- 1Botany and Microbiology Department, Faculty of Science, Beni-Suef University, Beni-Suef, Egypt
- 2Integrated Molecular Plant Physiology Research, Department of Biology, University of Antwerp, Antwerpen, Belgium
- 3Biochemistry Division, Chemistry Department, Faculty of Science, Helwan University, Helwan, Egypt
- 4Botany and Microbiology Department, Faculty of Science, Helwan University, Helwan, Egypt
- 5Department of Biology, College of Science, Princess Nourah bint Abdulrahman University, Riyadh, Saudi Arabia
The potential of microalgae to produce valuable compounds has garnered considerable attention. However, there are various challenges that hinder their large-scale industrial utilization, such as high production costs and the complexities associated with achieving optimal growth conditions. Therefore, we investigated the effects of glycine at different concentrations on the growth and bioactive compounds production of Synechocystis sp. PAK13 and Chlorella variabilis cultivated under nitrogen availability. Glycine supplementation resulted in increased biomass and bioactive primary metabolites accumulation in both species. Sugar production, particularly glucose content, significantly improved in Synechocystis at 3.33 mM glycine (1.4 mg/g). This led to enhanced organic acid, particularly malic acid, and amino acids production. Glycine stress also influenced the concentration of indole-3-acetic acid, which was significantly higher in both species compared to the control. Furthermore, fatty acids content increased by 2.5-fold in Synechocystis and by 1.36-fold in Chlorella. Overall, the exogenous application of glycine is a cheap, safe, and effective approach to enhancing sustainable microalgal biomass and bioproducts production.
Introduction
Microalgae have garnered much attention due to their ability to produce a diverse array of compounds with significant economic, medicinal, and industrial importance (Guedes et al., 2011; Santhosh et al., 2016). In this regard, microalgae have emerged as one of the most attractive and promising feedstocks for these industrial products due to their rapid growth rate, self-renewability, fast generation time, and high metabolites content (Liu et al., 2017; Fathy et al., 2021). For instance, microalgae produce astaxanthin, beta-carotene, phycocyanin, and omega-3 fatty acids (Yaakob et al., 2014; Rahman, 2020). These compounds have been shown to possess powerful antioxidant properties (Dantas et al., 2019). Recently, microalgae have been increasingly utilized in the production of cosmetic products, such as anti-aging creams and sunscreens (Yarkent et al., 2020). They also contain organic matter, which stores solar energy as biochemical energy in the form of carbon, hydrogen, oxygen, and nitrogen components (Cuellar-Bermudez et al., 2015). Despite their immense potential for a wide range of industrial products, the use of microalgae at an industrial scale is impeded by several obstacles. The high cost of production is a significant challenge, as microalgae require a controlled environment to get high biomass accumulation. Thus achieving optimal growth conditions for microalgae outdoors is a complex process (Yin et al., 2020). Consequently, the approach of combining microalgae cultivation with media containing cheap sources of nutrients (e.g., nitrogen) provided a cost-effective and eco-friendly perspective in microalgae-based bioproducts production (Nateghpour et al., 2021; Sarma et al., 2023). In this regard, enhancing the microalgal growth and bioproducts accumulation with chemicals or environmental factors had been recently used (Maity et al., 2014; Gao et al., 2023).
Nitrogen is a constituent in all structural and functional proteins such as peptides and enzymes, as well as chlorophyll, energy transfer molecules, and genetic elements in algal cells (Cai et al., 2013; Kim et al., 2016). This indeed makes nitrogen one of the most important dietary factors for algal growth. Providing enough nitrogen through the culture medium significantly speeds up cell development and enriches the biochemical content of microalgae (Wang et al., 2013; Song et al., 2023). On the other hand, nitrogen deficiency changes the organism’s metabolic route. For example, it shifted lipid metabolism away from membrane lipid production toward neutral lipid storage, resulting in the total lipid content being raised (De Bhowmick et al., 2015; Kozan et al., 2023). In this regard, most microalgae may use a range of nitrogen sources, and each source is first converted to ammonium and then metabolized into amino acids via different key metabolic enzymes (e.g., glutamine synthetase, glutamate synthase, or NADP glutamate dehydrogenase) (Cai et al., 2013; Salbitani and Carfagna, 2021). In a related study, ammonium induced a reciprocal increase in the amino acids level in Synechocystis sp. (Mérida et al. (1991). Through the synthesis of cyanophycin, Synechocystis has the remarkable capacity to internally store ammonium nitrogen (Yu et al., 2013). This nitrogen storage capacity is a promising potential for removing nitrogen from wastewater, and can be utilized as a valuable biofertilizer (Chittora et al., 2020). Increased Amino acids levels, consequently improved organic acids, and fatty acids production, where nitrogen supplementation as ammonium increased the protein content in Dunaliella salina (Norici et al., 2002), and lipid content in Chlorella sorokiniana (Wan et al., 2012). Microalgae fatty acids, for instance, are of great commercial importance in several fields, including bioenergy (Tabatabaei et al., 2011; Almomani et al., 2023). Therefore, more research is needed to increase the productivity of commercially valuable fatty acids and reduce the cost of their production. Particularly, saturated fatty acids are preferred in biodiesel production, while polyunsaturated fatty acids may have an unfavorable effect on biodiesel attributes such as ignition quality and oxidative stability (Doan et al., 2011). To this end, efficient technologies and highly productive strains were used (Maltsev and Maltseva, 2021).
Glycine, being an amino acid, contains both carbon and nitrogen that can be utilized by microalgae for many metabolic processes such as protein synthesis (De la Hoz Siegler et al., 2011; Matantseva et al., 2018). The bioavailability of glycine as a nitrogen source for microalgae is high due to its solubility and stability in the aquatic environment (Bowden et al., 2018). Glycine is readily assimilated by microalgae; thus, it can support the high growth rates and biomass production in certain microalgal species (Kim et al., 2016; Wang et al., 2016). Furthermore, glycine is a cheap and commercially available amino acid that can be easily obtained in massive quantities. Its application does not require complex and costly equipment, making it a simple and cost-effective alternative to traditional nitrogen sources such as nitrate and ammonium. This makes glycine as a potentially attractive way for large-scale microalgal cultivation.
Consequently, the cultivation of microalgae using glycine holds the potential to increase biomass production and this would also result in biomolecules accumulation including lipids, and essential amino acids. This bioproduct rich biomass has a potential as a feedstock for producing biofuel, bio fertilizers, pharmaceuticals, nutraceuticals and other bio-based products. Enhanced yield of valuable bioproducts per same microalgae biomass makes industrial production processes more efficient and profitable (Harun et al., 2010). Moreover, scaling up microalgae production on an industrial level can have cost-saving benefits, as it requires a smaller amount of cultivation space, energy, time and resources to generate the targeted level of biomolecules. These reduced production costs contribute to the increased competitiveness of microalgae-based technologies. Additionally, there is a growing market demand for products derived from microalgae. Bioactive substances such as omega-3 fatty acids, antioxidants, pigments, and biofuels are becoming more accessible and affordable (Michalak and Chojnacka, 2014; Khan et al., 2018). This accessibility has the potential to drive innovation in diverse industries including pharmaceuticals, cosmetics, and bioenergy.
The present investigation explores the impact of exogenous glycine as a nitrogenous source on the growth and bioproduct accumulation induction in the cyanobacterium Synechocystis sp. PAK13 and eukaryotic microalga Chlorella variabilis. To this end, this comprehensive study sheds light on the role of glycine in increasing the production of primary metabolites (carbohydrates, amino acids, organic acids, and lipids) and secondary metabolites such as IAA.
Materials and methods
Strains and cultural conditions
Microalgae strains Synechocystis sp. PAK13 and Chlorella variabilis DT025 were kindly provided from Algal Biotechnology Lab, Faculty of Science, Beni-Suef University, Egypt which were isolated from the marine habitat of the Red Sea and identified by using 16S and 18S rRNA gene (Supplementary Figures S1, S2). Then they were cultivated on Wuxal medium (WM), which is a universal dünger liquid plant fertilizer that has 8% N, 8% P2O5, 6% K2O, 0.01% B, 0.004% Cu, 0.02% Fe, 0.012% Mn and 0.004% Zn (Wilhelm Haug GmbH and Co., KG, Germany). Our strains have exhibited favorable growth in the synthetic Wuxal medium, indicating their adaptability to the employed salt concentration. To prepare the culture medium, 800 µL of WM per liter of tap water was used. To imitate the salinity of the Red Sea habitat, 1 g/L NaCl was added to the medium. This NaCl concentration was selected based on the salinity of the natural environment from which our strains were isolated (Winckelmann et al., 2015; Fathy et al., 2020). In addition to the selected NaCl concentration, we applied the optimal neutral pH 7.5 for the microalgae culture to achieve the highest yield of growth and byproducts (Wang et al., 2010). Microalgae strains were allowed to grow in the appropriate conditions, which included a temperature of 28°C ± 2°C, and light intensity was 30.4 µmolm−2s−1, a white, fluorescent light source used with a specific wavelength of 450–650 nm, and a photoperiod of 14 h light/10 h dark cycle. The temperature of microalgae cultivation was selected according to Ras et al. (2013), where the optimal temperature for our specific strains falls within the range of 28°C–30°C and they are very close to outdoors temperatures in Egypt. The photon flux density was measured using a digital lux meter (LX1330B, China). In addition, different amounts of glycine (1.66, 3.33, 6.66, 13.33, and 26.66 mM) were added to the culture mediums. These glycine concentrations were selected based on a thorough review of the literature, e.g., (Wang et al., 2016; Yue et al., 2021), and preliminary experiments conducted in our laboratory. This was also a logarithmic scale that covers a wide range of concentrations to investigate potential dose-dependent effects while maintaining a constant ratio between each concentration.
Growth curves
In this study, a spectrophotometer (NanBei Instrument®, China) was used to measure the optical density of the microalgae samples at 700 nm. This allowed us to construct growth curves that provide insights into the sample’s growth dynamics as reported by Elsayed et al. (2017). To decide the wet-weight biomass of microalgae culture, we utilized a centrifugation-based approach. Specifically, we centrifuged 5 mL of our culture, then we drained the remained liquid in an inverted position on a paper tissue for 5 min at room temperature. The weight of the wet biomass was then measured. Furthermore, the duplication time, the number of generations, and the specific growth rate of our sample were measured by the established protocols by Fabregas et al. (1986) and utilizing the methodologies described by Jin et al. (2011) and Krzemińska et al. (2014). These metrics are crucial in understanding the growth characteristics of microalgae samples and were calculated to ensure the accuracy and reproducibility of the results.
Biochemical composition investigation
Estimation of pigment content
To quantify the photosynthetic pigments in microalgae samples, the protocol of Moran and Porath (1980) was followed. Whereas, at the exponential phase of growth, 2 mL were collected from strains culture by centrifugation at 13,000 rpm. The resulting pellets were weighed and placed in a covered glass tube, which was mixed with 5 mL of 80% ice acetone at 4°C for 24 h. After centrifugation at 13,000 rpm for 3 min, the supernatants were collected for analysis. The estimation of pigments was performed following the established protocols by Metzner et al. (1965) and Pflanz and Zude (2008), while spectrophotometric measurements were measured at 480, 645, and 663 nm.
