q., Yang, W., Wang, Y., Sun, Z., and Wu, H. (2022). Mapping knowledge landscapes and emerging trends of the links between bone metabolism and diabetes mellitus: A bibliometric analysis from 2000 to 2021. Front. Public Health 10, 918483. doi:10.3389/fpubh.2022.918483
Chimene, D., Kaunas, R., and Gaharwar, A. K. (2020). Hydrogel bioink reinforcement for additive manufacturing: A focused review of emerging strategies. Adv. Mater. 32 (1), 1902026. doi:10.1002/adma.201902026
Choudhury, D., Tun, H. W., Wang, T., and Naing, M. W. (2018). Organ-derived decellularized extracellular matrix: A game changer for bioink manufacturing? Trends Biotechnol. 36 (8), 787–805. doi:10.1016/j.tibtech.2018.03.003
Cubo, N., Garcia, M., Del Cañizo, J. F., Velasco, D., and Jorcano, J. L. (2016). 3D bioprinting of functional human skin: Production and in vivo analysis. Biofabrication 9 (1), 015006. doi:10.1088/1758-5090/9/1/015006
Cui, X., and Boland, T. (2009). Human microvasculature fabrication using thermal inkjet printing technology. Biomaterials 30 (31), 6221–6227. doi:10.1016/j.biomaterials.2009.07.056
Dababneh, A. B., and Ozbolat, I. T. (2014). Bioprinting technology: A current state-of-the-art review. J. Manuf. Sci. Eng. 136 (6), 061016. doi:10.1115/1.4028512
Das, S., Pati, F., Choi, Y.-J., Rijal, G., Shim, J.-H., Kim, S. W., et al. (2015). Bioprintable, cell-laden silk fibroin-gelatin hydrogel supporting multilineage differentiation of stem cells for fabrication of three-dimensional tissue constructs. Acta Biomater. 11, 233–246. doi:10.1016/j.actbio.2014.09.023
De Filippo, R. E., Kornitzer, B. S., Yoo, J. J., and Atala, A. (2015). Penile urethra replacement with autologous cell-seeded tubularized collagen matrices. J. Tissue Eng. Regen. Med. 9 (3), 257–264. doi:10.1002/term.1647
Derby, B. (2012). Printing and prototyping of tissues and scaffolds. science 338 (6109), 921–926. doi:10.1126/science.1226340
Desimone, E., Schacht, K., Pellert, A., and Scheibel, T. (2017). Recombinant spider silk-based bioinks. Biofabrication 9 (4), 044104. doi:10.1088/1758-5090/aa90db
Diamantides, N., Wang, L., Pruiksma, T., Siemiatkoski, J., Dugopolski, C., Shortkroff, S., et al. (2017). Correlating rheological properties and printability of collagen bioinks: The effects of riboflavin photocrosslinking and pH. Biofabrication 9 (3), 034102. doi:10.1088/1758-5090/aa780f
Ding, Z., Ren, Y., Cao, H., and Li, J. (2022). Top 100 most cited articles on anterior cervical discectomy and fusion. Front. Surg. 9, 1000360. doi:10.3389/fsurg.2022.1000360
Du Chatinier, D. N., Figler, K. P., Agrawal, P., Liu, W., and Zhang, Y. S. (2021). The potential of microfluidics-enhanced extrusion bioprinting. Biomicrofluidics 15 (4), 041304. doi:10.1063/5.0033280
Duan, B., Hockaday, L. A., Kang, K. H., and Butcher, J. T. (2013). 3D bioprinting of heterogeneous aortic valve conduits with alginate/gelatin hydrogels. J. Biomed. Mater. Res. Part A 101 (5), 1255–1264. doi:10.1002/jbm.a.34420
Duarte Campos, D. F., Blaeser, A., Korsten, A., Neuss, S., Jäkel, J., Vogt, M., et al. (2015). The stiffness and structure of three-dimensional printed hydrogels direct the differentiation of mesenchymal stromal cells toward adipogenic and osteogenic lineages. Tissue Eng. Part A 21 (3-4), 740–756. doi:10.1089/ten.tea.2014.0231
Erdem, A., Darabi, M. A., Nasiri, R., Sangabathuni, S., Ertas, Y. N., Alem, H., et al. (2020). 3D bioprinting of oxygenated cell-laden gelatin methacryloyl constructs. Adv. Healthc. Mater. 9 (15), e1901794. doi:10.1002/adhm.201901794
Fan, H., Demirci, U., and Chen, P. (2019). Emerging organoid models: Leaping forward in cancer research. J. Hematol. Oncol. 12 (1), 142–210. doi:10.1186/s13045-019-0832-4
Farzanegan, R., Feizabadi, M., Ghorbani, F., Movassaghi, M., Vaziri, E., Zangi, M., et al. (2017). An overview of tracheal stenosis research trends and hot topics. Archives Iran. Med. 20 (9), 598–607.
