- 1Department of Agrochemistry, Environmental Microbiology and Soil Conservation, Institute of Natural Resources and Agrobiology of Seville, Spanish National Research Council (IRNAS-CSIC), Seville, Spain
- 2Department of Microbiology and Parasitology, Faculty of Pharmacy, University of Seville, Seville, Spain
- 3Department of Biotechnology and Biosciences, University of Milano-Bicocca, Milano, Italy
Phenanthrene (PHE) is a highly toxic compound, widely present in soils. For this reason, it is essential to remove PHE from the environment. Stenotrophomonas indicatrix CPHE1 was isolated from an industrial soil contaminated by polycyclic aromatic hydrocarbons (PAHs) and was sequenced to identify the PHE degrading genes. Dioxygenase, monooxygenase, and dehydrogenase gene products annotated in S. indicatrix CPHE1 genome were clustered into different trees with reference proteins. Moreover, S. indicatrix CPHE1 whole-genome sequences were compared to genes of PAHs-degrading bacteria retrieved from databases and literature. On these basis, reverse transcriptase-polymerase chain reaction (RT-PCR) analysis pointed out that cysteine dioxygenase (cysDO), biphenyl-2,3-diol 1,2-dioxygenase (bphC), and aldolase hydratase (phdG) were expressed only in the presence of PHE. Therefore, different techniques have been designed to improve the PHE mineralization process in five PHE artificially contaminated soils (50 mg kg−1), including biostimulation, adding a nutrient solution (NS), bioaugmentation, inoculating S. indicatrix CPHE1 which was selected for its PHE-degrading genes, and the use of 2-hydroxypropyl-β-cyclodextrin (HPBCD) as a bioavailability enhancer. High percentages of PHE mineralization were achieved for the studied soils. Depending on the soil, different treatments resulted to be successful; in the case of a clay loam soil, the best strategy was the inoculation of S. indicatrix CPHE1 and NS (59.9% mineralized after 120 days). In sandy soils (CR and R soils) the highest percentage of mineralization was achieved in presence of HPBCD and NS (87.3% and 61.3%, respectively). However, the combination of CPHE1 strain, HPBCD, and NS showed to be the most efficient strategy for sandy and sandy loam soils (LL and ALC soils showed 35% and 74.6%, respectively). The results indicated a high degree of correlation between gene expression and the rates of mineralization.
1 Introduction
Phenanthrene (PHE) is a low molecular weight polycyclic aromatic hydrocarbon (PAH) constituted by three fused benzene rings. It is mainly produced from anthropogenic sources such as oil mining, accidental discharge, pipeline vandalism, and poor municipal waste management causing adverse effects on public health and the environment (Prabhu and Phale, 2003; Varjani and Upasani, 2017). PHE is characterized by its ubiquity in the environment, and it is a pollutant of concern due to its persistence and toxicity, causing detrimental biological and environmental effects (Abdel-Shafy and Mansour, 2016); indeed, it has been included in the hazardous substances lists of the United States Environmental Protection Agency and the European Union (EPA, 2022).
In the environment, PAHs are removed in different ways such as photo-oxidation, chemical oxidation, bioaccumulation, volatilization, or adsorption to soil particles (Parab and Phadke, 2020). However, biological strategy is the main approach to removing PAHs in terrestrial and aquatic systems (Churchill et al., 2008; Nwankwegu et al., 2022), since microbial degradation is a cost-effective and eco-friendly technology (Brown et al., 2017).
Several studies showed PHE biodegradation in soil fostered by the presence of different bacterial genera: Bacillus (Mandree et al., 2021), Massilia (Gu et al., 2021), Sphingomonas (Waigi et al., 2015), or Rhodococcus (Wang et al., 2021). Additionally, bacterial strains from Stenotrophomonas genus have been described as PHE degraders (Kumari et al., 2017; Elufisan et al., 2020), such as S. maltophilia (Gao et al., 2013; Zafra et al., 2014; Xiao et al., 2021). However, as far as we know, the study of Lara-Moreno et al. (2021) was the first which demonstrated the ability of S. indicatrix to degrade PHE in solution. Stenotrophomonas indicatrix is a Gram-negative species that can be isolated from sunflower roots (Adeleke et al., 2021), soils (Lara-Moreno et al., 2021; Lozano-Andrade et al., 2021), oligotrophic water ponds (Friedrich et al., 2021), or from food-associated sources (Weber et al., 2018).
The degradation of PAH by bacteria is usually catalyzed by oxygenase and dehydrogenase enzymes. For instance, these enzymes include naphthalene dioxygenase (NahAc) and naphthalene monooxygenase (NdoB) (Pseudomonas putida G7, Cébron et al., 2008); pyrene dioxygenase (Mycobacterium sp. strain PYR-1, Stingley et al., 2004); dihydrodiol naphthalene dehydrogenase (NahB) (Pseudomonas stutzeri AN10, Bosch et al., 1999) or naphthalene dehydrogenase (NahB) (P. putida AK5, Izmalkova et al., 2013). Nevertheless, little is known about the genes responsible for PHE degradation in Stenotrophomonas genus. As far as we know, only Kumari et al. (2017) and Elufisan et al. (2020) have conducted research about the genes involved in PAHs biodegradation pathway in Stenotrophomonas genus and there are no scientific studies on Stenotrophomonas indicatrix species.
In the environment, the natural ability of soil is often exploited to remove contaminants. However, since natural attenuation is a very slow biological process for the restoration of contaminated soils (Zabbey et al., 2017), (bio)remediation techniques can be applied, including biostimulation, application of availability enhancers, and bioaugmentation. Biostimulation requires the addition of nutrients or electron acceptors into the soil. This option optimizes the strategy and critical factors for the remediation of polluted sites, including restricted oxygen, nutrient transfer, and competition between microorganisms (Masy et al., 2016). Regarding availability enhancers, PHE is considered a pollutant highly persistent in soil due to its strongly hydrophobic character (Humel et al., 2020). Therefore, the high adsorption could cause a decline in the PHE bioavailable fraction, which means a slowdown in the bioremediation progress (Humel et al., 2017). To counter these negative influences, bioavailability enhancers such as cyclodextrins (CDs), can be applied to the soil (Madrid et al., 2019; Madrid et al., 2022). The use of CDs is a greener alternative to organic solvents or synthetic surfactants used by several authors to remediate contaminated soils by PAHs (Sun et al., 2014; Liu et al., 2018). CDs have the capacity to form inclusion complexes with hydrophobic contaminants enhancing their water solubility and improving their elimination from soils (Morillo et al., 2020).
Bioaugmentation is considered a promising tool to enhance pollutant biodegradation. Bioaugmentation consists of the addition of exogenous or endogenous pollutant degraders into the contaminated site to accelerate contaminant removal (Tyagi et al., 2011). It has been recommended as the most suitable alternative to be used in bioremediation (Nwankwegu et al., 2022), using bacterial strains isolated from large-scale polluted areas where endogenous microbiota is generally adapted (Mrozik and Piotrowska-Seget, 2010). For this reason, in the present study, a bacterial strain isolated in our lab from highly contaminated industrial soil was selected for its capacity to degrade and mineralize PHE in an aqueous solution (Lara-Moreno et al., 2021). In the previous paper, the isolated bacterial strain was classified as Stenotrophomonas maltophilia CPHE1 (NCBI number: MT138842), but later, a more in-depth genetic study revealed that its classification corresponded to S. indicatrix (Lara-Moreno et al., 2023). Weber et al. (2018) classified for the first time this species as S. indicatrix, although they had previously classified it also as S. maltophilia due to their high genomic similarities. Up to now, no work has shown PHE degradation and mineralization to CO2 and H20 in the soil in the sole presence of S. indicatrix.
Therefore, this study aimed to characterize S. indicatrix CPHE1 at the genomic level to classify genes annotated as dioxygenases, monooxygenases, and dehydrogenases into phylogenetic trees to detect putative PHE-degrading genes. Moreover, S. indicatrix CPHE1 whole-genome sequences were compared to genes of PAHs-degrading bacteria retrieved from databases and literature. The expression of selected genes such as bphC, and phdG genes (encoding for an extradiol dioxygenase, and a hydratase-aldolase) was studied by RT-PCR for their involvement in the PHE degradation pathway. On this basis, several eco-friendly bioremediation treatments were conducted using biostimulation, bioaugmentation with S. indicatrix, and the use of CDs to enhance the extent and rate of PHE biodegradation and mineralization in five contaminated soils with different physicochemical properties.
2 Materials and methods
2.1 Materials
PHE (C14H10, purity 98%) was purchased from Sigma-Aldrich (Madrid, Spain) and radiolabeled compound 14C-PHE (36 mCi⋅mmol−1, purity 99.9%, and radiochemical purity 100%) was acquired from the Institute of Isotopes (Budapest, Hungary). HPBCD was obtained from CycloLab Cyclodextrin Research and Development Laboratory Ltd. (Budapest, Hungary).
Mineral Salt Medium (MSM) composition (g L−1): 4.0 Na2HPO4; 2.0 KH2PO4; 0.8 MgSO4; 0.8 NH4SO4. The micronutrients solution (SNs) was composed of (mg L-1): 12.5 NiCl2 6H2O; 25.0 SnCl2 2H2O; 12.5 ZnSO4 7H2O; 12.5 Al2(SO4)3 18H2O; 75.0 MnCl2 4H2O; 12.5 CoCl2 2H2O; 37.5 FeSO4 7H2O; 10.0 CaSO4 2H2O; 3.75 KBr; 3.75 KCl; 2.50 LiCl (Fenlon et al., 2011). The mixture of MSM and SNs (50:1) was named NS in the study.
Five soils (PLD, LL, ALC, CR, R) with diverse physicochemical properties were collected from different points in the South of Spain. PLD soil is an agricultural soil located in Los Palacios y Villafranca—Seville (37°10′20.0″N 5°55′21.9″W), where wheat, cereals, and vineyard crops are mainly cultivated. The agricultural soil LL from Vejer de la Frontera—Cádiz (36°17′52.6″N 5°52′45.2″W) is devoted to intensive agriculture of carrot, cotton, leek, etc. ALC soil was taken from Alcornocales Natural Park (36°20′54″N 5°36′14″O) and is characterized by high organic matter (OM) content. CR soil is an agricultural soil from an area of olives within the experimental farm La HAMPA (IRNAS-CSIC) (37°17′28.3″N, 6°3′55.4″W). R soil, collected from a palm trees zone in Conil de la Frontera—Cádiz (36°18′32.4″N, 6°08′58.6″W), has been managed with a huge number of insecticides. The soil samples collection was conducted from the superficial horizon (0–20 cm), air-dried for 24 h at room temperature, and sieved (2 mm). Table 1S shows the physicochemical properties of the studied soils and their textural classification. The pH was determined in a proportion of 1:2.5 soil/water extract. The particle size distribution was evaluated using Bouyoucos densimeter; the calcination or muffling method was used to estimate the OM content of the soil weight loss on ignition (LOI) or calcination, the quantification of OM was determined by K2Cr2O7 oxidation, and the manometric method was used to measure the total carbonate content.
The PHE-degrading microorganism selected, S. indicatrix CPHE1, corresponds to S. maltophilia CPHE1 (NCBI number: MT138842) published by Lara-Moreno et al. (2021). Subsequent studies indicated greater phylogenetic closeness with the species S. indicatrix rather than S. maltophilia (Lara-Moreno et al., 2023). CPHE1 strain was isolated in our lab from a highly contaminated industrial soil as reported by Lara-Moreno et al. (2021). The sequencing data of S. indicatrix CPHE1 were deposited in the National Center for Biotechnology Information (NCBI), under the BioProject ID PRJNA868539. The whole genome shotgun generated has been deposited in DDBJ/ENA/GenBank under the accession number JANQDV000000000. The version described in this paper is version JANQDV010000000. The raw data was deposited in the Sequence Read Archive (SRA) under the accession number SRR21098193 (Lara-Moreno et al., 2023).
2.2 Methods
2.2.1 Bioinformatic analysis: nucleotide and protein sequence analysis
The sequences annotated as monooxygenases, dioxygenases and dehydrogenases were individually aligned against PDB (Protein Data Bank) database (Berman et al., 2000) and BLASTp of NCBI pipeline to identify reference sequences. References proteins were selected based on percentage similarity.
Subsequently, the selected reference proteins together with CPHE1 proteins were aligned using Clustal Omega, a multiple sequence alignment program (Sievers et al., 2011). The used format was ClustalW with character counts (alignment format with base/residue numbering).
Monooxygenase, dioxygenase, and dehydrogenase phylogenetic trees were constructed using the maximum-likelihood method selected from the package MEGA (Molecular Evolutionary Genetic Analysis) version 7 (Kumar et al., 2016). The employed parameters were the following: JTT matrix, gamma distribution of mutation rates with gamma optimized to 2, and the tree robustness was assessed using 1000 bootstrap replicates.
2.2.2 RNA extraction, DNase treatment, cDNA synthesis, and Real-Time PCR (RT-PCR) analysis from S. indicatrix CPHE1
To evaluate the induction of PHE degrading genes, CPHE1 cells were collected by centrifugation (12,000 rpm for 10 min) at different times (0, 1, 3, 7, 14, and 21 days) of growth, after its cultivation in MSM supplemented with 10 mg L−1 PHE. The supernatant was removed, and RNA was extracted from the bacterial pellet by High Pure RNA Isolation Kit (Roche Molecular System, Switzerland). RNA samples from different times were treated first with DNAse, RNase-free (ThermoFisher Scientific, Massachusetts, EE.UU.), using 3U of DNase for 3 μg of RNA. Then complementary DNA (cDNA) synthesis was conducted using RevertAid First Strand cDNA Synthesis kit (ThermoFisher Scientific, Massachusetts, EE.UU.). The total RNA and the random hexamer primers were denatured at 65 C for 5 min. The remaining reagents (Buffer 5x, 20 U μL−1 of RNase Out, 10 mM dNTPs, and 200 U μL−1 retrotranscriptase enzyme) were added and incubated at 25°C for 5 min, 42°C for 60 min and kept at 70°C for 5 min to inactivate the enzyme. cDNAs were amplified with specific primers (Supplementary Table S2) for different PCR cycles depending on the analyzed gene (11–13 for 16s rDNA, and 30 cycles for considered degrading genes). 16S rDNA was used as a constitutive control of gene expression in S. indicatrix CPHE1. All RT-PCR experiments were performed in triplicate.