Estimation of soluble carbohydrates content
The quantitative estimation of soluble carbohydrates was performed by Yemm and Willis (1954). Whereas 2 mL of the exponential phase culture were centrifuged at 13.000 rpm and changed into glass-capped tubes soaked in 5 mL of absolute ethanol before boiling for 10 min in a water bath. In a test tube, 1 mL of the ethanolic extract was mixed with 4 mL of ice-concentrated anthrone reagent freshly made by dissolving 200 mg anthrone in 100 mL Conc. H2SO4 and vortexed. The tubes were then placed in a boiling water bath for 10 min before cooling in an ice bath for 5 min. Furthermore, di-saccharides were identified following van Handel (1968), whereas, to decompose reactive sucrose, 1 mL of the extract was added to 0.1 mL of 5.4 N KOH at 97°C for 10 min. The reaction mixture was then treated with 3 mL of anthrone reagent and placed in a boiling water bath for 10 min before cooling in an ice bath for 5 min. Finally, spectrophotometry was performed at 620 nm against a water reagent blank. The content of mono and di-saccharides was quantified using a standard curve of glucose and sucrose. Another standard method depends on the devices performed according to Al Jaouni et al. (2018), 2 mL microalgae was extracted in ethanol (80% v/v) by boiling for 30 s three times and once at room temperature. Derivation and resuspension of samples in dH2O, the supernatants were kept at 20°C for more investigation. The concentration of soluble sugars was figured out (CE in a Coulter PACE system 5500) and detected using a diode array detector. Using corresponding standards (glucose, fructose, and sucrose), concentrations were calculated.
Estimation of organic acids content
Organic acids from microalgae strains in a mixture of 0.3% (w/v) butylated hydroxy anisole and 0.1% phosphoric acid were extracted. HPLC with a SUPELCOGEL C-610H column equipped with a UV detection system operating at 210 nm was used to estimate the concentrations of citric, succinic, fumaric, and malic acids (LaChromL-7455 diode array, LaChrom, Tokyo, Japan). Phosphoric acid (0.1% v/v) was eluted at a rate of 0.45 mL/min as the mobile phase. Oxalic, malic, succinic, citric, isobutyric, and fumaric acids were used as standards.
Estimation of amino acids content
Amino acids were extracted from 2 mL of microalgae cultures in an aqueous ethanol concentration of 80% (v/v). The extraction buffer contained an internal standard (norvaline) to compensate for the loss of amino acids during ethanol extraction and centrifugation at 20,000 rpm for 20 min. The pellet was resuspended in chloroform after the supernatant had been evaporated. The microalgae residue was re-extracted in water (HPLC grade), and the supernatant was mixed with the chloroform-suspended pellet after centrifugation. The extracts were then centrifuged and filtered through Millipore microfilters with 0.2-μm pore size. Amino acids were separated using a BEH amide (2.1 mm × 50 mm) column and quantified using a Waters Acquity UPLC-tqd mass spectrometer (Sinha et al., 2013). A list of amino acids standards (glycine, alanine, isoleucine, leucine, methionine, valine, phenylalanine, glutamine, asparagine, threonine, serine, cystine, tyrosine, lysine, histidine, arginine, glutamic acid, and aspartate) was used as standard.
Estimation of fatty acids
Fatty acids were extracted from 100 mg of microalgae biomass in 50% aqueous methanol at 25°C. For identification, a GC/MS analysis (Hewlett Packard 6890, MSD 5975 mass spectrometer, United States) with an HP-5 MS column (30 μm × 0.25 µm x 0.25 µm) was used, followed by fatty acid identification using the NIST 05 database and the Golm Metabolome Database (http://gmd.mpimp-golm.mpg.de) (AbdElgawad et al., 2020). A list of fatty acids standards (myristic, palmitic, heptadecanoic, stearic, arachidic, docosanoic, tricosanoic, pentacosanoic, palmitoleic, heptadecenoic, oleic, linolenic, linoleic, and eicosenoic) was used as standard.
Statistical analysis
All trials were set up in triplicate using a completely randomized design. The data are reported as (means ± standard error) and visualized using GraphPad Prism 8.4.2 software. The Student’s t-test (p ≤ 0.05, p ≤ 0.01, p ≤ 0.001, and p ≤ 0.0001) were used to compare each experimental value was compared to the corresponding control value. A two-way ANOVA was performed (Tukey test, p ≤ 0.05) using the statistical package SPSS (version 20; IBM).
Results
Growth
To assess the effect of glycine on Synechocystis and Chlorella, the growth curves of the two microalgae strains were investigated (Figure 1). The growth pattern can be divided into three distinct phases based on the growth rate, i.e., lag phase, exponential phase, and stationary phase. During the lag phase, which was seen during the initial few days, Synechocystis and Chlorella acclimatized to the new environment and the growth is slow. This phase was reflected in the data as a plateau in the OD values at time points 0–4 days. The exponential phase was characterized by a rapid increase in growth rate as the microalgae population adapts to the environment and reaches its maximum growth rate. This phase was reflected in the data as an increase in OD values at 5–14 days. The stationary phase was the period in which the microalgae population reaches it carrying capacity and the growth rate becomes stable. This phase was reflected in the data as a plateau in the OD values at 14 and 17 days. Glycine at the concentrations of 6.6, 13.3, and 26.6 mM significantly increased the growth rate of both strains. On the other hand, low doses had a slight effect on growth compared to control samples. These findings were supported (Figure 2), which showed that the duplication time decreased with increasing glycine concentrations. This increased the number of generations and specific growth rate for both strains. Furthermore, estimating biomass analysis (Figure 3) revealed that glycine concentration at 26.66 mM increased Synechocystis and Chlorella biomass production by 194% and 395% respectively, compared to the control sample. Similarly, the glycine concentrations at 6.6- and 13.3-mM glycine increased the growth by 2 folds. In contrast, 1.66 and 3.33 mM of glycine decreased the Synechocystis biomass compared to the control growth strain. Subsequently, it boosted both strains' growth rates, and duplication time decreased as glycine concentrations increased, resulting in a rise in the number of generations and specific growth rate for both strains. Interestingly, glycine boosted biomass production more in Chlorella than Synechocystis.
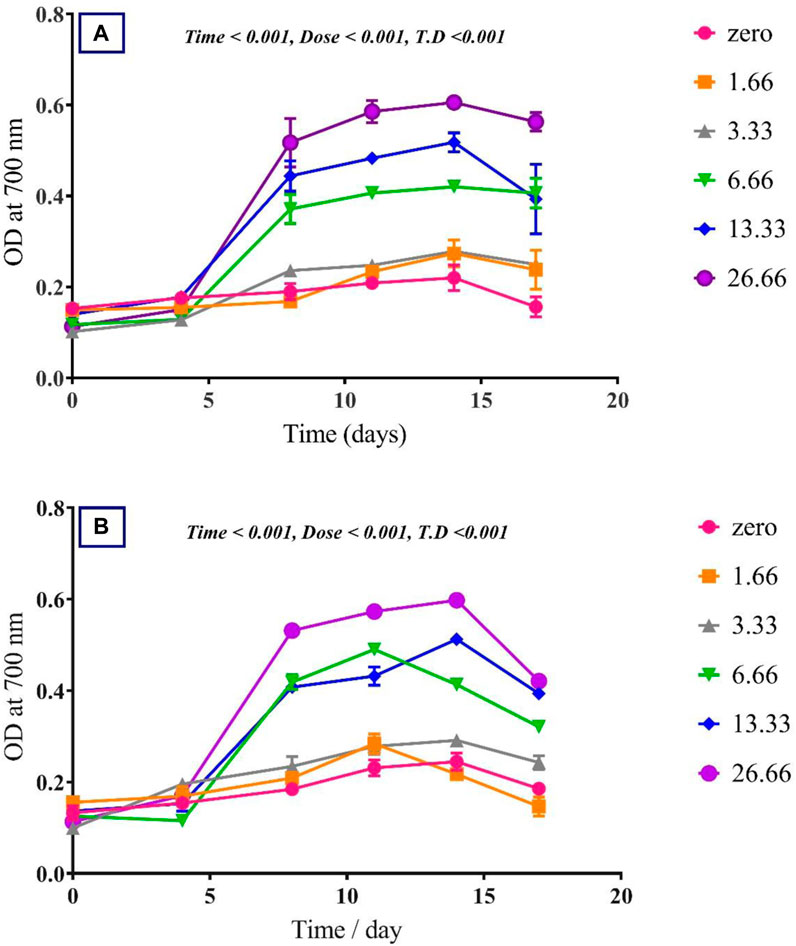
FIGURE 1. The effect of different glycine concentrations on the growth curve of (A) Synechocystis sp. and (B) Chlorella sp. Plotted points show mean daily averages ± SE, n = 3. The p-value is considered significant < 0.05 using t. test analysis.
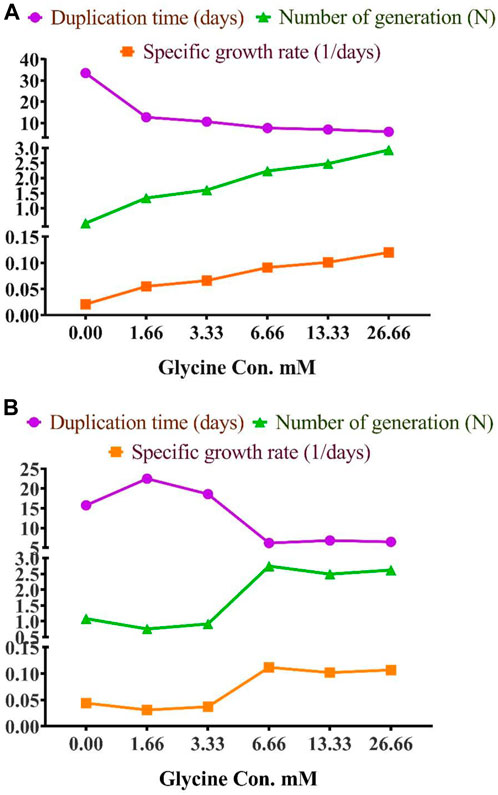
FIGURE 2. The effect of different glycine concentrations on duplication time, number of generations, and specific growth rate of (A) Synechocystis sp. and (B) Chlorella sp.
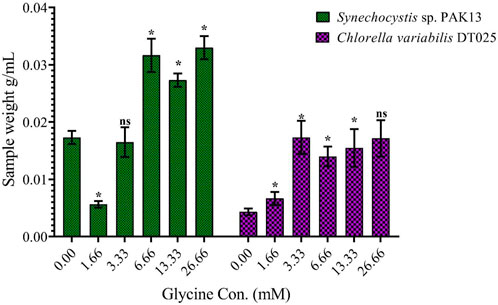
FIGURE 3. Explaining the effect of different glycine concentrations for 14 days on the wet-weight biomass of Synechocystis sp. and Chlorella sp. Data are presented as the average of three independent replicates ± SE. The statistical significances p > 0.05, p ≤ 0.05, p ≤ 0.01, p ≤ 0.001 and p ≤ 0.0001 were marked by the symbols ns, *, **, ***, and ****, respectively.
Photosynthetic pigments
To understand the observed increases in growth, the photosynthetic pigment was estimated (Figure 4). In line with increased growth, the highest glycine concentration (26.66 mM) increased chlorophyll in Synechocystis and Chlorella by 19.4 and 9.3 folds, respectively. Similarly, chlorophyll b was induced to 44.3 and 13.7 folds at 26.66 mM in Synechocystis and Chlorella, respectively. To protect the photosynthesis system, carotenoids were significantly increased in Synechocystis by 55.3 folds, and in Chlorella by 16.3 folds higher than non-treated strain. In a nutshell, in conjunction with enhanced growth, the greatest glycine boosted chlorophyll a, b, and carotenoids in Synechocystis by 19.4, 44.3, and 55.3 folds higher than in non-treated strain, and these levels were more pronounced in Chlorella.