Feng, C., Zhou, X., Wang, H., He, Y., Li, Z., and Tu, C. (2022). Research hotspots and emerging trends of deep learning applications in orthopedics: A bibliometric and visualized study. Front. Public Health 10, 949366. doi:10.3389/fpubh.2022.949366
Forget, A., Blaeser, A., Miessmer, F., Köpf, M., Campos, D. F. D., Voelcker, N. H., et al. (2017). Mechanically tunable bioink for 3D bioprinting of human cells. Adv. Healthc. Mater. 6 (20), 1700255. doi:10.1002/adhm.201700255
Gao, Y., Shi, S., Ma, W., Chen, J., Cai, Y., Ge, L., et al. (2019). Bibliometric analysis of global research on PD-1 and PD-L1 in the field of cancer. Int. Immunopharmacol. 72, 374–384. doi:10.1016/j.intimp.2019.03.045
Gillispie, G., Prim, P., Copus, J., Fisher, J., Mikos, A. G., Yoo, J. J., et al. (2020). Assessment methodologies for extrusion-based bioink printability. Biofabrication 12 (2), 022003. doi:10.1088/1758-5090/ab6f0d
Groll, J., Burdick, J. A., Cho, D.-W., Derby, B., Gelinsky, M., Heilshorn, S. C., et al. (2018). A definition of bioinks and their distinction from biomaterial inks. Biofabrication 11 (1), 013001. doi:10.1088/1758-5090/aaec52
Guagliano, G., Volpini, C., Briatico-Vangosa, F., Cornaglia, A. I., Visai, L., and Petrini, P. (2022). Toward 3D-bioprinted models of the liver to boost drug development. Macromol. Biosci. 22, e2200264. doi:10.1002/mabi.202200264
Guillemot, F., Mironov, V., and Nakamura, M. (2010). Bioprinting is coming of age: Report from the international conference on bioprinting and biofabrication in bordeaux (3B'09). Biofabrication 2 (1), 010201. doi:10.1088/1758-5082/2/1/010201
Gungor-Ozkerim, P. S., Inci, I., Zhang, Y. S., Khademhosseini, A., and Dokmeci, M. R. (2018). Bioinks for 3D bioprinting: An overview. Biomaterials Sci. 6 (5), 915–946. doi:10.1039/c7bm00765e
Gupta, S., and Bit, A. (2022). 3D bioprinting in tissue engineering and regenerative medicine. Cell Tissue Bank. 23 (2), 199–212. doi:10.1007/s10561-021-09936-6
Hansen, C. J., Saksena, R., Kolesky, D. B., Vericella, J. J., Kranz, S. J., Muldowney, G. P., et al. (2013). High-throughput printing via microvascular multinozzle arrays. Adv. Mater. 25 (1), 96–102. doi:10.1002/adma.201203321
Heid, S., and Boccaccini, A. R. (2020). Advancing bioinks for 3D bioprinting using reactive fillers: A review. Acta Biomater. 113, 1–22. doi:10.1016/j.actbio.2020.06.040
Hinderer, S., Layland, S. L., and Schenke-Layland, K. (2016). ECM and ECM-like materials - biomaterials for applications in regenerative medicine and cancer therapy. Adv. Drug Deliv. Rev. 97, 260–269. doi:10.1016/j.addr.2015.11.019
Hinton, T. J., Jallerat, Q., Palchesko, R. N., Park, J. H., Grodzicki, M. S., Shue, H.-J., et al. (2015). Three-dimensional printing of complex biological structures by freeform reversible embedding of suspended hydrogels. Sci. Adv. 1 (9), e1500758. doi:10.1126/sciadv.1500758
Hölzl, K., Fürsatz, M., Göcerler, H., Schädl, B., Žigon-Branc, S., Markovic, M., et al. (2022). Gelatin methacryloyl as environment for chondrocytes and cell delivery to superficial cartilage defects. J. Tissue Eng. Regen. Med. 16 (2), 207–222. doi:10.1002/term.3273
Hölzl, K., Lin, S., Tytgat, L., Van Vlierberghe, S., Gu, L., and Ovsianikov, A. (2016). Bioink properties before, during and after 3D bioprinting. Biofabrication 8 (3), 032002. doi:10.1088/1758-5090/8/3/032002
Hoshiba, T. (2019). Decellularized extracellular matrix for cancer research. Materials 12 (8), 1311. doi:10.3390/ma12081311
Hospodiuk, M., Dey, M., Sosnoski, D., and Ozbolat, I. T. (2017). The bioink: A comprehensive review on bioprintable materials. Biotechnol. Adv. 35 (2), 217–239. doi:10.1016/j.biotechadv.2016.12.006
Huang, Y., Zhang, X.-F., Gao, G., Yonezawa, T., and Cui, X. (2017). 3D bioprinting and the current applications in tissue engineering. Biotechnol. J. 12 (8), 1600734. doi:10.1002/biot.201600734
Jain, P., Kathuria, H., and Dubey, N. (2022). Advances in 3D bioprinting of tissues/organs for regenerative medicine and in-vitro models. Biomaterials 287, 121639. doi:10.1016/j.biomaterials.2022.121639
Jakab, K., Neagu, A., Mironov, V., Markwald, R. R., and Forgacs, G. (2004). Engineering biological structures of prescribed shape using self-assembling multicellular systems. Proc. Natl. Acad. Sci. U. S. A. 101 (9), 2864–2869. doi:10.1073/pnas.0400164101
Jana, S., and Lerman, A. (2015). Bioprinting a cardiac valve. Biotechnol. Adv. 33 (8), 1503–1521. doi:10.1016/j.biotechadv.2015.07.006
Jeon, O., Lee, Y. B., Jeong, H., Lee, S. J., Wells, D., and Alsberg, E. (2019). Individual cell-only bioink and photocurable supporting medium for 3D printing and generation of engineered tissues with complex geometries. Mater. horizons 6 (8), 1625–1631. doi:10.1039/c9mh00375d