Specific PCR primers (Supplementary Table S2) for degrading genes were designed in silico using the program Primer3 (primer3 https://primer3.ut.ee/). Designed primers were checked by primer-BLAST tool.
2.2.3 Phenanthrene degrading-microbial characterization of original soils
The total viable PHE degrading-bacteria in the studied soils were enumerated using the unit of measure, colony forming units per Gram of soil (CFU g−1 soil). 1 g of soil was added to 5 mL of MSM, and then 100 µL of the suspension was serially diluted (1:10). Aliquot (100 µL) of the resultant solutions were spread over petri dishes with MSM agar supplemented with 50 mg L−1 of PHE and incubated at 30°C ± 1. The plate count was conducted after 7 days.
2.2.4 Inoculum preparation of S. indicatrix CPHE1 for phenanthrene mineralization in soils
Stenotrophomonas indicatrix CPHE1 grown in LB agar plate was transferred to Luria−Bertani (LB) broth. The culture was incubated under orbital shaking (160 rpm) at 30°C. After 20 h (at the end of the exponential phase), CPHE1 strain was centrifuged and then washed twice using MSM to remove any LB residues. Then, the cell pellet was resuspended in the necessary volume of MSM to obtain a 108 CFU mL−1 density.
2.2.5 Phenanthrene mineralization test in soils
Mineralization studies using 14C-ring-labelled PHE were performed in the five studied soils (PLD, LL, ALC, CR, R) under slurry suspension condition. Tests were performed in triplicate, using respirometers, which consist in a modified 250 mL Erlenmeyers with a soda tramp, containing 1 mL of 0.5 N NaOH. All the microcosm components were sterilized (Matachana steam sterilizer model S100 with one cycle at 120°C, pressure of 101 kPa, for 20 min), except the investigated soil, to preserve their endogenous microbiota. 10 g of each soil were added to Erlenmeyer flasks. Soils were artificially contaminated with a mixture of 14C-PHE (450 Bq per flask) and unlabeled PHE to obtain a total concentration of 50 mg kg−1. 0.5 mL of a 1000 mg L−1 PHE stock solution in methanol, which also contained 14C-PHE (450 Bq), was added to 2.5 g of soil (25% of the total soil) and was stayed under a fume hood for 24 h at room temperature to evaporate the methanol. Subsequently, the remaining soil (7.5 g) was mixed, to avoid damage to soil endogenous microbiota. Finally, 50 mL MSM and 1 mL of SNs were added to Erlenmeyer flask and incubated at 30°C ± 1°C, under continuous slow stirring for 120 days.
Mineralization studies were conducted as follows: (A) Control: soil without treatment; (B) Biostimulation: soil + NS; (C) Bioaugmentation: soil + NS + S. indicatrix CPHE1 (108 CFU g−1); (D) Bioavailability enhancer: soil + NS + HPBCD solution (10 times the initial molar concentration of PHE); (E) Mixing techniques of biostimulation, bioaugmentation and bioavailability enhancer: soil + NS + S. indicatrix CPHE1+ HPBCD. Simultaneously, abiotic controls were set adding 200 mg L−1 of HgCl2 to the soil, to monitor abiotic PHE dissipation.
Samples were periodically taken to monitor the formation of 14CO2 trapped in the alkali trap. NaOH solution from the trap was mixed with 3 mL of a liquid scintillation cocktail (Ready safe from PerkinElmer, Inc., United States). Samples were kept in darkness for 24 h to dissipate the chemiluminescence. A liquid scintillation counter (Beckman Instruments Inc., Fullerton, California, model L55000TD) was used to measure the radioactivity.
The mineralization curves were adjusted to three kinetic models: a simple first-order model (SFO), a biphasic first-order sequential model (Hockey-Stick, HS), and a first-order multi-compartment model (FOMC). These models were selected according to the FOCUS (2006) work group. The Solver tool (included in Microsoft Excel statistical software) and the equations described in Lara-Moreno et al. (2022a) were used, depending on the kinetic model that fits the best to each experimental set of data. Kinetics parameters were obtained from kinetic models: k1, k2, α, β (mineralization rate constants), tb (time at which rate constant changes), and DT50 (time taken for 50% of substance to disappear by dissipation processes). The chi-square (χ2) test was calculated as an indicator of the goodness of fit (χ2 values should be < 15 to mean a good fit).
2.2.6 Phenanthrene availability in soil
NS and HPBCD solutions were used to determine their effect on soil PHE bioavailability. For this purpose, 1 g of soil contaminated with 50 mg kg−1 of PHE was added to corex glass centrifuge tubes. Samples were extracted with 5 mL of NS (MSM + SNs) or NS + HPBCD (10 times the molar concentration of PHE previously added in soil). The corex tubes were agitated on an orbital shaker for 72 h at 20°C ± 1°C and subsequently centrifuged (10 min, 7000 rpm) to separate the supernatant from the soil (Villaverde et al., 2012). The supernatant was filtered through a 0.22 μm Millipore glass fiber membrane. Then, 3 mL of the sample was taken and placed into a glass tube with 2 mL of hexane, which was shaken using a vortex device for 1 min and for 5 min in an ultrasonic bath. The hexane phase was placed in 2.5 mL glass vials. PHE concentration was measured using a gas chromatographer (Agilent GC 6890N) equipped with a mass spectrometer (MS, Agilent MD 5975B). The analytical method was described by Lara-Moreno et al. (2021).
3 Results and discussion
3.1 Bioinformatic analysis of genes involved in phenanthrene degradation pathways
Lara-Moreno et al. (2023) provided relevant information about the genome. The genome of S. indicatrix CPHE1 deposited in NCBI (JANQDV000000000) (Lara-Moreno et al., 2023) consists of 163 contigs, with a size of 4.553.664 bp, 66.1% of Guanine-Cytosine (G-C) content, and 4137 genes described.
Genes potentially associated with PHE biodegradation were bioinformatically predicted in S. indicatrix CPHE1 genome by two diverse strategies: (i) searching for specific annotation of gene products by RAST server and subsequently clustering them into phylogenetic trees using reference sequences; a bibliographic and database search of enzymes of other bacteria has been carried out to identify reference sequences. Data obtained allowed the improvement of the genome automatic annotation of the CPHE1 strain genes. Similarities between the whole set of selected enzymes have been analyzed by Clustal Omega obtaining a phylogenetic analysis. (ii) Analyzing the sequence homology with respect to reference sequences of bacterial strains able to biodegrade PHE (Cébron et al., 2008; Izmalkova et al., 2013) or bacteria frequently detected in the water systems by other studies (Stingley et al., 2004; Elufisan et al., 2020).
Considering the first approach, Supplementary Figure S1 shows the distribution of the annotated genes in different subsystems of S. indicatrix CPHE1 genome. Particular attention was focused on the subsystem of genes that participate in the aromatic metabolism compounds (boxed in red); these annotated genes could take part in the central catabolism of the metabolites formed during the degradation of aromatic compounds. Beta-ketoadipate pathway is an aromatic compound degradation route widely distributed in soil bacteria and fungi. On the one hand, catechol 1,2-dioxygenase converts catechol generated from aromatic hydrocarbons into intermediates of the tricarboxylic acid cycle (TAC). On the other hand, protocatechuic acid is turned into intermediates of the TAC catalyzed by protocatechuate 3,4-dioxygenase. Both enzymes act by cleaving aromatic ring. Catechol-1,2-dioxygenase, has been studied in species belonging to Pseudomonas genus due to its ability to cleave the aromatic ring of catechol molecule (Rodríguez-Salazar et al., 2020). Other annotated genes in the S. indicatrix CPHE1 genome could encode enzymes that participate in the salicylate and gentisate pathway, compounds that have been identified as intermediaries of PAH catabolism (Grund et al., 1992). The salicylic acid pathway has been described for several PHE-degrading bacterial genera or other PAHs, such as Pseudomonas (Lin et al., 2014), Streptomyces (Ishiyama et al., 2004), or Stenotrophomonas (Huang et al., 2013). Several authors have described monooxygenases (Medić et al., 2020) and dioxygenases (Moody et al., 2005; Gao et al., 2013; Kumari et al., 2017) as enzymes responsible for catalyzing the initial reaction of PAHs degradation pathway to produce hydrodiol or dihydrodiol.
For this reason, we initially focused on the study of genes annotated as monooxygenases in the genome of S. indicatrix CPHE1. A phylogenetic analysis was carried out using the proteins reported in Table 1 (Figure 1). The phylogenetic tree demonstrated no similarities between the annotated CPHE1 sequences and the reference monooxygenase (AlkB, UniProtKB: A4XPE8), described in Pseudomonas aeruginosa P6 as responsible for petroleum hydrocarbon degradation (Wang et al., 2019; Medić et al., 2020). Since the other reference sequences were not involved in PAH degradation pathways, it has been ruled out that monooxygenases encode the first step in the PHE degradation.
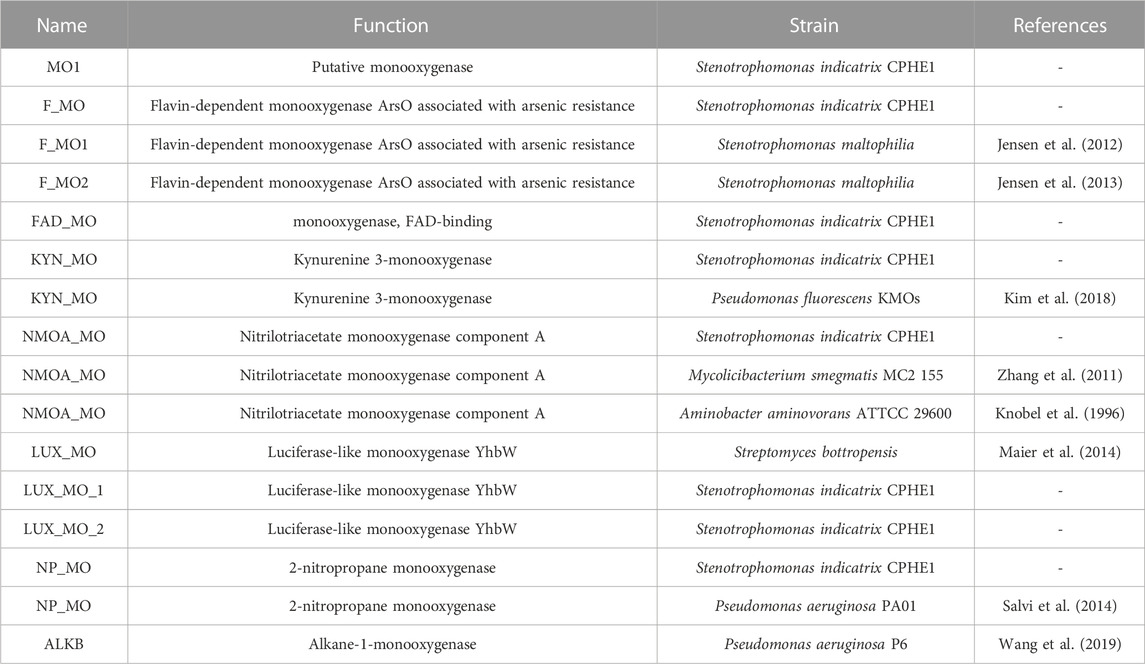
TABLE 1. Monooxygenases annotated in S. indicatrix CPHE1 genome and reference monooxygenases (literature, pdb, NCBI, etc.).
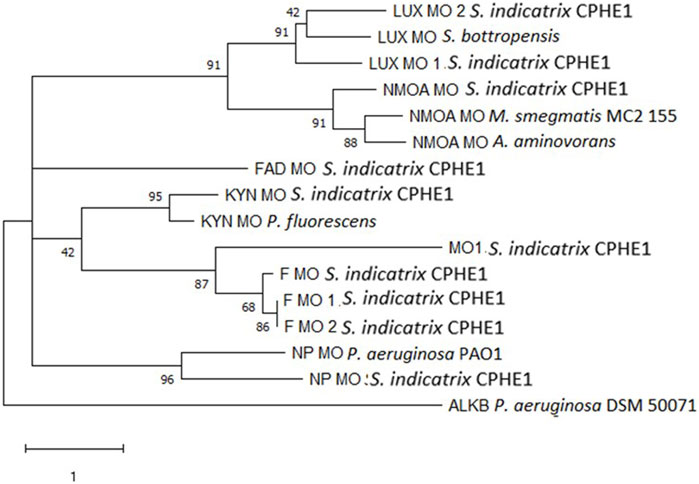
FIGURE 1. Maximum Likelihood tree based on the monooxygenase protein sequences annotated in the genome of S. indicatrix CPHE1 and on the reference monooxygenases involved in the degradation of polycyclic aromatic hydrocarbons. Bootstrap values (>30%) are expressed as percentages of 1000 replicates. Same branches representation was recovered by the neighbor-joining and maximum-parsimony algorithms. The scale bar indicates 1 substitution per nucleotide position in protein sequence tree. Phylogenetic tree was built using MEGA 6.