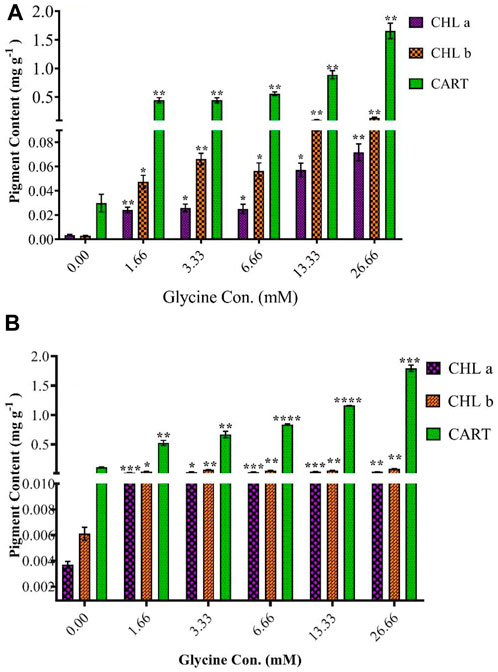
FIGURE 4. The effect of different glycine concentrations for 14 days on chlorophyll a (CHLa), chlorophyll b (CHLb), and carotenoids (CART) content in (A) Synechocystis sp. and (B) Chlorella sp. Data are presented as an average of three independent replicates ± SE. The statistical significances p > 0.05, p ≤ 0.05, p ≤ 0.01, p ≤ 0.001 and p ≤ 0.0001 were marked by the symbols ns, *, **, ***, and ****, respectively.
Indole-3-acetic acid
The effect of glycine on microalgae cell hormone levels was demonstrated by indole-3-acetic acid (IAA) content (Table 1). IAA levels gradually increased to 2.7 folds 26.66 mM in Synechocystis, which was also observed in Chlorella increased, which showed 3.6 times increase in IAA. In summary, glycine had a positive effect on microalgal cell hormone levels.
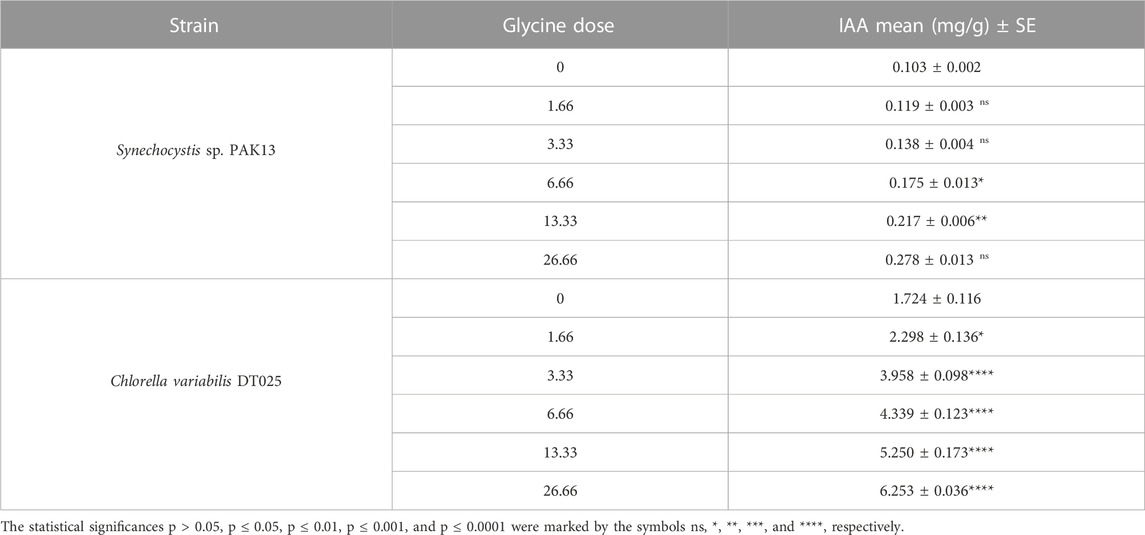
TABLE 1. IAA content in Synechocystis sp. and Chlorella sp. have grown under different concentrations of glycine for 14 days.
Soluble sugar production
Increased sugar production could reflect the improvement in photosynthesis under glycine exposure. Soluble sugar profiling revealed that glucose content was dramatically increased in Synechocystis with increasing glycine concentrations, specifically at 3.33 mM glycine (increased by 10.5 folds). On the other hand, glucose content was reduced in Chlorella. Moreover, sucrose levels in Synechocystis and Chlorella gradually were declined with increasing glycine concentrations at a range from 2.4 mg/g to 1.97 mg/g and 0.9 mg/g to 0.74 mg/g, respectively (Figure 5). We further measured the fructose, total soluble sugar, and glycogen content as reported in (Table 2). For Synechocystis, the fructose content was highest at the 1.66 glycine dose level (1.70 mg/g) and lowest in the 26.66 glycine dose level (0.66 mg/g). The total soluble sugar content was highest at the 6.66 glycine dose level (5.44 mg/g) and lowest at the control sample (3.03 mg/g). The glycogen content was highest at the 3.33 glycine dose level (88.29 mg/g) and lowest in the 26.66 glycine dose level (29.63 mg/g). While for Chlorella, the fructose content was highest in the 3.33 glycine dose level (1.08 mg/g) and lowest at the 1.66 glycine dose level (0.58 mg/g). The total soluble sugars content was highest in the 3.33 glycine dose level (4.00 mg/g) and lowest at the 13.33 glycine dose level (1.38 mg/g). The glycogen content was highest in the 3.33 glycine dose level (4.08 mg/g) and lowest in the control sample (3.54 mg/g). Overall, the results suggest that glycine concentration affects the fructose, total soluble sugars, and glycogen content of these two strains in different ways.
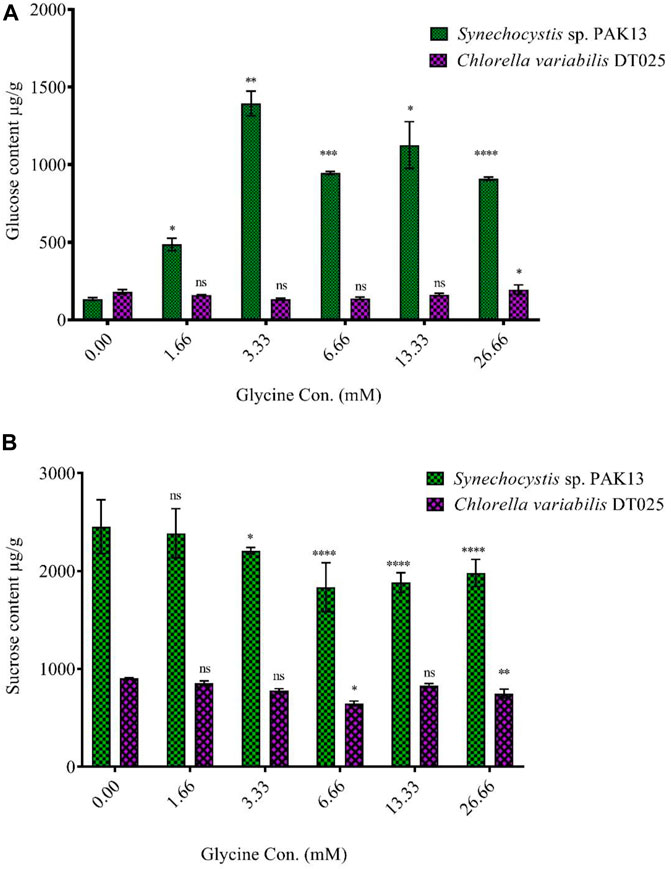
FIGURE 5. Effect of different glycine concentrations for 14 days on (A) glucose and (B) sucrose content in wet weight for Synechocystis sp. and Chlorella sp. Data are presented as the average of three independent replicates ± SE. The statistical significances p > 0.05, p ≤ 0.05, p ≤ 0.01, p ≤ 0.001 and p ≤ 0.0001 were marked by the symbols ns, *, **, ***, and ****, respectively.
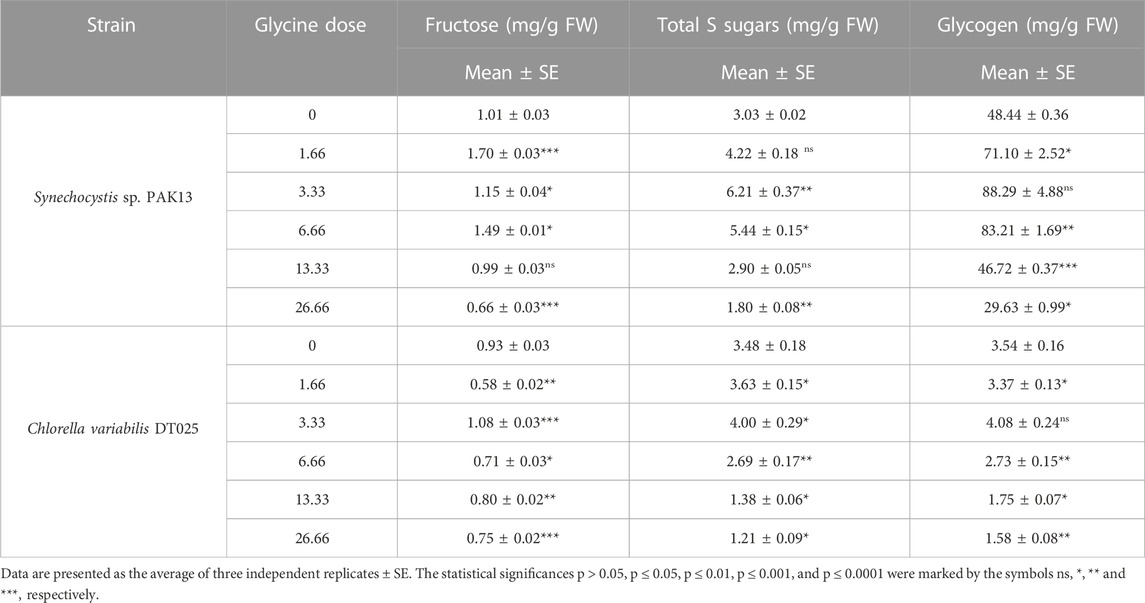
TABLE 2. Estimating fructose, total soluble sugars, and glycogen content in Synechocystis sp. and Chlorella sp. have grown under different concentrations of glycine for 14 days.
Organic acid
The changes in the levels of sugars under glycine treatment are expected to affect the tricarboxylic acid cycle intermediates, such as organic acids. Organic acids analysis indicated that they are quite different in the two strains (Figure 6) and (Supplementary Table S1). Synechocystis produced much higher levels of oxalic acid, citric acid, and isobutyric acid than Chlorella, while Chlorella produced higher levels of succinic acid. In Synechocystis, the production of oxalic acid and citric acid was significantly decreased at higher glycine doses, while the production of isobutyric acid was increased. On the other hand, the production of malic acid and fumaric acid increases at higher glycine concentrations. For Chlorella, the production of oxalic acid was significantly decreased at higher glycine doses, while the production of citric acid and isobutyric acid was increased. Interestingly, total organic acids concentrations were higher in Synechocystis. Whereas Synechocystis content declined in the initial dosages, after that it was gradually increased to hit 153.3% more than the control strain at a glycine dose of 26.66 mM. However, organic acid content was dramatically increased in Chlorella with rising glycine levels, reaching 152.7% higher than the control sample at a glycine dose of 3.33 mM. These results can be mainly attributable to malic acid. Under the most severe glycine stress, organic acid concentration was equally improved in Synechocystis and Chlorella. This is due to malic acid, which was greatly influenced by exogenous glycine. This may suggest that Synechocystis was more effective in organic acid metabolism and production. In conclusion, our data showed that the effect of glycine was species-specific.