Jia, J., Richards, D. J., Pollard, S., Tan, Y., Rodriguez, J., Visconti, R. P., et al. (2014). Engineering alginate as bioink for bioprinting. Acta Biomater. 10 (10), 4323–4331. doi:10.1016/j.actbio.2014.06.034
Jia, W., Gungor-Ozkerim, P. S., Zhang, Y. S., Yue, K., Zhu, K., Liu, W., et al. (2016). Direct 3D bioprinting of perfusable vascular constructs using a blend bioink. Biomaterials 106, 58–68. doi:10.1016/j.biomaterials.2016.07.038
Joddar, B., Natividad-Diaz, S. L., Padilla, A. E., Esparza, A. A., Ramirez, S. P., Chambers, D. R., et al. (2022). Engineering approaches for cardiac organoid formation and their characterization. Transl. Res. 250, 46–67. doi:10.1016/j.trsl.2022.08.009
Jorgensen, A. M., Varkey, M., Gorkun, A., Clouse, C., Xu, L., Chou, Z., et al. (2020). Bioprinted skin recapitulates normal collagen remodeling in full-thickness wounds. Tissue Eng. Part A 26 (9-10), 512–526. doi:10.1089/ten.tea.2019.0319
Jubelin, C., Muñoz-Garcia, J., Griscom, L., Cochonneau, D., Ollivier, E., Heymann, M.-F., et al. (2022). Three-dimensional in vitro culture models in oncology research. Cell and Biosci. 12 (1), 155–228. doi:10.1186/s13578-022-00887-3
Jung, M., Ghamrawi, S., Du, E. Y., Gooding, J. J., and Kavallaris, M. (2022). Advances in 3D bioprinting for cancer biology and precision medicine: From matrix design to application. Adv. Healthc. Mater. 11 (24), e2200690. doi:10.1002/adhm.202200690
Kačarević Ž, P., Rider, P. M., Alkildani, S., Retnasingh, S., Smeets, R., Jung, O., et al. (2018). An introduction to 3D bioprinting: Possibilities, challenges and future aspects. Mater. (Basel, Switz. 11 (11), 2199. doi:10.3390/ma11112199
Kang, H.-W., Lee, S. J., Ko, I. K., Kengla, C., Yoo, J. J., and Atala, A. (2016). A 3D bioprinting system to produce human-scale tissue constructs with structural integrity. Nat. Biotechnol. 34 (3), 312–319. doi:10.1038/nbt.3413
Kaur, S., Kidambi, S., Ortega-Ribera, M., Thanh Thuy, L. T., Nieto, N., Cogger, V. C., et al. (2022). In vitro models for the study of liver biology and diseases - advances and limitations. Cell Mol. Gastroenterol. Hepatol. 15, 559–571. doi:10.1016/j.jcmgh.2022.11.0
Kesti, M., Müller, M., Becher, J., Schnabelrauch, M., D'este, M., Eglin, D., et al. (2015). A versatile bioink for three-dimensional printing of cellular scaffolds based on thermally and photo-triggered tandem gelation. Acta Biomater. 11, 162–172. doi:10.1016/j.actbio.2014.09.033
Kim, B. S., Das, S., Jang, J., and Cho, D.-W. (2020). Decellularized extracellular matrix-based bioinks for engineering tissue- and organ-specific microenvironments. Chem. Rev. 120 (19), 10608–10661. doi:10.1021/acs.chemrev.9b00808
Kim, B. S., Kim, H., Gao, G., Jang, J., and Cho, D.-W. (2017). Decellularized extracellular matrix: A step towards the next generation source for bioink manufacturing. Biofabrication 9 (3), 034104. doi:10.1088/1758-5090/aa7e98
Kolesky, D. B., Homan, K. A., Skylar-Scott, M. A., and Lewis, J. A. (2016). Three-dimensional bioprinting of thick vascularized tissues. Proc. Natl. Acad. Sci. U. S. A. 113 (12), 3179–3184. doi:10.1073/pnas.1521342113
Kolesky, D. B., Truby, R. L., Gladman, A. S., Busbee, T. A., Homan, K. A., and Lewis, J. A. (2014). 3D bioprinting of vascularized, heterogeneous cell-laden tissue constructs. Adv. Mater. Deerf. Beach, Fla.) 26 (19), 3124–3130. doi:10.1002/adma.201305506
Kołodziejska, M., Jankowska, K., Klak, M., and Wszoła, M. (2021). Chitosan as an underrated polymer in modern tissue engineering. Nanomater. (Basel, Switz. 11 (11), 3019. doi:10.3390/nano11113019
Kong, Z., and Wang, X. (2023). Bioprinting technologies and bioinks for vascular model establishment. Int. J. Mol. Sci. 24 (1), 891. doi:10.3390/ijms24010891
Lancaster, M. A., and Knoblich, J. A. (2014). Organogenesis in a dish: Modeling development and disease using organoid technologies. science 345 (6194), 1247125. doi:10.1126/science.1247125
Landers, R., Hübner, U., Schmelzeisen, R., and Mülhaupt, R. (2002). Rapid prototyping of scaffolds derived from thermoreversible hydrogels and tailored for applications in tissue engineering. Biomaterials 23 (23), 4437–4447. doi:10.1016/s0142-9612(02)00139-4
Laschke, M. W., and Menger, M. D. (2012). Vascularization in tissue engineering: Angiogenesis versus inosculation. Rech. Chir. Eur. 48 (2), 85–92. doi:10.1159/000336876
Lazaridou, M., Bikiaris, D. N., and Lamprou, D. A. (2022). 3D bioprinted chitosan-based hydrogel scaffolds in tissue engineering and localised drug delivery. Pharmaceutics 14 (9), 1978. doi:10.3390/pharmaceutics14091978
Lee, A., Hudson, A. R., Shiwarski, D. J., Tashman, J. W., Hinton, T. J., Yerneni, S., et al. (2019). 3D bioprinting of collagen to rebuild components of the human heart. Sci. (New York, N.Y.) 365 (6452), 482–487. doi:10.1126/science.aav9051