A similar approach was then undertaken for another enzyme class since the initial reaction of PAH biodegradation in S. indicatrix CPHE1 could be catalyzed by a multicomponent system of a dioxygenase, producing dihydrodiol (Kumari et al., 2017). This hypothesis is supported by two fundamental facts; first, the presence of similar dioxygenases has been detected in several PAHs degrading bacteria such as Pseudomonas (Prabhu and Phale, 2003; Cébron et al., 2008), Mycobacterium (Stingley et al., 2004; Moody et al., 2005), and Stenotrophomonas (Kumari et al., 2017). Second, the metabolite dihydroxyphenanthrene was identified during the PHE biodegradation of S. indicatrix CPHE1 (Lara-Moreno et al., 2021). These data would suggest the intervention of dioxygenase in PHE biodegradation.
For this reason, nine dioxygenases annotated in the genome of S. indicatrix CPHE1 have been exhaustively analyzed and compared with similar dioxygenases of bacterial genomes deposited in several databases. All dioxygenases have been compared by multiple alignments with reference proteins (Table 2), including two dioxygenases known for their involvement in naphthalene biodegradation (NAHAc of P. putida G7 and NIDA of the only naphthalene dioxygenase described in Stenotrophomonas) (Cébron et al., 2008; Kumari et al., 2017). As shown in the tree in Figure 2, the enzyme DO2 is the closest phylogenetically to the reference proteins (NAHAc and NIDA). However, annotated dioxygenases have been considered for the subsequent RT-PCR expression experiments since there are no conclusive results to exclude any of them as putative responsible for deoxygenation.
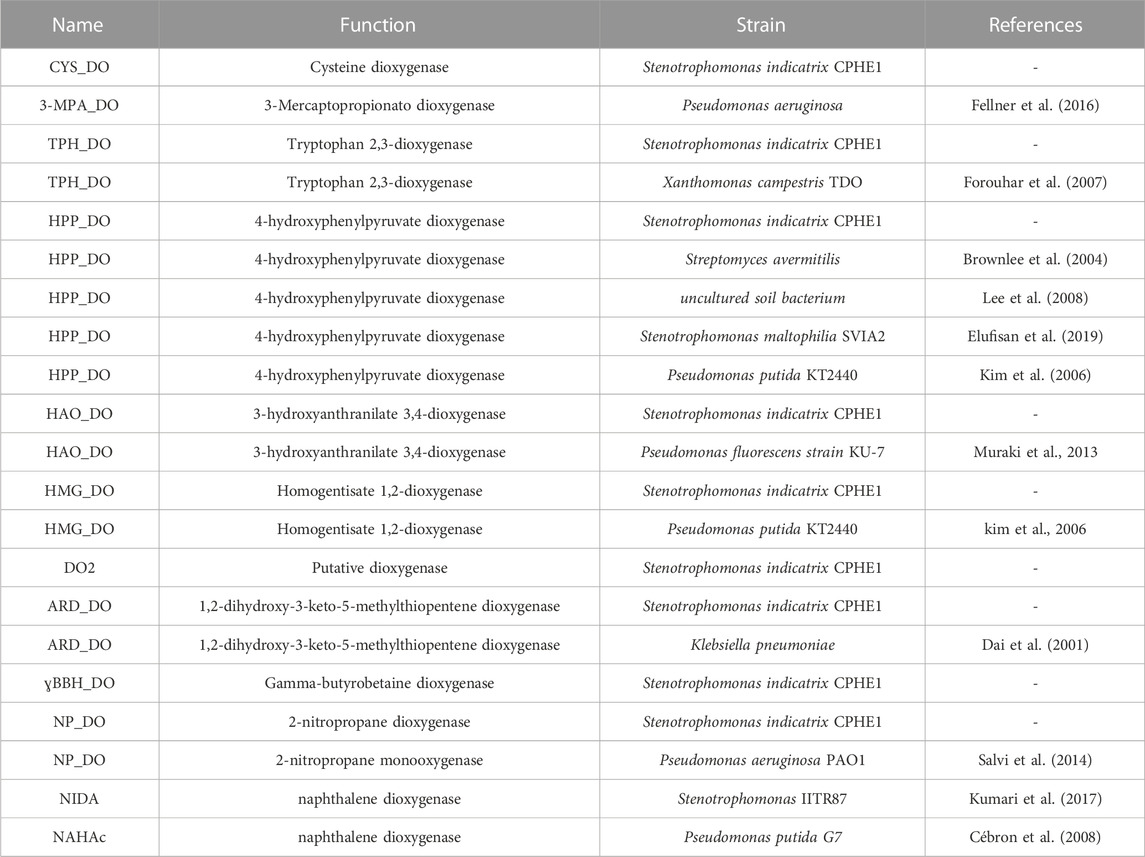
TABLE 2. Dioxygenases annotated in S. indicatrix CPHE1 genome and reference dioxygenases (literature, pdb, NCBI, etc.).
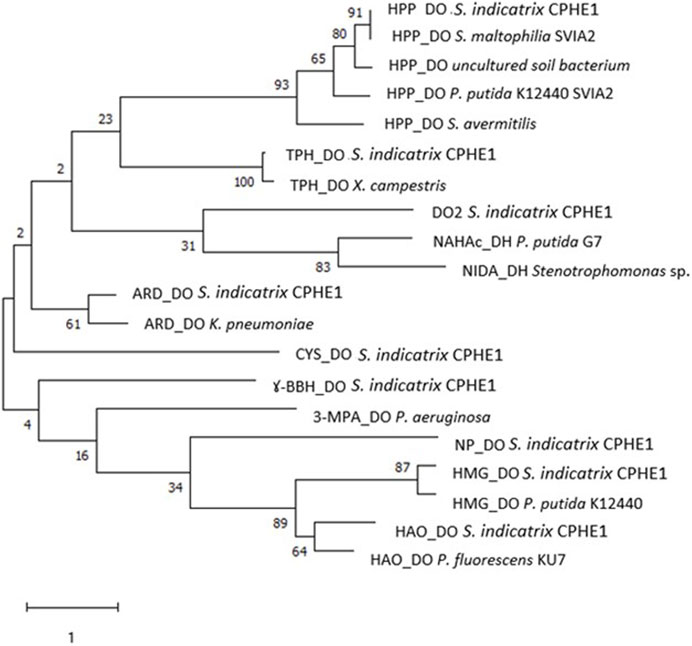
FIGURE 2. Maximum Likelihood tree based on the dioxygenase protein sequences annotated in the genome of S. indicatrix CPHE1 and in the reference dioxygenases involved in the degradation of polycyclic aromatic hydrocarbons. Bootstrap values (>30%) are expressed as percentages of 1000 replicates. Same branches representation was recovered by the neighbour-joining and maximum-parsimony algorithms. The scale bar indicates 1 substitution per nucleotide position in protein sequence tree. Phylogenetic tree was built using MEGA 6.
After the analysis of the possible dioxygenases, a thorough analysis of the annotated dehydrogenases of S. indicatrix CPHE1 was performed, since the dihydrodiol dehydrogenase enzyme catalyzes the second step of the PHE biodegradation route described by Stingley et al. (2004). This enzyme removes two hydrogen atoms from the molecule, oxidizing it, the electrons and protons from the hydrogen atoms are captured by the oxidized form of the coenzyme, which is reduced. Among 119 CPHE1 proteins annotated as dehydrogenases, nine were selected based on their function in relation to the biodegradation of PAHs (Table 3). These amino acid (aa) sequences were analyzed in a phylogenetic tree using diverse dehydrogenase references, including dehydrogenases known as catalysts of the second step of the PAHs biodegradation pathway (NahB, BphB, and NidD). Two different trees were obtained based on the considered aa references (Figure 3); the first tree (Figure 3A) comprises dihydrodiol naphthalene dehydrogenase (NahB) described for P. stutzeri AN10, naphthalene dehydrogenase (NahB) of P. putida AK5, and cis-2,3-dihydrobiphenyl-2,3-diol dehydrogenase (BphB) of P. putida 9816-4. Figure 3B includes naphthalene dehydrogenase (NidD) described in Stenotrophomonas sp. IITR87 which resulted in phylogenetically close to five (out of nine) CPHE1 dehydrogenases.
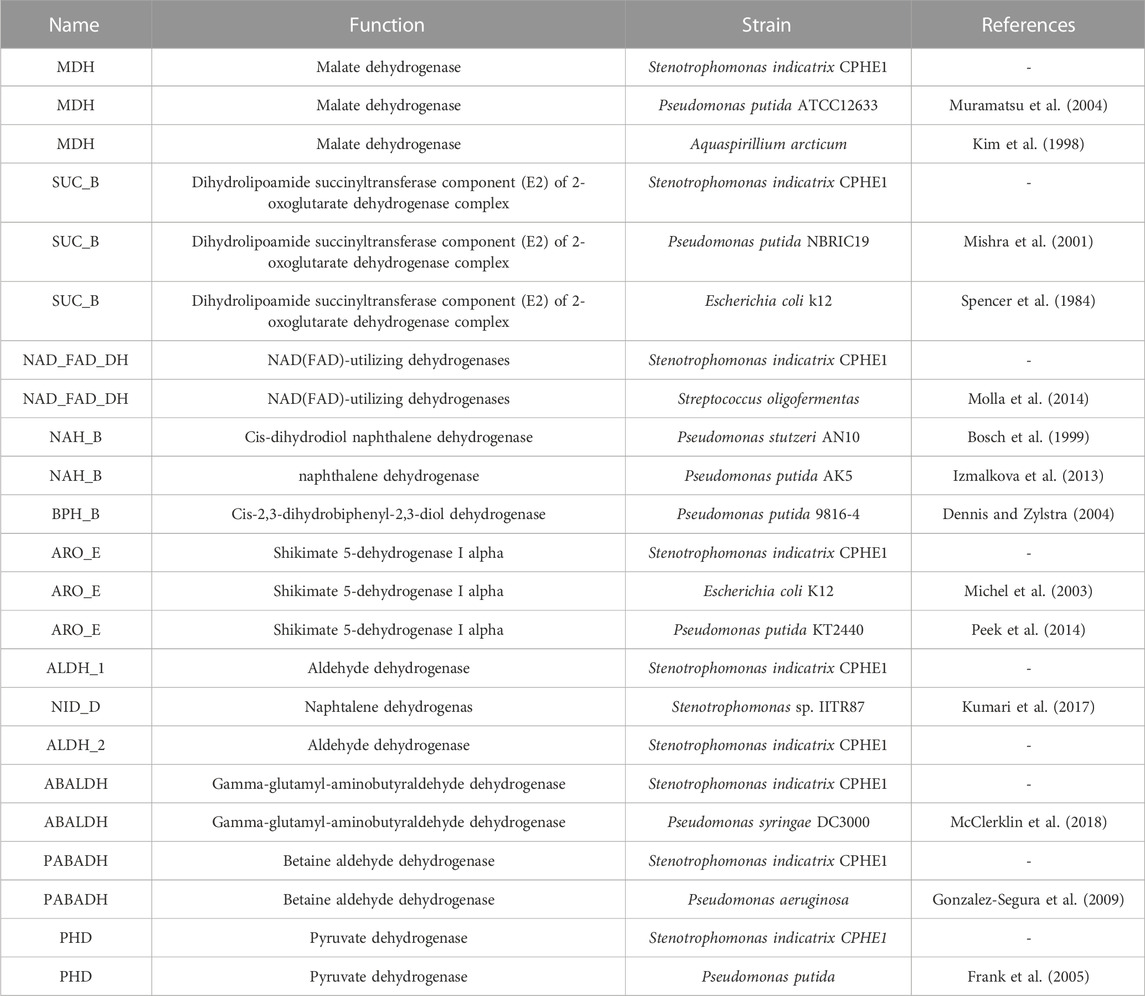
TABLE 3. Dehydrogenases annotated in S. indicatrix CPHE1 genome and reference dehydrogenases (literature, pdb, NCBI, etc.).
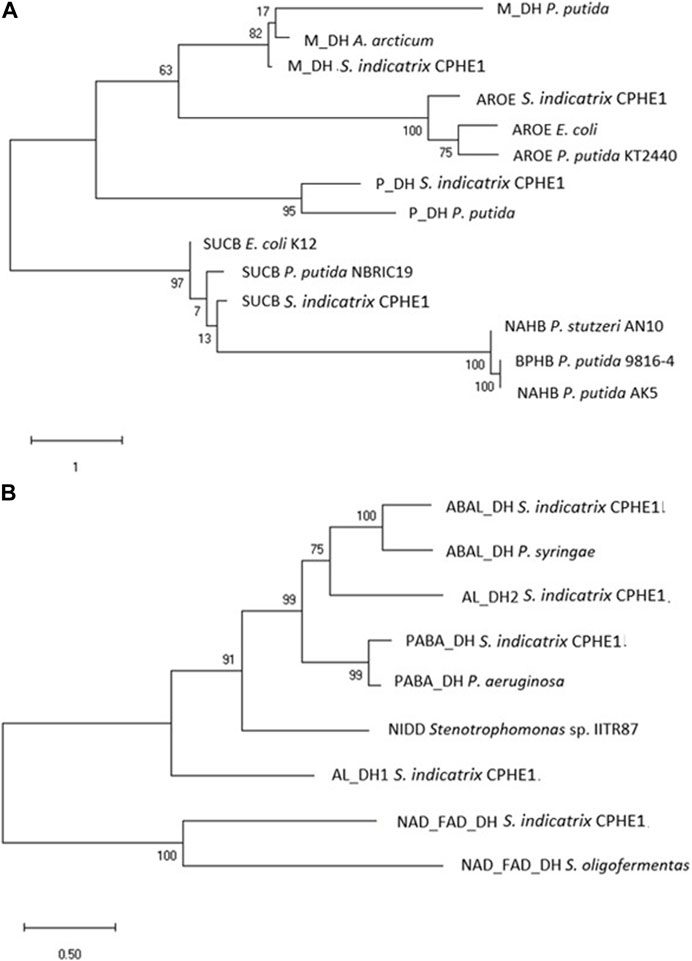
FIGURE 3. Maximum Likelihood tree based on the dehydrogenase protein sequences annotated in the genome of S. indicatrix CPHE1 and reference dehydrogenases NAHB, BPHB, and NIDD (A), and NIDD (B) involved in the degradation of polycyclic aromatic hydrocarbons. Bootstrap values (>30%) are expressed as percentages of 1000 replicates. Same branches representation was recovered by the neighbour-joining and maximum-parsimony algorithms. The scale bar indicates 1 or 0.5 substitution per nucleotide position in protein sequence tree. Phylogenetic tree was built using MEGA 6.