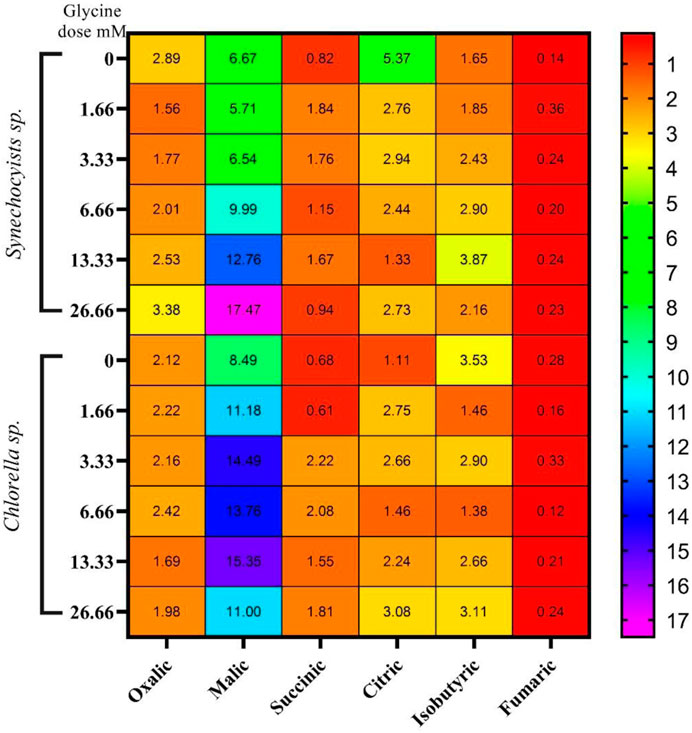
FIGURE 6. The heat map represents the effect of different glycine concentrations for 14 days on organic acids content (mg/g) wet-weight biomass Synechocystis sp. and Chlorella sp. Data are presented as the average of three independent replicates.
Amino acids
The impact of glycine provision on the amino acid profiles of the two strains was assessed. Glycine altered the levels of both nonpolar and polar amino acids. The concentrations of the nonpolar amino acidswere gradually increased with rising glycine concentration (Figure 7; Supplemenatry Table S2). Under 26.6 mM, nonpolar amino acids were increased to hit 4.8 folds in Synechocystis glycine compared to those in the non-treated strain. While Chlorella showed an increase of 2.2 folds higher than the control strain under 26.6 mM glycine. Consequently, it was obvious from the table interpretation that glycine was the main nonpolar amino acid affected by glycine exposure. Where 70% of the nonpolar amino acids content, specifically in Synechocystis was increased by 5.93 folds higher than the control one at the dose of 26.66 mM glycine. Overall, it can be observed that both Synechocystis and Chlorella had an increased nonpolar amino acids content with increasing glycine concentration. This trend is observed for most tested amino acids, including alanine, isoleucine, leucine, methionine and valine. Interestingly, Synechocystis had a much larger increase in nonpolar amino acid content with increasing glycine concentration. For example, at a glycine concentration of 26.66 mM, Synechocystis had a total nonpolar amino acid content of 144.47 mg/g, while Chlorella had a total nonpolar amino acid content of only 81.20 mg/g. Phenylalanine content also showed a different pattern, Synechocystis had a slight increase in phenylalanine content, while Chlorella showed a more significant increase in phenylalanine content at 13.33 mM and 26.66 mM. On the other hand, polar amino acids in Synechocystis (Figure 7) and (Supplementary Table S3) were significantly declined under all glycine dosages. For instance, at 26.66 mM, glycine was reduced by 3.5 folds compared to the control strain. Moreover, polar amino acids were decreased in Chlorella, with the most glycine doses around half the control one, except for 1.6- and 26.6-mM glycine. This suggested that the polar amino acids content in both species varies depending on the concentration of glycine. For example, in Synechocystis, the total polar amino acids content was decreased from 9.43 at the control to 7.79 at 13.33 mM glycine and it was decreased to 2.751 at 26.66 mM glycine. While, in Chlorella, the total polar amino acids content was steadily increased from 4.89 at control to 8.97 at 26.66 mM glycine. The differences in the specific polar amino acid content between the two species. For example, at control conditions, Synechocystis had a higher content of glutamine, asparagine, and tyrosine, while Chlorella had a higher content of threonine, serine and cysteine. However, as the glycine concentration was increased, the differences between the two species became less pronounced.
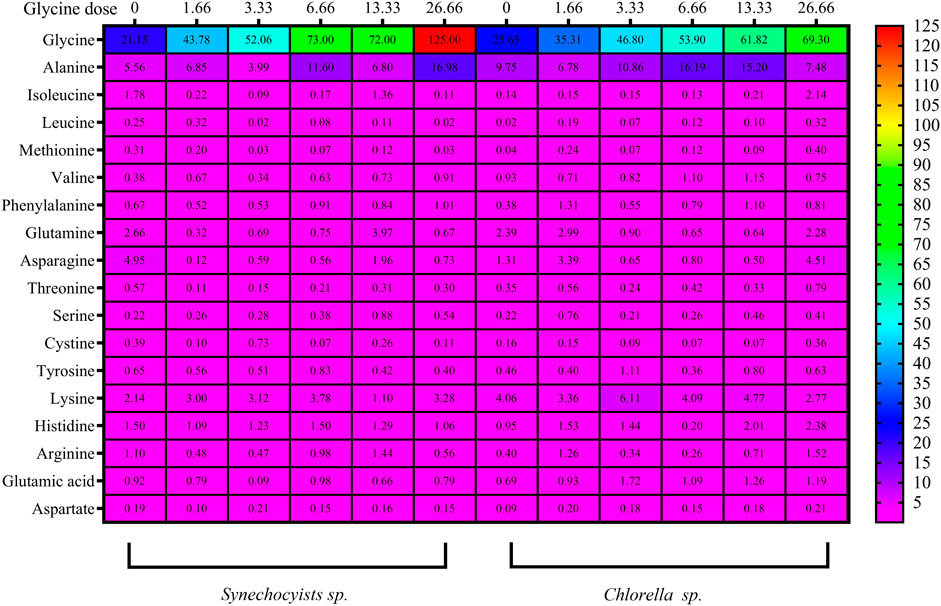
FIGURE 7. The heat map represents the effect of different glycine concentrations for 14 days on polar and nonpolar amino acids, besides acidic and basic amino acids content (mg/g) in Synechocystis sp. and Chlorella sp. Data are presented as the average of three independent replicates.
Furthermore, basic and acidic amino acid analyses revealed a steady state under all glycine treatments, while the greatest basic amino acid concentration in Synechocystis was 1.3 folds greater at 6.6 mM glycine (Figure 7; Supplementary Table 4). However, this was slightly different, but it was 1.5 folds higher at 3.3 mM glycine in Chlorella. The basic amino acids content was generally increased, except for lysine at the highest dose of 13.33, where it was significantly decreases. The acidic amino acids content, on the other hand, showed no consistent trend across the different glycine doses. For Chlorella, the results showed that the increased glycine dose, generally increased the basic amino acids content, except for histidine at 26.66 mM, where it was decreased significantly. The acidic amino acid content also showed no consistent trend across the different glycine doses.
Fatty acids
The induced effects of glycine could also be extended to include the tricarboxylic acid cycle intermediates, such as fatty acids. Thus, we measured the individual and total fatty acids (saturated and unsaturated), to get an overview of their potential for biodiesel production. To demonstrate these changes, we measured saturated fatty acids levels (Table 3). SFA levels were steadily increased, whereas SFA levels in Synechocystis reached 247% more than the control sample at 26.66 mM, and this was also observed in Chlorella (242% increase compared to control).
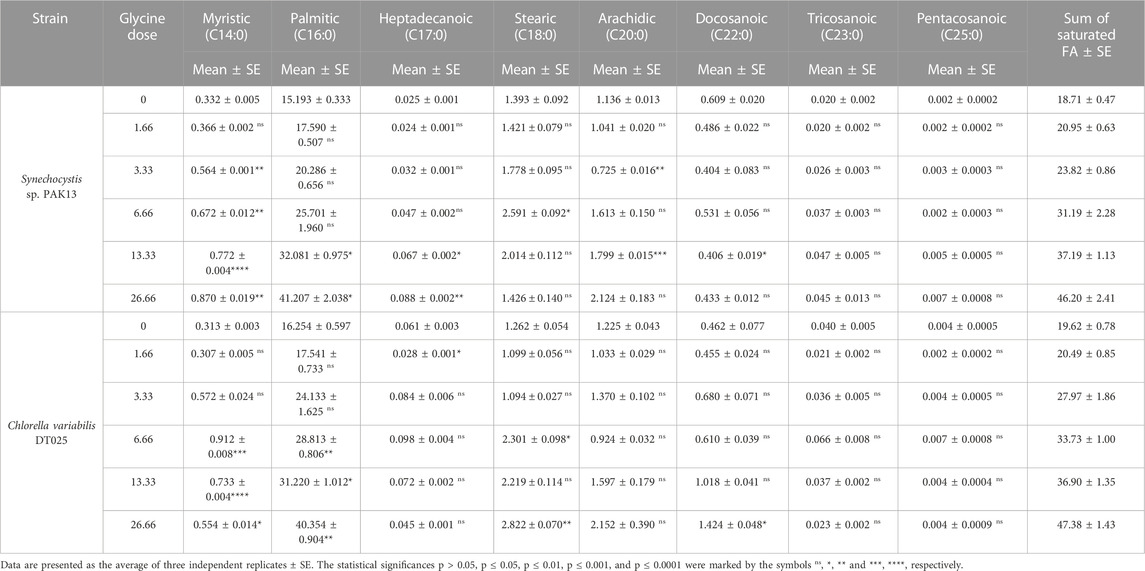
TABLE 3. Estimating saturated fatty acids content (mg/g) in Synechocystis sp. and Chlorella sp. have grown under different concentrations of glycine for 14 days.
The results showed that the total SFA content increased with increasing glycine concentration in both microalgal species. For instance, the total SFA in Synechocystis was increased from 18.71 to 46.20 mg/g, at 26.66 mM. While in Chlorella increased from 19.62 to 33.73 mg/g, over the same range of glycine concentrations. This indicated that glycine positively influenced the SFA synthesis in these microalgae. Regarding individual SFA, the results showed that the two microalgal species showed different types and amounts of FA produced in response to glycine. In Synechocystis, the major produced SFAs were palmitic (C16:0) and myristic (C14:0) acids. However, the content of heptadecanoic (C17:0) and stearic (C18:0) acids did not show significant changes, while arachidic (C20:0), docosanoic (C22:0), tricosanoic (C23:0), and pentacosanoic (C25:0) acids were significantly increased only at 6.66 and 13.33 mM. In contrast, in Chlorella, the major produced SFAs were palmitic (C16:0) and stearic (C18:0) acids. The content of myristic (C14:0) acid did not show significant changes, but heptadecanoic (C17:0), arachidic (C20:0), docosanoic (C22:0), tricosanoic (C23:0), and pentacosanoic (C25:0) acids were significantly increased only 13.33 mM. Overall, the results suggested that glycine supplementation differentially enhanced the production of SFA in both species.