Lee, J., Lee, S.-H., Kim, B. S., Cho, Y.-S., and Park, Y. (2018). Development and evaluation of hyaluronic acid-based hybrid bio-ink for tissue regeneration. Tissue Eng. Regen. Med. 15 (6), 761–769. doi:10.1007/s13770-018-0144-8
Levato, R., Visser, J., Planell, J. A., Engel, E., Malda, J., and Mateos-Timoneda, M. A. (2014). Biofabrication of tissue constructs by 3D bioprinting of cell-laden microcarriers. Biofabrication 6 (3), 035020. doi:10.1088/1758-5082/6/3/035020
Li, H., Tan, C., and Li, L. (2018). Review of 3D printable hydrogels and constructs. Mater. Des. 159, 20–38. doi:10.1016/j.matdes.2018.08.023
Liang, Q., Ma, Y., Yao, X., and Wei, W. (2022). Advanced 3D-printing bioinks for articular cartilage repair. Int. J. Bioprinting 8 (3), 511. doi:10.18063/ijb.v8i3.511
Liu, M., Yang, F., and Xu, Y. (2022). Global trends of stem cell precision medicine research (2018-2022): A bibliometric analysis. Front. Surg. 9, 888956. doi:10.3389/fsurg.2022.888956
Loessner, D., Meinert, C., Kaemmerer, E., Martine, L. C., Yue, K., Levett, P. A., et al. (2016). Functionalization, preparation and use of cell-laden gelatin methacryloyl-based hydrogels as modular tissue culture platforms. Nat. Protoc. 11 (4), 727–746. doi:10.1038/nprot.2016.037
López-Marcial, G. R., Zeng, A. Y., Osuna, C., Dennis, J., García, J. M., and O'connell, G. D. (2018). Agarose-based hydrogels as suitable bioprinting materials for tissue engineering. ACS Biomaterials Sci. Eng. 4 (10), 3610–3616. doi:10.1021/acsbiomaterials.8b00903
Luo, Y., Xu, X., Ye, Z., Xu, Q., Li, J., Liu, N., et al. (2022). 3D bioprinted mesenchymal stromal cells in skin wound repair. Front. Surg. 9, 988843. doi:10.3389/fsurg.2022.988843
Ma, X., Liu, J., Zhu, W., Tang, M., Lawrence, N., Yu, C., et al. (2018). 3D bioprinting of functional tissue models for personalized drug screening and in vitro disease modeling. Adv. Drug Deliv. Rev. 132, 235–251. doi:10.1016/j.addr.2018.06.011
Mahendiran, B., Muthusamy, S., Sampath, S., Jaisankar, S. N., Popat, K. C., Selvakumar, R., et al. (2021). Recent trends in natural polysaccharide based bioinks for multiscale 3D printing in tissue regeneration: A review. Int. J. Biol. Macromol. 183, 564–588. doi:10.1016/j.ijbiomac.2021.04.179
Malda, J., Visser, J., Melchels, F. P., Jüngst, T., Hennink, W. E., Dhert, W. J. A., et al. (2013). 25th anniversary article: Engineering hydrogels for biofabrication. Adv. Mater. Deerf. Beach, Fla.) 25 (36), 5011–5028. doi:10.1002/adma.201302042
Mandrycky, C., Wang, Z., Kim, K., and Kim, D.-H. (2016). 3D bioprinting for engineering complex tissues. Biotechnol. Adv. 34 (4), 422–434. doi:10.1016/j.biotechadv.2015.12.011
Matai, I., Kaur, G., Seyedsalehi, A., Mcclinton, A., and Laurencin, C. T. (2020). Progress in 3D bioprinting technology for tissue/organ regenerative engineering. Biomaterials 226, 119536. doi:10.1016/j.biomaterials.2019.119536
Merli, M., Sardelli, L., Baranzini, N., Grimaldi, A., Jacchetti, E., Raimondi, M. T., et al. (2022). Pectin-based bioinks for 3D models of neural tissue produced by a pH-controlled kinetics. Front. Bioeng. Biotechnol. 10, 1032542. doi:10.3389/fbioe.2022.1032542
Miller, J. S., Stevens, K. R., Yang, M. T., Baker, B. M., Nguyen, D.-H. T., Cohen, D. M., et al. (2012). Rapid casting of patterned vascular networks for perfusable engineered three-dimensional tissues. Nat. Mater. 11 (9), 768–774. doi:10.1038/nmat3357
Mironov, V., Kasyanov, V., and Markwald, R. R. (2011). Organ printing: From bioprinter to organ biofabrication line. Curr. Opin. Biotechnol. 22 (5), 667–673. doi:10.1016/j.copbio.2011.02.006
Mironov, V., Trusk, T., Kasyanov, V., Little, S., Swaja, R., and Markwald, R. (2009a). Biofabrication: A 21st century manufacturing paradigm. Biofabrication 1 (2), 022001. doi:10.1088/1758-5082/1/2/022001
Mironov, V., Visconti, R. P., Kasyanov, V., Forgacs, G., Drake, C. J., and Markwald, R. R. (2009b). Organ printing: Tissue spheroids as building blocks. Biomaterials 30 (12), 2164–2174. doi:10.1016/j.biomaterials.2008.12.084
Moniruzzaman, M., Dutta, S. D., Hexiu, J., Ganguly, K., Lim, K.-T., and Kim, J. (2022). Polyphenol derived bioactive carbon quantum dot-incorporated multifunctional hydrogels as an oxidative stress attenuator for antiaging and in vivo wound-healing applications. Biomaterials Sci. 10 (13), 3527–3539. doi:10.1039/d2bm00424k
Murab, S., Gupta, A., Włodarczyk-Biegun, M. K., Kumar, A., Van Rijn, P., Whitlock, P., et al. (2022). Alginate based hydrogel inks for 3D bioprinting of engineered orthopedic tissues. Carbohydr. Polym. 296, 119964. doi:10.1016/j.carbpol.2022.119964