Although PABA DH (betaine aldehyde dehydrogenase) and SUCB (oxoglutarate dehydrogenase dihydrolipoamide succinyltransferase component) were the closest CPHE1 sequences to the respective reference proteins (for each tree), it should be noted that reference proteins belong to different bacterial genera, consistent in a wide protein sequences variability (Keshavarz-Tohid et al., 2019).
3.2 Identification of the involvement of dioxygenases of S. indicatrix CPHE1 in phenanthrene degradation by RT-PCR experiments
Studies of gene expression have been carried out by RT-PCR experiments to know which annotated dioxygenase of S. indicatrix CPHE1 was expressed throughout the PHE biodegradation process after inoculating CPHE1 strain.
Validation of the samples is reported in Supplementary Figure S2 showing the PCR amplification of 16S rDNA gene using genomic DNA (positive control) from S. indicatrix CPHE1, cDNA obtained from RNA extracted from CPHE1 strain without contact with PHE (t0) and RNA samples taken at different times from the PHE biodegradation assay (t1, t3, t7, t14, and t21).
Several authors have used the semi-quantitative RT-PCR to confirm the involvement of genes in the biodegradation pathway of various pollutants. For example, Thanh et al. (2019) observed the expression of dbfA1A2RBC genes that share 99%–100% identity with Paenibacillus sp. YK5 genes (putatively involved in dibenzofuran degradation). The induction of four of these genes was observed after treatment with dibenzofuran through RT-PCR. Di Canito et al. (2018) studied the role of Rhodococcus opacus R7 oxygenases in the o-xylene degradation. The important involvement of akb genes was demonstrated using RNA obtained from R7 cells in the presence of o-xylene by RT-PCR. The result showed that the transcription was induced by the contaminant. In another study, Churchill et al. (2008) checked the ability of Mycobacterium sp. CH-2 to mineralize PHE, pyrene, and FLT. Primers were designed based on the dioxygenases nidAB and pdoA2B2 and an alkane monooxygenase. RT-PCR analysis indicated that alkane monooxygenase is constitutively expressed, however, nidAB, and pdoA2B2 were expressed only in the presence of PAHs.
The results of the CPHE1 dioxygenase gene expression are shown in Figure 4 obtained with specific primers designed on CPHE1 genes reported in Table 2. At the top of the figure are presented the results of the PCR when the 16S rRNA gene was amplified under non-limiting conditions, which serves as a reference gene. Signal intensity quantification (ImageJ.JS) of PCR results indicated that the cDNA of the t0, t1, t3, and t21 samples are the least concentrated, followed by the t14 sample. The t7 sample was twice more concentrated. These data will allow for assessing the expression levels of the rest of the studied genes.
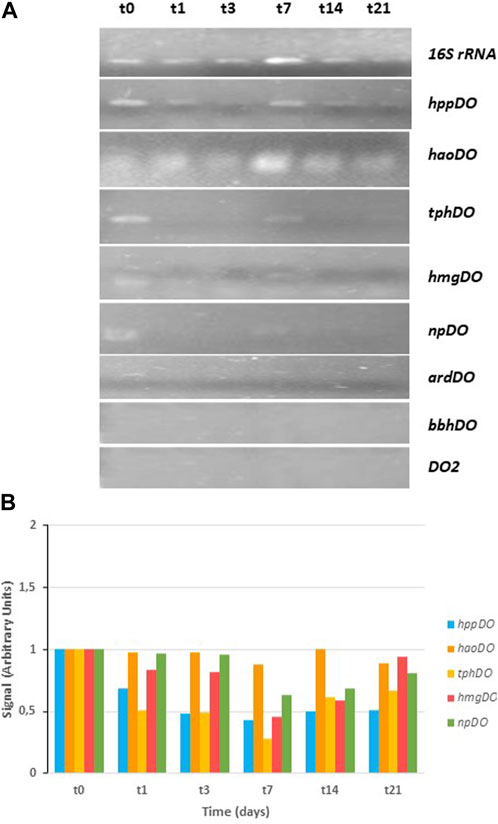
FIGURE 4. RT-PCR analysis of dioxygenase gene expression in S. indicatrix CPHE1. cDNAs were synthesized from RNA at different times (t0, t1, t3, t7, t14, and t21). (A) PCR with specific primers for hppDO, haoDO, tphDO, hmgDO, npDO, ardDO, bbhDO, and DO2 (30 cycles) and 16S rRNA (13 cycles) genes were performed. 16S rRNA expression was used as RNA-loading control for these different samples. (B) Quantification of dioxygenase expression in relation to the 16S rRNA and negative control (t0) signal is shown in the above histograms (in arbitrary units)..
A previous study carried out by Lara-Moreno et al. (2023) showed that the gene annotated as cysteine dioxygenase (cysDO) was expressed in the presence of PHE from the third day of treatment. The KEGG database indicates that the cysDO enzyme is involved in different biodegradation pathways of aromatic compounds, including naphthalene (M00534), salicylic acid (M00638) and phthalic acid (M00623). In addition, Wang et al. (2017) studied the participation of cysDO enzyme in the degradation of aromatic compounds. However, in the currently work, gene expression study has been expanded, all dioxygenases annotated in the genome of CPHE1 strain were analyzed with the aim to know their involvement in PHE biodegradation pathway. The electrophoresis gel obtained is shown in Figure 4. Gene expression of 1,2-dihydroxy-3-keto-5-methylthiopentene dioxygenase (ardDO), gamma-butyrobetaine dioxygenase (bbhDO) or putative dioxygenase (DO2) was not detected. In the case of tryptophan-2,3-dioxygenase (tphDO), homogentisate dioxygenase (hmgDO), 4-hydroxyphenylpyruvate dioxygenase (hppDO), and nitropropan dioxygenase (npDO) genes, a repression of expression was observed in the presence of PHE. And in case of 3-hydroxyanthranilate 3,4-dioxygenase a constituent expression was detected. It concludes that cysDO was the only one expressed in the presence of PHE. These results suggest the involvement of a deoxygenation in the PHE degradation pathway when S. indicatrix CPHE1 was inoculated. In addition, as indicated above, the presence of dihydrodiol metabolites was detected after inoculating CPHE1 strain (Lara-Moreno et al., 2021). These results suggest the involvement of a dioxygenation in the PHE degradation pathway when S. indicatrix CPHE1 was inoculated.
3.3 Phenanthrene degrading-genes expression
Beside the dioxygenase gene expression, the expression of other genes involved in PHE biodegradation route was investigated relying on the second bioinformatic approach based on the comparison of S. indicatrix CPHE1 genes with sequences belonging to bacterial strains known for their involvement in PHAs biodegradation.
Indeed, Stingley et al. (2004) described the most common PHE biodegradation pathways for Mycobacterium vanbaalenii strain PYR-1. These genes were compared to the only two genomic studies (Kumari et al., 2017; Elufisan et al., 2020) that have been published on PHE degradation route in Stenotrophomonas genus. For instance, the genes involved in this degradation pathway showed at least 97% identity compared with Stenotrophomonas IITR87 genome (Kumari et al., 2017).
Thus, CPHE1 genes showing a high identity with respect to the genes described in the M. vanbaalenii PYR-1 genome were taken into consideration as references for the gene expression studies in S. indicatrix CPHE1 (Table 4). In addition, genes described in other bacterial species, reported as PAH-degrading, were included in Table 4 as references, accordingly to the hypothesized metabolic pathway.
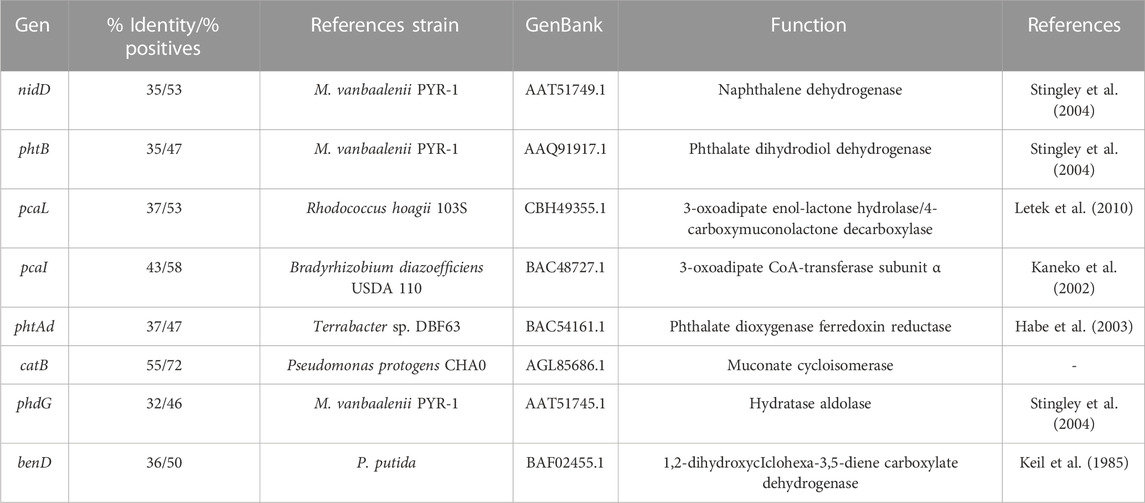
TABLE 4. Reference genes utilized to design the primers for cDNA amplification of S. indicatrix CPHE1 at different times.
Results of their expression in the presence of PHE are shown in Figure 5. The expression of the homologous gene encoding aldolase hydratase (phdG), responsible for transforming 2-hysroxybenzo(h)chromene-2-carboxylic acid to 4-[1-hydroxy(2-naphthyl)]-2-oxobut-3-enoic acid, was induced after 7 days in contact with the contaminant, suggesting its involvement in the PHE degradation pathway carried out by S. indicatrix CPHE1. Usually, in the majority of publications, the gene that encodes the α subunit hydroxylating dioxygenase is identified as a functional marker gene. However, it has been observed that due to low phylogenetic resolution and the lack of specificity, it is derived by erroneous estimation of bacteria that degrade PAHs (Liang et al., 2019). For this reason, Liang et al. (2019) proposed the hydratase-aldolase gene as a biomarker for bacteria involved in PAHs degradation. Therefore, this gene could represent a powerful biomarker to explore the potential of PAH-degrading bacteria in ecosystems, which would have a high impact on the bioremediation of contaminated soils with PAHs. Levieux et al. (2018) and Story et al. (2001) demonstrated that bifunctional enzymes hydratase-aldolase are present in bacterial strains which can use PAHs as the only carbon and energy source, indicating that despite catalyzing an important step in the PAHs degradation, their mechanisms are not very well established.
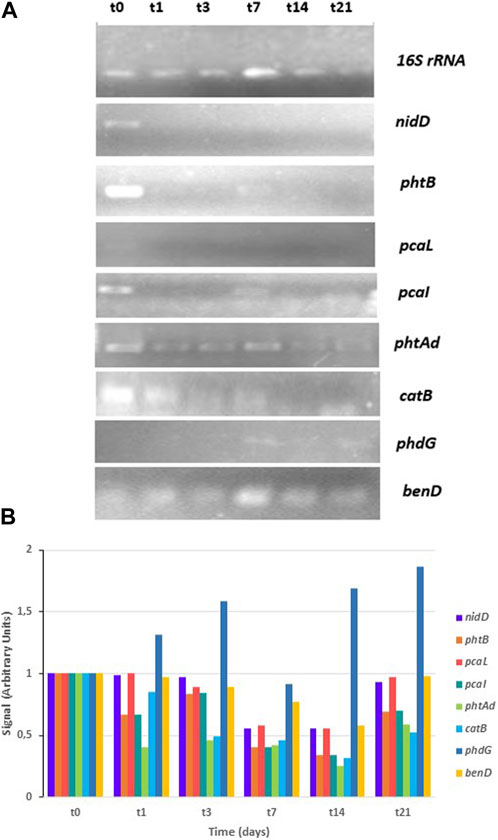
FIGURE 5. RT-PCR analysis of gene expression in S. indicatrix CPHE1. cDNAs were synthesized from RNA at different times (t0, t1, t3, t7, t14, and t21). (A) PCR with specific primers for nidD, phtB, pcaL, pcaI, phtAd, catB, and phdG (30 cycles) and 16S rRNA (13 cycles) genes were performed. 16S rRNA expression was used as RNA-loading control for these different samples. (B) Quantification of studied gene expression in relation to the 16S rRNA and negative control (t0) signal is shown in the above histograms (in arbitrary units).
With the aim of deepening the study of the genes responsible for the degradation of PHE, Stenotrophomonas sp. Pemsol genes were also considered, since Pemsol strain can degrade anthraquinone, naphthalene, PHE, phenanthridine, and xylene (Elufisan et al., 2020). Five genes from CPHE1 were selected due to their high homology with Pemsol genes involved in PHAs biodegradation pathway (Table 5). Figure 6 shows the gene expression at different times: the gene encoding catechol-2,3 dioxygenase was constitutively expressed while the expression of biphenyl-2,3-diol 1,2-dioxygenase (bphC) was induced after 7 days of treatment, the correspondent gene product catalyzes the transformation of 3,4-hydroxyphenanthrene to 2-acid hydroxycenzochromene-2-carboxylic. bphC gene has been also studied in Sphingomonas, and Sphingobium genera which are able to metabolize PHE via both meta- and ortho-cleavege pathways (Waigi et al., 2015; Macchi et al., 2019). Moreover, Yun et al. (2014) concluded that PHE induced a strong regulation of bphC gene, which is involved in the attack to cleavage the aromatic ring in the lower catabolic route of PAHs in Novosphingobium pentaromativoransus species.