Furthermore, unsaturated fatty acids (UFA) demonstrate that at a dose of 26.66 mM glycine, UFA in Synechocystis was increased to 250% greater than the control strain (Table 4). At 26.66 Mm, the UFA in Chlorella increased by 136%. In Synechocystis 26.66 mM of glycine led to the highest total fatty acid content (174.14 mg/g). The content of UFA at the highest dose led to the highest content of UFAs (127.943 mg/g). Among the UFAs, oleic acid (C18:1) showed the highest content in all samples, ranging from 27.631 to 72.671 mg/g. The other UFAs showed a slight increase, with linolenic acid (C18:3) of the most significant increase. While in Chlorella the total fatty acids content was also increased. At 26.66 mM lead to the highest total fatty acids content (118.54 mg/g). At UFAs levels, the content of palmitoleic acid (C16:1) was decreased, while the content of heptadecenoic acid (C17:1) showed a slight increase. Oleic acid (C18:1) showed a slight decrease, while linolenic acid (C18:3) and linoleic acid (C18:2) showed an increase. Overall, our findings suggested that glycine-treated Chlorella or Synechocystis cultivation can be used to produce biofuels and other bioproducts.
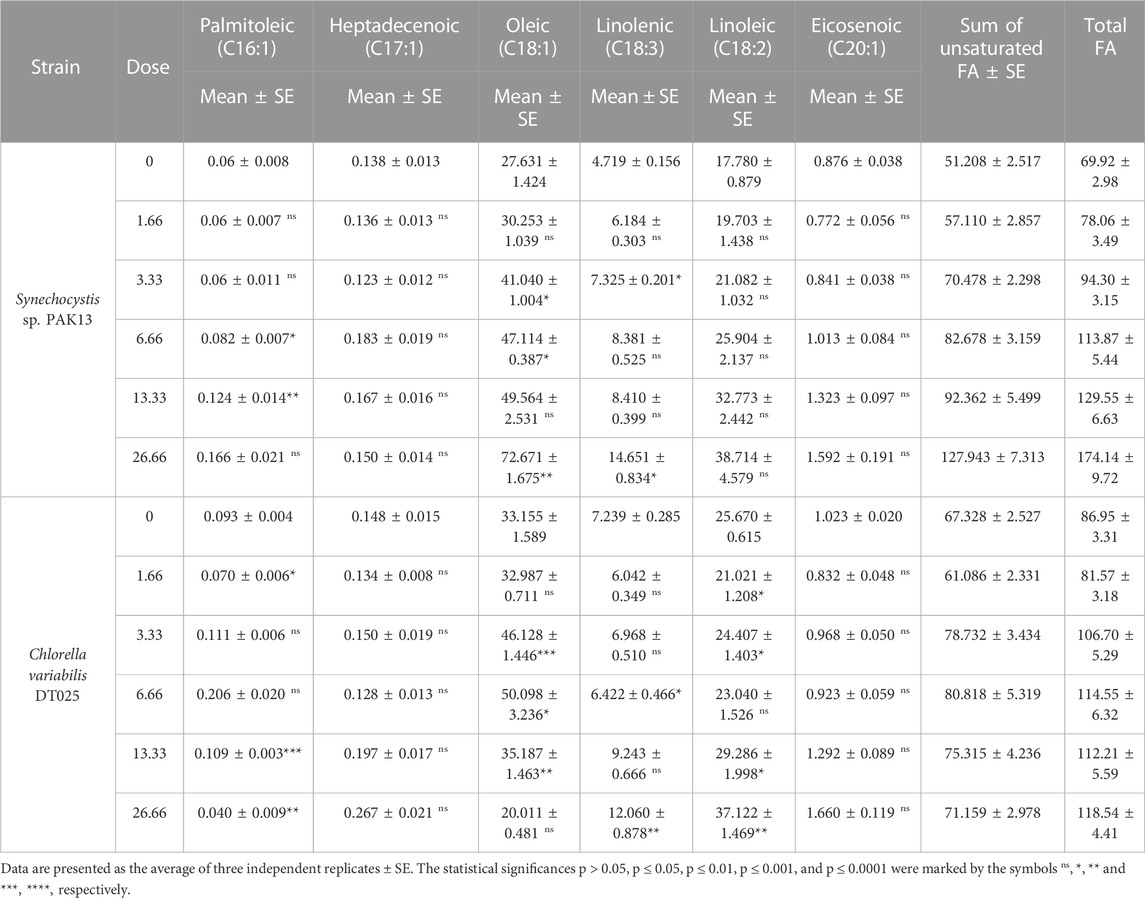
TABLE 4. Estimating unsaturated fatty acids and the total amount of fatty acids content (mg/g) in Synechocystis sp. and Chlorella sp. have grown under different concentrations of glycine for 14 days.
Discussion
Glycine improved biomass accumulation
The biomass results revealed that glycine at 6.6- and 13.3 and/or 26.66 mM increased biomass production in Synechocystis and Chlorella, compared to the control sample. In contrast, 1.66 and 3.33 mM of glycine decreased Synechocystis biomass compared to the control growth strain. To understand this increase in the biomass accumulation, the growth curves of both Synechocystis and Chlorella strains were analyzed. During the lag phase, the microalgae acclimatized to the new environment, resulting in slow growth. The exponential phase was characterized by a rapid increase in growth rate. The results of the present study showed that glycine significantly increased the growth rate of both strains, particularly at 6.6, 13.3, and 26.6 mM. The low concentration of glycine had a slight effect on growth compared to control samples. These findings were supported by the results of glycine concentration, which showed that the duplication time decreased with increasing glycine concentrations, increasing the number of generations and specific growth rate for both strains.
Furthermore, at high concentrations glycine showed the fastest cell growth rate. These findings were previously validated by Kumar et al. (2014) who reported that glycine treatment increased Chlorella cell production, furthermore, both biomass and growth rate were much improved. The highest concentration was higher (18.95%) than the control value (Garcıa et al., 2005). Similar to our results, Wang et al. (2016) found that Chlorella sorokiniana dry weight increased in a dose-dependent manner with biomass to 121% compared to the control. We hypothesized, that glycine boosts biomass and biomolecule productivity by stabilizing and improving the C4 system (Sun et al. (2016). Thus, enhancing photosynthetic efficiency allowed strains to use more solar energy when treated with glycine, this added energy was subsequently converted into more biomass and lipids. Similarly, mixotrophic cultures experience a rise in pigments that was proportional to the increase in biomass concentration (Camacho et al., 1999). Briefly, the increase in antioxidant carotenoids played a crucial role in the regulation of oxidative stress in microalgae, preventing oxidative damage to algal cells. Moreover, one of the most common antioxidants was glycine, which had been used to boost microalgae lipid production (Song et al., 2022).
Moreover, this study proved that glycine treatment increased the concentration of IAA, a plant hormone that promotes growth and development. The concentration of IAA was significantly higher in both strains at the highest glycine concentration. Glycine had a favorable effect on microalgae cell hormone (IAA) levels in Chlorella, which was higher than Synechocystis. Low dosages of IAA enhanced the cell proliferation by triggering genes that promote cell division (González-Garcinuño et al., 2016). It was reported before that, hormones such as IAA, gibberellic acid, and cytokinin-kinetin have a role in increasing the biomass percentages of Chlorella sorokiniana (Guldhe et al., 2019).
Glycine treatment directed algal metabolism toward fatty acid production
In this regard, microalgae prefer glycine as an organic nitrogen source because the metabolic cost is lower than the cost of other nitrogen forms (Mandal et al., 2018). Most microalgae may employ a variety of nitrogen sources, and each source is first converted to ammonium and then metabolized into amino acids via various mechanisms such as glutamine synthetase, glutamate synthase, or NADP glutamate dehydrogenase (Lasa et al., 2002). Moreover, glycine induced an increase in photosynthesis that induces sugar production. Sugars could supply building blocks for the biosynthesis of fatty acids, which are a part of the tricarboxylic acid cycle intermediates. Consistently, the total soluble sugar level increased at low glycine concentrations, but it decreased at the highest concentration, particularly in Chlorella. This decrease may indicate the shift of metabolism toward other primary or secondary metabolites. The results showed that organic acid concentration improved equally in Synechocystis and Chlorella. As a result, this sequence enhanced the generation of organic acids and fatty acids (Cai et al., 2013; Salbitani and Carfagna, 2021). Total fatty acids during our study in Synechocystis and Chlorella significantly boosted compared to the control sample. In agreement, Wang et al. (2016) indicated that Chlorella lipid yield was also upregulated by exogenous glycine treatment at a concentration of 500 mg/L increasing lipid production to 138%. In a previous study by Zhao et al. (2016), photo-chemical modulation enhanced lipid content in Monoraphidium sp. The study reported the largest lipid content of 48.5%, which represented a significant improvement in lipid accumulation compared to baseline levels. One of these enhancements, membrane lipids contain the majority of unsaturated fatty acids, and their primary role was to maintain membrane fluidity under various situations (Zhila et al., 2011).
Glycine-treated Synechocystis and Chlorella are promising sources of bioactive compounds
Microalgae have emerged as a potential feedstock for bioactive compound production due to their high content of essential and unsaturated fatty acids. Chemical environmental modulations can alter microalgae metabolic pathways to accumulate high amounts of neutral lipids from 20–50 percent dry cell weight, primarily in the form of TAGs, in addition to other compounds such as carbohydrates and secondary metabolites (Elsayed et al., 2017). Various methods, such as chemical treatments, growth environment modifications, and genetic engineering, have been employed to induce bioactive primary metabolite production in microalgae (Goh et al., 2019). Whereas researchers aimed to identify microalgae strains capable of high lipid synthesis under stressful conditions. They specifically investigated the lipid content of Chlorella and Synechocystis under temperature stress. Their findings revealed that Chlorella had a lipid content of 6.81E-13 g/cell, while Synechocystis had a slightly higher lipid content of 8.19E-13 g/cell. These studies further supported and confirmed our own results, indicating that cyanobacterium cells accumulated more lipids than chlorophyta cells under glycine stress conditions (Elsayed et al., 2017). Whereas, our findings showed that under glycine stress conditions, cyanobacterium cells (Synechocystis) accumulated more lipids than chlorophyta cells (Chlorella). Glycine-treated Synechocystis and Chlorella are therefore promising feedstocks for biofuel production due to their high lipid accumulation potential.
On utilizing the microalgae on a large industrial scale, we cannot deny the role of pH and temperature on microalgae growth and metabolism, whereas pH strongly affects microalgae enzymatic activities and metabolic pathways. In this context, pH variations had significant implications for biomolecule production as they can either enhance or hinder microbial metabolism (Sassenhagen et al., 2015). While acidic pH conditions can have a detrimental effect on microbial metabolism. Enzyme activity can be inhibited, cellular processes disrupted and metabolic pathways impaired under low pH values (Lund et al., 2014). When NH4Cl was added to Synechocystis cells that were utilizing nitrate as their nitrogen source, there was a rapid decline in growth and cell activity. However, by adjusting the pH and transferring the cells to a medium without ammonium, the initial activity was fully restored after washing the cells (Kolodny et al., 2006). On the other hand, high pH levels can disrupt enzyme stability and function, leading to reduced metabolic activity (Frankenberger Jr and Johanson, 1982). Thus, here to achieve the highest yield of growth and byproducts, we employed the optimal neutral pH for the microalgae culture as reported before by Wang et al. (2010).