Murphy, S. V., and Atala, A. (2014). 3D bioprinting of tissues and organs. Nat. Biotechnol. 32 (8), 773–785. doi:10.1038/nbt.2958
Murphy, S. V., De Coppi, P., and Atala, A. (2020). Opportunities and challenges of translational 3D bioprinting. Nat. Biomed. Eng. 4 (4), 370–380. doi:10.1038/s41551-019-0471-7
Nam, S. Y., and Park, S.-H. (2018). ECM based bioink for tissue mimetic 3D bioprinting. Adv. Exp. Med. Biol. 1064, 335–353. doi:10.1007/978-981-13-0445-3_20
Naranda, J., Bračič, M., Vogrin, M., and Maver, U. (2021). Recent advancements in 3D printing of polysaccharide hydrogels in cartilage tissue engineering. Mater. (Basel, Switz. 14 (14), 3977. doi:10.3390/ma14143977
Naveau, A., Smirani, R., Catros, S., De Oliveira, H., Fricain, J.-C., and Devillard, R. (2017). A bibliometric study to assess bioprinting evolution. Appl. Sci. 7 (12), 1331. doi:10.3390/app7121331
Neufeld, L., Yeini, E., Pozzi, S., and Satchi-Fainaro, R. (2022). 3D bioprinted cancer models: From basic biology to drug development. Nat. Rev. Cancer 22 (12), 679–692. doi:10.1038/s41568-022-00514-w
Nishiyama, Y., Nakamura, M., Henmi, C., Yamaguchi, K., Mochizuki, S., Nakagawa, H., et al. (2009). Development of a three-dimensional bioprinter: Construction of cell supporting structures using hydrogel and state-of-the-art inkjet technology. J. Biomechanical Eng. 131 (3), 035001. doi:10.1115/1.3002759
Noor, N., Shapira, A., Edri, R., Gal, I., Wertheim, L., and Dvir, T. (2019). 3D printing of personalized thick and perfusable cardiac patches and hearts. Adv. Sci. (Weinheim, Baden-Wurttemberg, Ger. 6 (11), 1900344. doi:10.1002/advs.201900344
Norotte, C., Marga, F. S., Niklason, L. E., and Forgacs, G. (2009). Scaffold-free vascular tissue engineering using bioprinting. Biomaterials 30 (30), 5910–5917. doi:10.1016/j.biomaterials.2009.06.034
Osidak, E. O., Kozhukhov, V. I., Osidak, M. S., and Domogatsky, S. P. (2020). Collagen as bioink for bioprinting: A comprehensive review. Int. J. Bioprinting 6 (3), 270. doi:10.18063/ijb.v6i3.270
Ozbolat, I. T. (2015). Bioprinting scale-up tissue and organ constructs for transplantation. Trends Biotechnol. 33 (7), 395–400. doi:10.1016/j.tibtech.2015.04.005
Ozbolat, I. T., and Hospodiuk, M. (2016). Current advances and future perspectives in extrusion-based bioprinting. Biomaterials 76, 321–343. doi:10.1016/j.biomaterials.2015.10.076
Ozbolat, I. T., and Yu, Y. (2013). Bioprinting toward organ fabrication: Challenges and future trends. IEEE Trans. Bio-medical Eng. 60 (3), 691–699. doi:10.1109/tbme.2013.2243912
Panda, S., Hajra, S., Mistewicz, K., Nowacki, B., -Na, P., Krushynska, A. O., et al. (2022). A focused review on three-dimensional bioprinting technology for artificial organ fabrication. Biomaterials Sci. 10 (18), 5054–5080. doi:10.1039/d2bm00797e
Pataky, K., Braschler, T., Negro, A., Renaud, P., Lutolf, M. P., and Brugger, J. (2012). Microdrop printing of hydrogel bioinks into 3D tissue-like geometries. Adv. Mater. Deerf. Beach, Fla.) 24 (3), 391–396. doi:10.1002/adma.201102800
Pati, F., Jang, J., Ha, D.-H., Won Kim, S., Rhie, J.-W., Shim, J.-H., et al. (2014). Printing three-dimensional tissue analogues with decellularized extracellular matrix bioink. Nat. Commun. 5, 3935. doi:10.1038/ncomms4935
Pati, F., Jang, J., Lee, J. W., and Cho, D.-W. (2015). Essentials of 3D biofabrication and translation. Amsterdam, Netherlands: Elsevier, 123–152.
Paxton, N., Smolan, W., Böck, T., Melchels, F., Groll, J., and Jungst, T. (2017). Proposal to assess printability of bioinks for extrusion-based bioprinting and evaluation of rheological properties governing bioprintability. Biofabrication 9 (4), 044107. doi:10.1088/1758-5090/aa8dd8
Petta, D., Armiento, A. R., Grijpma, D., Alini, M., Eglin, D., and D'este, M. (2018). 3D bioprinting of a hyaluronan bioink through enzymatic-and visible light-crosslinking. Biofabrication 10 (4), 044104. doi:10.1088/1758-5090/aadf58
Petta, D., D'amora, U., Ambrosio, L., Grijpma, D. W., Eglin, D., and D'este, M. (2020). Hyaluronic acid as a bioink for extrusion-based 3D printing. Biofabrication 12 (3), 032001. doi:10.1088/1758-5090/ab8752
Phang, S. J., Basak, S., Teh, H. X., Packirisamy, G., Fauzi, M. B., Kuppusamy, U. R., et al. (2022). Advancements in extracellular matrix-based biomaterials and biofabrication of 3D organotypic skin models. ACS Biomaterials Sci. Eng. 8 (8), 3220–3241. doi:10.1021/acsbiomaterials.2c00342
Picchio, V., Floris, E., Derevyanchuk, Y., Cozzolino, C., Messina, E., Pagano, F., et al. (2022). Multicellular 3D models for the study of cardiac fibrosis. Int. J. Mol. Sci. 23 (19), 11642. doi:10.3390/ijms231911642