TABLE 5. S. indicatrix CPHE1 genes homologous to the genes of Pemsol strain utilized to design primers for cDNA amplification of S. indicatrix CPHE1 at different times.
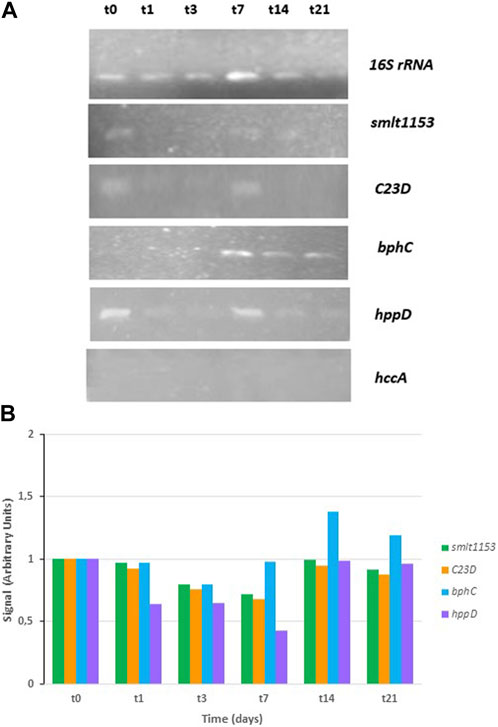
FIGURE 6. RT-PCR analysis in S. indicatrix CPHE1 for the expression study of homologous genes described by Elufisan et al., (2020). cDNAs were synthesized from RNA at different times (t0, t1, t3, t7, t14, and t21). (A) PCR with specific primers for smlt1153, C23D, bphC and hppD (30 cycles) and 16S rRNA (13 cycles) genes were performed. 16S rRNA expression was used as RNA-loading control for these different samples. (B) Studied genes quantification expression in relation to the 16S rRNA and negative control (t0) signal is shown in the above histograms (in arbitrary units).
All these results together revealed that PHE induces the expression of specific genes (cysDO, phdG, and bphC) in S. indicatrix CPHE1 degradation pathway. Figure 7 summarizes the metabolic steps and the time of activation of expressed genes. The gene annotated as cysDO was induced after 3 days of inoculation, coinciding with the point where PHE biodegradation begins to be significant (Lara-Moreno et al., 2021), so this dioxygenase could be responsible for catalyzing the first step of the PHE biodegradation pathway. In addition, the induction of bphC and phdG genes by the presence of PHE from respectively 14 days and 21 days indicates that they could act in intermediate steps as extradiol dioxygenase responsible for the first aromatic ring cleavage and hydratase aldolase responsible for transforming 2-hysroxybenzo(h)chromene-2-carboxylic acid to 4-[1-hydroxy(2-naphthyl)]-2-oxobut-3-enoic acid, respectively.
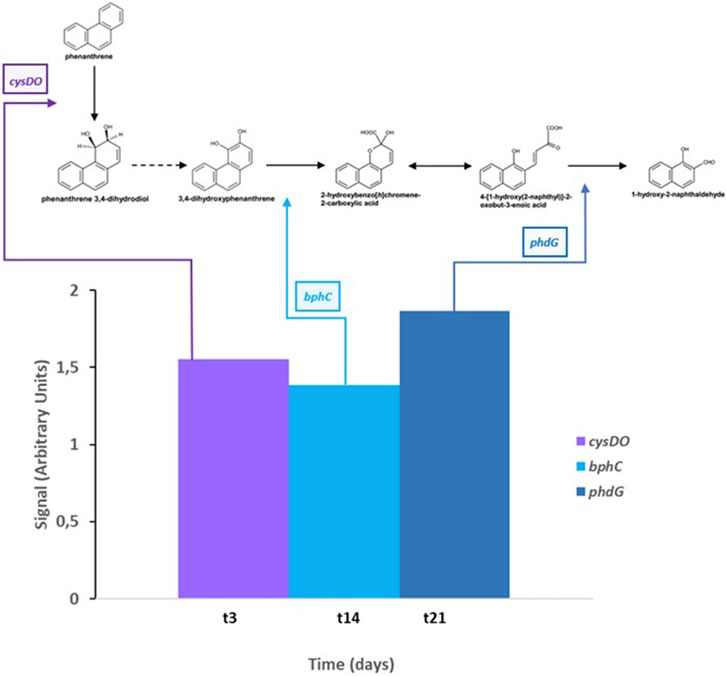
FIGURE 7. Gene expression results of cysDO (cysteine dioxygenase), bphC (biphenyl-2,3-diol 1,2-dioxygenase), and phdG (aldolase hydratase) according to the time of maximum expression of each gene and step of PHE degradation pathway. Dashed arrow indicates a metabolic step not attributable to a determined gene of S. indicatrix CPHE1; double arrow indicates an isomerase reaction.
3.4 Phenanthrene mineralization in contaminated soils. Effect of biostimulation, bioaugmentation, and HPBCD application
PHE abiotic dissipation was assessed in the five selected soils, adding HgCl2 solution to kill the soil endogenous microbiota. The results concluded that abiotic dissipation was not observed in this study (data not shown). Figure 8 and Table 6 show the PHE mineralization curves and kinetic parameters obtained after the application of the different treatments in the investigated soils. An extension of mineralization of 3.6%, 1.7%, 2.3%, 8.7%, and 1.5% after 120 days for PLD, LL, ALC, CR and R soils was respectively observed when the contaminated soils are not subjected to any bioremediation treatment, with DT50 values in the range 1133–6343 days (3–17 years). These results evidenced the need to resort to assisted natural attenuation using biostimulation and bioaugmentation.
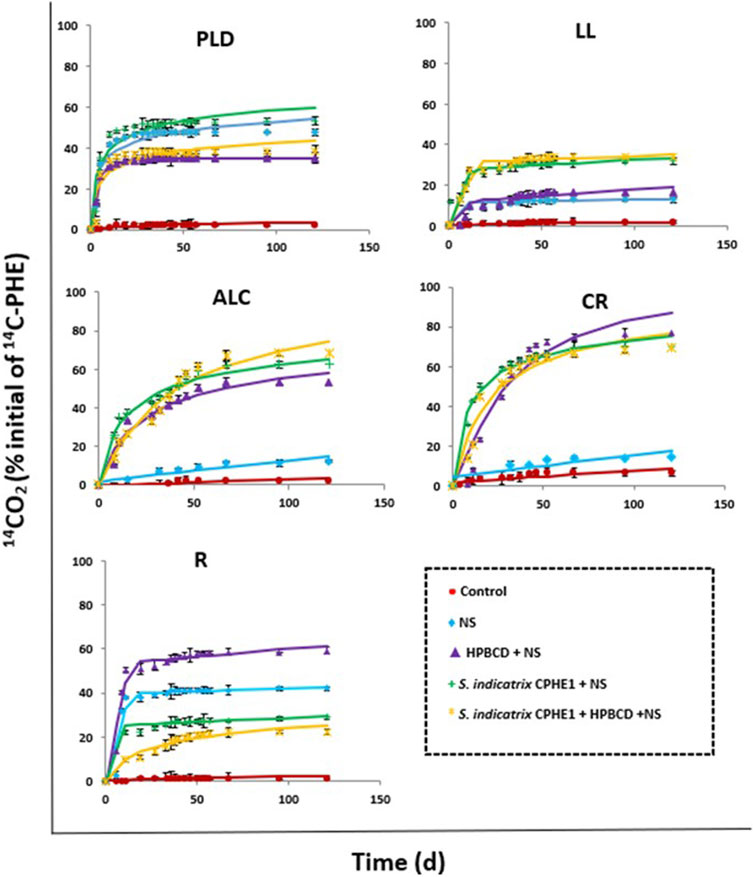
FIGURE 8. Phenanthrene mineralization (120 d) in PLD, LL, ALC, CR, R soils in slurry systems after application of the different treatments studied.
The addition of inorganic nutrients (NS in Figure 8 and treatment B in Table 6) produced a slight activation of the indigenous microbiota of LL, ALC, and CR soils, and therefore PHE mineralization slightly increased (after 120 days 13.1, 12.3, and 17.3%, respectively). On the contrary, its effect was much greater in the cases of PLD and R soils, in which 53.2% and 42.6% of PHE mineralization were achieved, respectively, decreasing DT50 values from 3243 days to only 61.1 d for PLD soil, and from 6343 days to 335 days for R soil. To confirm the presence of PHE-degrading bacterial strains in the studied soils, an enumeration of colony forming units per Gram of soil (CFU g−1 soil) in presence of PHE as the only carbon source was carried out (Supplementary Table S3). The CFU counts were in line with the observed mineralization results when the soil microbiota was stimulated using NS. The microbiota of PLD and R soils turn out to be the most active in PHE mineralization, containing the highest number of CFU g−1 (1.5 × 107 and 8.6 × 107, respectively) of PHE degrading bacteria; CFU g−1 for LL and CR soils were an order of magnitude less than in PLD and R soils, likewise a lower extent of mineralization. ALC soil only reached 12.3% of mineralization and its microbiota was the least rich in potential PHE degraders (1.9 × 105 CFU g−1).
OM influences the control of PAH bioavailability in soils (Abdel-Shafy and Mansour, 2016). It has been demonstrated that the interaction between PAHs and the soil increases to longer aging time, causing a decrease in the bioavailability of the pollutants (Ehlers and Loibner, 2006). To increase their bioavailability, extractants such as cyclodextrins have been used. In a previously published work, Morillo et al. (2012) confirmed the formation of inclusion complexes between HPBCD and PHE which caused an increase in its water solubility (376 times higher in the presence of 100 mM HPBCD). HPBCD is considered suitable for application to contaminated soils since the addition of HPBCD to these soils would cause an increase in the concentration of PHE in the soil solution making it more bioavailable (Duán et al., 2021; Lara-Moreno et al., 2022b). Figure 8 (Treatment C) shows an increase in the percentage of mineralization with respect to treatment with only NS (Treatment B) in all cases except in PLD soil. In ALC, CR, and R soils the extent of mineralization reached up to 58.4%, 87.3%, and 61.3%, respectively (Table 6). In addition, the kinetic processes were accelerated, showing a decrease of DT50 from 2307, 573 and 335 days to 61.6, 26.4, and 12.1 days after treatment, respectively.
Gao et al. (2014) also evaluated the effect of OM on the biodegradation process and the effect of HPBCD to improve PHE bioavailability. The study concluded that there is a significant inverse relationship between the PHE adsorption capacity to the soil and the extracted fraction with HPBCD and PHE biodegradable fraction. Morillo et al. (2014) performed fluorene (FLU) and fluoranthene (FLT) adsorption-desorption in different soils in the presence of HPBCD. The results confirmed that FLU is more resistant to extraction in soils since its initial adsorption to minerals and OM of the soil is greater due to its smaller size in the case of FLT.
PHE extraction experiments from soils have been carried out to know the effect produced by NS solution in comparison to HPBCD on the PHE bioavailability (Figure 9). The OM content is very different in the studied soils. This difference influenced the PHE amounts present in the soil solution when NS was added: 9.6% (CR), 10% (LL), 9.2% (PLD), 2% (R), 0.4% (ALC) (listed in increased order of OM content). PHE concentration in the soil solution was higher in all soils when HPBCD was added: 18.8% (CR), 20.4% (LL), 19.2% (PLD), 3.9% (R), 1.2% (ALC), and the observed increase was due to the formation of complexes between HPBCD, and PAHs as previously observed by Morillo et al. (2014). The results confirmed the inverse relationship between the soil OM content (Supplementary Table S1) and the PHE extracted. However, the determined PHE fraction in solution in the presence of NS or HPBCD was not directly correlated with the observed PHE biodegradation curves and the extent of extraction reached for the different soils, concluding that, PHE degradation depends on other factors apart from the fact that HPBCD is able to increase PHE bioavailability.
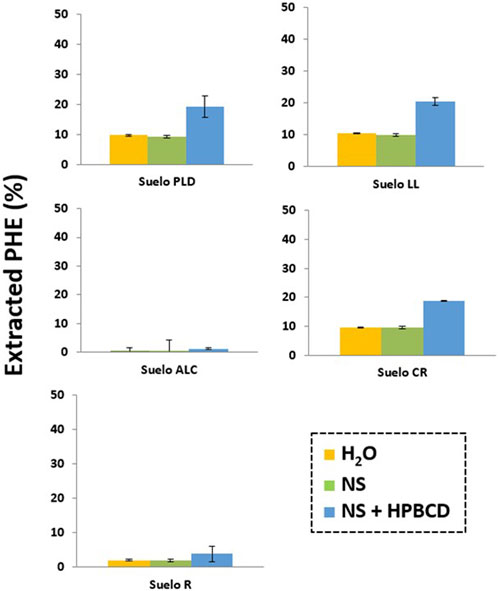
FIGURE 9. PHE extracted from PLD, LL, ALC, CR, R soil in presence of H20, nutrient solution (NS) and HPBCD.
After a thorough analysis of the CPHE1 strain genome and the degrading genes expression, the ability of S. indicatrix CPHE1 to remove PHE was used to improve the bioremediation with a bioaugmentation strategy. When S. indicatrix CPHE1 was inoculated in PLD, LL, ALC, and CR soils, improvements in the global extent of PHE mineralization were observed (59.9%, 33.5%, 65.3%, 75%, respectively), and in the mineralization kinetic parameters, reducing the values of DT50 (31.5, 499, 36.3, and 17.2 days, respectively). The lowest values of DT50 in these 4 soils were obtained when S. indicatrix CPHE1 was inoculated as compared to NS or HPBCD application. Shon et al. (2020) observed that Sphingopyxis soli KIT-001 showed interactions between the bacterial membrane and PAHs exerting a strong influence on biological processes such as metabolic activity and substrate absorption due to changes in membrane lipids, improving the PHE degradation efficiency.