Similar to pH, environmental conditions, such as temperature showed a notable impact on the biochemicals production of microalgae. Elevated temperatures during growth had been associated with a significant decrease in protein content, accompanied by increases in lipids and carbohydrates (Renaud et al., 2002). Moreover, different studies have shown that the response of microalgal chemical composition to high and low growth temperatures varies across species. The ideal growth temperatures are those that enable the cells to undergo photosynthesis without altering their inherent biochemical or physiological characteristics (Ras et al., 2013). In the context of Egypt, where microalgae are cultivated outdoors, they must thrive within a wide diurnal temperature range of 25°C–35°C consistently throughout the year. According to Ras et al. (2013) and our preliminary results, the optimal temperature for our specific strains falls within the range of 28°C–30°C.
Overall, our study contributes to the growing body of research on microalgae as a potential feedstock for biofuel production. Additionally, glycine can serve as both a carbon and nitrogen source, making it useful for promoting the heterotrophic growth of microalgae under stressful conditions and increasing biomass production (Yu et al., 2021). Glycine can then be utilized in various metabolic pathways within the cell, such as protein synthesis and photorespiration (Wingler et al., 2000; Ren et al., 2022). In protein synthesis, glycine can serve as one of the building blocks of proteins and can be directly incorporated into the growing polypeptide chain during translation. In photorespiration, glycine is converted into serine with the release of carbon dioxide and ammonia (Keys, 2006).
Glycine uptake can explain the differential response of Synechocystis and Chlorella responded to glycine treatment
Glycine treatment positively changed the growth and biomass besides IAA, organic acid, and amino acids and decreased total soluble sugars in Synechocystis. However, glycine treatment did not significantly affect any notable change in total FA and total soluble sugar levels. Overall, Synechocystis shows a more pronounced effect on various metabolites. While Chlorella shows a more specific effect on amino acids and IAA production. Specific metabolic pathways and physiological characteristics of these two microalgal strains could also play a role in differential response to glycine. The microalgae can uptake the glycine using a transport system that enables direct transportation into the cell, where it can serve as a nitrogen source for the cell (Yu et al., 2021). Here the difference in the rate of glycine uptake between prokaryotic Synechocystis and eukaryotic Chlorella cells may contribute to their different responses to glycine (Gao et al., 2009; Kanwal and De-Eknamkul, 2023). In this regard, some microalgae species have been shown to preferentially utilize glycine over other nitrogen sources such as ammonium and nitrate (Flynn and Butler, 1986; Tyler et al., 2005).
Our growth WM medium contains nitrogen in forms that could potentially affect glycine uptake. In this regard, the study of Gopalakrishnan et al. (2015) that used glycine as a nitrogen source revealed that the majority of the glycine and serine detected in the Chlorella protothecoides intracellular were unlabeled, indicating that these amino acids were primarily taken up from the extracellular medium. Interestingly, nitrogen-limited growth conditions decreased glycine uptake, indicating that nitrogen availability in the growth media improved glycine uptake (Gopalakrishnan et al., 2015).
A noteworthy consequence of using glycine as the nitrogen source was the partial activation of the glyoxylate shunt. Therefore, glycine provided in the extracellular growth medium as a nitrogen source can either be catabolized through glycine dehydrogenase for amino acids production or directed into the glyoxylate shunt for TCA cycle intermediates as shown in (Figure 8) (Gopalakrishnan et al., 2015). Overall, algal metabolism utilizing glycine highlights the significant impact that the nature of the nitrogen source can have on the labeling patterns of intracellular metabolites and metabolite flows.
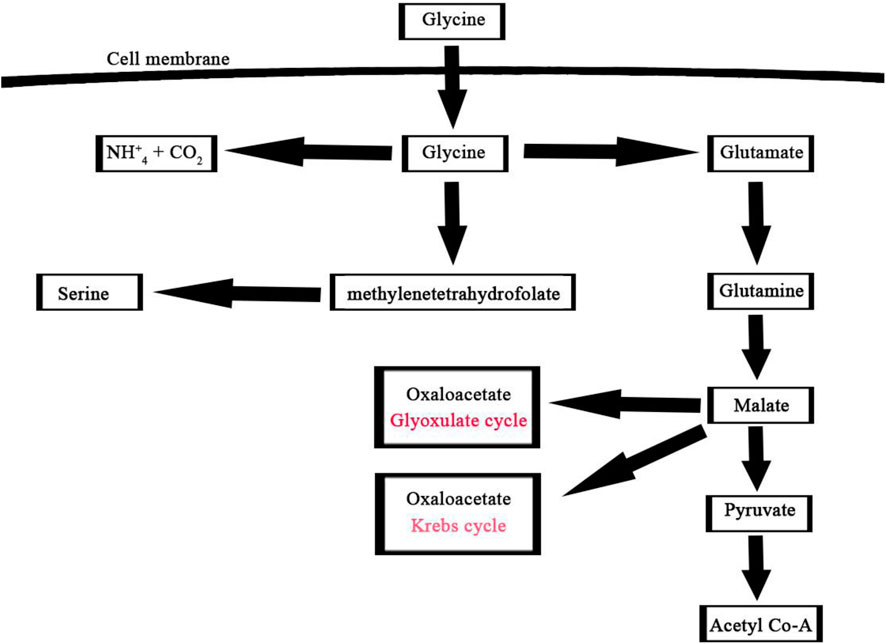
FIGURE 8. Glycine assimilation pathway in microalgae, this pathway illustrates the main three steps involved in the utilization of glycine by microalgae for metabolic processes such as glutamine, methylenetetrahydrofolate, and ammonium pathways.
Conclusion
In a nutshell, the present study explored the effect of glycine on two microalgal strains, Synechocystis and Chlorella, and found that glycine had a positive impact on their growth, photosynthetic pigments, cell hormone levels, organic acid production, amino acid and fatty acid synthesis in microalgae as presented in (Figure 9). Glycine also had a positive effect on the levels of the microalgae cell hormone indole-3-acetic acid. Total soluble sugar was lowered in both strains, but to a greater extent in Chlorella. This study demonstrated the impact of glycine supplementation on the amino acid and fatty acid profiles of Synechocystis and Chlorella. The response to glycine concentration differs between the two strains, with Chlorella showing a significant increase in phenylalanine content at higher glycine concentrations. The findings suggest that glycine supplementation can enhance the production of nonpolar amino acids and potentially increase the potential for bioactive compounds and biodiesel production by altering the fatty acid profiles of these strains. The findings presented in this article have wide-ranging ramifications for the industry. Whereas this information is valuable for microalgae-cultivating industries because it provides insight into the potential use of glycine as a growth stimulant. In addition, the results suggest that glycine supplementation can modify the fatty acid profiles of microalgal strains, thereby potentially boosting the production of bioactive compounds and biodiesel. This has implications for industries focused on biofuel production and those investigating microalgae as a sustainable dietary source. Overall, the study emphasizes the promising potential of glycine supplementation in large-scale microalgae cultivation as a possible alternative to conventional crops for food and biofuel production.
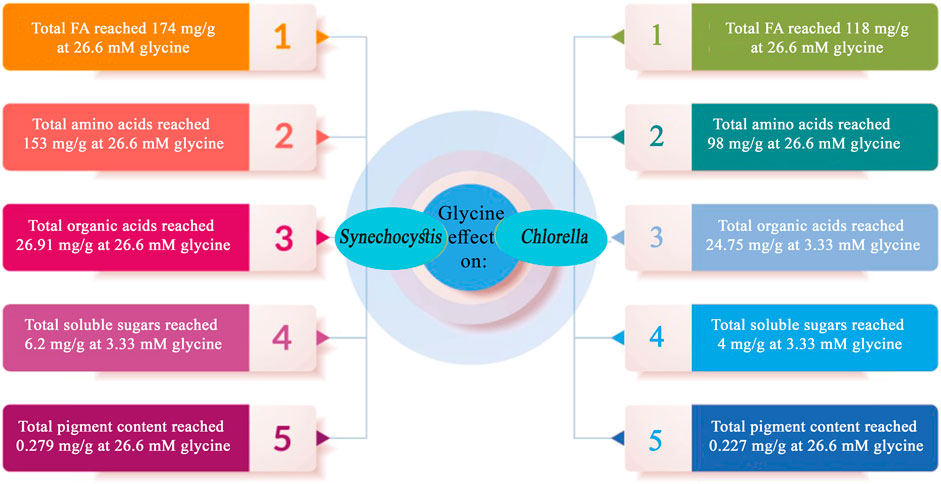
FIGURE 9. Summary of the key findings obtained through the application of external glycine on Synechocystis sp. and Chlorella sp.
Data availability statement
The original contributions presented in the study are included in the article/Supplementary Material, further inquiries can be directed to the corresponding author.
Author contributions
WF: Conceptualization, methodology, investigation, data curation, formal analysis, writing an original draft, visualization, validation. HA: Conceptualization, methodology, investigation, data curation, formal analysis, visualization, validation. EE: Investigation, data curation, formal analysis. SK and ET: Investigation, data curation, formal analysis. MA and OH: Conceptualization, methodology, investigation, data curation, formal analysis, writing-review and editing, supervision, visualization. KE: Conceptualization, methodology, investigation, data curation, formal analysis, writing-original draft, visualization, validation. All authors contributed to the article and approved the submitted version.
Funding
Princess Nourah bint Abdulrahman University Researchers Supporting Project number (PNURSP 2023R214), Princess Nourah bint Abdulrahman University, Riyadh, Saudi Arabia.
Acknowledgments
Princess Nourah bint Abdulrahman University Researchers Supporting Project number (PNURSP2023R214), Princess Nourah bint Abdulrahman University, Riyadh, Saudi Arabia.
Conflict of interest
The authors declare that the research was conducted in the absence of any commercial or financial relationships that could be construed as a potential conflict of interest.
Publisher’s note
All claims expressed in this article are solely those of the authors and do not necessarily represent those of their affiliated organizations, or those of the publisher, the editors and the reviewers. Any product that may be evaluated in this article, or claim that may be made by its manufacturer, is not guaranteed or endorsed by the publisher.