Pourmasoumi, P., Moghaddam, A., Nemati Mahand, S., Heidari, F., Salehi Moghaddam, Z., Arjmand, M., et al. (2022). A review on the recent progress, opportunities, and challenges of 4D printing and bioprinting in regenerative medicine. J. Biomaterials Sci. Polym. Ed. 34, 108–146. doi:10.1080/09205063.2022.2110480
Qin, Y., Zhang, Q., and Liu, Y. (2020). Analysis of knowledge bases and research focuses of cerebral ischemia-reperfusion from the perspective of mapping knowledge domain. Brain Res. Bull. 156, 15–24. doi:10.1016/j.brainresbull.2019.12.004
Rajabi, N., Rezaei, A., Kharaziha, M., Bakhsheshi-Rad, H. R., Luo, H., Ramakrishna, S., et al. (2021). Recent advances on bioprinted gelatin methacrylate-based hydrogels for tissue repair. Tissue Eng. Part A 27 (11-12), 679–702. doi:10.1089/ten.tea.2020.0350
Ramadan, Q., and Zourob, M. (2020). 3D bioprinting at the frontier of regenerative medicine, pharmaceutical, and food industries. Front. Med. Technol. 2, 607648. doi:10.3389/fmedt.2020.607648
Ravnic, D. J., Leberfinger, A. N., Koduru, S. V., Hospodiuk, M., Moncal, K. K., Datta, P., et al. (2017). Transplantation of bioprinted tissues and organs: Technical and clinical challenges and future perspectives. Ann. Surg. 266 (1), 48–58. doi:10.1097/sla.0000000000002141
Romero, L., and Portillo-Salido, E. (2019). Trends in sigma-1 receptor research: A 25-year bibliometric analysis. Front. Pharmacol. 10, 564. doi:10.3389/fphar.2019.00564
Rossi, G., Manfrin, A., and Lutolf, M. P. (2018). Progress and potential in organoid research. Nat. Rev. Genet. 19 (11), 671–687. doi:10.1038/s41576-018-0051-9
Santisteban-Espejo, A., Campos, F., Martin-Piedra, L., Durand-Herrera, D., Moral-Munoz, J. A., Campos, A., et al. (2018). Global tissue engineering trends: A scientometric and evolutive study. Tissue Eng. Part A 24 (19-20), 1504–1517. doi:10.1089/ten.tea.2018.0007
Santoni, S., Gugliandolo, S. G., Sponchioni, M., Moscatelli, D., and Colosimo, B. M. (2022). 3D bioprinting: Current status and trends—a guide to the literature and industrial practice. Bio-Design Manuf. 5 (1), 14–42. doi:10.1007/s42242-021-00165-0
Santos-Beato, P., Midha, S., Pitsillides, A. A., Miller, A., Torii, R., and Kalaskar, D. M. (2022). Biofabrication of the osteochondral unit and its applications: Current and future directions for 3D bioprinting. J. Tissue Eng. 13, 204173142211334. doi:10.1177/20417314221133480
Schmitt, T., Kajave, N., Cai, H. H., Gu, L., Albanna, M., and Kishore, V. (2021). In vitro characterization of xeno-free clinically relevant human collagen and its applicability in cell-laden 3D bioprinting. J. Biomaterials Appl. 35 (8), 912–923. doi:10.1177/0885328220959162
Seyedmahmoud, R., Çelebi-Saltik, B., Barros, N., Nasiri, R., Banton, E., Shamloo, A., et al. (2019). Three-dimensional bioprinting of functional skeletal muscle tissue using GelatinMethacryloyl-alginate bioinks. Micromachines 10 (10), 679. doi:10.3390/mi10100679
Shakir, S., Hackett, T. L., and Mostaço-Guidolin, L. B. (2022). Bioengineering lungs: An overview of current methods, requirements, and challenges for constructing scaffolds. Front. Bioeng. Biotechnol. 10, 1011800. doi:10.3389/fbioe.2022.1011800
Shen, J., Shen, H., Ke, L., Chen, J., Dang, X., Liu, B., et al. (2022). Knowledge mapping of immunotherapy for hepatocellular carcinoma: A bibliometric study. Front. Immunol. 13, 815575. doi:10.3389/fimmu.2022.815575
Shi, S., Gao, Y., Liu, M., Bu, Y., Wu, J., Tian, J., et al. (2021). Top 100 most-cited articles on exosomes in the field of cancer: A bibliometric analysis and evidence mapping. Clin. Exp. Med. 21 (2), 181–194. doi:10.1007/s10238-020-00624-5
Skoog, S. A., Goering, P. L., and Narayan, R. J. (2014). Stereolithography in tissue engineering. J. Mater. Sci. Mater. Med. 25 (3), 845–856. doi:10.1007/s10856-013-5107-y
Sonaye, S. Y., Ertugral, E. G., Kothapalli, C. R., and Sikder, P. (2022). Extrusion 3D (bio) printing of alginate-gelatin-based composite scaffolds for skeletal muscle tissue engineering. Materials 15 (22), 7945. doi:10.3390/ma15227945
Sun, H.-L., Bai, W., Li, X.-H., Huang, H., Cui, X.-L., Cheung, T., et al. (2022). Schizophrenia and inflammation research: A bibliometric analysis. Front. Immunol. 13, 907851. doi:10.3389/fimmu.2022.907851
Tang, G., Chen, L., Lian, L., Li, F., Ravanbakhsh, H., Wang, M., et al. (2021a). Designable dual-power micromotors fabricated from a biocompatible gas-shearing strategy. Chem. Eng. J. 407, 127187. doi:10.1016/j.cej.2020.127187
Tang, N., Zhang, W., George, D. M., Su, Y., and Huang, T. (2021b). The top 100 most cited articles on anterior cruciate ligament reconstruction: A bibliometric analysis. Orthop. J. Sports Med. 9 (2), 232596712097637. doi:10.1177/2325967120976372