On the contrary, in the case of contaminated R soil inoculated with S. indicatrix CPHE1, a reduction in the extent of mineralization (29.3%) was observed after 120 days, with respect to the degrading capacity shown by the soil microbiota stimulated with NS (42.6%) or the use of HPBCD (61.3%). This fact has been widely observed in the literature because of the competition between the degrading endogenous and exogenous bacteria, which indicates that the presence of S. Indicatrix CPHE1 would have partially disabled PHE degradation carried out by the soil endogenous microbiota, and vice versa (Cycoń et al., 2017; Nwankwegu et al., 2022). The presence of a high density of potential PHE-degrading bacteria in R soil (8.6 × 107 CFU g−1, Supplementary Table S3) would support this statement.
The combined application of S. indicatrix CPHE1 and HPBCD was also tested. The results showed that this co-application clearly increased the extent of PHE mineralization only in soil ALC (with the highest OM content, 13.9%) but the increase was not significant in LL and CR soils, and even a negative effect on the mineralization rate was observed in PLD and R soils in relation to other treatments. Besides, and not less important, DT50 values were higher for all soils when S. indicatrix CPHE1 and HPBCD were co-applicated, indicating that both treatments should not be added together in these soils.
Previously, Stroud et al. (2009) also observed that HPBCD interfered with the microbial mineralization of PHE and hexadecane resulting in lower mineralization extents, but these authors gave no explanation for this fact. Equally, Villaverde et al. (2019) observed that pyrene mineralization in three soils was not increased or even reduced when using HPBCD together with the exogenous bacterial strains Achromobacter xylosoxidans 2BC8 and S. maltophilia JR62. The possibility that the increased concentration of PHE in soil solution due to HPBCD addition could be toxic for S. indicatrix CPHE1 is discarded because Lara-Moreno et al. (2021) demonstrated its capacity to degrade and mineralize 10 mg L−1 PHE in aqueous solution, and its concentration in the soil solution in the present studies is substantially lower. However, since HPBCD could be biodegraded by soil microorganisms (Fenyvesi et al., 2005), the preference of S. indicatrix CPHE1 for HPBCD as a carbon and energy source instead of PHE could slow down the mineralization process (Supplementary Table S4).
Each of the studied soils showed different results after the application of the different treatments, so it is considered important to select a bioremediation strategy based on each specific soil and pollutant properties. LL, PLD, ALC, and CR soils required S. indicatrix CPHE1 inoculation to improve mineralization kinetics, although in the case of CR soil a higher biodegradation profile was observed only applying HPBCD. The most effective strategy for R soil turned out to be the use of HPBCD, which makes more bioavailable PHE in the soil through the formation of an inclusion complex, since its endogenous microbiota showed to be especially active as PHE-degrader.
4 Conclusion
In this work, genome-based analysis and gene expression experiments of S. indicatrix CPHE1 involved in PHE degradation unveiled specific genetic determinants that are induced in the presence of PHE. The cysteine dioxygenase (cysDO) could be responsible for catalyzing the first step of the PHE biodegradation pathway. phdG and bphC genes were induced in the presence of PHE later than cysDO, in accordance with their role in the degradation pathway, since these enzymes act in intermediate steps, while dioxygenase would act at the beginning of the metabolic pathway. Based on gene expression studies, S. indicatrix CPHE1 was selected as a bioaugmentation strategy to bioremediate five soils (PLD, LL, ALC, CR, and R) contaminated with PHE, showing different mineralization results depending on soil properties. It is remarkable that S. indicatrix has never been described as PHE mineralizing bacterium in soils.
Several authors have isolated PHE-degrading bacteria; however, PAHs contamination remains a major global concern, being biostimulation and bioaugmentation the most useful strategies to conduct decontamination of PAHs polluted environment. In soils with an active microbiota to degrade PHE (soils PLD and R), the only addition of NS was sufficient to achieve PHE mineralization. On the contrary, in the cases of LL, ALC, and CR soils, the inoculation of S. indicatrix CPHE1 was crucial to achieving an improvement in PHE mineralization kinetics. HPBCD application provoked an improvement in PHE biodegradation rate in LL, ALC, CR, and R soils, and it was observed that HPBCD was able to achieve better results in the mineralization of PHE in soils with a high OM content. However, the combined application of HPBCD and S. indicatrix CPHE1 evidenced a negative effect presumably due to CPHE1 strain preference for CD instead of PHE as a primary carbon source. In general, selecting the best bioremediation strategy based on the physicochemical and microbial properties of each treated soil has been crucial.
Data availability statement
The sequencing data generated were deposited in the National Center for Biotechnology Information (NCBI), under the BioProject ID PRJNA868539. The whole genome shotgun of S. indicatrix CPHE1 has been deposited in DDBJ/ENA/GenBank under the accession number JANQDV000000000. The version described in this paper is version JANQDV010000000. The raw data was deposited in the Sequence Read Archive (SRA) under the accession number SRR21098193.
Author contributions
AL-M: conceptualization, methodology, investigation, formal analysis, data curation, writing—original draft. FM: methodology, resources, supervision. EM: writing—review and editing, funding acquisition. JZ: investigation, writing—review and editing. PD: supervision, writing—review and editing. JV: methodology, supervision, writing—review and editing.
Funding
This work was supported by the Spanish Ministry of Economy and Competitiveness under the research project CMT 2017-82472-C2-1-R (AEI/FEDER, UE) and by Junta de Andalucía (Council of Economic Transformation, Industry, Knowledge, and University) under the research project PY20_01069 (FEDER Andalucía PO 2014–2020).
Acknowledgments
AL-M acknowledges the Spanish Ministry of Education, Culture and Sports for her FPU fellowship (FPU15/03740), the Erasmus + traineeship program and University of Seville for her Margarita Salas grant funded by the European Union’s Next-Generation EU.
Conflict of interest
The authors declare that the research was conducted in the absence of any commercial or financial relationships that could be construed as a potential conflict of interest.
Publisher’s note
All claims expressed in this article are solely those of the authors and do not necessarily represent those of their affiliated organizations, or those of the publisher, the editors and the reviewers. Any product that may be evaluated in this article, or claim that may be made by its manufacturer, is not guaranteed or endorsed by the publisher.
Supplementary material
The Supplementary Material for this article can be found online at: https://www.frontiersin.org/articles/10.3389/fbioe.2023.1158177/full#supplementary-material
References
Abdel-Shafy, H. I., and Mansour, M. S. M. (2016). A review on polycyclic aromatic hydrocarbons: Source, environmental impact, effect on human health and remediation. Egypt. J. Pet. 25 (1), 107–123. doi:10.1016/j.ejpe.2015.03.011
Adeleke, B. S., Ayangbenro, A. S., and Babalola, O. O. (2021). Genomic assessment of Stenotrophomonas indicatrix for improved sunflower plant. Curr. Genet. 67, 891–907. doi:10.1007/s00294-021-01199-8
Berman, H. M., Westbrook, J., Feng, Z., Gilliland, G., Bhat, T. N., Weissig, H., et al. (2000). The protein Data Bank. Nucleic Acids Res. 8 (1), 235–242. doi:10.1093/nar/28.1.235
Bosch, R., García-Valdés, E., and Moore, E. R. B. (1999). Genetic characterization and evolutionary implications of a chromosomally encoded naphthalene-degradation upper pathway from Pseudomonas stutzeri AN10. Gene 236 (1), 149–157. doi:10.1016/S0378-1119(99)00241-3
Brown, D. M., Okoro, S., van Gils, J., van Spanning, R., Bonte, M., Hutchings, T., et al. (2017). Comparison of landfarming amendments to improve bioremediation of petroleum hydrocarbons in Niger Delta soils. STOTEN 596–597, 284–292. doi:10.1016/j.scitotenv.2017.04.072
Brownlee, M. J., Johnson-Winters, K., Harrison, D. H. T., and Moran, G. R. (2004). Structure of the ferrous form of (4-Hydroxyphenyl)pyruvate dioxygenase from Streptomyces vermitilis in complex with the herapeutic herbicide, NTBC. Biochemistry 43, 6370–6377. doi:10.1021/bi049317s
Cébron, A., Norini, M., Beguiristain, T., and Leyval, C. (2008). Real-Time PCR quantification of PAH-ring hydroxylating dioxygenase (PAH-RHDα) genes from Gram positive and Gram negative bacteria in soil and sediment samples. J. Microbiol. Mater. 73 (2), 148–159. doi:10.1016/j.mimet.2008.01.009
Churchill, P. F., Morgan, A. C., and Kitchens, E. (2008). Characterization of a pyrene-degrading Mycobacterium sp. strain CH-2. J. Environ. Sci. Heal. Part B 43 (8), 698–706. doi:10.1080/03601230802388801
Cycoń, M., Mrozik, A., and Piotrowska-Seget, Z. (2017). Bioaugmentation as a strategy for the remediation of pesticide-polluted soil: A review. Chemosphere 172, 52–71. doi:10.1016/j.chemosphere.2016.12.129
Dai, Y., Pochapsky, T. C., and Abeles, R. H. (2001). Mechanistic studies of two dioxygenases in the methionine salvage pathway of Klebsiella pneumoniae Biochemistry. Biochemistry 40 (21), 6379–6387. doi:10.1021/bi010110y
Dennis, J. J., and Zylstra, G. J. (2004). Complete sequence and genetic organization of pDTG1, the 83 kilobase naphthalene degradation plasmid from Pseudomonas putida strain NCIB 9816-4. J. Mol. Biol. 341 (3), 753–768. doi:10.1016/j.jmb.2004.06.034
Di Canito, A., Zampolli, J., Orro, A., D’Ursi, P., Milanesi, L., Sello, G., et al. (2018). Genome-based analysis for the identification of genes involved in o-xylene degradation in Rhodococcus opacus R7. BMC Genomics 19 (1), 587–617. doi:10.1186/s12864-018-4965-6
Duán, F., Wang, J., Ismail, S., Sung, S., Cui, Z., and Ni, S. (2021). Hydroxypropyl-β-cyclodextrin improves the removal of polycyclic aromatic hydrocarbons by aerobic granular sludge. Environ. Technol. 43, 3262–3268. doi:10.1080/09593330.2021.1921045
Ehlers, G. A. C., and Loibner, A. P. (2006). Linking organic pollutant (bio) availability with geosorbent properties and biomimetic methodology: A review of geosorbent characterisation and (bio) availability prediction. Environ. Pollut. 141 (3), 494–512. doi:10.1016/j.envpol.2005.08.063
Elufisan, T. O., Lozano, L., Bustos, P., Rodríguez-Luna, I. C., Sánchez-Varela, A., Oyedara, O. O., et al. (2019). Complete genome sequence of Stenotrophomonas maltophilia strain SVIA2, isolated from crude oil-contaminated soil in Tabasco, Mexico. Microbiol. Res. Announ. 8, 005299–e619. doi:10.1128/MRA.00529-19
Elufisan, T. O., Rodríguez-Luna, I. C., Oyedara, O. O., Sánchez-Varela, A., Hernández-Mendoza, A., Gonzalez, E. D., et al. (2020). The Polycyclic Aromatic Hydrocarbon (PAH) degradation activities and genome analysis of a novel strain Stenotrophomonas sp. Pemsol isolated from Mexico. PeerJ 8, e8102. doi:10.7717/peerj.8102
EPA (2022). Consolidated list of chemicals subject to the emergency planning and community right-to-know act (EPCRA), comprehensive environmental response, compensation and liability act (CERCLA), and section 112(r) of the clean air act (CAA). Available at: https://www.epa.gov/system/files/documents/2022-12/(Accessed December 21, 2022).
Fellner, M., Aloi, S., Tchesnokov, E. P., Wilbanks, S. M., and Jameson, G. N. L. (2016). Substrate and pH-dependent kinetic profile of 3-Mercaptopropionate Dioxygenase from Pseudomonas aeruginosa. Biochemistry 55, 1362–1371. doi:10.1021/acs.biochem.5b01203
Fenlon, K. A., Andreou, K., Jones, K. C., and Semple, K. T. (2011). The extractability and mineralisation of cypermethrin aged in four UK soils. Chemosphere 82, 187–192. doi:10.1016/j.chemosphere.2010.10.025
Fenyvesi, E., Gruiz, K., Verstichel, S., De Wilde, B., Leitgib, L., Csabai, K., et al. (2005). Biodegradation of cyclodextrins in soil. Chemosphere 60, 1001–1008. doi:10.1016/j.chemosphere.2005.01.026
FOCUS, 2006. Guidance document on estimating persistence and degradation kinetics from environmental fate studies onPesticides in EU registration, report of the FOCUS work group on degradation kinetics. EC documents reference sanco/10058/2005. Available at: https://esdac.jrc.ec.europa.eu/public_path/projects_data/focus/dk/docs/finalreportFOCDegKinetics.pdf.