Supplementary material
The Supplementary Material for this article can be found online at: https://www.frontiersin.org/articles/10.3389/fbioe.2023.1161911/full#supplementary-material
References
AbdElgawad, H., Abuelsoud, W., Madany, M. M. Y., Selim, S., Zinta, G., Mousa, A. S. M., et al. (2020). Actinomycetes enrich soil rhizosphere and improve seed quality as well as productivity of legumes by boosting nitrogen availability and metabolism. Biomolecules 10, 1675. doi:10.3390/biom10121675
Al Jaouni, S., Saleh, A. M., Wadaan, M. A., Hozzein, W. N., Selim, S., and AbdElgawad, H. (2018). Elevated CO2 induces a global metabolic change in basil (Ocimum basilicum L) and peppermint (Mentha piperita L) and improves their biological activity. J. plant physiology 224, 121–131. doi:10.1016/j.jplph.2018.03.016
Almomani, F., Hosseinzadeh-Bandbafha, H., Aghbashlo, M., Omar, A., Joo, S.-W., Vasseghian, Y., et al. (2023). Comprehensive insights into conversion of microalgae to feed, food, and biofuels: Current status and key challenges towards implementation of sustainable biorefineries. Chem. Eng. J. 455, 140588. doi:10.1016/j.cej.2022.140588
Bowden, N. A., Sanders, J. P. M., and Bruins, M. E. (2018). Solubility of the proteinogenic α-amino acids in water, ethanol, and ethanol–water mixtures. J. Chem. Eng. Data 63, 488–497. doi:10.1021/acs.jced.7b00486
Cai, T., Park, S. Y., and Li, Y. (2013). Nutrient recovery from wastewater streams by microalgae: Status and prospects. Renew. Sustain. Energy Rev. 19, 360–369. doi:10.1016/j.rser.2012.11.030
Camacho, F. G., Gómez, A. C., Fernández, F. A., Sevilla, J. F., and Grima, E. M. (1999). Use of concentric-tube airlift photobioreactors for microalgal outdoor mass cultures. Enzyme Microb. Technol. 24, 164–172. doi:10.1016/s0141-0229(98)00103-3
Chittora, D., Meena, M., Barupal, T., Swapnil, P., and Sharma, K. (2020). Cyanobacteria as a source of biofertilizers for sustainable agriculture. Biochem. biophysics Rep. 22, 100737. doi:10.1016/j.bbrep.2020.100737
Cuellar-Bermudez, S. P., Garcia-Perez, J. S., Rittmann, B. E., and Parra-Saldivar, R. (2015). Photosynthetic bioenergy utilizing CO2: An approach on flue gases utilization for third generation biofuels. J. Clean. Prod. 98, 53–65. doi:10.1016/j.jclepro.2014.03.034
Dantas, D. M., Oliveira, C. Y. B., Costa, R. M. P. B., Carneiro-da-Cunha, M., Gálvez, A. O., and Bezerra, R. D. S. (2019). Evaluation of antioxidant and antibacterial capacity of green microalgae Scenedesmus subspicatus. Food Sci. Technol. Int. 25, 318–326. doi:10.1177/1082013218825024
De Bhowmick, G., Koduru, L., and Sen, R. (2015). Metabolic pathway engineering towards enhancing microalgal lipid biosynthesis for biofuel application—A review. Renew. Sustain. Energy Rev. 50, 1239–1253. doi:10.1016/j.rser.2015.04.131
De la Hoz Siegler, H., Ben-Zvi, A., Burrell, R. E., and McCaffrey, W. C. (2011). The dynamics of heterotrophic algal cultures. Bioresour. Technol. 102, 5764–5774. doi:10.1016/j.biortech.2011.01.081
Doan, T. T. Y., Sivaloganathan, B., and Obbard, J. P. (2011). Screening of marine microalgae for biodiesel feedstock. Biomass Bioenergy 35, 2534–2544. doi:10.1016/j.biombioe.2011.02.021
Elsayed, K. N. M., Kolesnikova, T. A., Noke, A., and Klöck, G. (2017). Imaging the accumulated intracellular microalgal lipids as a response to temperature stress. 3 Biotech. 7, 41. doi:10.1007/s13205-017-0677-x
Fabregas, J., Herrero, C., Cabezas, B., and Abalde, J. (1986). Biomass production and biochemical composition in mass cultures of the marine microalga Isochrysis galbana Parke at varying nutrient concentrations. Aquaculture 53, 101–113. doi:10.1016/0044-8486(86)90280-2
Fathy, W., Elsayed, K., Essawy, E., Tawfik, E., Zaki, A., Abdelhameed, M., et al. (2020). Biosynthesis of silver nanoparticles from Synechocystis sp. to be used as a flocculant agent with different microalgae strains. Curr. Nanomater. 5, 175–187. doi:10.2174/2468187310999200605161200
Fathy, W., Essawy, E., Tawfik, E., Khedr, M., Abdelhameed, M. S., Hammouda, O., et al. (2021). Recombinant overexpression of the Escherichia coli acetyl-CoA carboxylase gene in Synechocystis sp. boosts lipid production. J. Basic Microbiol. 61, 330–338. doi:10.1002/jobm.202000656
Flynn, K. J., and Butler, I. (1986). Nitrogen sources for the growth of marine microalgae: Role of dissolved free amino acids. Mar. Ecol. Prog. Ser. 34, 281–304. doi:10.3354/meps034281
Frankenberger, W., and Johanson, J. (1982). Effect of pH on enzyme stability in soils. Soil Biol. Biochem. 14, 433–437. doi:10.1016/0038-0717(82)90101-8
Gao, Y., Cui, Y., Xiong, W., Li, X., and Wu, Q. (2009). Effect of UV-C on algal evolution and differences in growth rate, pigmentation and photosynthesis between prokaryotic and eukaryotic algae. Photochem. Photobiol. 85, 774–782. doi:10.1111/j.1751-1097.2008.00493.x
Gao, L., Ding, W., Xi, J., Gao, S., Zhou, X., Chen, Y., et al. (2023). Effects of different nitrogen/phosphorus ratios on the growth and metabolism of microalgae Scenedesmus obliquus cultured in the mixed wastewater from primary settling tank and sludge thickener. Process Saf. Environ. Prot. 170, 824–833. doi:10.1016/j.psep.2022.12.059
Garcıa, M. C., Mirón, A. S., Sevilla, J. F., Grima, E. M., and Camacho, F. G. (2005). Mixotrophic growth of the microalga phaeodactylum tricornutum: Influence of different nitrogen and organic carbon sources on productivity and biomass composition. Process Biochem. 40, 297–305. doi:10.1016/j.procbio.2004.01.016
Goh, B. H. H., Ong, H. C., Cheah, M. Y., Chen, W.-H., Yu, K. L., and Mahlia, T. M. I. (2019). Sustainability of direct biodiesel synthesis from microalgae biomass: A critical review. Renew. Sustain. Energy Rev. 107, 59–74. doi:10.1016/j.rser.2019.02.012
González-Garcinuño, Á., Sánchez-Álvarez, J. M., Galán, M. A., and Martin del Valle, E. M. (2016). Understanding and optimizing the addition of phytohormones in the culture of microalgae for lipid production. Biotechnol. Prog. 32, 1203–1211. doi:10.1002/btpr.2312
Gopalakrishnan, S., Baker, J., Kristoffersen, L., and Betenbaugh, M. J. (2015). Redistribution of metabolic fluxes in Chlorella protothecoides by variation of media nitrogen concentration. Metab. Eng. Commun. 2, 124–131. doi:10.1016/j.meteno.2015.09.004
Guedes, A. C., Amaro, H. M., and Malcata, F. X. (2011). Microalgae as sources of high added-value compounds—A brief review of recent work. Biotechnol. Prog. 27, 597–613. doi:10.1002/btpr.575
Guldhe, A., Renuka, N., Singh, P., and Bux, F. (2019). Effect of phytohormones from different classes on gene expression of Chlorella sorokiniana under nitrogen limitation for enhanced biomass and lipid production. Algal Res. 40, 101518. doi:10.1016/j.algal.2019.101518
Harun, R., Singh, M., Forde, G. M., and Danquah, M. K. (2010). Bioprocess engineering of microalgae to produce a variety of consumer products. Renew. Sustain. energy Rev. 14, 1037–1047. doi:10.1016/j.rser.2009.11.004
Jin, L., Junchao, H., and Feng, C. (2011). “Microalgae as feedstocks for biodiesel production,” in Biodiesel. IntechOpen, rijeka. Editors S. Margarita, and M. Gisela, 7.
Kanwal, S., and De-Eknamkul, W. (2023). A non-functional γ-aminobutyric acid shunt pathway in cyanobacterium Synechocystis sp. PCC 6803 enhances δ-aminolevulinic acid accumulation under modified nutrient conditions. Int. J. Mol. Sci. 24, 1213. doi:10.3390/ijms24021213
Keys, A. J. (2006). The re-assimilation of ammonia produced by photorespirationand the nitrogen economy of C3 higher plants. Photosynth. Res. 87, 165–175. doi:10.1007/s11120-005-9024-x
Khan, M. I., Shin, J. H., and Kim, J. D. (2018). The promising future of microalgae: Current status, challenges, and optimization of a sustainable and renewable industry for biofuels, feed, and other products. Microb. Cell. factories 17, 36–21. doi:10.1186/s12934-018-0879-x
Kim, G., Mujtaba, G., and Lee, K. (2016). Effects of nitrogen sources on cell growth and biochemical composition of marine chlorophyte Tetraselmis sp. for lipid production. Algae 31, 257–266. doi:10.4490/algae.2016.31.8.18
Kolodny, N. H., Bauer, D., Bryce, K., Klucevsek, K., Lane, A., Medeiros, L., et al. (2006). Effect of nitrogen source on cyanophycin synthesis in Synechocystis sp. strain PCC 6308. J. Bacteriol. 188, 934–940. doi:10.1128/jb.188.3.934-940.2006
Kozan, D. W., Derrick, J. T., Ludington, W. B., and Farber, S. A. (2023). From worms to humans: Understanding intestinal lipid metabolism via model organisms. Biochimica Biophysica Acta (BBA)-Molecular Cell. Biol. Lipids 1868, 159290. doi:10.1016/j.bbalip.2023.159290
Krzemińska, I., Pawlik-Skowrońska, B., Trzcińska, M., and Tys, J. (2014). Influence of photoperiods on the growth rate and biomass productivity of green microalgae. Bioprocess Biosyst. Eng. 37, 735–741. doi:10.1007/s00449-013-1044-x
Kumar, V., Muthuraj, M., Palabhanvi, B., Ghoshal, A. K., and Das, D. (2014). High cell density lipid rich cultivation of a novel microalgal isolate Chlorella sorokiniana FC6 IITG in a single-stage fed-batch mode under mixotrophic condition. Bioresour. Technol. 170, 115–124. doi:10.1016/j.biortech.2014.07.066
Lasa, B., Frechilla, S., Aparicio-Tejo, P. M., and Lamsfus, C. (2002). Role of glutamate dehydrogenase and phosphoenolpyruvate carboxylase activity in ammonium nutrition tolerance in roots. Plant Physiology Biochem. 40, 969–976. doi:10.1016/s0981-9428(02)01451-1
Liu, Y., Zhan, J.-j., and Hong, Y. (2017). Effects of metal ions on the cultivation of an oleaginous microalga Chlorella sp. Environ. Sci. Pollut. Res. 24, 26594–26604. doi:10.1007/s11356-017-0258-x
Lund, P., Tramonti, A., and De Biase, D. (2014). Coping with low pH: Molecular strategies in neutralophilic bacteria. FEMS Microbiol. Rev. 38, 1091–1125. doi:10.1111/1574-6976.12076
Maity, J. P., Bundschuh, J., Chen, C.-Y., and Bhattacharya, P. (2014). Microalgae for third generation biofuel production, mitigation of greenhouse gas emissions and wastewater treatment: Present and future perspectives–A mini review. Energy 78, 104–113. doi:10.1016/j.energy.2014.04.003
Maltsev, Y., and Maltseva, K. (2021). Fatty acids of microalgae: Diversity and applications. Rev. Environ. Sci. Bio/Technology 20, 515–547. doi:10.1007/s11157-021-09571-3
Mandal, S., Shurin, J. B., Efroymson, R. A., and Mathews, T. J. (2018). Functional divergence in nitrogen uptake rates explains diversity–productivity relationship in microalgal communities. Ecosphere 9, e02228. doi:10.1002/ecs2.2228
Matantseva, O., Pozdnyakov, I., Voss, M., Liskow, I., and Skarlato, S. (2018). The uncoupled assimilation of carbon and nitrogen from urea and Glycine by the bloom-forming dinoflagellate prorocentrum minimum. Protist 169, 603–614. doi:10.1016/j.protis.2018.05.006
Mérida, A., Candau, P., and Florencio, F. J. (1991). Regulation of glutamine synthetase activity in the unicellular cyanobacterium Synechocystis sp. strain PCC 6803 by the nitrogen source: Effect of ammonium. J. Bacteriol. 173, 4095–4100. doi:10.1128/jb.173.13.4095-4100.1991
Metzner, H., Rau, H., and Senger, H. (1965). Untersuchungen zur synchronisierbarkeit einzelner pigmentmangel-mutanten von Chlorella. Planta 65, 186–194. doi:10.1007/bf00384998
Michalak, I., and Chojnacka, K. (2014). Algal extracts: Technology and advances. Eng. Life Sci. 14, 581–591. doi:10.1002/elsc.201400139
Moran, R., and Porath, D. (1980). Chlorophyll determination in intact tissues using N,N-Dimethylformamide. Plant Physiol. 65, 478–479. doi:10.1104/pp.65.3.478
Nateghpour, B., Kavoosi, G., and Mirakhorli, N. (2021). Amino acid profile of the peel of three citrus species and its effect on the combination of amino acids and fatty acids Chlorella vulgaris. J. Food Compos. Analysis 98, 103808. doi:10.1016/j.jfca.2021.103808
Norici, A., Dalsass, A., and Giordano, M. (2002). Role of phosphoenolpyruvate carboxylase in anaplerosis in the green microalga Dunaliella salina cultured under different nitrogen regimes. Physiol. Plant. 116, 186–191. doi:10.1034/j.1399-3054.2002.1160207.x
Pflanz, M., and Zude, M. (2008). Spectrophotometric analyses of chlorophyll and single carotenoids during fruit development of tomato (Solanum lycopersicum L) by means of iterative multiple linear regression analysis. Appl. Opt. 47, 5961–5970. doi:10.1364/ao.47.005961
Rahman, K. M. (2020). Food and high value products from microalgae: Market opportunities and challenges. Microalgae Biotechnol. food, health high value Prod., 3–27. doi:10.1007/978-981-15-0169-2_1
Ras, M., Steyer, J.-P., and Bernard, O. (2013). Temperature effect on microalgae: A crucial factor for outdoor production. Rev. Environ. Sci. Bio/Technology 12, 153–164. doi:10.1007/s11157-013-9310-6
Ren, J., Wang, W., Nie, J., Yuan, W., and Zeng, A.-P. (2022). “Understanding and engineering Glycine cleavage system and related metabolic pathways for C1-based biosynthesis,” in One-carbon feedstocks for sustainable bioproduction. Editors A.-P. Zeng, and N. J. Claassens (Cham: Springer International Publishing), 273–298.