Tang, N., Zhang, W., George, D. M., Wei, C., Su, Y., and Huang, T. (2021c). The top 100 most-cited articles on arthroscopy: Most popular topic is rotator cuff rather than cartilage in the last 5 years. Arthrosc. J. Arthrosc. Relat. Surg. 37 (6), 1779–1797. doi:10.1016/j.arthro.2021.01.039
Tang, X., Daneshmandi, L., Awale, G., Nair, L. S., and Laurencin, C. T. (2019). Skeletal muscle regenerative engineering. Regen. Eng. Transl. Med. 5, 233–251. doi:10.1007/s40883-019-00102-9
Tasoglu, S., and Demirci, U. (2013). Bioprinting for stem cell research. Trends Biotechnol. 31 (1), 10–19. doi:10.1016/j.tibtech.2012.10.005
Teixeira, M. C., Lameirinhas, N. S., Carvalho, J. P. F., Silvestre, A. J. D., Vilela, C., and Freire, C. S. R. (2022). A guide to polysaccharide-based hydrogel bioinks for 3D bioprinting applications. Int. J. Mol. Sci. 23 (12), 6564. doi:10.3390/ijms23126564
Thangadurai, M., Ajith, A., Budharaju, H., Sethuraman, S., and Sundaramurthi, D. (2022). Advances in electrospinning and 3D bioprinting strategies to enhance functional regeneration of skeletal muscle tissue. Biomater. Adv. 142, 213135. doi:10.1016/j.bioadv.2022.213135
Tiwari, A. P., Thorat, N. D., Pricl, S., Patil, R. M., Rohiwal, S., and Townley, H. (2021). Bioink: A 3D-bioprinting tool for anticancer drug discovery and cancer management. Drug Discov. Today 26 (7), 1574–1590. doi:10.1016/j.drudis.2021.03.010
Tozar, T., Nistorescu, S., Boni, M., Gradisteanu Pircalabioru, G., Negut, I., and Staicu, A. (2022). Pulsed laser photo-crosslinking of gelatin methacryloyl hydrogels for the controlled delivery of chlorpromazine to combat antimicrobial resistance. Pharmaceutics 14 (10), 2121. doi:10.3390/pharmaceutics14102121
Unagolla, J. M., and Jayasuriya, A. C. (2020). Hydrogel-based 3D bioprinting: A comprehensive review on cell-laden hydrogels, bioink formulations, and future perspectives. Appl. Mater. Today 18, 100479. doi:10.1016/j.apmt.2019.100479
Van Den Bulcke, A. I., Bogdanov, B., De Rooze, N., Schacht, E. H., Cornelissen, M., and Berghmans, H. (2000). Structural and rheological properties of methacrylamide modified gelatin hydrogels. Biomacromolecules 1 (1), 31–38. doi:10.1021/bm990017d
Van Hoorick, J., Tytgat, L., Dobos, A., Ottevaere, H., Van Erps, J., Thienpont, H., et al. (2019). (Photo-)crosslinkable gelatin derivatives for biofabrication applications. Acta Biomater. 97, 46–73. doi:10.1016/j.actbio.2019.07.035
Vázquez-González, M., and Willner, I. (2020). Stimuli-responsive biomolecule-based hydrogels and their applications. Angew. Chem. Int. Ed. 59 (36), 15342–15377. doi:10.1002/anie.201907670
Visconti, R. P., Kasyanov, V., Gentile, C., Zhang, J., Markwald, R. R., and Mironov, V. (2010). Towards organ printing: Engineering an intra-organ branched vascular tree. Expert Opin. Biol. Ther. 10 (3), 409–420. doi:10.1517/14712590903563352
Wang, H., Fu, H., Fu, Y., Jiang, L., Wang, L., Tong, H., et al. (2022a). Knowledge mapping concerning applications of nanocomposite hydrogels for drug delivery: A bibliometric and visualized study (2003-2022). Front. Bioeng. Biotechnol. 10, 1099616. doi:10.3389/fbioe.2022.1099616
Wang, J., Chi, Y., Yang, B., Zhang, Q., Wang, D., He, X., et al. (2022b). The application of biomaterials in osteogenesis: A bibliometric and visualized analysis. Front. Bioeng. Biotechnol. 10, 998257. doi:10.3389/fbioe.2022.998257
Wang, R. M., Johnson, T. D., He, J., Rong, Z., Wong, M., Nigam, V., et al. (2017). Humanized mouse model for assessing the human immune response to xenogeneic and allogeneic decellularized biomaterials. Biomaterials 129, 98–110. doi:10.1016/j.biomaterials.2017.03.016
Wang, Z., Lee, S. J., Cheng, H.-J., Yoo, J. J., and Atala, A. (2018). 3D bioprinted functional and contractile cardiac tissue constructs. Acta Biomater. 70, 48–56. doi:10.1016/j.actbio.2018.02.007
Wang, Z., Xiang, L., Lin, F., Tang, Y., and Cui, W. (2023). 3D bioprinting of emulating homeostasis regulation for regenerative medicine applications. J. Control. Release 353, 147–165. doi:10.1016/j.jconrel.2022.11.035
Weis, M., Shan, J., Kuhlmann, M., Jungst, T., Tessmar, J., and Groll, J. (2018). Evaluation of hydrogels based on oxidized hyaluronic acid for bioprinting. Gels (Basel, Switz. 4 (4), 82. doi:10.3390/gels4040082
Włodarczyk-Biegun, M. K., and Del Campo, A. (2017). 3D bioprinting of structural proteins. Biomaterials 134, 180–201. doi:10.1016/j.biomaterials.2017.04.019
Wüst, S., Godla, M. E., Müller, R., and Hofmann, S. (2014). Tunable hydrogel composite with two-step processing in combination with innovative hardware upgrade for cell-based three-dimensional bioprinting. Acta Biomater. 10 (2), 630–640. doi:10.1016/j.actbio.2013.10.016