Forouhar, F., Anderson, J. L. R., Mowat, C. G., Tong, L., Hussain, A., Abashidze, M., et al. (2007). Molecular insights into substrate recognition and catalysis by tryptophan 2,3-dioxygenase. PNAS 104 (2), 473–478. doi:10.1073/pnas.0610007104
Frank, R. A. W., Pratap, J. V., Pei, X. Y., Perham, R. N., and Luisi, B. F. (2005). The molecular origins of specificity in the assembly of a multienzyme complex. Structure 13 (8), 1119–1130. doi:10.1016/j.str.2005.04.021
Friedrich, I., Hollensteiner, J., Scherf, J., Weyergraf, J., Klassen, A., Poehlein, A., et al. (2021). Complete genome sequence of Stenotrophomonas indicatrix DAIF1. Microbiol. Resour. Announc. 10, 014844–e1520. doi:10.1128/MRA.01484-20
Gao, H., Ma, J., Xu, L., and Jia, L. (2014). Hydroxypropyl-β-cyclodextrin extractability and bioavailability of phenanthrene in humin and humic acid fractions from different soils and sediments. Environ. Sci. Pollut. Res. 21 (14), 8620–8630. doi:10.1007/s11356-014-2701-6
Gao, S., Seo, J. S., Wang, J., Keum, Y. S., Li, J., and Li, Q. X. (2013). Multiple degradation pathways of phenanthrene by Stenotrophomonas maltophilia C6. Int. Biodeter. Biodegrad. 79, 98–104. doi:10.1016/j.ibiod.2013.01.012
González-Segura, L., Rudiño-Piñera, E., Muñoz-Clares, R. A., and Horjales, E. (2009). The crystal structure of a ternary complex of betaine aldehyde dehydrogenase from Pseudomonas aeruginosa provides new insight into the reaction mechanism and shows a novel binding mode of the 2'-phosphate of NADP+ and a novel cation binding site. J. Mol. Biol. 385 (2), 542–557. doi:10.1016/j.jmb.2008.10.082
Grund, E., Denecke, B., and Eichenlaub, R. (1992). Naphthalene degradation via salicylate and gentisate by Rhodococcus sp. strain B4. Appl. Environ. Microbiol. 58 (6), 1874–1877. doi:10.1128/aem.58.6.1874-1877.1992
Gu, H., Yan, K., You, Q., Chen, Y., Pan, Y., Wang, H., et al. (2021). Soil indigenous microorganisms weaken the synergy of Massilia sp. WF1 and Phanerochaete chrysosporium in phenanthrene biodegradation. STOTEN 781, 146655. doi:10.1016/j.scitotenv.2021.146655
Habe, H., Miyakoshi, M., Chung, J., Kasuga, K., Yoshida, T., Nojiri, H., et al. (2003). Phthalate catabolic gene cluster is linked to the angular dioxygenase gene in Terrabacter sp. strain DBF63. Appl. Microbiol. Biotech. 61 (1), 44–54. doi:10.1007/s00253-002-1166-6
Huang, Y. W., Hu, R. M., Chu, F. Y., Lin, H. R., and Yang, T. C. (2013). Characterization of a major facilitator superfamily (MFS) tripartite efflux pump emrcabsm from Stenotrophomonas maltophilia. J. Antimicrob. Chemother. 68 (11), 2498–2505. doi:10.1093/jac/dkt250
Humel, S., Schmidt, S. N., Sumetzberger-Hasinger, M., Mayer, P., and Loibner, A. P. (2017). Enhanced accessibility of polycyclic aromatic hydrocarbons (PAHs) and heterocyclic PAHs in industrially contaminated soil after passive dosing of a competitive sorbate. Environ.l Sci. Technol. 51 (14), 8017–8026. doi:10.1021/acs.est.7b01198
Humel, S., Schritter, J., Sumetzberger-Hasinger, M., Ottner, F., Mayer, P., and Loibner, A. P. (2020). Atmospheric carbonation reduces bioaccessibility of PAHs in industrially contaminated soil. J. Hazard. Mat. 383, 121092. doi:10.1016/j.jhazmat.2019.121092
Ishiyama, D., Vujaklija, D., and Davies, J. (2004). Novel pathway of salicylate degradation by Streptomyces sp. strain WA46. Appl. Environ. Microbiol. 70 (3), 1297–1306. doi:10.1128/AEM.70.3.1297-1306.2004
Izmalkova, T. Y., Sazonova, O. I., Nagornih, M. O., Sokolov, S. L., Kosheleva, I. A., and Boronin, A. M. (2013). The organization of naphthalene degradation genes in Pseudomonas putida strain AK5. Res. Microbiol. 164 (3), 244–253. doi:10.1016/j.resmic.2012.12.007
Jensen, C. n., Ali, S. T., Allen, M. J., and Grogan, G. (2013). Mutations of an NAD(P)H-dependent flavoprotein monooxygenase that influence cofactor promiscuity and enantioselectivity. FEBS Open Bio 3, 473–478. doi:10.1016/j.fob.2013.09.008
Jensen, C. N., Cartwright, J., Ward, J., Hart, S., Turkenburg, J. P., Ali, S. T., et al. (2012). A flavoprotein monooxygenase that catalyses a baeyer–villiger reaction and thioether oxidation using NADH as the nicotinamide cofactor. ChemBioChem 13, 872–878. doi:10.1002/cbic.201200006
Kaneko, T., Nakamura, Y., Sato, S., Minamisawa, K., Uchiumi, T., Sasamoto, S., et al. (2002). Complete genomic sequence of nitrogen-fixing symbiotic bacterium Bradyrhizobium japonicum USDA110. DNA Res. 9 (6), 189–197. doi:10.1093/dnares/9.6.189
Keil, H., Keil, S., Pickup, R. W., and Williams, P. A. (1985). Evolutionary conservation of genes coding for meta pathway enzymes within TOL plasmids PWW0 and PWW53. J. Bacteriol. 164 (2), 887–895. doi:10.1128/jb.164.2.887-895.1985
Keshavarz-Tohid, V., Vacheron, J., Dubost, A., Prigent-Combaret, C., Taheri, P., Tarighi, S., et al. (2019). Genomic, phylogenetic and catabolic re-assessment of the Pseudomonas putida clade supports the delineation of Pseudomonas alloputida sp. nov., Pseudomonas inefficax sp. nov., Pseudomonas persica sp. nov., and Pseudomonas shirazica sp. nov. Syst. Appl. Microbiol. 42 (4), 468–480. doi:10.1016/j.syapm.2019.04.004
Kim, H. T., Na, b. k., Chung, J., Kim, S., Kwon, S. K., Cha, H., et al. (2018). Structural basis for inhibitor-induced hydrogen peroxide production by kynurenine 3-monooxygenase. Cell. Chem. Biol. 25, 426–438.e4. doi:10.1016/j.chembiol.2018.01.008
Kim, S. Y., Hwang, K. Y., Kim, S. H., Sung, H. C., Han, Y. S., and Cho, Y. (1998). Sequence, biochemical properties, and crystal structure of malate dehydrogenase from a Psychrophile Aquaspirillium arcticum. J. Biol. Chem. 274 (17), 11761–11767. doi:10.1074/jbc.274.17.11761
Kim, Y. H., Kim, Y. H., Cho, K., Yun, S. H., Kim, J. Y., Kwon, K. H., et al. (2006). Analysis of aromatic catabolic pathways in Pseudomonas putida KT 2440 using a combined proteomic approach: 2-DE/MS and cleavable isotope-coded affinity tag analysis. Proteomics 6, 1301–1318. doi:10.1002/pmic.200500329
Knobel, H. R., Egli, T., and VAn der meer, J. R. (1996). Impact of solids on cloning and characterization of the genes encoding nitrilotriacetate monooxygenase of Chelatobacter heintzii ATCC 29600. J. Bacteriol. 178 (21), 6123–6132. doi:10.1128/jb.178.21.6123-6132.1996
Kumar, S., Stecher, G., and Tamura, K. (2016). MEGA7: Molecular evolutionary genetics analysis version 7.0 for bigger datasets. Mol. Biol. Evol. 33 (7), 1870–1874. doi:10.1093/molbev/msw054
Kumari, S., Regar, R. K., Bajaj, A., Ch, R., Satyanarayana, G. N. V., Mudiam, M. K. R., et al. (2017). Simultaneous biodegradation of polyaromatic hydrocarbons by a Stenotrophomonas sp: Characterization of nid genes and effect of surfactants on degradation. Indian J. Microbiol. 57 (1), 60–67. doi:10.1007/s12088-016-0612-6
Lara-Moreno, A., Morillo, E., Merchán, F., González-Pimentel, J. L., and Villaverde, J. (2023). Genome sequence of Stenotrophomonas indicatrix CPHE1, a powerful phenanthrene-degrading bacterium. 3 Biotech. 13, 53. doi:10.1007/s13205-023-03469-3
Lara-Moreno, A., Morillo, E., Merchán, F., Madrid, F., and Villaverde, J. (2022b). Bioremediation of a trifluralin contaminated soil using bioaugmentation with novel isolated bacterial strains and cyclodextrin. STOTEN 840, 156695. doi:10.1016/j.scitotenv.2022.156695
Lara-Moreno, A., Morillo, E., Merchán, F., Madrid, F., and Villaverde, J. (2022a). Chlorpyrifos removal in an artificially contaminated soil using novel bacterial strains and cyclodextrin. Evaluation of its effectiveness by ecotoxicity studies. Agronomy 12, 1971. doi:10.3390/agronomy12081971
Lara-Moreno, A., Morillo, E., Merchán, F., and Villaverde, J. (2021). A comprehensive feasibility study of effectiveness and environmental impact of PAH bioremediation using an indigenous microbial degrader consortium and a novel strain Stenotrophomonas maltophilia CPHE1 isolated from an industrial polluted soil. J. Environ. Manag. 289, 112512. doi:10.1016/j.jenvman.2021.112512
Lee, C. M., Yeo, Y. S., Lee, J. H., Kim, S. J., Kim, J. B., Han, N. S., et al. (2008). Identification of a novel 4-hydroxyphenylpyruvate dioxygenase from the soil metagenome. Biochem. Biophys. Res. Commun. 370, 322–326. doi:10.1016/j.bbrc.2008.03.102
Letek, M., González, P., Macarthur, I., Rodríguez, H., Freeman, T. C., Valero-Rello, A., et al. (2010). The genome of a pathogenic Rhodococcus: Cooptive virulence underpinned by key gene acquisitions. PLoS Genet. 6 (9), e1001145. doi:10.1371/journal.pgen.1001145
Levieux, J. A., Medellin, B., Johnson, W. H., Erwin, K., Li, W., Johnson, I. A., et al. (2018). Structural characterization of the Hydratase-Aldolases, NahE and PhdJ: Implications for the specificity, catalysis, and N-acetylneuraminate lyase subgroup of the aldolase superfamily. Biochemistry 57 (25), 3524–3536. doi:10.1021/acs.biochem.8b00532
Liang, C., Huang, Y., and Wang, H. (2019). pahE, a functional marker gene for polycyclic aromatic hydrocarbon-degrading bacteria. Appl. Environ. Microbiol. 85 (3), e02399. doi:10.1128/AEM.02399-18
Lin, M., Hu, X., Chen, W., Wang, H., and Wang, C. (2014). Biodegradation of phenanthrene by Pseudomonas sp: BZ-3, isolated from crude oil contaminated soil. Int. Biodeter. Biodegrad. 94, 176–181. doi:10.1016/j.ibiod.2014.07.011
Liu, T., Ding, K., Guo, G., Yang, F., and Wang, L. (2018). Effects of hydroxypropyl-β-cyclodextrin on pyrene and benzo[α]pyrene: Bioavailability and degradation in sotil. Chem. Ecol. 34 (6), 519–531. doi:10.1080/02757540.2018.1462343
Lozano-Andrade, C. N., Strube, M. L., and Kovács, Á. T. (2021). Complete genome sequences of four soil-derived isolates for studying synthetic bacterial community assembly. Microbiol. Resour. Announc. 10, 008488–e921. doi:10.1128/MRA.00848-21
Macchi, M., Festa, S., Vega-Vela, N. E., Morelli, I. S., and Coppotelli, B. M. (2019). Assessing interactions, predicting function, and increasing degradation potential of a PAH-degrading bacterial consortium by effect of an inoculant strain. Environ. Sci. Pollut. Res. 26 (25), 25932–25944. doi:10.1007/s11356-019-05760-4
Madrid, F., Florido, M. C., Rubio-Bellido, M., Villaverde, J., and Morillo, E. (2022). Dissipation of a mix of priority PAHs in soils by using availability enhancers. Effect of aging and pollutant interactions. Sci. Total. Environ. 837, 155744. doi:10.1016/j.scitotenv.2022.155744
Madrid, F., Rubio-Bellido, M., Villaverde, J., Peña, A., and Morillo, E. (2019). Natural and assisted dissipation of polycyclic aromatic hydrocarbons in a long-term co-contaminated soil with creosote and potentially toxic elements. Sci. Total. Environ. 660, 705–714. doi:10.1016/j.scitotenv.2018.12.376
Maier, S., Pflüger, T., Loesgen, S., Asmus, K., Brötz, E., Paululat, T., et al. (2014). Insights into the bioactivity of mensacarcin and epoxide formation by MsnO8. ChemBioChem 15, 749–756. doi:10.1002/cbic.201300704
Mandree, P., Masika, W., Naicker, J., Moonsamy, G., Ramchuran, S., and Lalloo, R. (2021). Bioremediation of polycyclic aromatic hydrocarbons from industry contaminated soil using indigenous Bacillus spp. Processes 9 (9), 1606. doi:10.3390/pr9091606
Masy, T., Demanèche, S., Tromme, O., Thonart, P., Jacques, P., Hiligsmann, S., et al. (2016). Hydrocarbon biostimulation and bioaugmentation in organic carbon and clay-rich soils. Soil Biol. biochem. 99, 66–74. doi:10.1016/j.soilbio.2016.04.016
McClerklin, S. A., Lee, S. G., Harper, C. P., Nwumeh, R., Jez, J. M., and Kunkel, B. N. (2018). Indole-3-acetaldehyde dehydrogenase-dependent auxin synthesis contributes to virulence of Pseudomonas syringae strain DC3000. PLoS Pathog. 14 (1), e1006811. doi:10.1371/journal.ppat.1006811