Renaud, S. M., Thinh, L.-V., Lambrinidis, G., and Parry, D. L. (2002). Effect of temperature on growth, chemical composition and fatty acid composition of tropical Australian microalgae grown in batch cultures. Aquaculture 211, 195–214. doi:10.1016/s0044-8486(01)00875-4
Salbitani, G., and Carfagna, S. (2021). Ammonium utilization in microalgae: A sustainable method for wastewater treatment. Sustainability 13, 956. doi:10.3390/su13020956
Santhosh, S., Dhandapani, R., and Hemalatha, N. (2016). Bioactive compounds from Microalgae and its different applications-a review. Adv. Appl. Sci. Res. 7, 153–158.
Sarma, S., Sharma, S., Patel, A., Upadhyay, J., Rathod, V., and Narra, M. (2023). Statistical optimization of microalgal biodiesel production and protein extraction from Chlorella sorokiniana cultivated in dairy effluent. Mater. Today Proc. 72, 2731–2740. doi:10.1016/j.matpr.2022.09.512
Sassenhagen, I., Wilken, S., Godhe, A., and Rengefors, K. (2015). Phenotypic plasticity and differentiation in an invasive freshwater microalga. Harmful Algae 41, 38–45. doi:10.1016/j.hal.2014.11.001
Sinha, A. K., Giblen, T., AbdElgawad, H., De Rop, M., Asard, H., Blust, R., et al. (2013). Regulation of amino acid metabolism as a defensive strategy in the brain of three freshwater teleosts in response to high environmental ammonia exposure. Aquat. Toxicol. 130-131, 86–96. doi:10.1016/j.aquatox.2013.01.003
Song, X., Liu, B.-F., Kong, F., Ren, N.-Q., and Ren, H.-Y. (2022). Overview on stress-induced strategies for enhanced microalgae lipid production: Application, mechanisms and challenges. Resour. Conservation Recycl. 183, 106355. doi:10.1016/j.resconrec.2022.106355
Song, X., Liu, B.-F., Kong, F., Song, Q., Ren, N.-Q., and Ren, H.-Y. (2023). Lipid accumulation by a novel microalga Parachlorella kessleri R-3 with wide pH tolerance for promising biodiesel production. Algal Res. 69, 102925. doi:10.1016/j.algal.2022.102925
Sun, Z., Chen, Y.-F., and Du, J. (2016). Elevated CO2 improves lipid accumulation by increasing carbon metabolism in Chlorella sorokiniana. Plant Biotechnol. J. 14, 557–566. doi:10.1111/pbi.12398
Tabatabaei, M., Tohidfar, M., Jouzani, G. S., Safarnejad, M., and Pazouki, M. (2011). Biodiesel production from genetically engineered microalgae: Future of bioenergy in Iran. Renew. Sustain. Energy Rev. 15, 1918–1927. doi:10.1016/j.rser.2010.12.004
Tyler, A. C., McGlathery, K. J., and Macko, S. A. (2005). Uptake of urea and amino acids by the macroalgae Ulva lactuca (Chlorophyta) and Gracilaria vermiculophylla (Rhodophyta). Mar. Ecol. Prog. Ser. 294, 161–172. doi:10.3354/meps294161
van Handel, E. (1968). Direct microdetermination of sucrose. Anal. Biochem. 22, 280–283. doi:10.1016/0003-2697(68)90317-5
Wan, M. X., Wang, R. M., Xia, J. L., Rosenberg, J. N., Nie, Z. Y., Kobayashi, N., et al. (2012). Physiological evaluation of a new Chlorella sorokiniana isolate for its biomass production and lipid accumulation in photoautotrophic and heterotrophic cultures. Biotechnol. Bioeng. 109, 1958–1964. doi:10.1002/bit.24477
Wang, C., Li, H., Wang, Q., and Wei, P. (2010). [Effect of pH on growth and lipid content of Chlorella vulgaris cultured in biogas slurry]. Sheng Wu Gong Cheng Xue Bao 26, 1074–1079.
Wang, J., Sommerfeld, M. R., Lu, C., and Hu, Q. (2013). Combined effect of initial biomass density and nitrogen concentration on growth and astaxanthin production of Haematococcus pluvialis (Chlorophyta) in outdoor cultivation. Algae 28, 193–202. doi:10.4490/algae.2013.28.2.193
Wang, Y., He, B., Sun, Z., and Chen, Y.-F. (2016). Chemically enhanced lipid production from microalgae under low sub-optimal temperature. Algal Res. 16, 20–27. doi:10.1016/j.algal.2016.02.022
Winckelmann, D., Bleeke, F., Bergmann, P., and Klöck, G., 2015. Growth of Cyanobacterium aponinum influenced by increasing salt concentrations and temperature. 3 Biotech. 5, 253–260. doi:10.1007/s13205-014-0224-y
Wingler, A., Lea, P. J., Quick, W. P., and Leegood, R. C. (2000). Photorespiration: Metabolic pathways and their role in stress protection. Philosophical Trans. R. Soc. Lond. Ser. B Biol. Sci. 355, 1517–1529. doi:10.1098/rstb.2000.0712
Yaakob, Z., Ali, E., Zainal, A., Mohamad, M., and Takriff, M. S. (2014). An overview: Biomolecules from microalgae for animal feed and aquaculture. J. Biol. Research-Thessaloniki 21, 6–10. doi:10.1186/2241-5793-21-6
Yarkent, Ç., Gürlek, C., and Oncel, S. S. (2020). Potential of microalgal compounds in trending natural cosmetics: A review. Sustain. Chem. Pharm. 17, 100304. doi:10.1016/j.scp.2020.100304
Yemm, E., and Willis, A. (1954). The estimation of carbohydrates in plant extracts by anthrone. Biochem. J. 57, 508–514. doi:10.1042/bj0570508
Yin, Z., Zhu, L., Li, S., Hu, T., Chu, R., Mo, F., et al. (2020). A comprehensive review on cultivation and harvesting of microalgae for biodiesel production: Environmental pollution control and future directions. Bioresour. Technol. 301, 122804. doi:10.1016/j.biortech.2020.122804
Yu, Y., You, L., Liu, D., Hollinshead, W., Tang, Y. J., and Zhang, F. (2013). Development of Synechocystis sp. PCC 6803 as a phototrophic cell factory. Mar. Drugs 11, 2894–2916. doi:10.3390/md11082894
Yu, Q., He, J., Zhao, Q., Wang, X., Zhi, Y., Li, X., et al. (2021). Regulation of nitrogen source for enhanced photobiological H2 production by co-culture of Chlamydomonas reinhardtii and Mesorhizobium sangaii. Algal Res. 58, 102422. doi:10.1016/j.algal.2021.102422
Yue, H., Zhao, C., Yang, S., and Jia, Y. (2021). Effects of glycine on cell growth and pigment biosynthesis in Rhodobacter azotoformans. J. basic Microbiol. 61, 63–73. doi:10.1002/jobm.202000503
Zhao, Y., Li, D., Ding, K., Che, R., Xu, J.-W., Zhao, P., et al. (2016). Production of biomass and lipids by the oleaginous microalgae Monoraphidium sp. QLY-1 through heterotrophic cultivation and photo-chemical modulator induction. Bioresour. Technol. 211, 669–676. doi:10.1016/j.biortech.2016.03.160
Keywords: microalgae, mixotrophic medium, glycine, biochemical composition, fatty acid profile
Citation: Fathy WA, AbdElgawad H, Essawy EA, Tawfik E, Abdelhameed MS, Hammouda O, Korany SM and Elsayed KNM (2023) Glycine differentially improved the growth and biochemical composition of Synechocystis sp. PAK13 and Chlorella variabilis DT025. Front. Bioeng. Biotechnol. 11:1161911. doi: 10.3389/fbioe.2023.1161911
Received: 09 February 2023; Accepted: 23 May 2023;
Published: 01 June 2023.
Edited by:
Hyun-Dong Shin, Bereum Co., Ltd., Republic of KoreaReviewed by:
Mostafa Elshobary, Tanta University, EgyptRanjith Kumar Manoharan, Yeungnam University, Republic of Korea
Copyright © 2023 Fathy, AbdElgawad, Essawy, Tawfik, Abdelhameed, Hammouda, Korany and Elsayed. This is an open-access article distributed under the terms of the Creative Commons Attribution License (CC BY). The use, distribution or reproduction in other forums is permitted, provided the original author(s) and the copyright owner(s) are credited and that the original publication in this journal is cited, in accordance with accepted academic practice. No use, distribution or reproduction is permitted which does not comply with these terms.
*Correspondence: Wael A. Fathy, d2FlbC5haG1lZEBzY2llbmNlLmJzdS5lZHUuZWc=