Xiao, F., Li, C., Sun, J., and Zhang, L. (2017). Knowledge domain and emerging trends in organic photovoltaic technology: A scientometric review based on CiteSpace analysis. Front. Chem. 5, 67. doi:10.3389/fchem.2017.00067
Xu, H.-Q., Liu, J.-C., Zhang, Z.-Y., and Xu, C.-X. (2022a). A review on cell damage, viability, and functionality during 3D bioprinting. Mil. Med. Res. 9 (1), 70. doi:10.1186/s40779-022-00429-5
Xu, J., Zhang, M., Du, W., Zhao, J., Ling, G., and Zhang, P. (2022b). Chitosan-based high-strength supramolecular hydrogels for 3D bioprinting. Int. J. Biol. Macromol. 219, 545–557. doi:10.1016/j.ijbiomac.2022.07.206
Xu, Y., Xu, Y., Bi, B., Hou, M., Yao, L., Du, Q., et al. (2020). A moldable thermosensitive hydroxypropyl chitin hydrogel for 3D cartilage regeneration in vitro and in vivo. Acta Biomater. 108, 87–96. doi:10.1016/j.actbio.2020.03.039
Yeo, Y., Geng, W., Ito, T., Kohane, D. S., Burdick, J. A., and Radisic, M. (2007). Photocrosslinkable hydrogel for myocyte cell culture and injection. J. Biomed. Mater. Res. Part B, Appl. Biomaterials 81 (2), 312–322. doi:10.1002/jbm.b.30667
Yu, Y., Moncal, K. K., Li, J., Peng, W., Rivero, I., Martin, J. A., et al. (2016). Three-dimensional bioprinting using self-assembling scalable scaffold-free “tissue strands” as a new bioink. Sci. Rep. 6 (1), 28714–28811. doi:10.1038/srep28714
Yue, K., Trujillo-De Santiago, G., Alvarez, M. M., Tamayol, A., Annabi, N., and Khademhosseini, A. (2015). Synthesis, properties, and biomedical applications of gelatin methacryloyl (GelMA) hydrogels. Biomaterials 73, 254–271. doi:10.1016/j.biomaterials.2015.08.045
Zennifer, A., Senthilvelan, P., Sethuraman, S., and Sundaramurthi, D. (2021). Key advances of carboxymethyl cellulose in tissue engineering and 3D bioprinting applications. Carbohydr. Polym. 256, 117561. doi:10.1016/j.carbpol.2020.117561
Zhang, J., Haghiashtiani, G., Hübscher, T., Kelly, D. J., Lee, J. M., Lutolf, M., et al. (2021c). 3D extrusion bioprinting. Nat. Rev. Methods Prim., 1(1): 75 doi:10.1038/s43586-021-00073-8
Zhang, J., Song, L., Xu, L., Fan, Y., Wang, T., Tian, W., et al. (2021a). Knowledge domain and emerging trends in ferroptosis research: A bibliometric and knowledge-map analysis. Front. Oncol. 11, 686726. doi:10.3389/fonc.2021.686726
Zhang, J., Wehrle, E., Rubert, M., and Müller, R. (2021b). 3D bioprinting of human tissues: Biofabrication, bioinks, and bioreactors. Int. J. Mol. Sci. 22 (8), 3971. doi:10.3390/ijms22083971
Zhang, X., Chen, X., Hong, H., Hu, R., Liu, J., and Liu, C. (2022). Decellularized extracellular matrix scaffolds: Recent trends and emerging strategies in tissue engineering. Bioact. Mater. 10, 15–31. doi:10.1016/j.bioactmat.2021.09.014
Zhang, X.-L., Zheng, Y., Xia, M.-L., Wu, Y.-N., Liu, X.-J., Xie, S.-K., et al. (2020). Knowledge domain and emerging trends in vinegar research: A bibliometric review of the literature from WoSCC. Foods (Basel, Switz. 9 (2), 166. doi:10.3390/foods9020166
Zhang, Y. S., Arneri, A., Bersini, S., Shin, S.-R., Zhu, K., Goli-Malekabadi, Z., et al. (2016). Bioprinting 3D microfibrous scaffolds for engineering endothelialized myocardium and heart-on-a-chip. Biomaterials 110, 45–59. doi:10.1016/j.biomaterials.2016.09.003
Zhao, M., Zhang, H., and Li, Z. (2022). A bibliometric and visual analysis of nanocomposite hydrogels based on VOSviewer from 2010 to 2022. Front. Bioeng. Biotechnol. 10, 914253. doi:10.3389/fbioe.2022.914253
Keywords: 3D bioprinting, tissue engineering, bio-ink, hydrogels, extrusion-based bioprinting, bibliometrics
Citation: Ding Z, Tang N, Huang J, Cao X and Wu S (2023) Global hotspots and emerging trends in 3D bioprinting research. Front. Bioeng. Biotechnol. 11:1169893. doi: 10.3389/fbioe.2023.1169893
Received: 20 February 2023; Accepted: 08 May 2023;
Published: 25 May 2023.
Edited by:
George Alexander Truskey, Duke University, United StatesReviewed by:
Yi-Chen Ethan Li, Feng Chia University, TaiwanBo-Wen Zheng, Peking University, China
Amedeo Franco Bonatti, University of Pisa, Italy
Krishna Pramanik, National Institute of Technology Rourkela, India
Copyright © 2023 Ding, Tang, Huang, Cao and Wu. This is an open-access article distributed under the terms of the Creative Commons Attribution License (CC BY). The use, distribution or reproduction in other forums is permitted, provided the original author(s) and the copyright owner(s) are credited and that the original publication in this journal is cited, in accordance with accepted academic practice. No use, distribution or reproduction is permitted which does not comply with these terms.
*Correspondence: Junjie Huang, aGpqaHVhbmdAaG90bWFpbC5jb20=; Xu Cao, aHVnaGNhb3h1QGhvdG1haWwuY29t; Song Wu, eHkzd3MxOTY5QGhvdG1haWwuY29t
†These authors have contributed equally to this work