Medić, A., Lješević, M., Inui, H., Beškoski, V., Kojić, I., Stojanović, K., et al. (2020). Efficient biodegradation of petroleum: N-Alkanes and polycyclic aromatic hydrocarbons by polyextremophilic Pseudomonas aeruginosa sanai with multidegradative capacity. RSC Adv. 10 (24), 14060–14070. doi:10.1039/c9ra10371f
Michel, G., Roszak, A. W., Sauvé, V., Maclean, J., Matte, A., Coggins, J. R., et al. (2003). Structures of shikimate dehydrogenase AroE and its paralog YdiB. J. Biol. Chem. 278 (21), 19463–19472. doi:10.1074/jbc.M300794200
Mishra, S., Mishra, A., Chauhan, P. S., Mishra, S. K., Kumari, M., Niranjan, A., et al. (2001). Pseudomonas putida NBRIC19 dihydrolipoamide succinyltransferase (SucB) gene controls degradation of toxic allelochemicals produced by Parthenium hysterophorus. J. Appl. Microbiol. 112, 793–808. doi:10.1111/j.1365-2672.2012.05256.x
Molla, G., Nardini, M., Motta, P., D'Arrigo, P., Panzeri, W., and Pollegioni, L. (2014). Aminoacetone oxidase from Streptococcus oligofermentans belongs to a new three-domain family of bacterial flavoproteins. Biochem. J. 464, 387–399. doi:10.1042/bj20140972
Moody, J. D., Freeman, J. P., and Cerniglia, C. E. (2005). Degradation of benz[a]anthracene by Mycobacterium vanbaalenii strain PYR-1. Biodegradation 16 (6), 513–526. doi:10.1007/s10532-004-7217-1
Morillo, E., Madrid, F., Lara-Moreno, A., and Villaverde, J. (2020). Soil bioremediation by cyclodextrins. a review. Int. J. Pharm. 591, 119943. doi:10.1016/j.ijpharm.2020.119943
Morillo, E., Sanchez-Trujillo, M. A., Moyano, J. R., Villaverde, J., Gomez-Pantoja, M. E., and Perez-Martinez, J. I. (2012). Enhanced solubilisation of six PAHs by three synthetic cyclodextrins for remediation applications: Molecular modelling of the inclusion complexes. PLoS ONE 7, e44137. doi:10.1371/journal.pone.0044137
Morillo, E., Villaverde, J., Madrid, F., and Undabeytia, T. (2014). Effect of contact time and the use of hydroxypropyl- β -cyclodextrin in the removal of fluorene and fluoranthene from contaminated soils. STOTEN 496, 144–154. doi:10.1016/j.scitotenv.2014.07.027
Mrozik, A., and Piotrowska-Seget, Z. (2010). Bioaugmentation as a strategy for cleaning up of soils contaminated with aromatic compounds. Microbiol. Res. 165 (5), 363–375. doi:10.1016/j.micres.2009.08.001
Muramatsu, H., Mihara, H., Kakutani, R., Yasuda, M., Ueda, M., Kurihara, T., et al. (2004). The putative malate/lactate dehydrogenase from Pseudomonas putida is an NADPH-dependent 1-Piperideine-2-carboxylate/1-Pyrroline-2-carboxylate reductase involved in the catabolism of D-Lysine and D-Proline. J. Biol. Chem. 280 (7), 5329–5335. doi:10.1074/jbc.M411918200
Nwankwegu, A. S., Zhang, L., Xie, D., Onwosi, C. O., Muhammad, W. I., Odoh, C. K., et al. (2022). Bioaugmentation as a green technology for hydrocarbon pollution remediation. Problems and prospects. J. Environ. Manag. 304, 114313. doi:10.1016/j.jenvman.2021.114313
Parab, V., and Phadke, M. (2020). Co-biodegradation studies of naphthalene and phenanthrene using bacterial consortium. J. Environ. Sci. Health - Part A Toxic/Hazardous Subst. Environ. Eng. 55 (7), 912–924. doi:10.1080/10934529.2020.1754054
Peek, J., Shi, T., and Christendat, D. (2014). Identification of novel polyphenolic inhibitors of shikimate dehydrogenase (AroE). J. Biomol. Screen. 19 (7), 1090–1098. doi:10.1177/1087057114527127
Prabhu, Y., and Phale, P. S. (2003). Biodegradation of phenanthrene by Pseudomonas sp. strain PP2: Novel metabolic pathway, role of biosurfactant and cell surface hydrophobicity in hydrocarbon assimilation. Appl. Microbiol. Biotech. 61 (4), 342–351. doi:10.1007/s00253-002-1218-y
Rodríguez-Salazar, J., Almeida-Juarez, A. G., Ornelas-Ocampo, K., Millán-López, S., Raga-Carbajal, E., Rodríguez-Mejía, J. L., et al. (2020). Characterization of a novel functional trimeric catechol 1,2-dioxygenase from a Pseudomonas stutzeri isolated from the Gulf of Mexico. Front. Microbiol. 11, 1100–1117. doi:10.3389/fmicb.2020.01100
Salvi, F., Agniswamy, J., Yuan, H., Vercammen, K., Pelicaen, R., Cornelis, P., et al. (2014). The combined structural and kinetic characterization of a bacterial nitronate monooxygenase from Pseudomonas aeruginosa PAO1 establishes NMO Class I and II. J. Biol. Chem. 289 (34), 23764–23775. doi:10.1074/jbc.M114.577791
Shon, J. C., Noh, Y. J., Kwon, Y. S., Kim, J. H., Wu, Z., and Seo, J. S. (2020). The impact of phenanthrene on membrane phospholipids and its biodegradation by Sphingopyxis soli. Ecotoxicol. Environ. Saf. 192, 110254. doi:10.1016/j.ecoenv.2020.110254
Sievers, F., Wilm, A., Dineen, D., Gibson, T. J., Karplus, K., Li, W., et al. (2011). Fast, scalable generation of high-quality protein multiple sequence alignments using Clustal Omega. Mol. Syst. Biol. 7, 539. doi:10.1038/msb.2011.75
Spencer, M. E., Stephens, P. E., Duckenfield, I. K., and Guest, J. R. (1984). Nucleotide sequence of the sucB gene encoding the dihydrolipoamide succinyltransferase of Escherichia coli K12 and homology with the corresponding acetyltransferase. Eur. J. Biochem. 141, 361–374. doi:10.1111/j.1432-1033.1984.tb08200.x
Stingley, R. L., Khan, A. A., and Cerniglia, C. E. (2004). Molecular characterization of a phenanthrene degradation pathway in Mycobacterium vanbaalenii PYR-1. Biochem. Biophys. Res. Commun. 322, 133–146. doi:10.1016/j.bbrc.2004.07.089
Story, S. P., Parker, S. H., Hayasaka, S. S., Riley, M. B., and Kline, E. L. (2001). Convergent and divergent points in catabolic pathways involved in utilization of fluoranthene, naphthalene, anthracene, and phenanthrene by Sphingomonas paucimobilis var. EPA505. J. Ind. Microbiol. Biotechnol. 26 (6), 369–382. doi:10.1038/sj.jim.7000149
Stroud, J. L., Tzima, M., Paton, G. I., and Semple, K. T. (2009). Influence of hydroxypropyl-β-cyclodextrin on the biodegradation of 14C-phenanthrene and 14C-hexadecane in soil. Environ. Pollut. 157 (10), 2678–2683. doi:10.1016/j.envpol.2009.05.009
Sun, M., Ye, M., Hu, F., Li, H., Teng, Y., Luo, Y., et al. (2014). Tenax extraction for exploring rate-limiting factors in methyl-β-cyclodextrin enhanced anaerobic biodegradation of PAHs under denitrifying conditions in a red paddy soil. J. Hazard. Mat. 264, 505–513. doi:10.1016/j.jhazmat.2013.10.032
Thanh, L. T. H., Thi, T. V. N., Shintani, M., Moriuchi, R., Dohra, H., Loc, N. H., et al. (2019). Isolation and characterization of a moderate thermophilic Paenibacillus naphthalenovorans strain 4B1 capable of degrading dibenzofuran from dioxin-contaminated soil in Vietnam. J. Biosci. Bioeng. 128 (5), 571–577. doi:10.1016/j.jbiosc.2019.05.006
Tyagi, M., da Fonseca, M. M. R., and de Carvalho, C. C. C. R. (2011). Bioaugmentation and biostimulation strategies to improve the effectiveness of bioremediation processes. Biodegradation 22 (2), 231–241. doi:10.1007/s10532-010-9394-4
Varjani, S. J., and Upasani, V. N. (2017). A new look on factors affecting microbial degradation of petroleum hydrocarbon pollutants. Int. Biodeterior. Biodegrad. 120, 71–83. doi:10.1016/j.ibiod.2017.02.006
Villaverde, J., Láiz, L., Lara-Moreno, A., González-Pimentel, J. L., and Morillo, E. (2019). Bioaugmentation of PAH-contaminated soils with novel specific degrader strains isolated from a contaminated industrial site. Effect of hydroxypropyl-β-cyclodextrin as PAH bioavailability enhancer. Front. Microbiol. 10, 2588. doi:10.3389/fmicb.2019.02588
Villaverde, J., Posada-Baquero, R., Rubio-Bellido, M., Laiz, L., Saiz-Jimenez, C., Sanchez-Trujillo, M. A., et al. (2012). Enhanced mineralization of diuron using a cyclodextrin-based bioremediation technology. J. Agric. Food Chem. 60, 9941–9947. doi:10.1021/jf3021909
Waigi, M. G., Kang, F., Goikavi, C., Ling, W., and Gao, Y. (2015). Phenanthrene biodegradation by sphingomonads and its application in the contaminated soils and sediments: A review. Int. Biodet. Biodegrad. 104, 333–349. doi:10.1016/j.ibiod.2015.06.008
Wang, J. di, Li, X. X., and Qu, C. T. (2019). A global proteomic change in petroleum hydrocarbon-degrading Pseudomonas aeruginosa in response to high and low concentrations of petroleum hydrocarbons. Curr. Microbiol. 76 (11), 1270–1277. doi:10.1007/s00284-019-01754-0
Wang, Y., Li, J., and Liu, A. (2017). Oxygen activation by mononuclear nonheme iron dioxygenases involved in the degradation of aromatics. J. Biol. Inorg. Chem. 22 (2-3), 395–405. doi:10.1007/s00775-017-1436-5
Wang, Y., Nie, M., Diwu, Z., Chang, F., Nie, H., Zhang, B., et al. (2021). Toxicity evaluation of the metabolites derived from the degradation of phenanthrene by one of a soil ubiquitous PAHs-degrading strain Rhodococcus qingshengii FF. J. Hazard. Mat. 415, 125657. doi:10.1016/j.jhazmat.2021.125657
Weber, M., Schünemann, W., Fuß, J., Kämpfer, P., and Lipski, A. (2018). Stenotrophomonas lactitubi sp. nov. and Stenotrophomonas indicatrix sp. nov., isolated from surfaces with food contact. Int. J. Syst. Evol. Microbiol. 68, 1830–1838. doi:10.1099/ijsem.0.002732
Xiao, Y., Jiang, R., Wu, X., Zhong, Q., Li, Y., and Wang, H. (2021). Comparative genomic analysis of Stenotrophomonas maltophilia strain W18 reveals its adaptative genomic features for degrading polycyclic aromatic hydrocarbons. Microbiol. Spect. 9 (3), e0142021. doi:10.1128/Spectrum.01420-21
Yun, S. H., Choi, C. H., Lee, S. Y., Lee, Y. G., Kwon, J., Leem, S. H., et al. (2014). Proteomic characterization of plasmid pLA1 for biodegradation of polycyclic aromatic hydrocarbons in the marine bacterium, Novosphingobium pentaromativoransus 6-1. PLoS ONE 9 (3), e90812. doi:10.1371/journal.pone.0090812
Zabbey, N., Sam, K., and Onyebuchi, A. T. (2017). Remediation of contaminated lands in the Niger Delta, Nigeria: Prospects and challenges. STOTEN 586, 952–965. doi:10.1016/j.scitotenv.2017.02.075
Zafra, G., Absalón, Á. E., Cuevas, M. D. C., and Cortés-Espinosa, D. V. (2014). Isolation and selection of a highly tolerant microbial consortium with potential for PAH biodegradation from heavy crude oil-contaminated soils. Water Air Soil Pollut. 225 (2), 1826. doi:10.1007/s11270-013-1826-4
Keywords: phenanthrene, Stenotrophomonas indicatrix CPHE1, mineralization, phenanthrene biodegradation pathway, genes expression
Citation: Lara-Moreno A, Merchán F, Morillo E, Zampolli J, Di Gennaro P and Villaverde J (2023) Genome analysis for the identification of genes involved in phenanthrene biodegradation pathway in Stenotrophomonas indicatrix CPHE1. Phenanthrene mineralization in soils assisted by integrated approaches. Front. Bioeng. Biotechnol. 11:1158177. doi: 10.3389/fbioe.2023.1158177
Received: 03 February 2023; Accepted: 14 April 2023;
Published: 04 May 2023.
Edited by:
Datta Madamwar, Charotar University of Science and Technology, IndiaReviewed by:
Inna Solyanikova, Belgorod National Research University, RussiaQing Hong, Nanjing Agricultural University, China
Copyright © 2023 Lara-Moreno, Merchán, Morillo, Zampolli, Di Gennaro and Villaverde. This is an open-access article distributed under the terms of the Creative Commons Attribution License (CC BY). The use, distribution or reproduction in other forums is permitted, provided the original author(s) and the copyright owner(s) are credited and that the original publication in this journal is cited, in accordance with accepted academic practice. No use, distribution or reproduction is permitted which does not comply with these terms.
*Correspondence: Alba Lara-Moreno, YWxhcmE5QHVzLmVz